- 1School of Energy and Materials, Shanghai Key Laboratory of Engineering Materials Application and Evaluation, Shanghai Polytechnic University, Shanghai, China
- 2Shanghai Thermophysical Properties Big Data Professional Technical Service Platform, Shanghai Engineering Research Center of Advanced Thermal Functional Materials, Shanghai, China
MXene is a novel two-dimensional layered nanomaterial with a very large specific surface area and abundant surface functional groups, endowing it with unique physical and chemical properties. MXene can be compounded with other functional materials to significantly improve the performance of MXene composites or broaden their application scope. Meanwhile, with the development of flexible composite preparation technology, it has promoted the continuous expansion of its application fields. The introduction and combination of different materials can improve the performance of flexible composites and make them have a broader application prospect. In recent years, researchers have started preparing MXene materials as flexible composites for applications such as supercapacitors, sensors, electromagnetic shielding and thermal management. This paper gives a brief introduction to flexible composites and MXene materials, reviews the applications of MXene based flexible composites in various fields as well as the research progress, and provides an outlook on their future development direction.
1 Introduction
MXene-based flexible composites, comprising a flexible substrate integrated with MXene layers, exhibit significant flexibility and plasticity compared to traditional rigid MXene materials, thus broadening their application scope. MXenes, two-dimensional transition metal carbides, nitrides, or carbonitrides, possess a unique synergy of electrical, thermal, and mechanical properties. Their high electrical conductivity, attributable to their metallic nature, makes them ideal for applications requiring efficient charge transport. Their commendable thermal conductivity aids in effective heat management in electronic devices, while their inherent strength and elasticity contribute to the durability and flexibility of the composites (Yan et al., 2015). In the energy sector, MXene-based flexible composites have been extensively researched and employed in electrochemical capacitors and lithium-ion batteries. The superior electrical conductivity and ion transport capabilities of MXenes significantly enhance the energy density and power density of electrochemical capacitors. The rapid electron transfer facilitated by high conductivity, combined with the layered structure that promotes efficient ion intercalation and deintercalation, optimizes the performance of these capacitors. For lithium-ion batteries, MXene composites offer exceptional cycling stability and high specific capacity, making them suitable for electrode material development (Liu et al., 2021). The mechanical flexibility of these composites maintains structural integrity throughout charge-discharge cycles, thereby prolonging battery lifespan. In environmental applications, MXene-based flexible composites serve as effective adsorbents due to their high specific surface area and tailored surface chemistry (Peng and Yan, 2022). These properties enable the adsorption of various pollutants, including heavy metal ions, organic compounds, and radionuclides. The large surface area ensures extensive interaction with contaminants, while the adjustable surface chemistry can be optimized for specific pollutant targets. The capability of MXene composites to alter their surface chemistry via simple ion exchange processes further enhances their potential in environmental pollution remediation, making them versatile in diverse conditions and pollution types (Li et al., 2018). In the biomedical field, MXene-based flexible composites offer numerous potential applications such as tissue engineering scaffolds, drug delivery systems, and biosensors (Han et al., 2020). Their excellent electrical conductivity can stimulate cell growth in tissue engineering, while their high surface area and customizable chemistry are ideal for loading and releasing therapeutic agents in drug delivery. Additionally, the biocompatibility and mechanical flexibility of MXenes support their use in developing flexible and wearable biosensors for real-time health monitoring. Beyond these fields, MXene-based flexible composites show promise in applications such as sensors, flexible electronics, and catalysis (Yao et al., 2016). They can be employed to fabricate flexible electronic devices, including transistors and wearable gadgets, as well as highly sensitive and selective sensors. The high electrical conductivity and flexibility of MXenes ensure the reliability and durability of flexible electronics. Furthermore, MXene composites can be utilized to create efficient catalysts for environmental applications, such as the treatment of hazardous gases and liquid waste (Ahmadpoor and Sharma, 2017). The large surface area and active sites on MXenes enhance catalytic activity, making them effective in various catalytic processes for environmental remediation.
MXene based flexible composites can be prepared in a variety of ways. Among them, the most common method involves dispersing MXene material in a suitable solvent and then coating it onto a flexible substrate. Due to the layered structure of the MXene material, it is possible to form composites with a multilayer structure. This multilayer structure can be adjusted by controlling the concentration of MXene material and the number of coatings (Miao et al., 2019). In addition, MXene materials can be combined with other nanomaterials (e.g., carbon nanotubes and silica nanoparticles) to form composites to enhance their properties (Naguib et al., 2014). Based on this paper, we firstly summarize the properties and preparation of MXene, and the preparation of flexible composites, and then review the latest research progress of MXene based flexible composites from supercapacitor, sensor and electromagnetic shielding, and summarize the application of MXene based flexible composites, and finally outlook the future development trend. Finally, the future development trend of MXene based flexible composites is summarized, in order to produce some guidance for the future research of MXene based flexible composites.
2 MXene based flexible composite
2.1 Introduction to flexible composites
Flexible composites are a new class of materials that have gained a lot of attention recently because of their distinctive qualities and prospective uses. The majority of the time, these materials are composed of two or more different elements, such as polymers, metals, ceramics, and nanoparticles, which are combined in a controlled manner to achieve a desired set of properties (Jiang et al., 2019a). The resulting composites exhibit a combination of properties that the individual components do not possess, such as high strength, toughness, flexibility and electrical conductivity and enhanced heat and mass transfer capabilities. One of the main advantages of flexible composites is their ability to be adapted to specific applications (Sankaran et al., 2018). For example, in aerospace, these materials can be made to have great strength and stiffness while also being lightweight and efficient in heat dissipation, making them excellent for aircraft construction. In electronics, flexible composites can be designed with excellent electrical conductivity and thermal stability, making them suitable for use in flexible electronic devicflexible composites offer a wide range of uses beyond the specific applications listed above including antimicrobial treatment for environmentales, such as sensors, displays, and batteries. In the biomedical field, flexible composites can be used to make implantable devices that are biocompatible, mechanically robust, and can interact with biological systems in a controlled manner (Zeng et al., 2015).
The fabrication of flexible composites typically involves a combination of top-down and bottom-up methods. Top-down methods involve manipulation and assembly of pre-existing materials, such as patterning of graphene sheets or etching of metal foils. Bottom-up approaches, on the other hand, involve the creation of novel materials with precise qualities by the self-assembly of molecules or nanoparticles. Combining these approaches allows the creation of materials with a diverse range of qualities and uses.
Recent developments in nanotechnology and materials science have fueled the creation of flexible composites. For instance, the development of materials with extraordinary mechanical, electrical, and thermal properties has been made possible by the use of nanoscale fillers like carbon nanotubes, graphene, and metal nanoparticles (Lu et al., 2016). The development of new polymers and composites has also led to the creation of biocompatible, environmentally friendly and easy-to-process materials.
In summary, flexible composites are a promising type of substances with a vast vary of functions in aerospace, electronics and biomedical fields. Their capacity to be tailor-made to precise applications, coupled with their unique properties, make them an attractive option for a variety of industries (Shen et al., 2016). The continued development of these materials will require advances in nanotechnology, materials science and manufacturing processes, and is likely to lead to new breakthroughs and applications in the coming years.
2.2 Introduction and synthesis of MXene materials
2.2.1 Properties of MXene materials
Since the first generation of MXene (Ti3C2Tx) was introduced in 2011 (Naguib et al., 2011) More than 30 types of MXene have been studied and reported, including Ti3C2Tx, Ti2CTx, V2CTx, Nb2CTx, Ti3CNTx, (Ti0.5Nb)0.52CTx, Nb4C3Tx, Ta4C3Tx, etc. (Halim et al., 2016). However, there is a wide variety of MXene materials with different types of structures and properties, so understanding the primary structure and synthesis of MXene and its surface functional groups is necessary for specific flexible sensor applications. The synthesis of MXene is commonly accomplished with the aid of etching the A-layer of the MAX section of the precursor, with A representing the component in IIIA or IVA. The chemical method of MXene is M n+1XnTx (n = 1,2,3), the place M represents transition metals, such as Ti, Mo, Cr, Nb, V, etc.; X represents carbon or nitrogen atoms; Tx represents a number of floor practical groups, such as -OH, -O, -F, etc. (Deng et al., 2019). The crystal structure of MXene is inherited from MAX phase, which is a hexagonal crystal structure (Rajavel et al., 2020). MXene consists of countless layers of hexagonal units, the place the “M” layer atoms and the “X” layer atoms alternate in the order M/X/M---M/X/M, with the outermost layer continually being the “M” layer atoms. The “M” layer atoms are organized in a compact hexagonal shape and the “X” layer atoms occupy octahedral positions. The arrangement of the MXene atoms changes due to the change in the stoichiometric number n in the chemical formula, with the M2X type MXene being hexagonally tightly arranged and M3X2 type and M4X3 type MXene are cubic tightly arranged. Since the coordination variety of transition metallic ions is normally 6, the “M” layer atoms in MXene shape 6 chemical bonds with the adjacent “X” layer atoms and chemical useful agencies and connect to the surface, forming MXene with surface useful team Tx (Boota et al., 2017). These surprisingly tunable surface purposeful businesses are one of the points that distinguish MXene from different 2D substances such as graphene and transition metallic sulfides. MXene materials are normally acquired with the aid of selective etching of crew A factors (usually Al or Ga) in the precursor cloth MAX. Since MX bonds are more advantageous than MA bonds, the greater chemically lively team A factors can be eliminated with etchants to maintain the layered shape of Mn+1Xn (Hieu et al., 2021) (Figure 1) MXene has excellent electrical/thermal conductivity, good bending resistance and rigidity, large specific surface area, light weight and easy processing properties, all of which make MXene materials can be widely used, and there are also many polar groups on the surface of MXene, which makes it very compatible with other substances with polar groups, which further makes the MXene material’s the various properties of MXene materials have been improved.
2.2.2 Synthesis of MXene materials
The top-down method and the bottom-up strategy are the two main methods used to synthesize MXene. The top-down approach requires that the MAX phase be etched in order to prepare MXene. In this approach, a MAX phase is selected as the raw material and then a strong acid (usually hydrofluoric acid) is used to etch the MAX phase to remove the A-layer from the MAX phase to obtain MXene (Malaki and Varma, 2020). For example, for the synthesis of MXene from Ti3AlC2, the Ti3AlC2 powder can be soaked in a 50 wt% HF solution for 2 h to selectively etch away the Al elemental layer in the MAX precursor. The resulting suspension is then washed several times with deionized water and centrifuged to obtain relaxed accordion-like Ti3C2, but to avoid the safety risks associated with the use of HF acids in the synthesis of MXene, scientists have developed non-HF acid synthesis routes. These routes include the use of LiF/HCl or LiF + NaF/HCl solutions (Ghidiu et al., 2014). The use of these alternative solutions can effectively reduce the hazards and risks during the synthesis of MXene (Anasori et al., 2017). However, this method may be difficult to achieve uniform etching and consistent quality of MXene flakes, which may affect the material properties. Thus further control of etching conditions (e.g., concentration, temperature and time) needs to be enhanced and a standardised protocol can improve the reproducibility and quality of MXene synthesis.
In addition to top-down approaches, some researchers have also tried to prepare MXene materials using bottom-up methods. These methods include atomic layer deposition and chemical vapor deposition (CVD) techniques. An example is the method of preparing Ti3AlC2 using DC magnetron sputtering to deposit titanium, aluminum and carbon elements on an insulating sapphire substrate. Ti3C2 films with a size of 1 cm × 1 cm can be obtained by selective etching of the aluminum layer. Although bottom-up methods are costly and have low yields, some researchers are still exploring the feasibility of these methods (Kajiyama et al., 2017). This method requires complex equipment and precise control of deposition parameters, which makes them less accessible and more difficult to implement. There is also a need to continue to simplify synthesis protocols and improve the reliability of deposition equipment, which could make these methods more practical and widely available.
In recent years, some researchers have also tried to use non-HF acids to prepare MXene, such as NH4F aqueous solution etching method, high temperature molten salt or alkali etching method. Non-HF etchants are generally less toxic and pose fewer health risks to researchers. For instance, NH₄F is less hazardous than HF, reducing the potential for severe chemical burns and inhalation risks. These methods produce less hazardous waste, making waste management easier and less detrimental to the environment, thereby reducing the ecological footprint of MXene production. The by-products of non-HF etching methods are often easier to neutralize and dispose of safely; for example, the waste from NH₄F etching can be treated with standard chemical neutralization techniques. While some non-HF methods, such as molten salt etching, may require high temperatures, potentially impacting energy efficiency, the trade-off is a safer and potentially more sustainable process (Anasori et al., 2015).
In general, there is still much room to explore the synthesis methods of MXene, especially the exploration of low-cost, green and high-yield MXene material preparation methods is still a great challenge.
2.3 Preparation of MXene based flexible composites
MXene based flexible composites are usually prepared by blending MXene nanosheets with flexible polymers. Among them, the polymers can be various polymeric materials (Han et al., 2017) such as polyvinyl alcohol (PVA), polystyrene (PS), polyethylene (PE), etc. (Yu et al., 2019).The polymers can also be polymers with functional groups, such as fluoropolymers, silicon-containing polymers, etc. (Cao Y. et al., 2017). In the preparation process, the weight ratio of MXene to polymer, mixing method, mixing time and other conditions need to be controlled (Wu et al., 2016) in order to ensure the uniformity of MXene dispersion in the polymer and the performance of the composite (Sajid et al., 2017).
MXene based flexible composites are usually prepared by solution spin coating, spraying, dip coating and flexible template methods (Liu Y. et al., 2016). Among them, the solution spin coating method is one of the most commonly used preparation methods. In this method, the preparation process includes preparing a solution of MXene and polymer, spin-coating the MXene/polymer mixture on the flexible substrate, drying and curing. In the spin coating process, conditions such as spin coating speed, spin coating time, and coating concentration need to be controlled to obtain a uniform and dense MXene based flexible composite film (Jiao et al., 2019). Spray coating method is also a commonly used preparation method. This method uses a special nozzle to spray the MXene and polymer mixture onto the flexible substrate, followed by drying and curing (Cao X. et al., 2017). Compared with the spin coating method, the spray method has higher preparation efficiency and lower cost, but the quality of the film may be somewhat affected due to the particle size and uniformity in the coating process (Shao et al., 2019). Another common preparation method is the dip-coating method, in which the flexible substrate is immersed in a solution of MXene and polymer mixture, followed by drying and curing (Sun et al., 2017). This method has high preparation efficiency and is suitable for preparing large-area MXene based flexible composite films, but the quality and thickness of the films are susceptible to difficulties in control (Wu et al., 2019). The flexible template method is a relatively new preparation method in which a flexible template is used to mold the MXene and polymer blend, followed by drying and curing (Wei et al., 2019). This technique has the benefits of excessive controllability and reproducibility, and is appropriate for the coaching of MXene based totally flexible composites with regular microstructures (Sheng et al., 2019). In conclusion, there are many techniques for the practise of MXene based totally flexible composites, and a suitable preparation method wishes to be chosen in accordance to the specific utility eventualities and requirements. At the same time, the process parameters need to be controlled during the preparation process to obtain MXene-based flexible composites with good performance. Hollow structure that has a decrea
MXene based flexible composites also need to undergo subsequent treatment steps, such as heat treatment, compression treatment, surface modification, etc. to further improve their performance (Fei et al., 2017). For example, heat treatment can assist MXene nanosheets form stronger interactions with every different and extend the mechanical electricity and electrical conductivity of the films (Mirkhani et al., 2019) the surface modification can introduce new useful agencies and enlarge the performance of MXene based flexible composites (Hu et al., 2017).
In general, the preparation of MXene based flexible composites includes the preparation of MXene, preparation of polymers, preparation of mixed solutions, preparation of flexible substrates, preparation of solution spin coating or other coating methods, drying, curing, and subsequent processing steps. The preparation process requires precise control of various parameters to obtain the desired MXene based flexible composites. These materials have a wide range of promising applications and can be used to prepare high-performance flexible sensors, supercapacitors, thermoelectric materials and many other electronic devices.
3 Application of MXene based flexible composites
3.1 Supercapacitor
Wearable electronics require flexible energy storage devices to power them, including foldable phones, smart watches, etc. To achieve this goal, many scientists and engineers are working on creating flexible and wearable power storage devices, which encompass supercapacitors and batteries (such as lithium and sodium batteries) MXene is a promising cloth for electricity storage in wearable units due to its amazing electrical conductivity (Yan et al., 2017), high specific surface area, abundant electrochemical active sites and unique morphological geometry. In this paper, we present MXene based wearable supercapacitors with high power density, stable charge/discharge cycle performance and good safety (Wang et al., 2020).
Specific capacitance is a crucial parameter in the evaluation of supercapacitors, representing the amount of electric charge stored per unit mass or per unit area of the electrode material. The importance of specific capacitance in the context of supercapacitor performance lies in several aspects. Firstly, it is directly related to the energy density, indicating a material’s ability to store charge and thus leading to higher energy densities for applications requiring prolonged energy supply, such as portable electronics and electric vehicles. Secondly, specific capacitance affects the power density, with higher values allowing for greater power output, essential for applications needing quick energy bursts like regenerative braking systems and power backup systems. Additionally, higher specific capacitance contributes to improved efficiency in energy storage and retrieval, minimizing energy losses and maximizing performance and longevity. Lastly, it indicates the effective utilization of the electrode material for charge storage, which is critical for developing compact and lightweight energy storage devices. MXene materials can be used as electrodes for supercapacitors due to the abundance of surface functional groups, high electrical conductivity, and stable layer structure, which are properties that have a significant effect on the enhancement of specific capacitance. But there are certain drawbacks to pure MXene, including weak mechanical properties, ease of re-stacking, modest lateral dimensions, and poor stability in oxidizing environments (Li et al., 2021). As a result, numerous modification techniques have been created to successfully enhance the performance of MXenes.
MXene nanosheets can be combined with low-dimensional substances including graphene, carbon nanotubes, carbon nanofibers, and polymers to efficiently inhibit interlayer repacking and improve interlayer space (Aslam et al., 2021). Li et al. (2023) constructed a flexible sandwich-shaped MXene/CNTs@Ni film. CNTs@Ni’s interlayer spacing effectively stabilized MXene nanosheets. Using the improved MXene/CNTs@Ni thin film electrode, a binder-free self-supported electrode with good mechanical stability and electrochemical properties was produced. The specific capacitance can be as high as 990.8 F cm−3, which is 1.4 and 2.4 times more capacitance than a pure MXene thin film electrode and an MXene/CNTs thin film electrode, respectively. Flexible symmetrical supercapacitors (FSMS) based on MXene/CNTs@Ni films were developed, demonstrating their potential usage in portable energy technologies with a high energy density of 14.5 Wh kg−1, a high power density of 2,571.4 W kg−1, and good cycling stability. The addition of CNTs@Ni offers redox active sites, accelerating the transport of electrons between the electrode and electrolyte and enhancing the electrochemical performance of MXene nanosheets. In order to create composites based on MXene for flexible electrode materials and energy storage applications, the study offers a potential method. Zhang P. et al. (2023) proposed a method for carbon dot intercalation that involved producing flexible MXene thin-film electrodes with a high density and large ion-accessible active surfaces by charring calcium alginate (CA) gel within MXene nanosheets. High density MXene/CA films were produced through the production of CA hydrogel within MXene nanosheets and evaporative drying. To widen the interlayer space and encourage electrolytic diffusion inside the MXene films, CA-derived carbon dots may be incorporated into the MXene nanosheets during the carbonization process. High filling density and wide layer spacing in the developed MXene/CAC films allow for outstanding capacitance-capacitance performance. The MXene/CAC films provide high weight and capacity of 372.6 F g−1 and 1,244.6 F cm−3, respectively, at 1 Ag−1 capacitance and still maintain 198.3 F g−1 and 662.5 F cm−3 at an ultra-high current density of 1,000 Ag−1. In addition, the MXene/CAC film has good cycling stability with 93.5% capacitance retention after 30,000 cycles. Even under high load, MXene/CAC films exhibit high capacity capacitance and high area capacitance. When assembled into an ASSC, the MXene/CAC film has a high bulk energy density of 27.2 wh L−1 and a maximum bulk power density of 13.1 kW L−1.
Changing the surface chemistry and structure of MXene can also effectively improve its performance in energy storage devices, such as introducing functional groups on the MXene surface or compounding with other compounds to change its surface chemistry and structure; and also controlling the specific surface area and pore size structure by adjusting the number of layers and morphology of MXene. These modifications can effectively improve the capacitance performance, cycling stability, reaction rate and other capabilities of MXene electrodes. For example, Ma et al. (2022) used changes in the structural design of the electrode, the surface chemistry, and optimized the fabrication process. Self-supported, binder-free and flexible Ti3C2Tx MXene electrodes with highly improved capacitance, rate capability and cycling stability were prepared. The designed and prepared P-MXene/CPAQ-A electrodes exhibit extremely high capacitance performance, as well as excellent rate performance and cycling stability. Its capacitance reached 532.9 F g−1 at 5 mV s−1. The capacitance retention was 97.1% after 40,000 cycles during 20 ag−1 cycling, while the coulombic efficiency was maintained at approximately 100%. The researchers systematically analyzed the mechanism of the high performance and found that charring of the in situ grown polymer removed some of the -F croup and -F canbe effects. In addition, the asymmetric supercapacitor constructed based on P-MXene/CPAQA electrodes exhibited the state-of-the-art so far (Figure 2).
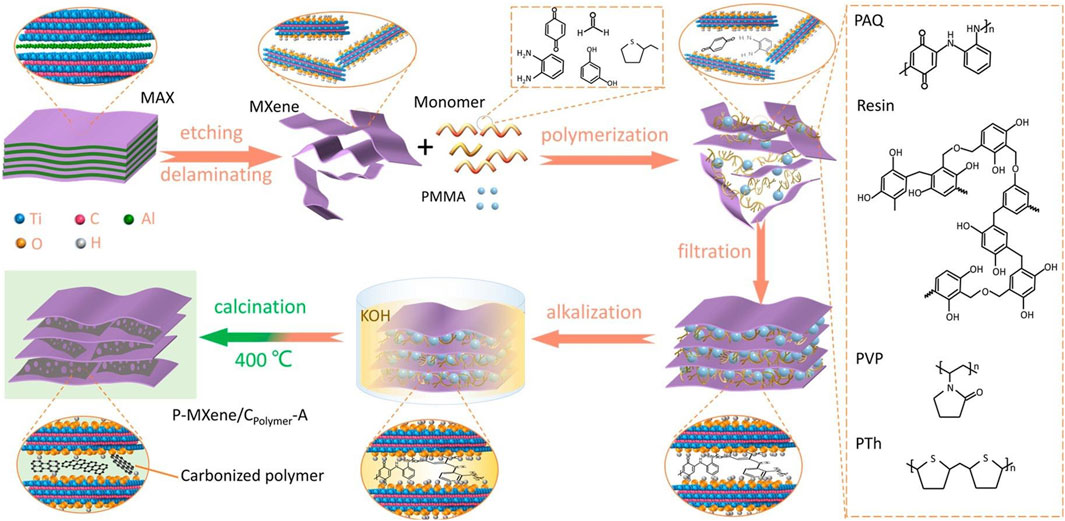
Figure 2. Schematic design of self-supporting flexible P-MXene/CPAQ-A film electrode (Ma et al., 2022).
In addition, because MXene has many polar functional groups on its surface, these functional groups allow MXene to be well dispersed in a variety of polar solvents and show high potential for printing of energy storage devices. The advantages of inkjet printing include simplicity, speed, flexibility, high resolution, cost-effectiveness, and functional materials that can be used for large-scale direct plate making. For example, Wen et al. (2022) they was discovered that the layers of MXene and graphene can be joined by hydrogen bonds, and that the addition of graphene nanosheets can further improve the interlayer spacing and specific surface area of MXene while lowering the self-stacking effect. As a result, they created an MXene/1 wt% graphene composite electrode utilizing inkjet printing technique. This electrode has a high capacitance of 183.5 F cm−3 and good electrical conductivity. With a power density of 10 W cm−2, the surface capacitance was 3.84 mF cm−2, and the energy density amounted to 0.53 Wh cm−2 in the flexible supercapacitor that was created using this composite electrode. Modern flexible electronic energy storage devices are designed and manufactured on the foundation of the inkjet-printed MXene/graphene composite film electrode.
3.2 Sensors
MXene has been widely employed in a variety of flexible sensors, including strain sensors, biosensors, and gas sensors. It has superior mechanical properties, a low specific heat capacity per unit area, is biocompatible, and has a high selectivity for specific gases.
3.2.1 Strain sensors
Flexible strain sensors translate tensile strain of a device into an electrical signal output by leveraging structural changes in the internal network of sensitive materials. These sensors are crucial for human motion detection and health monitoring. The crack extension mechanism and the disconnection mechanism are the two primary sensing methods used by MXene as a sensitive material. The term “crack expansion mechanism” describes how the resistance of MXene changes as a result of the generation of cracks to disperse the stress during the stretching process. MXene’s cracks will first form in the area where the tension is concentrated and will progressively increase as the stress is elevated. The disconnection mechanism refers to the minor relative slippage between MXene layers, where the resistance rises because the connecting path is lessened and the overlap area between MXene layers diminishes as a result of the slippage as the stress increases (Jiang et al., 2019b). Both of these detecting techniques are efficient up to the critical strain range, after which the flexible strain sensor’s resistance will infinity.
Due to the special conductivity and self-stacking properties of MXene materials between layers, the resulting low initial resistance and small variations have an impact on pressure sensitivity. In addition, the low mechanical strength of the MXene material makes it difficult to keep the structure intact under high pressure (Liu Q. et al., 2016). Since the 1D nanomaterials have high aspect ratios and can easily form highly conductive percolation networks, they can be compounded with MXene to build a bridge-like structure. During the stretching process, this structure can connect up the microcracks formed by MXene, thus ensuring the integrity of the conductive paths and thus expanding the strain range, and thus can significantly and effectively improve the mechanical and electrochemical properties of macroscopic MXene nanosheets. Su et al. (2022) bacterial cellulose (BC) polymer interlayer to MXene material to create MXene/bacterial cellulose films, which can distinguish between various natural sounds not only by observing the movement of laryngeal muscles to recognize different speech signals and sound properties, but also by sensing the air pressure waves caused by sound transmission. This method was used to create a three-dimensional isolation layer paper pressure sensor for wearable sound detectors. It is able to record and playback musical impulses. It also has advantages such as small thickness, light weight, biodegradable raw materials, etc., along with good sensing performance, wide linear range, fast response/recovery time (99/93 ms), high stability (5,000 cycles), and can monitor traditional body movements such as finger pressing and knocking, exhaling and blowing, and head nodding, swallowing, and coughing. Changes in the smallest units and vocal properties of different languages can also be identified by monitoring the movement of the laryngeal muscles. This shows the potential application of strain sensors for sound visualization and provides creative solutions for this purpose (Figure 3).
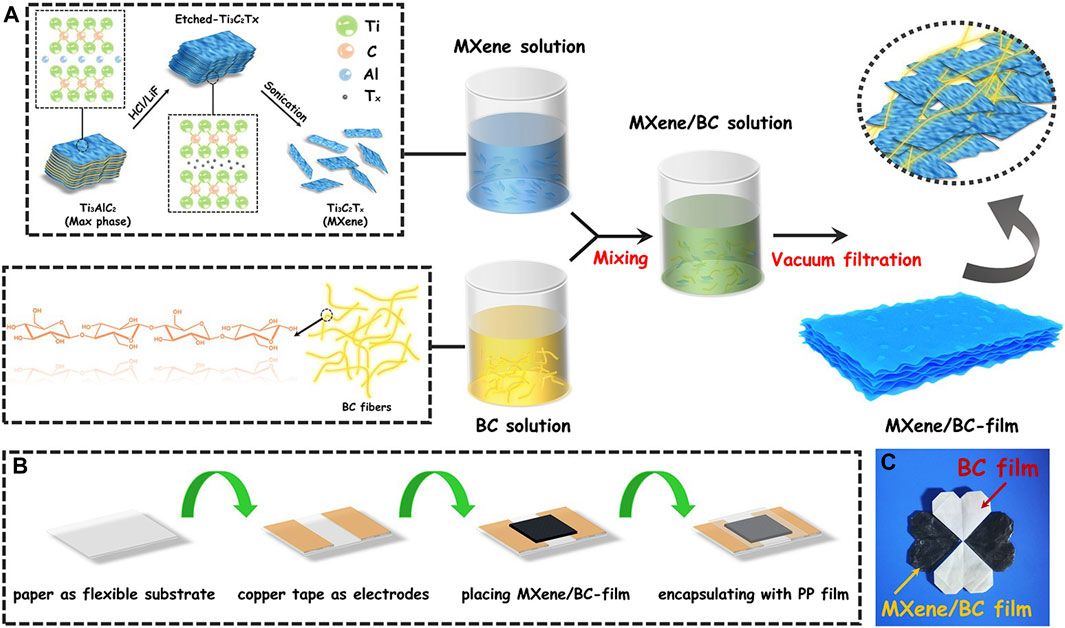
Figure 3. (A) Flow-chart for the preparation of the MXene/BC film. (B) Fabrication method of the MXene/BC film pressure sensor. (C) Photo of the folded BC film and MXene/BC film (Su et al., 2022).
The introduction of other conductive materials for sensor preparation complicates the fabrication process and requires consideration of compatibility and interfacial interactions between different materials. To simplify the process and improve the compatibility, some researchers have controlled the microscopic morphology of MXene by changing the etching conditions, and then combined different morphologies of MXene to construct micro/nano structures to maintain the integrity of the conductive paths during strain. Zhang Y. et al. (2023) introduced and prepared a flexible pressure sensor using reduced graphene oxide (rGO) with a hierarchical structure, and used molecular dynamics simulations to study the formation of reduced graphene oxide microstructures, which were finely stacked from reduced graphene oxide on a polydimethylsiloxane (PDMS) substrate. The resulting sensor has a wide detection range of 0–300 kPa (>10 kPa−1) and high sensitivity of 133.003 kPa−1 (<40 kPa), and multiple pressure points are created at the interface to obtain higher signal output. The sensor exhibits fast response time (<27 ms) in detecting child door locks and excellent reversibility and durability in monitoring over 10,000 cycles of robotic arm loads and heartbeats.
Cheng et al. (2023) presented the study and preparation of a MXene film possessing high strength and used for self-healing flexible pressure sensors. To overcome the weak bonding between the layers of the two-dimensional material, hydrophilic functional groups were used to modify the MXene surface and strong hydrogen bonds were introduced through high strength ANF. The obtained MXene films have excellent mechanical strength, sensitivity (208.7 kPa−1), fast response time (<35 ms), and excellent cycling stability (>45,000 cycles). The addition of 30% ANF further improves the tensile strength (65.5 MPa) and toughness (210.9 MJ m−3) compared to pure MXene. And the sensor also has self-healing capability, maintaining sensitivity at 98% after the first healing and 80% after the sixth healing. The MXene film combined with the self-healing housing provides robustness against external damage, making the sensor more suitable for future wearable electronic devices.
3.2.2 Biosensors
A biosensor is a device that uses the biological reactions that take place in living things to identify particular organic molecules. Biosensors provide advantages over conventional chemical analysis techniques, such as excellent selectivity, minimal sample requirements, and quick detection times. In numerous sectors, including medicine, food and drug analysis, agricultural science, and environmental science, biosensors have been crucial.
MXene materials have hydrophilic surfaces and highly tunable surface groups. These properties allow MXene to selectively adsorb biomolecules, such as glucose and dopamine, through morphology control and surface modification. These biomolecules can be fully contacted with enzymes, thus increasing the sensitivity of the biosensor. In addition, the main elements of MXene are transition metals, such as Ta, Ti, and Nb, which present inert, and thus MXene has good biocompatibility. Feng et al. (2023) constructed three-dimensional porous flexible composites Ti3C2Tx MXene/AuNPs (gold nanoparticles) by mixing MXene materials with gold nanoparticles and then applying a self-reduction process after freeze-drying. This composite has a highly hydrophilic microenvironment and a more open structure, which contributes to the stable fixation and retention of GOx (glucose oxidase) in the membrane. The introduction of AuNPs further enhances the electron transfer in the 3D porous composite and reduces the redox potential, while AuNPs also have a catalytic effect on glucose molecules. This composite can be used to make a glucose sensor, which has the advantages of wide response range, high sensitivity, low response limit, and fast response, and the sensitivity is 169.49 μA mM−1 cm−2 for detection within the glucose concentration range of 2 μM to 0.4 mM. In addition, the sensor has a detection limit of 2 μM and a response time of less than 3 s. Therefore, it can be used to detect glucose in human sweat. In addition, the performance of the sensor can be optimized by simply adjusting the size of the pores inside the MGA composite film.
3D printing as an advanced manufacturing technology has received wide attention in the field of electrochemistry. Compared with traditional manufacturing techniques, 3D printing has the advantages of high speed, low price, and low waste. The fabrication of electrochemical sensors for the detection of disease-related biomarkers using 3D printing technology has gained attention in the field of disease diagnosis. Wan et al. (2023) presented the fabrication of a novel thin-film dopamine (DA) sensor using 3D printed electrodes (3DE) made of graphene/polylactic acid (PLA) filaments and then modified with synthetic MXene quantum dots (MQDs) for highly sensitive detection of DA. The sensor has a detection range of 0.01 ∼ 20 μM for DA concentration with an ultra-low limit of detection (LOD) of 3 nM and high selectivity, and still shows good stability after a week of detecting 1 μM and 10 μM DA and exhibits good anti-interference ability. This enhanced 3D printed sensor provides a new way to use 2D material quantum dots in 3D printed electrodes for biosensing applications and broadens the application scope of 3DEs (Figure 4).
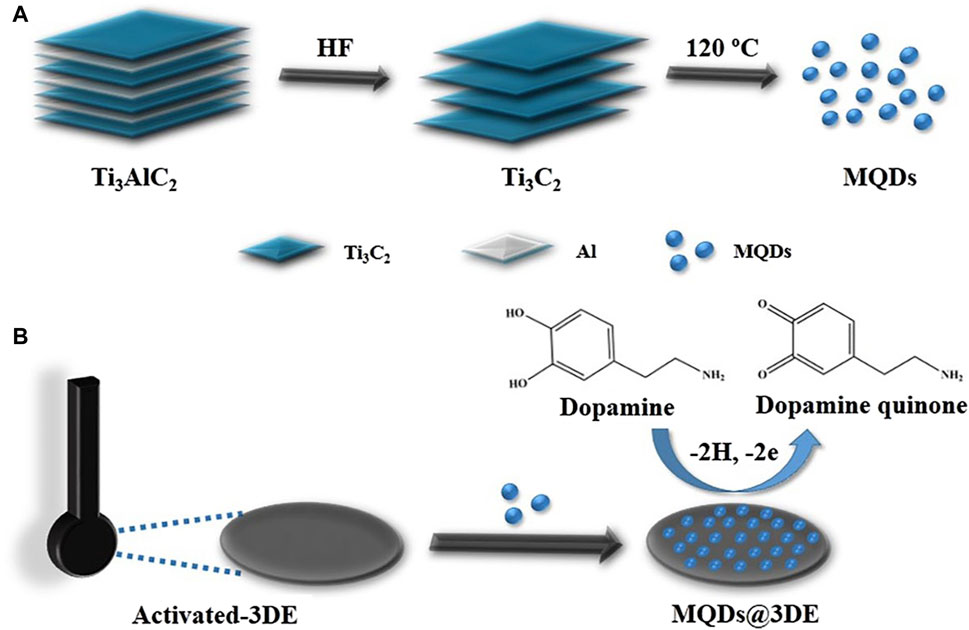
Figure 4. Synthetic route of (A) MQDs and (B) MQDs@3DE, and oxidation mechanism of DA on MQDs@3DE sensor (Wan et al., 2023).
3.2.3 Gas sensors
A gas sensor is a sensor that detects a particular gas composition and transforms data about gas concentration into an electrical signal. Applications for gas sensors include monitoring car exhaust, detecting greenhouse gases, evaluating air quality, and more. Additionally, by identifying endogenous marker gas components linked to human diseases, gas sensors can be employed in dental medical diagnostics to rapidly, accurately, and safely carry out initial screening for a number of disorders.
Surface adsorption of gas molecules to the surface of 2D materials usually occurs, and this interaction leads to charge transfer and changes in the concentration of carriers on the surface of the 2D material, which results in changes in the surface conductivity of the 2D material. Such changes can be used to detect various gas concentrations. Liu Z. et al. (2023) studied the development of Ti3C2Tx/Ti3AlC2 flexible composite gas sensor and found that Ti3C2Tx material has limitations in gas sensing due to high detection limit and poor long-term stability. So on this basis, Ti3C2Tx/Ti3AlC2 composites were prepared using Ti3AlC2 as the backbone with a high molar ratio of HCl and LiF. The prepared composites showed high sensitivity and selectivity for NH3 gas with a detection limit of 50 ppb at room temperature. The composites also exhibited good repeatability and long-term stability with almost no response change even after 120 days. The structure and gas-sensitive properties of the composite were investigated, and the intrinsic mechanism behind its excellent gas-sensitive performance was explored. Meanwhile, the Ti3C2Tx/Ti3AlC2 composite prepared by using a high LiF/HCl solution avoids the use of harmful hydrofluoric acid and produces more -O and -OH functional groups on the material surface. This study provides a theoretical basis for the research and practical application of MXene materials for the preparation of gas sensors (Figure 5). In addition Quan et al. (2023) introduced and prepared a fully flexible Ti3C2Tx -MXene electrode combined with Ti3C2Tx/WS2 paper-based gas sensor design for high-sensitivity NO2 detection. Ti3C2Tx/WS2 exhibited high conductivity, effective charge transfer and abundant gas-sensitive active sites, resulting in a gas-sensitive sensor response of 15.2% at room temperature for 1 ppm NO2 152 times higher than the AuE + Ti3C2Tx gas sensor, with an LOD of 11.0 ppb NO2 gas. The design exhibits excellent stability and repeatability under high humidity conditions. Additionally, calculations using the density general function theory show that elements like the heterojunction modulation effect, power function matching, and the suppression of metal-induced gap states are crucial in enhancing gas-sensitive performance. This work shows the relevance of high-performance gas sensors based on MXene’s nonmetallic electrodes and gas-sensitive films at room temperature and offers new ideas for constructing flexible gas sensors based on conductive electrodes and gas sensing materials on paper. Numerous investigations on MXene flexible gas sensors have been conducted recently. The goal of these experiments is still to create very flexible gas sensors that respond precisely to particular gases by manipulating the groups on the MXene surface or by mixing the material with others.
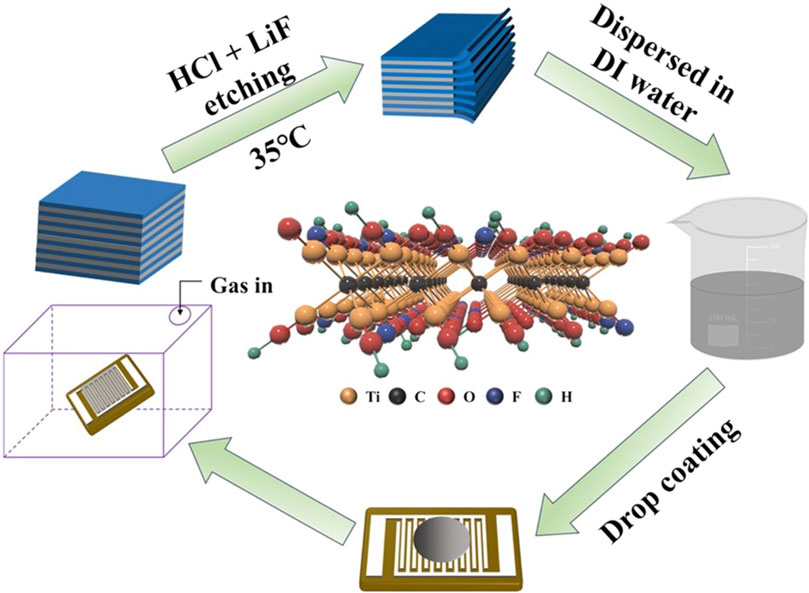
Figure 5. Schematic diagram of the atomic structure of Ti3C2Tx and preparation of Ti3C2Tx/Ti3AlC2 planar composite gas sensor (Liu Z. et al., 2023).
3.3 Thermoelectric materials
Thermoelectric material is a new type of energy material that can convert heat and electricity to each other without additional energy input. When a certain temperature difference exists between the two ends of the material, an electrical potential difference is created and a stable current loop is formed, which is known as the Seebeck effect. Conversely, when an electric current flows through a thermoelectric material, it causes a directional flow of heat, resulting in a temperature difference between the two ends of the material, which is known as the Peltier effect. This material has a wide range of promising applications in the fields of manned spaceflight, defense industry, automotive manufacturing and micro/nano electronics, especially in the fields of temperature difference power generation and thermoelectric cooling (Shi et al., 2020).
Potential options for thermoelectric materials that can be combined to enhance their thermoelectric properties include MXene and single-walled carbon nanotubes (SWCNTs). SWCNTs and MXene materials can be combined to create an interface that functions as a barrier to phonon transport, effectively lowering the material’s thermal conductivity. MXene and SWCNTs may interact d- and electrostatically, enhancing charge transport and maintaining excellent electrical conductivity. Further enhancing the Seebeck coefficient is possible by energy filtering the various energy levels of MXene and SWCNTs. A three-dimensional hollow structure with decreased thermal conductivity can be created in the SWCNTs/MXene composite films. Wei et al. (2022) prepared the first series of SWCNTs/MXene composite films with three-dimensional hollow structures as effective p-Type TE materials using the structural characteristics of one-dimensional SWCNTs and two-dimensional MXene. The Seebeck coefficients and power factors of these composites are higher than those of single SWCNTs materials, while the thermal conductivity is significantly lower. At 10 wt% MXene loading, the composite films exhibited the best TE performance with a Seebeck coefficient of 39.64 μV K−1 and a power factor of 203.29 μW m−1 K−2 at room temperature. These results suggest that this simple vacuum filtration method is an effective way to prepare SWCNTs-based TE materials and provides a simple strategy to design high-performance SWCNTs-based TE materials. Wei et al. (2023) further enhanced the performance of SWCNTs/MXene composites by adding polyaniline (PANI) nanoparticles to them, and the electrodes they studied and prepared were ternary composites consisting of Ti3C2Tx MXene, polyaniline (PANI), and single-walled carbon nanotubes (SWCNTs). A comparison with the conventional Pt electrode reveals that the porous layered structure of the electrode has a large electrochemically active surface area, which significantly improves the thermoelectric chemistry performance. The synergistic effect of MXene and PANI further promotes the mass and charge transport at the electrolyte interface with an output power of 13.15 μW cm−2 at a temperature difference of 40 K. and exhibited a fast response to the small temperature difference between the human body and the environment, indicating its potential for harvesting low-grade heat to power small electronic devices (Figure 6).
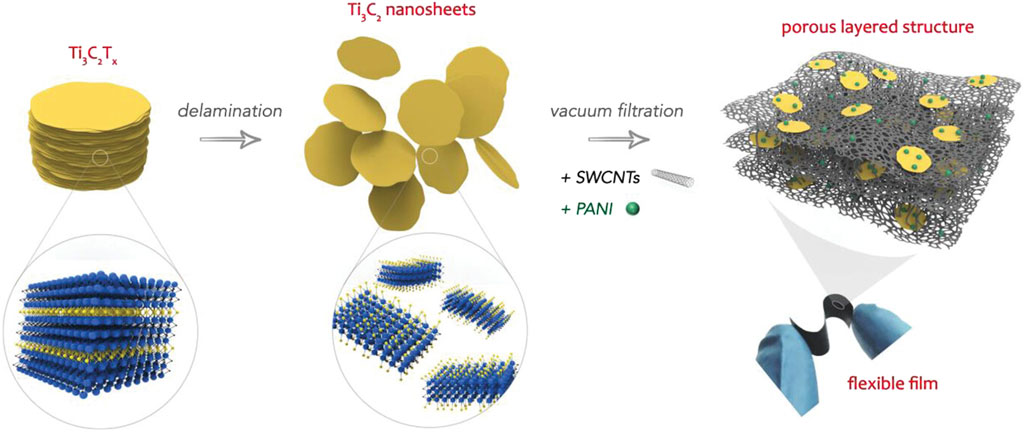
Figure 6. Formation of porous layered ternary composite film electrodes (Wei et al., 2023).
Deposition of a thin layer of inorganic material on a flexible substrate or porous structure results in some flexibility and good thermoelectricity (TE) performance. The insertion of organic molecules into some layered inorganic TE materials has been shown to be effective in synergizing TE performance and flexibility. The electron injection effect is one of the important mechanisms to optimize the TE properties. Therefore, the intercalation of ionic organic compounds is expected to improve the carrier concentration in MXene films by a similar mechanism. Wang et al. (2022) constructed MXene nanosheets/organic superlattices (SL) by intercalating hexamine (HA) and N-methylformamide NMF, followed by exfoliation and self-assembly to enhance the carrier concentration by electron injection. Subsequently, annealing was performed at different temperatures to tune the interlayer spacing, enhance interlayer coupling, reduce edge oxidation, and improve intra/interlayer mobility to obtain better TE performance and stability. The resulting SL structure with increased carrier concentration and reduced resistance resulted in an 8-fold improvement in power factor to 33 μW m−1 K−2, and the TE module consisting of four SL legs generated 58.6 nW at a temperature gradient of 50 K. The results provide a strategy for tailoring the TE performance of 2D material films by SL structures and composite engineering, which has potential applications in flexible electronic devices.
Although a number of flexible MXene materials with good thermoelectric potential have been reported theoretically, there are still few flexible MXene materials that have been successfully prepared and characterized in experiments. As highly conductive materials, MXene materials have a certain Seebeck coefficient and thus have potential thermoelectric applications. Although the currently prepared MXene materials have high conductivity, their power factor is not very high due to the low Seebeck coefficient, so increasing the Seebeck coefficient is the focus of current research. In addition, the thermoelectric properties of MXene can be tuned by various means, which is one of the hot spots of current research.
3.4 Electromagnetic shielding
MXene is a material with efficient electromagnetic shielding properties, good electrical conductivity, large specific surface area, light weight and easy processing properties. MXene has tunable surface chemistry and excellent mechanical strength, which facilitates the development of composite materials with controlled structural design. The two-dimensional Ti3C2Tx MXene films exhibit excellent electromagnetic shielding, with single films shielding 20% of electromagnetic waves and 24 films of 55 nm thickness shielding 99% of electromagnetic waves. However, pristine MXene exhibits poor mechanical properties, so composites composed of MXene are key to EMI shielding substrates due to their enhanced mechanical properties and improved processability (Li et al., 2019). The excellent mechanical properties and electrical conductivity of composites can effectively attenuate electromagnetic waves that penetrate the material.
Due to their high electrical conductivity, robust interlayer contacts, and large aspect ratio, two-dimensional nanomaterials like graphene oxide and MXene are frequently utilized to make conductive films and porous derivatives. Usually, porous morphology is preformed or postprocessed to create these nanomaterials. Ice crystals and polymer microsphere templates can form connected cytosolic structures in the liquid dispersion of nanosheets, and gaseous material can gather in the interlayer of the formed dense film and then expand under sufficiently high pressure to introduce a significant number of pores within the film (Tang et al., 2020). However, the numerous pores that are introduced in this fashion may make it difficult for nanosheets to link to one another and make contact with one another, which may damage the mechanical and electrical properties of porous films. Additionally, the constructed materials’ EMI shielding efficacy can be increased by the functional groups and structural flaws suspended on the nanoparticles. Zhang et al. (2022) in this study, a porous mechanically flexible and electrically conductive film consisting of reduced graphene oxide (rGO)-Ti3C2Tx MXene (rG-M) was created by hydrazine foaming. This porous membrane’s continuous cell shape is optimized, and its mechanical and electrical qualities are exceptional. MXene stops the membrane from over-expanding and enhances the electron conduction pathways, improving the mechanical characteristics. With improved cell shape and density in comparison to the pure rGO porous membrane, the rG-M homologue achieves high tensile strength of 18.7 MPa and electrical conductivity of 37.7 S cm−1 with an initial MXene concentration of 50%. This is accomplished by providing efficient load transfer and better conduction routes. The high enhancing electromagnetic interference (EMI) shielding effect of 52.6 dB was achieved by adjusting the thickness of the porous film and the treatment process, which provides a feasible way for the preparation of lightweight high-performance EMI shielding materials. This chemically controlled foaming technique aids in the creation of new porous conductive films for high-performance EMI shielding materials that have a density that is properly specified and an interconnected structure (Figure 7).
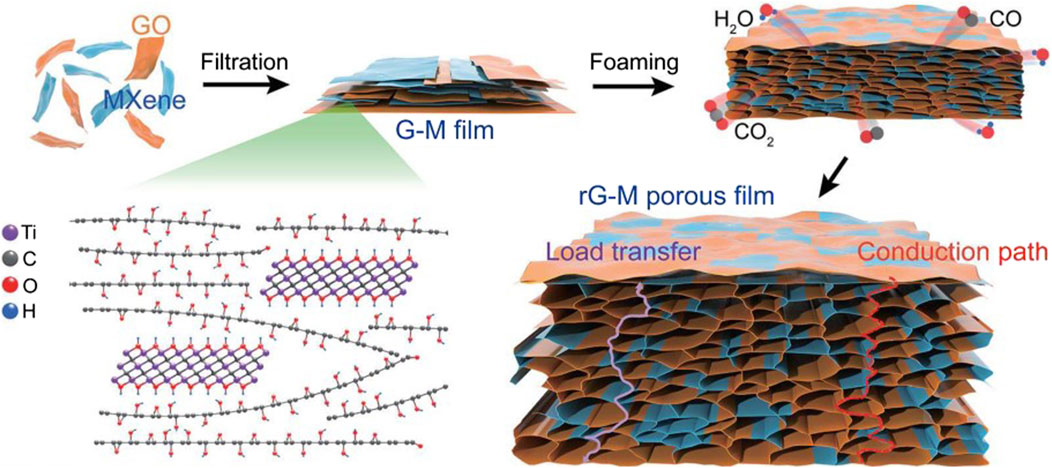
Figure 7. Schematic representation of the fabrication of rG-M porous membrane and its loading and electron transport paths (Zhang et al., 2022).
Although MXene materials have great potential for EMI shielding applications, however, the material is prone to degradation in humid environments, a characteristic that limits further research and commercial applications of these materials. Li et al. (2022a) discussed and proposed a strategy to protect MXene films by coating a layer of graphene oxide (GO) on MXene films using a sequential filtration method. Graphene oxide not only protects MXene from oxidation, but also forms a heterojunction interface, which increases the loss and optimizes the impedance matching between MXene and air, EMI shielding. The amount of GO can be optimized to improve the EMI shielding performance of MXene films. The resulting GO/Ti3C2Tx MXene/GO (GMG) films exhibited relatively stable EMI shielding performance for up to 70 days. This strategy is cost-effective and allows the development of practical and durable MXene based EMI shielding materials. The graphene oxide layers also retard the degradation of MXene films by preventing air and water from penetrating the closely stacked graphene oxide layers. However, the thickness of the graphene oxide layers should be reasonably optimized to prevent excessive degradation of MXene during processing. Overall, the proposed strategy is beneficial to improve the performance and lifetime of MXene based EMI shielding materials.
The weak interactions between MXene sheets are detrimental to the construction of strong and pure MXene porous structures, which is one of the reasons for their limited applications. To solve this problem, polymers are widely used as “binder phases” to improve the mechanical strength and flexibility of MXene-based macrostructures (Zeng et al., 2022). Introduced a composite foam based on dip-coating and chemical cross-linking methods for the preparation of lightweight, flexible, durable and large-area cross-linked Ti3C2 MXene coated polyimide (PI) (MXene/PI). By utilizing a porous and robust PI support, coated MXene nanoflakes and an effective chemical cross-linking treatment, Mxene/PI foams are hydrophobic, water resistant, oxidation resistant and extremely temperature stable. Also, the combination facilitates high utilization of MXene conductivity and interfacial polarization between MXene and PI, which improves EMI shielding performance. The prepared Mxene/PI foams exhibit ultra-high EMI shielding performance with SSE and SSE/d values of 1,971 dB cm3 g−1 and 21,317 dB cm2 g−1, respectively, which are significantly better than other typical porous structures. In addition, Mxene/PI foams offer fast reproducibility, stable electrothermal effects and sensitive and reliable human motion detection. However, these polymeric carrier materials are usually derived from fossil resources and are complicated to obtain and process. As a natural renewable resource, wood, in contrast, has a high strength-to-weight ratio and is used extensively in many different industries. Wood can be utilized to strengthen composite materials because of its strong mechanical strength and chemical activity. Jiang et al. (2022) introduced a method to prepare flexible MXene/wood composites (F-MWC) by immersing delignified wood in MXene suspension and then making it more flexible and robust by hot pressing. This composite has excellent electromagnetic shielding and flame retardant properties and can be used in electronic devices, packaging and construction materials. F-MWC demonstrates excellent performance and holds great promise. F-MWC displays excellent mechanical qualities during mechanical compression, with a tensile strength of 68.1 MPa. Additionally, MXene nanosheets and mechanical compression work together to produce materials with high flame retardancy and self-extinguishing properties. F-MWC has a conductivity of around 1858 S m−1 at a thickness of 0.38 mm, and its EMI SE in the X-band is 32.7 dB. The F-MWC is also constructed as a multilayer composite to achieve a 42 dB ultra-high EMI shielding effect. The amount of MXene and the number of F-MWC layers stacked together can affect how much EMI is present in MXene/wood composites. The weak water resistance of this composite must be improved in the future, and MXene/wood composites should be avoided in areas with high relative humidity.
In conclusion, this collection of studies and pioneering methods underscores the substantial promise and adaptability of MXene-based materials for electromagnetic interference shielding. Utilizing a range of innovative fabrication techniques and composite configurations, researchers are enhancing the mechanical, electrical, and protective qualities of MXene, expanding its applications across diverse sectors from electronics to construction. These advancements not only tackle pressing technological challenges but also set the stage for future innovations that could transform the performance and longevity of EMI shielding materials.
3.5 Thermal management
In the current landscape of electronic device manufacturing, polymers are extensively utilized for thermal management applications. As electronic devices trend towards miniaturization, ultra-thinness, integration, and multifunctionality, the demands on the thermal conductivity and carrier mobility of polymer-based materials have intensified. However, the intrinsic amorphous structure of polymers, coupled with inconsistent lattice vibrations and inherent defects, inhibits effective phonon transmission and causes significant phonon scattering. This not only impairs the thermal stability and dissipative performance of polymers but also restricts their use in cooling high-power electronic devices. To overcome these challenges, the current strategy involves incorporating highly conductive nanofillers such as metal nanoparticles, graphene nanosheets, carbon nanotubes, and hexagonal boron nitride nanosheets, which establish efficient thermal conduction paths. Despite these enhancements, the high electrical conductivity of these fillers does not satisfy the insulation requirements of electronic devices, and a high filler content often reduces the mechanical strength of polymers, increasing their brittleness.
In this context, the use of two-dimensional (2D) materials like MXene as nanofillers proves vital. MXene, known for its exceptional surface termination groups and superior thermal conductivity, not only fortifies the thermal interface but also curtails phonon scattering through improved interface compatibility with the polymer matrix. The robust structural stability and adjustable surface chemistry of MXene amplify both the thermal and electrical insulation properties of composites, making it an exemplary material for managing heat in electronic devices. Moreover, MXene’s flexibility allows for its integration into flexible electronics, providing efficient thermal management solutions without compromising on device reliability or performance (Safarkhani et al., 2023). Liu Y. et al. (2023) research the successful development of high-performance poly (p-phenylene-2, 6-benzobisoxazole) (PBO)/MXene nanocomposite films through a sol-gel-film conversion method facilitated by a homogeneous gelation process. Leveraging the unique brick and mortar structure, enhanced by the intricate bridging and caging effects of PBO nanofibers on MXene nanosheets, these films exhibit exceptional mechanical properties with a tensile strength of 416.7 MPa, Young’s modulus of 9.1 GPa, and toughness of 97.3 MJ m−3. Moreover, the strategic alignment of PBO nanofibers and MXene nanosheets imparts the film with a high in-plane thermal conductivity of 42.2 W m−1 K−1 while maintaining electrical insulation at 2.5 × 109 Ω cm. Coupled with superior thermal stability and flame retardancy, these attributes render the PBO/MXene composite films highly suitable for advanced thermal management applications in flexible electronics, demonstrating the potential to create high-performance thermally conductive yet electrically insulating composites by integrating electrically conductive fillers within a meticulously designed structure. Zhan et al. (2023) introduces a novel fabrication method for flexible, high-strength, thermally conductive nanocomposite films, employing a non-toxic solvent displacement system to create a homogeneous hydrogel from MXene nanosheets and aramid nanofibers (ANF). The process utilizes strong intermolecular hydrogen bonding to form large-scale, shape-controlled structures. Subsequent drying and hot-pressing significantly enhance both in-plane (15.50 W m−1 K−1) and through-plane (0.82 W m−1 K−1) thermal conductivity—improvements of 3.2 and 7.5 times over pure ANF, respectively. This method not only optimizes mechanical properties, achieving a tensile strength of 52.7 MPa and a tensile strain of 14.3%, but also catalyzes carbonization, reducing surface degradation and enhancing flame retardancy. The resultant cross-linked structure decreases total heat release to 8.2 kJg−1 and mitigates the emission of toxic gases, offering a robust solution for advanced thermal management applications in electronic devices. This one-step gelation technique, distinguished by its simplicity, efficiency, and environmental friendliness, demonstrates significant potential for producing multifunctional, flame-retardant thermal management materials.
In summary, the development of MXene-based nanocomposite films marks a significant breakthrough in materials science, particularly within the domain of thermal management for electronic devices. These advancements effectively tackle the pressing challenges associated with heat dissipation in devices that are progressively becoming more miniaturized and multifunctional, while simultaneously establishing new benchmarks for integrating flexibility with mechanical robustness in electronic components.
MXene-based flexible composites offer a wide range of uses beyond the specific applications listed above, including antimicrobial treatment for environmental protection, filtration and separation, as well as electrode materials and catalysts. Li et al. (2022b) studied and created a new technique to create Janus porous PLA fiber membranes by combining hydrophilic TiO2 and hydrophobic PDMS/MXene coating for the purification of medical wastewater, the separation of fresh water from salt water, and other environmental protection applications. In solar thermal tests, the fiber membranes exhibited 60% solar thermal conversion efficiency and 1 kg m−2 h−1 freshwater yield, along with high salt drainage and antibacterial activity.
4 Summary and outlook
In conclusion, as was already noted, there are numerous papers on the uses of flexible MXene-based composites for things like sensors (such as strain, pressure, and gas sensors), supercapacitors, EMI shielding and thermal management. Through several significant instances, the synthesis, structure, characteristics, and development of flexible MXene matrix composites in these sectors are discussed. Although flexible MXene matrix composites exhibit a number of benefits, there are still certain difficulties to overcome in order to advance the development. Accordingly, the following future research trends and directions for flexible MXene matrix composites are anticipated:
1. To expand the use of MXene composites in practical applications, its stability must be improved. This goal has been pursued in a number of ways, including the adsorption of polyanionic groups at the edges of MXene nanosheets to block water molecules from oxidizing MXene, high temperature hydrogen annealing, and silylation treatments to give MXene nanosheets high oxidation resistance. Design guidelines for composites made of MXene. Top-down and bottom-up tactics are frequently used in material synthesis, and a participatory strategy is advised. Designing materials based on intended applications should replace finding and creating materials based on compatible applications.
2. By combining different types of materials, complex structures such as 3D structures, porous structures, Janus structures and bionic structures (e.g., “brick and mortar” structures in pearl layers) are carefully designed to further improve flexibility, toughness, and tensile and compressive strength.
3. A deeper comprehension of the connection between the flexible composites based on MXene’s structure and its properties. For instance, heat processing of Ti3CNTX results in a porous structure, improved electrical conductivity, and the removal of molecular species adsorbed between MXene sheets. However, within the confines of the pre-existing shielding theory, these considerations are insufficient to adequately account for the increase in electrical conductivity seen.
4. If the application of MXene-based flexible composites to wearable devices needs to take into account the human body structure, wearing comfort (e.g., breathability/moisture wicking), and a better user experience, and further development of MXene-based fibers with adjustable overall performance.
5 Additional Requirements
Recent Advances and Future Perspectives in Thermal Energy Systems.
Author contributions
PL: Conceptualization, Investigation, Methodology, Validation, Writing–original draft. XZ: Funding acquisition, Writing–review and editing. YD: Conceptualization, Supervision, Writing–review and editing. LC: Funding acquisition, Supervision, Writing–review and editing. XW: Funding acquisition, Supervision, Writing–review and editing. HX: Funding acquisition, Supervision, Writing–review and editing.
Funding
The author(s) declare that financial support was received for the research, authorship, and/or publication of this article. University Capacity Building Project of Shanghai Science and Technology Commission (21010502800), Program for Professor of Special Appointment (Eastern Scholar) at SIHL, the Sailing Project from the Science and Technology Commission of Shanghai Municipality (17YF1406600), Chenguang project supported by Shanghai Municipal Education Commission and Shanghai Education Development Foundation (18CG68), Shanghai Engineering Research Center of Advanced Thermal Functional Materials and Gaoyuan Discipline of Shanghai-Materials Science and Engineering. Shanghai Engineering Research Center of Advanced Thermal Functional Materials.
Conflict of interest
The authors declare that the research was conducted in the absence of any commercial or financial relationships that could be construed as a potential conflict of interest.
Publisher’s note
All claims expressed in this article are solely those of the authors and do not necessarily represent those of their affiliated organizations, or those of the publisher, the editors and the reviewers. Any product that may be evaluated in this article, or claim that may be made by its manufacturer, is not guaranteed or endorsed by the publisher.
References
Ahmadpoor, F., and Sharma, P. (2017). A perspective on the statisticalmechanics of 2D materials. Extreme Mech. Lett. 14, 38–43. doi:10.1016/j.eml.2016.12.007
Anasori, B., Lukatskaya, M. R., and Gogotsi, Y. (2017). 2D metal carbides and nitrides (MXenes) for energy storage. Nat. Rev. Mater. 2 (2), 16098–16117. doi:10.1038/natrevmats.2016.98
Anasori, B., Xie, Y., Beidaghi, M., Lu, J., Hosler, B. C., Hultman, L., et al. (2015). Two-dimensional, ordered, double transition metals carbides (MXenes). ACS Nano 9 (10), 9507–9516. doi:10.1021/acsnano.5b03591
Aslam, M. K., Niu, Y., and Xu, M. (2021). MXenes for non-lithium-ion (Na, K, Ca, Mg, and Al) batteries and supercapacitors. Adv. Energy Mater. 11 (2), 2000681. doi:10.1002/aenm.202000681
Boota, M., Pasini, M., Galeotti, F., Porzio, W., Zhao, M. Q., Halim, J., et al. (2017). Interaction of polar and nonpolar polyfluorenes with layers of two-dimensional titanium carbide (MXene). Intercalation and pseudocapacitance. Chem. Mater. 29 (7), 2731–2738. doi:10.1021/acs.chemmater.6b03933
Cao, X., Wu, M., Zhou, A., Wang, Y., He, X., and Wang, L. (2017b). Non-isothermal crystallization and thermal degradation kinetics of MXene/linear low-density polyethylene nanocomposites. e-Polymers 17 (5), 373–381. doi:10.1515/epoly-2017-0017
Cao, Y., Deng, Q., Liu, Z., Shen, D., Wang, T., Huang, Q., et al. (2017a). Enhanced thermal properties of poly (vinylidene fluoride) composites with ultrathin nanosheets of MXene. RSC Adv. 7 (33), 20494–20501. doi:10.1039/c7ra00184c
Cheng, Y., Xie, Y., Cao, H., Li, L., Liu, Z., Yan, S., et al. (2023). High-strength MXene sheets through interlayer hydrogen bonding for self-healing flexible pressure sensor. Chem. Eng. J. 453, 139823. doi:10.1016/j.cej.2022.139823
Deng, R., Chen, B., Li, H., Zhang, K., Zhang, T., Yu, Y., et al. (2019). MXene/Co3O4 composite material: stable synthesis and its enhanced broadband microwave absorption. Appl. Surf. Sci. 488, 921–930. doi:10.1016/j.apsusc.2019.05.058
Fei, M., Lin, R., Deng, Y., Xian, H., Bian, R., Zhang, X., et al. (2017). Polybenzimidazole/Mxene composite membranes for intermediate temperature polymer electrolyte membrane fuel cells. Nanotechnology 29 (3), 035403. doi:10.1088/1361-6528/aa9ab0
Feng, L., Qin, W., Wang, Y., Gu, C., Li, X., Chen, J., et al. (2023). Ti3C2Tx MXene/Graphene/AuNPs 3D porous composites for high sensitivity and fast response glucose biosensing. Microchem. J. 184, 108142. doi:10.1016/j.microc.2022.108142
Ghidiu, M., Lukatskaya, M. R., Zhao, M. Q., Gogotsi, Y., and Barsoum, M. W. (2014). Conductive two-dimensional titanium carbide “clay” with high volumetric capacitance. Nature 516 (7529), 78–81. doi:10.1038/nature13970
Halim, J., Kota, S., Lukatskaya, M. R., Naguib, M., Zhao, M., Moon, E. J., et al. (2016). Synthesis and characterization of 2D molybdenum carbide (MXene). Adv. Funct. Mater. 26 (18), 3118–3127. doi:10.1002/adfm.201505328
Han, M., Maleski, K., Shuck, C. E., Yang, Y., Glazar, J. T., Foucher, A. C., et al. (2020). Tailoring electronic and optical properties of MXenes through forming solid solutions. J. Am. Chem. Soc. 142 (45), 19110–19118. doi:10.1021/jacs.0c07395
Han, R., Ma, X., Xie, Y., Teng, D., and Zhang, S. (2017). Preparation of a new 2D MXene/PES composite membrane with excellent hydrophilicity and high flux. RSC Adv. 7 (89), 56204–56210. doi:10.1039/c7ra10318b
Hieu, V. Q., Phung, T. K., Nguyen, T. Q., Khan, A., Doan, V. D., Tran, V. A., et al. (2021). Photocatalytic degradation of methyl orange dye by Ti3C2-TiO2 heterojunction under solar light. Chemosphere 276, 130154. doi:10.1016/j.chemosphere.2021.130154
Hu, C., Shen, F., Zhu, D., Zhang, H., Xue, J., and Han, X. (2017). Characteristics of Ti3C2Tx -chitosan films with enhanced mechanical properties. Front. Energy Res. 4, 41. doi:10.3389/fenrg.2016.00041
Jiang, D., Murugadoss, V., Wang, Y., Lin, J., Ding, T., Wang, Z., et al. (2019a). Electromagnetic interference shielding polymers and nanocomposites-a review. Polym. Rev. 59 (2), 280–337. doi:10.1080/15583724.2018.1546737
Jiang, D., Wang, Y., Li, B., Sun, C., Wu, Z., Yan, H., et al. (2019b). Flexible sandwich structural strain sensor based on silver nanowires decorated with self-healing substrate. Macromol. Mater. Eng. 304 (7), 1900074. doi:10.1002/mame.201900074
Jiang, Y., Ru, X., Che, W., Jiang, Z., Chen, H., Hou, J., et al. (2022). Flexible, mechanically robust and self-extinguishing MXene/wood composite for efficient electromagnetic interference shielding. Compos. Part B Eng. 229, 109460. doi:10.1016/j.compositesb.2021.109460
Jiao, S., Zhou, A., Wu, M., and Hu, H. (2019). Kirigami patterning of MXene/bacterial cellulose composite paper for all-solid-state stretchable micro-supercapacitor arrays. Adv. Sci. 6 (12), 1900529. doi:10.1002/advs.201900529
Kajiyama, S., Szabova, L., Iinuma, H., Sugahara, A., Gotoh, K., Sodeyama, K., et al. (2017). Enhanced Li-ion accessibility in MXene titanium carbide by steric chloride termination. Adv. Energy Mater. 7 (9), 1601873. doi:10.1002/aenm.201601873
Li, G., Wyatt, B. C., Song, F., Yu, C., Wu, Z., Xie, X., et al. (2021). 2D titanium carbide (MXene) based films: expanding the frontier of functional film materials. Adv. Funct. Mater. 31 (46), 2105043. doi:10.1002/adfm.202105043
Li, J., Peng, W. J., Fu, Z. J., Tang, X. H., Wu, H., Guo, S., et al. (2019). Achieving high electrical conductivity and excellent electromagnetic interference shielding in poly (lactic acid)/silver nanocomposites by constructing large-area silver nanoplates in polymer matrix. Compos. Part B Eng. 171, 204–213. doi:10.1016/j.compositesb.2019.05.003
Li, S., Zhang, Q., Liu, L., Wang, J., Shi, M., et al. (2023). Ultra-stable sandwich shaped flexible MXene/CNT@Ni films for high performance supercapacitor. J. Alloys Compd. 941, 168963. doi:10.1016/j.jallcom.2023.168963
Li, X., Wang, C., Cao, Y., and Wang, G. (2018). Functional MXene materials: progress of their applications. Chemistry-An Asian J. 13 (19), 2742–2757. doi:10.1002/asia.201800543
Li, Y., Wu, K., Zhang, M., Yang, X., Feng, W., Wang, P., et al. (2022a). Flexible MXene/Graphene oxide films with long-lasting electromagnetic interference shielding performance. Ceram. Int. 48 (24), 37032–37038. doi:10.1016/j.ceramint.2022.08.275
Li, Y., Wu, T., Shen, H., Yang, S., Qin, Y., Zhu, Z., et al. (2022b). Flexible MXene-based Janus porous fibrous membranes for sustainable solar-driven desalination and emulsions separation. J. Clean. Prod. 347, 131324. doi:10.1016/j.jclepro.2022.131324
Liu, C., Li, X., Hao, L. F., et al. (2021). Research progress of functional modification of MXene and its applications. Acta Mater. Compos. Sin. 38 (4), 1020–1028. doi:10.13801/j.cnki.fhclxb.20201218.003
Liu, Q., Chen, J., Li, Y., and Shi, G. (2016b). High-performance strain sensors with fish-scale-like graphene-sensing layers for full-range detection of human motions. ACS Nano 10 (8), 7901–7906. doi:10.1021/acsnano.6b03813
Liu, Y., Zhang, J., Zhang, X., Li, Y., and Wang, J. (2016a). Ti3C2Tx filler effect on the proton conduction property of polymer electrolyte membrane. ACS Appl. Mater. Interfaces 8 (31), 20352–20363. doi:10.1021/acsami.6b04800
Liu, Y., Zou, W., Zhao, N., and Xu, J. (2023b). Electrically insulating PBO/MXene film with superior thermal conductivity, mechanical properties, thermal stability, and flame retardancy. Nat. Commun. 14 (1), 5342. doi:10.1038/s41467-023-40707-x
Liu, Z., Han, D., Liu, L., Han, X., Chen, Y., et al. (2023a). Ultrasensitive ammonia gas sensor based on Ti3C2Tx/Ti3AlC2 planar composite at room temperature. Sensors Actuators B Chem. 378, 133149. doi:10.1016/j.snb.2022.133149
Lu, Z. G., Ma, L. M., Tan, J. B., Wang, H., and Ding, X. (2016). Transparent multi-layer graphene/polyethylene terephthalate structures with excellent microwave absorption and electromagnetic interference shielding performance. Nanoscale 8 (37), 16684–16693. doi:10.1039/c6nr02619b
Ma, R., Zhang, X., Zhuo, J., Cao, L., Song, Y., Yin, Y., et al. (2022). Self-supporting, binder-free, and flexible Ti3C2Tx MXene-based supercapacitor electrode with improved electrochemical performance. ACS Nano 16 (6), 9713–9727. doi:10.1021/acsnano.2c03351
Malaki, M., and Varma, R. S. (2020). Mechanotribological aspects of MXene-reinforced nanocomposites. Adv. Mater. 32 (38), 2003154. doi:10.1002/adma.202003154
Miao, L., Wan, J., Song, Y., Guo, H., Chen, H., Cheng, X., et al. (2019). Skin-inspired humidity and pressuresensor with a wrinkle-on-sponge structure. ACS AppliedMaterials Interfaces 11 (42), 39219–39227. doi:10.1021/acsami.9b13383
Mirkhani, S. A., Shayesteh Zeraati, A., Aliabadian, E., Naguib, M., and Sundararaj, U. (2019). High dielectric constant and low dielectric loss via poly (vinyl alcohol)/Ti3C2Tx MXene nanocomposites. ACS Appl. Mater. interfaces 11 (20), 18599–18608. doi:10.1021/acsami.9b00393
Naguib, M., Kurtoglu, M., Presser, V., Lu, J., Niu, J., Heon, M., et al. (2011). Two-dimensional nanocrystals produced by exfoliation of Ti3AlC2. Adv. Mater. 23 (37), 4248–4253. doi:10.1002/adma.201102306
Naguib, M., Mochalin, V. N., Barsoum, M. W., and Gogotsi, Y. (2014). 25th anniversary article: MXenes: a new family of two-dimensional materials. Adv. Mater. 26 (7), 992–1005. doi:10.1002/adma.201304138
Peng, L., and Yan, B. (2022). Research progress on MXene based flexible piezoresistive sensors. Mater. Guide 36 (14), 82–92.
Quan, W., Shi, J., Luo, H., Fan, C., Lv, W., Chen, X., et al. (2023). Fully flexible MXene-based gas sensor on paper for highly sensitive room-temperature nitrogen dioxide detection. ACS Sensors 8 (1), 103–113. doi:10.1021/acssensors.2c01748
Rajavel, K., Luo, S., Wan, Y., Yu, X., Hu, Y., Zhu, P., et al. (2020). 2D Ti3C2Tx MXene/polyvinylidene fluoride (PVDF) nanocomposites for attenuation of electromagnetic radiation with excellent heat dissipation. Compos. Part A Appl. Sci. Manuf. 129, 105693. doi:10.1016/j.compositesa.2019.105693
Safarkhani, M., Far, B. F., Huh, Y. S., and Rabiee, N. (2023). Thermally conductive MXene. ACS biomaterials Sci. Eng. 9 (12), 6516–6530. doi:10.1021/acsbiomaterials.3c01420
Sajid, M., Kim, H. B., Siddiqui, G. U., Na, K. H., and Choi, K. H. (2017). Linear bi-layer humidity sensor with tunable response using combinations of molybdenum carbide with polymers. Sensors Actuators A Phys. 262, 68–77. doi:10.1016/j.sna.2017.05.029
Sankaran, S., Deshmukh, K., Ahamed, M. B., and Khadheer Pasha, S. (2018). Recent advances in electromagnetic interference shielding properties of metal and carbon filler reinforced flexible polymer composites: a review. Compos. Part A Appl. Sci. Manuf. 114, 49–71. doi:10.1016/j.compositesa.2018.08.006
Shao, J., Wang, J. W., Liu, D. N., Wei, L., Wu, S. Q., and Ren, H. (2019). A novel high permittivity percolative composite with modified MXene. Polymer 174, 86–95. doi:10.1016/j.polymer.2019.04.057
Shen, B., Li, Y., Yi, D., Zhai, W., Wei, X., and Zheng, W. (2016). Microcellular graphene foam for improved broadband electromagnetic interference shielding. Carbon 102, 154–160. doi:10.1016/j.carbon.2016.02.040
Sheng, X., Zhao, Y., Zhang, L., and Lu, X. (2019). Properties of two-dimensional Ti3C2 MXene/thermoplastic polyurethane nanocomposites with effective reinforcement via melt blending. Compos. Sci. Technol. 181, 107710. doi:10.1016/j.compscitech.2019.107710
Shi, X. L., Zou, J., and Chen, Z. G. (2020). Advanced thermoelectric design: from materials and structures to devices. Chem. Rev. 120 (15), 7399–7515. doi:10.1021/acs.chemrev.0c00026
Su, T., Liu, N., Lei, D., Wang, L., Ren, Z., Zhang, Q., et al. (2022). Flexible MXene/bacterial cellulose film sound detector based on piezoresistive sensing mechanism. ACS Nano 16 (5), 8461–8471. doi:10.1021/acsnano.2c03155
Sun, R., Zhang, H. B., Liu, J., Xie, X., Yang, R., Li, Y., et al. (2017). Highly conductive transition metal carbide/carbonitride (MXene)@polystyrene nanocomposites fabricated by electrostatic assembly for highly efficient electromagnetic interference shielding. Adv. Funct. Mater. 27 (45), 1702807. doi:10.1002/adfm.201702807
Tang, X. H., Li, J., Tan, Y. J., Cai, J. H., Liu, J. H., and Wang, M. (2020). Achieve high performance microwave shielding in poly (ε-caprolactone)/multi-wall carbon nanotube composites via balancing absorption in conductive domains and multiple scattering at interfaces. Appl. Surf. Sci. 508, 145178. doi:10.1016/j.apsusc.2019.145178
Wan, M., Jimu, A., Yang, H., Zhou, J., Dai, X., Zheng, Y., et al. (2023). MXene quantum dots enhanced 3D-printed electrochemical sensor for the highly sensitive detection of dopamine. Microchem. J. 184, 108180. doi:10.1016/j.microc.2022.108180
Wang, H., Li, J., Kuai, X., Bu, L., Gao, L., Xiao, X., et al. (2020). Enhanced rate capability of ion-accessible Ti3C2Tx -NbN hybrid electrodes. Adv. Energy Mater. 10 (35), 2001411. doi:10.1002/aenm.202001411
Wang, Z., Chen, M., Cao, Z., Liang, J., Liu, Z., Xuan, Y., et al. (2022). MXene nanosheet/organics superlattice for flexible thermoelectrics. ACS Appl. Nano Mater. 5 (11), 16872–16883. doi:10.1021/acsanm.2c03813
Wei, H., Dong, J., Fang, X., Zheng, W., Sun, Y., Qian, Y., et al. (2019). Ti3C2Tx MXene/polyaniline (PANI) sandwich intercalation structure composites constructed for microwave absorption. Compos. Sci. Technol. 169, 52–59. doi:10.1016/j.compscitech.2018.10.016
Wei, J., Wu, D., Liu, C., Zhong, F., Cao, G., Li, B., et al. (2022). Free-standing p-Type SWCNT/Mxene composite films with low thermal conductivity and enhanced thermoelectric performance. Chem. Eng. J. 439, 135706. doi:10.1016/j.cej.2022.135706
Wei, S., Ma, J., Wu, D., Chen, B., Du, C., Liang, L., et al. (2023). Constructing flexible film electrode with porous layered structure by MXene/SWCNTs/PANI ternary composite for efficient low -grade thermal energy harvest. Adv. Funct. Mater. 33, 2209806. doi:10.1002/adfm.202209806
Wen, D., Ying, G., Liu, L., Li, Y., Sun, C., Hu, C., et al. (2022). Direct inkjet printing of flexible MXene/graphene composite films for supercapacitor electrodes. J. Alloys Compd. 900, 163436. doi:10.1016/j.jallcom.2021.163436
Wu, W., Wei, D., Zhu, J., Niu, D., Wang, F., Wang, L., et al. (2019). Enhanced electrochemical performances of organ-like Ti C32 MXenes/polypyrrole composites as supercapacitors electrode materials. Ceram. Int. 45 (6), 7328–7337. doi:10.1016/j.ceramint.2019.01.016
Wu, X., Hao, L., Zhang, J., Zhang, X., Wang, J., and Liu, J. (2016). Polymer-Ti3C2Tx composite membranes to overcome the trade-off in solvent resistant nanofiltration for alcohol-based system. J. Membr. Sci. 515, 175–188. doi:10.1016/j.memsci.2016.05.048
Yan, D. X., Pang, H., Li, B., Vajtai, R., Xu, L., Ren, P., et al. (2015). Structured reduced graphene oxide/polymer composites for ultra-efficient electromagnetic interference shielding. Adv. Funct. Mater. 25 (4), 559–566. doi:10.1002/adfm.201403809
Yan, J., Ren, C. E., Maleski, K., Hatter, C. B., Anasori, B., Urbankowski, P., et al. (2017). Flexible MXene/graphene films for ultrafast supercapacitors with outstanding volumetric capacitance. Adv. Funct. Mater. 27 (30), 1701264. doi:10.1002/adfm.201701264
Yao, L., Ou, G., Liu, W., Zhao, X., Nishijima, H., and Pan, W. (2016). Fabrication of high performance oxygensensors using multilayer oxides with high interfacialconductivity. J. Mater. Chem. A 4 (29), 11422–11429. doi:10.1039/c6ta01052k
Yu, B., Tawiah, B., Wang, L. Q., Yin Yuen, A. C., Zhang, Z. C., Shen, L. L., et al. (2019). Interface decoration of exfoliated MXene ultra-thin nanosheets for fire and smoke suppressions of thermoplastic polyurethane elastomer. J. Hazard. Mater. 374, 110–119. doi:10.1016/j.jhazmat.2019.04.026
Zeng, Z. H., Jin, H., Chen, M. J., Li, W., Zhou, L., and Zhang, Z. (2015). Lightweight and anisotropic porous MWCNT/WPU composites for ultrahigh performance electromagnetic interference shielding. Adv. Funct. Mater. 26 (2), 303–310. doi:10.1002/adfm.201503579
Zeng, Z. H., Wu, N., Wei, J. J., Yang, Y. F., Wu, T. T., Li, B., et al. (2022). Porous and ultra-flexible crosslinked MXene/polyimide composites for multifunctional electromagnetic interference shielding. Nano-micro Lett. 14 (1), 59. doi:10.1007/s40820-022-00800-0
Zhan, Y., Zheng, X., Nan, B., Lu, M., Shi, J., and Wu, K. (2023). Flexible MXene/aramid nanofiber nanocomposite film with high thermal conductivity and flame retardancy. Eur. Polym. J. 186, 111847. doi:10.1016/j.eurpolymj.2023.111847
Zhang, P., Li, J., Yang, D., Soomro, R. A., and Xu, B. (2023a). Flexible carbon dots-intercalated MXene film electrode with outstanding volumetric performance for supercapacitors. Adv. Funct. Mater. 33 (1), 2209918. doi:10.1002/adfm.202209918
Zhang, Y., Wang, Y., Wang, C., Zhao, Y., Jing, W., Wang, S., et al. (2023b). Superior performances via designed multiple embossments within interfaces for flexible pressure sensors. Chem. Eng. J. 454, 139990. doi:10.1016/j.cej.2022.139990
Keywords: MXene, flexible composite, supercapacitor, sensor, electromagnetic shielding
Citation: Li P, Zhao X, Ding Y, Chen L, Wang X and Xie H (2024) Application studies on MXene-based flexible composites. Front. Therm. Eng. 4:1440165. doi: 10.3389/fther.2024.1440165
Received: 29 May 2024; Accepted: 23 July 2024;
Published: 05 August 2024.
Edited by:
Luca Bergamasco, Polytechnic University of Turin, ItalyReviewed by:
Dong Liu, Nanjing University of Science and Technology, ChinaMeijie Chen, Central South University, China
Copyright © 2024 Li, Zhao, Ding, Chen, Wang and Xie. This is an open-access article distributed under the terms of the Creative Commons Attribution License (CC BY). The use, distribution or reproduction in other forums is permitted, provided the original author(s) and the copyright owner(s) are credited and that the original publication in this journal is cited, in accordance with accepted academic practice. No use, distribution or reproduction is permitted which does not comply with these terms.
*Correspondence: Xueling Zhao, eGx6aGFvQHNzcHUuZWR1LmNu; Lifei Chen, bGZjaGVuQHNzcHUuZWR1LmNu