- 1School of Chemical Engineering and Technology, Xi’an Jiaotong University, Xi’an, China
- 2Department of Mechanical Engineering, Xi’an Jiaotong University City College, Xi’an, China
- 3Department of Mechanical Engineering Science, University of Johannesburg, Johannesburg, South Africa
- 4School of Engineering, University of Kent, Canterbury, United Kingdom
- 5South African Institute for Advanced Materials Chemistry, University of the Western Cape, Bellville, South Africa
Hydrogen, as a kind of green and efficient energy, plays an increasingly important role in current social development. Hydrogen storage technology is considered to be one of the main bottlenecks in limiting the large-scale application of hydrogen energy. The solid-state hydrogen storage technology based on Mg-based materials has received extensive attention due to its advantages of high hydrogen capacity, good reversibility, and low cost, but there are still shortcomings such as high reaction temperature, large energy consumption, and slow reaction kinetics. In order to solve these problems, this article proposes a new method of using microwave plasma to ionize hydrogen into H− ion. The possible activation mechanism of microwave plasma to improve the hydrogen storage properties is put forward. Based on the activation mechanism, the thermodynamic performance of Mg-based hydrogen storage is evaluated using density functional theory. It is concluded that the reaction temperature is significantly reduced from 339°C to 109°C with the help of microwave plasma. In addition, the comparison between the conventional heating hydrogen storage process based on MgH2 and microwave enhanced advanced hydrogen storage process based on MgH2 systems coupled with solid oxide fuel cells for heat and power generation is conducted to evaluate the economic feasibility. The results show that the energy consumption cost of the proposed microwave plasma enhancing hydrogen storage system is approximately 1.71 $/kgH2, which is about 50% of the energy consumption cost of the conventional system.
Introduction
In recent years, the low-carbon process of energy has attracted national strategic attention (Hepburn et al., 2021; He et al., 2022). Hydrogen has been widely popular as a potential alternative energy source. Among the hydrogen energy full chain, hydrogen storage and transportation technology are the key points to realizing the commercial application of hydrogen energy (Ding et al., 2022). Among various hydrogen storage methods, the solid-state hydrogen storage method could realize high-capacity storage of hydrogen by reversible adsorption/desorption of solid adsorption materials.
Figure 1 indicates the key role of hydrogen in the renewable energy system. The hydrogen sources from many kinds of renewable energy include wind energy, solar energy, nuclear energy, and others. Green hydrogen is produced by electrolyzation of water (Song et al., 2021), using an alkaline electrolyzer, proton exchange membrane (PEM) electrolyzer (Wang et al., 2021), or solid oxide electrolysis cell (SOEC). Hydrogen is transported by vehicle carriers and stored through high-pressure storage, low-temperature storage, and solid-state storage. Soon afterward, hydrogen needs to be used to supply power residents live and industry. The released hydrogen from storage materials is returned to the fuel cell to generate electricity and heat. It is a benefit for the continuous operation of the renewable energy system to store energy through hydrogen media. At the same time, hydrogen also realizes the recycling when accompanying renewable energy utilization.
MgH2 is regarded as one of the most promising solid hydrogen storage materials due to its high hydrogen storage density, abundant resource, low cost, high safety, excellent reversible cycle, long life, and mature preparation process (Hirscher et al., 2020). The reaction enthalpy change could even reach as high as 85 kJ/mol H2 (Bogdanović et al., 1999). The operating temperature is too high. For example, the dehydrogenation temperature is as high as 350°C (Reiser et al., 2000), which is much higher than the vehicle-mounted hydrogen release temperature requirement of the U.S. Department of Energy (DOE) (lower than 85°C) (Department of Energy and United States of America, 2017). The ideal hydrogen storage process is that the reaction process has a low reaction temperature and fast kinetic characteristics.
Actually, a lot of efforts have been made to improve the Mg-based hydrogen storage performance by nanocrystallization, element doping, catalytic modification, and other hydrogen storage methods. Zaluska et al. (Zaluski et al., 1997) found that the hydrogen storage capacity and kinetic properties will be significantly improved when the grain size is reduced to 50 nm. Zhu et al. (Cao et al., 2015) doped In, Al, and Ti elements into Mg-based hydrogen storage materials. The reaction enthalpy is changed by the affinity of doped elements. As a result, the reaction enthalpy is reduced to 65.2 kJ/mol H2. In addition, Shaw et al. (Zhong et al., 2016) formed Li2B12H12 intermediate product and changed the reaction path of MgH2 to achieve reversible hydrogen absorption and desorption at 150°C by compositing MgH2, LiBH4, phase and C powder. However, these methods cannot simultaneously guarantee the high performance of thermodynamics and kinetics. The improvement of thermodynamics is often at the expense of kinetics and hydrogen storage. The Mg4NiPd alloy with a BCC-based CsCl-type structure could achieve hydrogen storage at near room temperature but with the hydrogen capacity of 0.7 wt% only (Edalati et al., 2018). Therefore, it is significant and essential to developing a new hydrogen storage approach with good thermal and kinetic properties and also low energy consumption.
Recently, attention in different fields of chemistry has been increasingly paid to using microwave energy to improve reaction properties. Omran (Omran et al., 2020) investigated the effect of microwave energy on the carbothermic reduction of zinc oxide. It was found that the required temperature is reduced from 950°C to 550°C. This indicates that microwave energy has the potential for improving the thermodynamic performance of the reaction process. In addition, microwave plasma is often used as an effective auxiliary way to enhance mass transfer and activate reactions due to its strong penetration, non-contact effect, and various special effects caused by highly active plasma. This is because that microwave plasma can stimulate the reaction substances to produce plasma containing rich active particles, which is especially suitable for adverse reactions of thermodynamics or kinetics. For example, ammonia synthesis generally requires high temperature and pressure, which inevitably results in large energy consumption and a poor economy. In Kunimori’s scheme, the nature of plasma is used to atomize nitrogen (Kunimori et al., 1992). The high-energy particles and catalysts interact with each other with the help of plasma to improve the reaction kinetics by the E-R (Eley-Rideal) mechanism. Accordingly, the ammonia synthesis with the increase of ammonia yield by 6.5 times can be obtained at room temperature and atmospheric pressure (Hong et al., 2018). Eun et al. (Lee et al., 2019) used microwave plasma to remove the solvent that coordinates and fills the gaps in metal-organic frameworks (MOF) and finally achieved a good activation effect. It takes only 30 min to complete the whole activation process, which greatly improves efficiency. In the reaction of methane catalytic conversion to hydrocarbon and hydrogen, microwave plasma forms highly active free radicals by interacting with the catalyst surface, thus changing the theoretical reaction path, but the actual energy efficiency is only 5.5% (Suib and Zerger, 1993). These facts show that microwave plasma enables to strengthen the chemical reaction process by virtue of its own heating effect, plasma effect, and other properties.
In this article, the effect of microwave plasma on improving the thermodynamic performance of Mg-based hydrogen storage is investigated by using density functional theory (DFT) calculations. The activation mechanism of microwave plasma on Mg-based hydrogen storage is uncovered. In addition, the economic feasibility of this kind of newly enhanced technology is evaluated based on the potential application of supplying hydrogen to solid oxide fuel cells (SOFC) for heat and power co-generation.
Principles of Microwave Plasma
In recent years, there are more and more research focusing on a microwave-assisted method to enhance chemical reactions. Microwave is a kind of non-ionic radiation energy. It is generally believed that, under the action of microwave, the energy levels of polar molecules are matched with the rotational energy levels of polar molecules. The microwave promotes the polar conversion movement of substances, including the rapid rotation of molecules, the fracture of chemical bonds, and the friction and collision between the molecules, thus increasing the energy of activated molecules. As a result, the reaction activity of substances is greatly improved (Sun et al., 2016). Owing to the special “plasma effect” and “discharge effect”, microwave-induced metal discharge technology has been widely concerned (Chehade et al., 2020). When the metal satisfies certain conditions, the electromagnetic field produced by the microwave enriches the charge around the material. When the charge reaches a certain strength, it will produce electric discharge and break down the ambient gas, showing the “discharge effect” and “plasma effect”. Therefore, the microwave plasma has the characteristics of high ionization degree and high energy density. This contributes to producing active components which have a certain catalytic potential for chemical reactions. The active components are even able to trigger physical and chemical reactions that could not be completed under the normal operating conditions. That’s to say, microwave plasma enables to stimulate the active media of chemical reaction and to self-produces the heat in the meanwhile. By comparison, for the common methods, the catalyst is always used as active media to accelerate the reaction. However, auxiliary heating equipment should be used to provide heat energy.
The plasma effect requires that the working fluid can be ionized at least. In fact, hydrogen is the best easily ionized or broken-down gas except for inert helium and argon. The required energy for ionizing hydrogen is also small due to the low dielectric strength of only 0.5 MV/m. The dielectric strength coefficient of common working fluids is summarized in Table 1. Moreover, the electron escape ability from Mg metal is not high. The work function of the electron from the Mg element is about 3.66 eV, which is lower than those from transition metals such as Fe, Cu, Ni, and so on. That is to say, the electron is easy escape from the metal surface of Mg after external stimulation.

Table 1. Comparison table of dielectric strength of common working fluids (dielectric strength referring to nitrogen in gas measurement).
In this article, two kinds of MgH2 hydrogen storage processes are analyzed. One is a conventional heating hydrogen storage process based on MgH2 (marked as conventional heating/MgH2 thereinafter) with a conventional heat source as reaction energy. The other one is microwave enhanced advanced hydrogen storage process based on MgH2 (marked as MW/MgH2 -AHSP thereinafter).
Figure 2 shows the schematic diagram of a solid-state hydrogen storage system with conventional heat source as reaction energy (conventional heating/MgH2 system). Figure 3 illustrates the schematic diagram of solid-state hydrogen storage system with microwave as the auxiliary approach. This system is MW/MgH2 -AHSP system. Both of the hydrogen storage systems are connected to SOFC as terminal applications of hydrogen. The heat of the conventional heating/MgH2 system is mainly supplied by boiler steam, while the microwave hydrogen storage system is supplied by microwave energy. At the same time, due to the special effect of microwaves, a large number of plasma containing highly active radicals, ions, and molecules are produced. In addition, plasma can regulate the gas velocity, making the most reactions involving gas media work. These advantages usually lead to the reduction of reaction time, which is also beneficial to reducing reactor size and manufacturing cost.
The process of hydrogen storage assisted by microwave plasma is shown in three basic stages in this model:
(1) Microwave generated by controlling magnetrons
(2) The propagation of microwave
(3) Ionizing hydrogen in the Mg/MgH2 system, inducing the formation of H− ion
In the second process, due to the special nature of the microwave, the material of the reactor that transmits the microwave must be guaranteed to make the microwave easy to penetrate. The reactor material is suggested to use ceramics, plastics, and glass with a small dielectric loss coefficient, which contributes to minimizing microwave losses.
In the third process, appropriate high-frequency electromagnetic fields can ionize the reaction medium, and produce high-energy electrons, activated H atoms, hydrogen ions, and other high-energy particles.
Thermodynamic Performance Evaluation
DFT Calculations
Theoretical calculation based on DFT is widely used to study the reaction mechanism of the hydrogen storage system. Because this study involves microwave field and H− ion, it is difficult to carry out experiments at this stage. Therefore, the crystal structure and energy change of the reaction system are calculated by simulation calculation in the first place. Based on the DFT calculations, the change in thermodynamic properties of the reaction before and after the introduction of the microwave field could be deduced. It is essential to study the activation mechanism of microwave plasma to improve the thermodynamics of Mg-based hydrogen storage from the theoretical aspect.
Before the calculations of energy and properties, the geometry optimization of the unit cell should be first performed to achieve the most stable structure of the crystal. The projection augmented wave method (PAW) was employed to describe the interaction between ions and electrons in the simulation calculation. The iteration stops when the force on all atoms during the ion relaxation process is less than 0.02 eV
As for free hydrogen molecules, the gas molecule is put into a huge cubic unit cell (a = b = c = 10 Å) under the periodic boundary condition for isolating these molecules. As a result, the interaction between different unit cells of the H2 molecules could be ignored. Moreover, the Monkhorst-Pack mesh k-point for the free gas molecules was set as 3 × 3×3.
The structure models of magnesium and magnesium hydride are constructed as shown in Figure 4. Figure 4A is the crystal structure of Mg, whose space group is P1(#1–1) with the lattice constant of a = b = c = 3.186 Å, α = β = γ = 60°. Figure 4B shows the crystal structure model of magnesium hydride with 2 Mg atoms and 4 H atoms in the cell, whose space group is P42/mn (#136–1) with lattice constant of a = b = 4.517 Å, c = 3.02 Å, α = β = γ = 90°.
Comparison of Thermodynamic Properties
Mg and hydrogen molecular generally react in the process of storage as shown in the following equation:
The reaction enthalpy change (
where

Table 2. Energy of species involved in the reaction (Park et al., 2009).
As is well known, the reaction could happen and reach the equilibrium when the Gibbs free energy change is ∆G = 0. The relationship between ∆H and reaction temperature T is expressed in the following equation:
The corresponding value of reaction entropy change ∆S is 130 J/(mol∙K) (Bogdanović et al., 1999). As a result, the reaction temperature of conventional Mg-based hydrogen storage without the microwave plasma is calculated to be about 339°C, which is also close to the reported reaction temperature of 350°C (Bogdanović et al., 1999). The calculation error is approximately 3.2%, indicating that the prediction results by DFT are reasonable to evaluate the thermodynamic properties of the MgH2 hydrogen storage system.
Figure 5 shows the detailed mechanism of the MW/MgH2 -AHSP system. When the microwave works, the reactant is stimulated to produce high-energy electrons and other high-activity particles. Next, high-energy electrons in the plasma collide with the vibration-state hydrogen molecules, thus producing the excited H− ions and H atoms (Janev et al., 1987). The produced H atoms further capture low-energy electrons in plasma to generate H− ions. That is to say, H− ions are provided in two ways. Finally, H− ions spread along the gap in the lattice, and combine with Mg2+ ions freely.
In this case, the hydrogen storage in plasma is channeled by vibrational and electron excitation as follows:
Microwave irradiates on nano-scale magnesium particles with sharp tips, giving rise to a large energy focus in the microwave region. At the same time, the nano-scale surface roughness further enhances the plasma effect, making Mg convert into Mg2+ and releasing active electrons. Subsequently, H2 and H are ionized as H− via vibrational and active electron excitation.
That is to say, the active components produced in the atmosphere of microwave plasma change the reaction path. Accordingly, the overall reaction equation under microwave plasma is deduced as follows:
where e is a low-energy electron and EA is external energy to overcome energy potential barriers, whose value is 0.75 eV. Species activated by microwave plasma require different energy levels to overcome the different barriers of ion hop during diffusion. Furthermore, when electrons combine with the hydrogen atom, the surface electrons need to break through a potential barrier. It is considered that microwave provides energy (EA) to overcome this potential barrier.
The reaction enthalpy
It is noteworthy that the energy of the low-energy electron is assumed to be -0.1 eV (Janev et al., 1987). In addition, the hydrogen anion is difficult to obtain energy values directly by DFT calculations. Hence, the energy of hydrogen anion is calculated by the following formula:
where E(Hground-state) represents the ground state energy of hydrogen atom, Reference(H) is the value of H in POTCAR file, which is calculated as -1.96 eV.
As a result, the reaction enthalpy
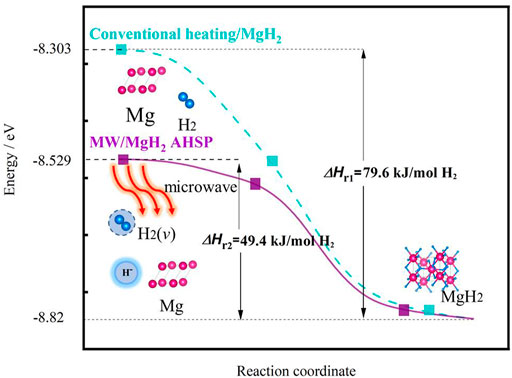
Figure 6. Reaction enthalpy comparison between Mg-based hydrogen storage with and without the microwave plasma.
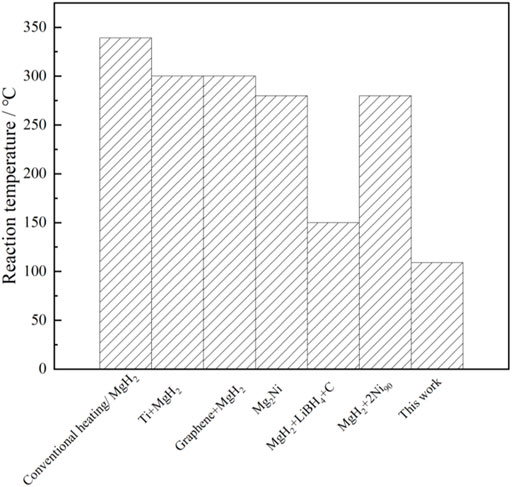
Figure 7. Hydrogen storage temperature of different enhancing methods (Hanada et al., 2005; Yang et al., 2010; Setijadi et al., 2013; Cho et al., 2016; Zhong et al., 2016)
Economic Feasibility Evaluation
Comparison of Energy Consumption
Based on the previous analysis, it can be seen that a high ionization and dissociation are needed to ensure the production of active particles. It was reported the microwave power of 6 kW is stable for methane transformation (Zamri et al., 2021). Since the dielectric constant of hydrogen is 0.5 MW/m only, which is much lower than that of methane, hydrogen will be more easily ionized by microwave to produce plasma, so the microwave power of 5 kW is supposed to be enough for completing the reaction of producing high-energy hydrogen species.
In the Mg-based hydrogen storage system, the supply of heat is indispensable for the release of hydrogen. Therefore, it is necessary to provide thermal energy for the whole system with the help of external heat sources. Generally, heat is generated by steam via the boiler. Such heat sources need a long-term operation to ensure the stability of the reaction. Usually, the annual operation number is about 8,000 h. By comparison, the process of microwave plasma activating reaction does not require so much time. Specific time is ascertained based on reaction systems, microwave power, and other factors in experiments.
Therefore, it is hard to accurately calculate the reaction time. Some systems need several minutes (Ricard et al., 2001), while some need a few hours (Wongjaikham et al., 2021). But it is certain that the microwave plasma takes much less time than the conventional heating type. In a project funded by DOE, a pulsed microwave catalytic reactor was mentioned, which did not continuously supply energy. Only when the catalyst and reaction need an energy supply, does the microwave work. It was concluded that the pulsed microwave reactor almost doubles the reaction conversion and the product yield (Hu, 2018). This shows that the microwave can achieve a good cost-performance ratio of input to output.
In addition, the microwave promotes the generation of plasma owing to its own thermal effect. The existence of hot spots caused by microwave plasma makes the reactants vulnerable to activation. Microwave energy can be selectively transmitted between the active site and the reaction intermediate, and will not lose to the surrounding environment, thus improving the energy utilization efficiency (Tiwari et al., 2020).
The hydrogen storage system mentioned in this article is a magnesium hydride reactor coupled with a 300 kW SOFC for a heat and power co-generation system. The difference is that the conventional heating/MgH2 system employs the boiler steam heated by natural gas or coal as the external heat source to provide energy for the whole reaction process. However, the microwave plasma is employed to activate and heat the hydrogen storage reaction as the external energy supply. On one hand, the thermal effect of microwave is used to make the reactants quickly reach the reaction temperature. On the other hand, the generated plasma activates the hydrogen ion and improves the reaction thermodynamics.
In the following analysis, system 1 represents the conventional hydrogen storage system without microwave plasma (conventional heating/MgH2 system), while system 2 is the proposed microwave plasma activating hydrogen storage system (MW/MgH2 -AHSP system). For the two reactor systems, it is assumed that the target hydrogen production is 100 kg. The hydrogen production rate of the magnesium hydride reactor is 40 NL/min (de Rango et al., 2016). The steam boiler generating hot fluid by combustion of natural gas fuel (0.33 $/m3) is used to supply energy for system 1. The efficiency of combustion converting to heat is usually 30%. The energy supply of generating microwave for system 2 is consuming electric energy with the price of 0.15 $/kWh. The reaction time of the pulse microwave reactor is assumed to be half of the conventional reaction time.
Figure 8 shows the comparison of energy consumption between conventional heating/MgH2 system and MW/MgH2 -AHSP system when coupling with SOFC for heat and power co-generation. It can be seen that the energy consumption cost per unit hydrogen mass of system 1 is approximately3.20 $/kg H2, which is almost twice as large as that of system 2 (1.71 $/kg H2). The comparison indicates that the microwave is cost-optimal and energy-saving for the large-scale hydrogen storage system.
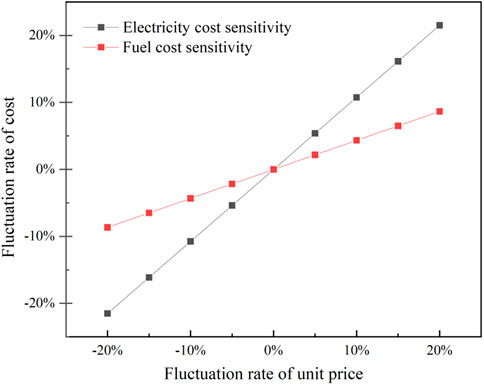
Figure 8. Sensitivity analysis of energy consumption cost with the fluctuation of electricity and fuel price.
Sensitivity Analysis
Sensitivity analysis is further carried out with the change of electricity unit price and natural gas fuel unit price as the conditions. Assuming that the fluctuation range of fuel unit price is −20%∼+20%, the corresponding change range of total fuel cost is −8.7%∼ +8.7%. For the same fluctuation range of unit price of electricity, the total electricity cost changes from −21% to +21%. By comparison, the sensitivity of fuel or electricity price on the energy consumption cost of systems 1 and 2 could be illustrated as shown in Figure 9. It can be seen that the electricity price is more sensitive for system 2 compared with the fuel price for system 1. Therefore, for the application of microwave plasma enhancing hydrogen storage systems, the decision-makers should pay attention to the impact of the fluctuation of electricity prices on the economic benefits of the project.
Conclusion
In this article, microwave plasma is introduced to enhance Mg-based hydrogen storage performance. The hydrogenation reaction enthalpy of the MW/MgH2 –AHSP system is about 49.4 kJ/mol H2, which is much lower than the value of 79.6 kJ/mol H2 of the conventional heating/MgH2 system. Accordingly, the reaction temperature is significantly reduced from the initial 339°C–109°C. Compared with the previously reported other enhancing methods, the reaction temperature of microwave plasma activating Mg-based hydrogen storage is also the lowest. Moreover, the economic feasibility of this kind of newly enhanced technology is evaluated based on the potential application of supplying hydrogen to SOFC for heat and power co-generation. The energy consumption cost is only 1.71 $/kg H2, which is nearly half of the energy consumption cost without the microwave plasma. These results show that the proposed microwave plasma activating Mg-based hydrogen storage is feasible and promising to achieve the low-energy-consumption solid-state hydrogen storage in the future.
Data Availability Statement
The original contributions presented in the study are included in the article/Supplementary Material; further inquiries can be directed to the corresponding author.
Author Contributions
HW: simulation operation, investigation, and writing—original draft. HY: model development and investigation. JR: investigation. BL: review and editing. SNN: review and editing. ZW: conceptualization, supervision, and writing—review and editing.
Funding
This work was financially supported by the National Natural Science Foundation of China (Nos. 52176203 and 21736008), the Natural Science Foundation Project of Shaanxi Province (No. 2021JQ-890), the Young Talent Fund for Science and Technology in Xi’an City, China (No. 095920211329), and the Guangdong Provincial Key Laboratory of Distributed Energy Systems (No. 2020B1212060075).
Conflict of Interest
The authors declare that the research was conducted in the absence of any commercial or financial relationships that could be construed as a potential conflict of interest.
Publisher’s Note
All claims expressed in this article are solely those of the authors and do not necessarily represent those of their affiliated organizations, or those of the publisher, the editors, and the reviewers. Any product that may be evaluated in this article, or claim that may be made by its manufacturer, is not guaranteed or endorsed by the publisher.
References
Bogdanović, B., Bohmhammel, K., Christ, B., Reisera, A., Schlichtea, K., Vehlena, R., et al. (1999). Thermodynamic Investigation of the Magnesium–Hydrogen System. J. Alloys Compd. 282 (1), 84–92.
Cao, Z., Ouyang, L., Wu, Y., Wang, H., Liu, J., Fang, F., et al. (2015). Dual-tuning Effects of in, Al, and Ti on the Thermodynamics and Kinetics of Mg 85 in 5 Al 5 Ti 5 Alloy Synthesized by Plasma Milling. J. Alloys Compd. 623, 354–358. doi:10.1016/j.jallcom.2014.10.200
Chehade, G., Lytle, S., Ishaq, H., and Dincer, I. (2020).Hydrogen Production by Microwave Based Plasma Dissociation of Water. Fuel 264, 116831. doi:10.1016/j.fuel.2019.116831
Cho, E. S., Ruminski, A. M., Aloni, S., Liu, Y. S., Guo, J., and Urban, J. J. (2016). Graphene Oxide/metal Nanocrystal Multilaminates as the Atomic Limit for Safe and Selective Hydrogen Storage. Nat. Commun. 7, 10804–10808. doi:10.1038/ncomms10804
de Rango, P., Marty, P., and Fruchart, D. (2016). Hydrogen Storage Systems Based on Magnesium Hydride: from Laboratory Tests to Fuel Cell Integration. Appl. Phys. A 122 (2), 126. doi:10.1007/s00339-016-9646-1
Department of Energy, United States of America (2017). Target Explanation Document: Onboard Hydrogen Storage for Light-Duty Fuel Cell Vehicles. Available at: https://www.energy.gov/eere/fuelcells/downloads/target-explanation-document-onboard-hydrogen-storage-light-duty-fuel-cell.
Ding, X., Chen, R., Zhang, J., Cao, W., Su, Y., and Guo, J. (2022). Recent Progress on Enhancing the Hydrogen Storage Properties of Mg-Based Materials via Fabricating Nanostructures: A Critical Review. J. Alloys Compd. 897, 163137. doi:10.1016/j.jallcom.2021.163137
Edalati, K., Uehiro, R., Ikeda, Y., Li, H.-W., Emami, H., Filinchuk, Y., et al. (2018). Design and Synthesis of a Magnesium Alloy for Room Temperature Hydrogen Storage. Acta Mater. 149, 88–96. doi:10.1016/j.actamat.2018.02.033
Hanada, N., Ichikawa, T., and Fujii, H. (2005). Catalytic Effect of Ni Nano-Particle and Nb Oxide on H-Desorption Properties in MgH2 Prepared by Ball Milling. J. Alloys Compd. 404-406, 716–719. doi:10.1016/j.jallcom.2004.12.166
He, J., Li, Z., Zhang, X., Wang, H., Dong, W., Du, E., et al. (2022). Towards Carbon Neutrality: A Study on China's Long-Term Low-Carbon Transition Pathways and Strategies. Environ. Sci. Ecotechnology 9, 100134. doi:10.1016/j.ese.2021.100134
Hepburn, C., Qi, Y., Stern, N., Ward, B., Xie, C., and Zenghelis, D. (2021). Towards Carbon Neutrality and China's 14th Five-Year Plan: Clean Energy Transition, Sustainable Urban Development, and Investment Priorities. Environ. Sci. Ecotechnology 8, 100130. doi:10.1016/j.ese.2021.100130
Hirscher, M., Yartys, V. A., Baricco, M., Bellosta von Colbe, J., Blanchard, D., Bowman, R. C., et al. (2020). Materials for Hydrogen-Based Energy Storage - Past, Recent Progress and Future Outlook. J. Alloys Compd. 827, 153548. doi:10.1016/j.jallcom.2019.153548
Hong, J., Prawer, S., and Murphy, A. B. (2018). Plasma Catalysis as an Alternative Route for Ammonia Production: Status, Mechanisms, and Prospects for Progress. ACS Sustain. Chem. Eng. 6 (1), 15–31. doi:10.1021/acssuschemeng.7b02381
Hu, J. L. (2018). “Direct Non-oxidative Conversion of Shale Gas to Chemicals: Catalyst Selective Activation, Regeneration, and Process Intensification,” in Abstr Pap Am Chem S (Washington, DC : Amer. Chemical Soc.).
Janev, R. K., Langer, W. D., Evans, K., and Post, D. E. (1987). Elementary Processes in Hydrogen-Helium Plasmas. Cross Sections and Reaction Rate Coefficients. viii+326.
Kunimori, K., Osumi, M., Kameoka, S., and Ito, S. (1992). Plasma-induced Nitrogen Chemisorption on a Ruthenium Black Catalyst: Formation of NH3 by Hydrogenation of the Chemisorbed Nitrogen. Catal. Lett. 16 (4), 443–446. doi:10.1007/bf00764903
Lee, E. J., Bae, J., Choi, K. M., and Jeong, N. C. (2019). Exploiting Microwave Chemistry for Activation of Metal-Organic Frameworks. ACS Appl. Mat. Interfaces 11 (38), 35155–35161. doi:10.1021/acsami.9b12201
Omran, M., Fabritius, T., Heikkinen, E.-P., Vuolio, T., Yu, Y., Chen, G., et al. (2020). Microwave Catalyzed Carbothermic Reduction of Zinc Oxide and Zinc Ferrite: Effect of Microwave Energy on the Reaction Activation Energy. RSC Adv. 10 (40), 23959–23968. doi:10.1039/d0ra04574h
Park, M. S., Janotti, A., and Van de Walle, C. G. (2009). Formation And Migration of Charged Native Point Defects in MgH2: First-Principles Calculations. Phys. Rev. B 80 (6), 064102. doi:10.1103/physrevb.80.064102
Reiser, A., Bogdanovic, B., and Schlichte, K. (2000). The Application of Mg-Based Metal-Hydrides as Heat Energy Storage Systems. Int. J. Hydrogen Energy 25 (5), 425–430. doi:10.1016/s0360-3199(99)00057-9
Ricard, A., Gaillard, M., Monna, V., Vesel, A., and Mozetic, M. (2001). Excited Species in H2, N2, O2 Microwave Flowing Discharges and Post-discharges. Surf. Coatings Technol. 142-144, 333–336. doi:10.1016/s0257-8972(01)01276-2
Setijadi, E. J., Boyer, C., and Aguey-Zinsou, K.-F. (2013). MgH2 with Different Morphologies Synthesized by Thermal Hydrogenolysis Method for Enhanced Hydrogen Sorption. Int. J. Hydrogen Energy 38 (14), 5746–5757. doi:10.1016/j.ijhydene.2013.02.128
Song, Y., Ji, K., Duan, H., and Shao, M. (2021). Hydrogen Production Coupled with Water and Organic Oxidation Based on Layered Double Hydroxides. Exploration 1 (3), 20210050. doi:10.1002/exp.20210050
Suib, S. L., and Zerger, R. P. (1993). A Direct, Continuous, Low-Power Catalytic Conversion of Methane to Higher Hydrocarbons via Microwave Plasmas. J. Catal. 139 (2), 383–391. doi:10.1006/jcat.1993.1034
Sun, J., Wang, W., Yue, Q., Ma, C., Zhang, J., Zhao, X., et al. (2016). Review on Microwave-Metal Discharges and Their Applications in Energy and Industrial Processes. Appl. Energy 175, 141–157. doi:10.1016/j.apenergy.2016.04.091
Tiwari, S., Caiola, A., Bai, X., Lalsare, A., and Hu, J. (2020). Microwave Plasma-Enhanced and Microwave Heated Chemical Reactions. Plasma Chem. Plasma Process 40 (1), 1–23. doi:10.1007/s11090-019-10040-7
Wang, L., Liu, T., Wu, T., and Lu, J. (2021). Exploring New Battery Knowledge by Advanced Characterizing Technologies. Exploration 1 (3), 20210130. doi:10.1002/exp.20210130
Wongjaikham, W., Kongprawes, G., Wongsawaeng, D., Ngaosuwan, K., Kiatkittipong, W., Hosemann, P., et al. (2021). Highly Effective Microwave Plasma Application for Catalyst-free and Low Temperature Hydrogenation of Biodiesel. Fuel 305, 121524. doi:10.1016/j.fuel.2021.121524
Wu, Z., Zhu, L. Y., Zhang, Z. X., Yang, F. S., and Wang, Y. Q. (2018). DFT Insights into the Interactive Effect of Ni + N Cosubstitution on Enhanced Dehydrogenation Properties of Mg(BH4)(NH2)-like Complex Hydride for Hydrogen Energy Storage. J. Phys. Chem. C 122 (11), 5956–5966. doi:10.1021/acs.jpcc.8b00963
Yang, W. N., Shang, C. X., and Guo, Z. X. (2010). Site Density Effect of Ni Particles on Hydrogen Desorption of MgH2. Int. J. Hydrogen Energy 35 (10), 4534–4542. doi:10.1016/j.ijhydene.2010.02.047
Zaluski, L., Zaluska, A., and Ström-Olsen, J. O. (1997). Nanocrystalline Metal Hydrides. J. Alloys Compd. 253-254, 70–79. doi:10.1016/s0925-8388(96)02985-4
Zamri, A. A., Ong, M. Y., Nomanbhay, S., and Show, P. L. (2021). Microwave Plasma Technology for Sustainable Energy Production and the Electromagnetic Interaction within the Plasma System: A Review. Environ. Res. 197, 111204. doi:10.1016/j.envres.2021.111204
Zhong, Y., Wan, X., Ding, Z., and Shaw, L. L. (2016). New Dehydrogenation Pathway of LiBH4 + MgH2 Mixtures Enabled by Nanoscale LiBH4. Int. J. Hydrogen Energy 41 (47), 22104–22117. doi:10.1016/j.ijhydene.2016.09.195
Nomenclature
Abbreviation
PEM Proton exchange membrane
SOEC Solid oxide electrolysis cell
DOE U.S. Department of Energy
E-R mechanism Eley-Rideal mechanism
MOF Metal-organic frameworks
DFT Density functional theory
SOFC Solid oxide fuel cell
Conventional heating hydrogen storage process based on MgH2 Conventional heating/MgH2
Microwave enhanced advanced hydrogen storage process based on MgH2 MW/MgH2-AHSP
PAW Projection augmented wave
Symbols
∆G The change of the free Gibbs energy
T Reaction temperature
EA The external energy
Subscripts
r1 The conventional hydrogen storage reaction
r2 Microwave plasma enhancing hydrogen storage reaction
ground-state Ground state
Keywords: hydrogen storage, magnesium hydride, thermodynamics, microwave plasma, reaction temperature
Citation: Wang H, Yan H, Ren J, Li B, Nyallang Nyamsi S and Wu Z (2022) Microwave Plasma Enhancing Mg-Based Hydrogen Storage: Thermodynamics Evaluation and Economic Analysis of Coupling SOFC for Heat and Power Generation. Front. Therm. Eng. 2:886322. doi: 10.3389/fther.2022.886322
Received: 28 February 2022; Accepted: 09 May 2022;
Published: 23 June 2022.
Edited by:
Dibakar Rakshit, Indian Institute of Technology Delhi, IndiaReviewed by:
Davide Papurello, Politecnico di Torino, ItalyPeng Tan, University of Science and Technology of China, China
Copyright © 2022 Wang, Yan, Ren, Li, Nyallang Nyamsi and Wu. This is an open-access article distributed under the terms of the Creative Commons Attribution License (CC BY). The use, distribution or reproduction in other forums is permitted, provided the original author(s) and the copyright owner(s) are credited and that the original publication in this journal is cited, in accordance with accepted academic practice. No use, distribution or reproduction is permitted which does not comply with these terms.
*Correspondence: Zhen Wu, d3V6MjAxNUBtYWlsLnhqdHUuZWR1LmNu