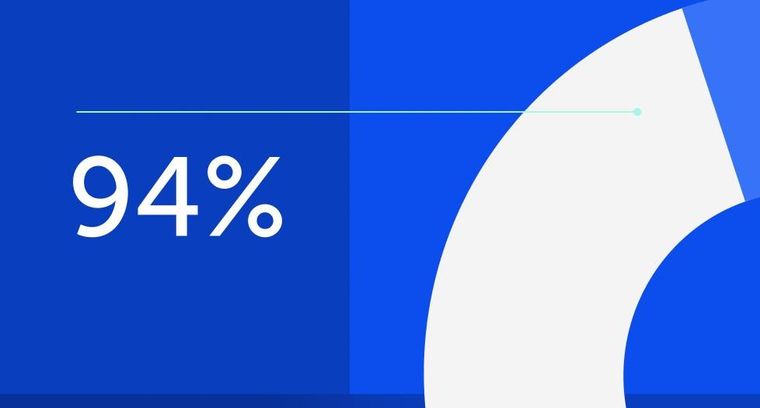
94% of researchers rate our articles as excellent or good
Learn more about the work of our research integrity team to safeguard the quality of each article we publish.
Find out more
REVIEW article
Front. Syst. Neurosci., 02 May 2023
Volume 17 - 2023 | https://doi.org/10.3389/fnsys.2023.1145643
This article is part of the Research TopicHorizons in Systems Neuroscience 2022View all 10 articles
Perception and action are fundamental processes that characterize our life and our possibility to modify the world around us. Several pieces of evidence have shown an intimate and reciprocal interaction between perception and action, leading us to believe that these processes rely on a common set of representations. The present review focuses on one particular aspect of this interaction: the influence of action on perception from a motor effector perspective during two phases, action planning and the phase following execution of the action. The movements performed by eyes, hands, and legs have a different impact on object and space perception; studies that use different approaches and paradigms have formed an interesting general picture that demonstrates the existence of an action effect on perception, before as well as after its execution. Although the mechanisms of this effect are still being debated, different studies have demonstrated that most of the time this effect pragmatically shapes and primes perception of relevant features of the object or environment which calls for action; at other times it improves our perception through motor experience and learning. Finally, a future perspective is provided, in which we suggest that these mechanisms can be exploited to increase trust in artificial intelligence systems that are able to interact with humans.
At the basis of a successful behavior there is the interplay between perception and action. Typically, perception informs the action mechanism regarding the features of the environment and this mechanism is responsible for changes in the environment. If, on the one hand, it is doubtless that perception influences the action, the influence of action on perception cannot be taken for granted to the same extent. Starting from such a consideration, this review aims to examine the influence of action on visual perception of different properties of objects (i.e., size, orientation, and location) focusing on the actions performed by different motor effectors such as the eye, the hand, and the leg. Since two phases can be distinguished when looking at the influence of action on perception, namely planning and execution, in the following sections we provide a separate overview of some of the studies that explore the effect of action planning on the perception of the object/stimulus, and of those that examine action execution and its effect on perception.
As perceivers, we receive, on a daily basis, a wide variety of information concerning the features of the surrounding environment. As active players, we constantly explore this environment based on the sensory processing of those stimuli related to our goals/intentions and subsequent actions. For example, everyday tasks such as grasping a cup or the handle of a frying pan are highly precise actions that we perform automatically; however, these involve a complex sensorimotor approach, many aspects of which are still unknown.
An action, an intended and targeted movement, is distinguished by several sequential sections that organize its processing and work in close coordination with perception (Hommel et al., 2016). Within this processing (assessment of environmental information, location in three-dimensional space, and selection, integration, and initiation of the action), action planning represents a fundamental component (Hommel et al., 2016; Mattar and Lengyel, 2022).
Action planning is specified as a process which considers the execution of actions based on the environment and expected outcomes (Sutton and Barto, 1998; Mattar and Lengyel, 2022). Action planning could be referred to as the period between the decision phase and the initial impulse phase. During the action planning phase, the player generates an action goal (based on the temporal and spatial properties of the environment) which is then transferred to the motor system to achieve that specific purpose. That is, first the information is organized and subsequently integrated into a plan. This particularity provides plasticity and favors the adaptation to possible changes to the input information and goals (Mattar and Lengyel, 2022). For example, when grasping an object, it can be observed how the hand adjusts to the intrinsic properties of that object (Jeannerod, 1981), which hints at the relevance of action planning in the interaction between the environment and the final goal. In fact, input information is processed in parallel by pathways acting in a shared action-perception framework (Prinz, 1990; Hommel et al., 2001), within which planning itself has been observed to influence (Hommel et al., 2001, 2016; Witt, 2018).
Notwithstanding the considerable scientific literature on how planning contributes to cognitive processes, the current findings merely give us a glimpse of the long road ahead. Here, in the following sections, we outline the most important behavioral studies regarding the impact of action planning on perception.
Our visual system captures primordial information which guides our actions. Once the visual environment and objects of interest are defined, the visuo-spatial information is then transferred in order to plan, execute, and control those goal-directed actions (Hayhoe, 2017). The impact of vision on motor actions has always been a topic of great scientific interest (Prablanc et al., 1979; Desmurget et al., 1998; Land, 2006, 2009). Several decades ago, groundbreaking studies were already describing how vision improves goal-directed movement accuracy (Woodworth, 1899; Bernstein, 1967). Since then, subsequent studies have sought to investigate how vision influences planning, execution, and control of movements.
Visual information greatly contributes to the action planning phase. During planning, the presence of visual feedback regarding the limb is paramount. For example, motor actions are more accurate when visual feedback is provided during action planning, regardless of whether the limb is visible or not during the action (Prablanc et al., 1979; Conti and Beaubaton, 1980; Pelisson et al., 1986; Velay and Beaubaton, 1986; Elliott et al., 1991, 2014; Rossetti et al., 1994; Desmurget et al., 1995, 1997; Coello and Grealy, 1997; Bagesteiro et al., 2006; Bourdin et al., 2006; Sarlegna and Sainburg, 2009).
Indeed, vision plays a key role in action planning since movements are apparently planned as vectors based on the extrinsic coordinates of the visual environment (Morasso, 1981; Flanagan and Rao, 1995; Wolpert et al., 1995; Sarlegna and Sainburg, 2009). Once visual information has been extracted, planning should consider those properties that will shape further actions. An example may be that of driving a car and approaching an intersection. Our visual system extracts information regarding the location and movement of other cars, pedestrians, and traffic signals at the intersection. Based on the extrinsic coordinates of the visual environment, during action planning we determine the appropriate vectors for our movements, such as accelerating, braking, or turning, that allow us to navigate the intersection safely and efficiently.
In a common framework, two stages within action planning have been suggested: the primary stage, in which vision is fundamental to determine the visuo-spatial attributes (target, limbs, and environment), and the secondary stage, in which the primary input is transformed into motor commands to generate the action (Sarlegna and Sainburg, 2009). Therefore, in a normal context, during action planning, vision provides the relevant information which facilitates the success of an action.
Acquiring visuo-spatial information would not be possible without eye movements. If a target falls in the peripheral visual field, eye movements assist in conveying the exact location of the target. Suppose we want to reach for an object. Considering the retinal spatial resolution, when a target of interest to be reached is identified, the region of highest retinal resolution should be focused on that target (Liversedge and Findlay, 2000; Land, 2006). To this end, before the reaching action begins, the eyes perform a saccadic movement toward the object and then fixate it constantly until it is reached by the hand. Within this brief scenario, the relevance of eye movements, which continuously support the coupling of vision and action, can be appreciated (Land, 2006, 2009; Hayhoe, 2017; de Brouwer et al., 2021).
Research into the interaction between the visual and motor systems has shown how the eyes constantly support and guide our actions in multiple dynamic tasks (Angel et al., 1970; Biguer et al., 1982; Pelisson et al., 1986; Land, 1992; Land et al., 1999; Neggers and Bekkering, 1999, 2000, 2001, 2002; Johansson et al., 2001; Patla and Vickers, 2003). For example, Land et al. (1999) demonstrated that eye movements are directed to those objects involved in our daily actions. In Neggers and Bekkering (1999, 2000, 2001, 2002) studies, a mechanism of gaze anchoring during hand actions was elegantly demonstrated. They observed that during reaching movements observers did not make saccadic movements toward another target until the hand had arrived at the target of interest. Similar findings were reported by Johansson et al. (2001). They instructed participants to reach and grasp a bar which they subsequently had to move while avoiding obstacles and finally attach to a switch. They reported that gaze fixation was focused on those points that were critical to the action. That is, eye movements continuously guided the action to grasp, navigate, and attach the object (Johansson et al., 2001). In other studies, it was shown that fixation patterns differ when an object is grasped or viewed passively (Vishwanath and Kowler, 2003; Brouwer et al., 2009). Both studies showed that during visualization, fixation patterns were focused on the object's center of gravity, whereas during grasping, fixation was affected by the contact zone of the index and thumb digits. Interestingly, Brouwer et al. (2009) observed that saccadic reaction times were slower in the grasping task as compared to the visualization task. This outcome reflects that the onset of eye movement was dependent on action planning, i.e., in those conditions in which the eye and hand participated in the same process.
The eye reaction time latencies relative to the action have already been reported in several studies (Bekkering et al., 1994, 1995; Lünenburger et al., 2000; Pelz et al., 2001; Hayhoe et al., 2003). Bekkering et al. (1994) measured eye and hand motor response latencies using single- and dual-task methodologies. Like Brouwer et al. (2009), and as can be appreciated in Figure 1, saccade reaction time latencies were highest in the dual approach, i.e., when both the eye and hand simultaneously moved toward the visual target. Hand latencies were similar in both the single and dual tasks (Bekkering et al., 1994). Conversely, in another study, lower saccadic reaction time latencies were reported when the eye and hand moved simultaneously toward a common target (Lünenburger et al., 2000). Perhaps the type of planned action (pointing, reaching, grasping, etc.) is decisive within this interference effect. Longer processing times may be required according to the type of action planned, and, thus, eye reaction times could be affected differently (Brouwer et al., 2009). These findings demonstrate that these motor systems (eye-limb) are not independent from each other, and that they share synergistic processes when targeted to the same goal.
Figure 1. Averaged latencies of eye and hand responses, measured in milliseconds, under both experimental conditions: single- and dual-task. Modified from Bekkering et al. (1994).
Recent studies have revealed how eye movements support selection and action planning toward a goal. Particularly, exploration of the eye-limb relationship in naturalistic tasks has revealed how eye movements provide continuous information from the visual environment, generating a context of intrinsic properties and spatial coordinates during action planning to effectively guide future movements (Zelinsky et al., 1997; Land et al., 1999; Pelz et al., 2001; Brouwer et al., 2009). In tasks involving jar-opening or hand-washing it has been observed that reaching actions are preceded by anticipatory fixations toward the target of interest (Pelz et al., 2001; Hayhoe et al., 2003). These fixations occur during action planning and help the observer to obtain decisive spatial information to assist in the future action (Hayhoe et al., 2003). Other activities, such as walking or driving over difficult and tortuous surfaces, have shown how visuo-spatial information derived from eye movements is primordial during action planning (Land and Lee, 1994; Patla and Vickers, 2003; Land, 2006). For example, while walking, gaze fixation anticipates action by 0.8–1.1 s on average (Patla and Vickers, 2003; Land, 2006). This suggests that during planning, the visual system acts as an anticipatory system, in a feedforward manner, for the execution of the action.
Although previous studies focused on the role of the eyes as support in the planning of actions performed by other motor effectors, multiple studies have extensively examined the impact of eye motor planning on visual perception within the oculomotor system. These investigations have shown that spatial perception is enhanced at the location where the eye movement is intended to go, shortly before its execution (Hoffman and Subramaniam, 1995; Deubel and Schneider, 1996; Neggers et al., 2007). For example, research has shown that saccade target selection is influenced by object recognition (Deubel and Schneider, 1996), and that visual attention can influence the planning and execution of saccadic eye movements (Hoffman and Subramaniam, 1995). Additionally, a coupling between visuospatial attention and eye movements has been observed (Neggers et al., 2007), with attention often following the gaze. This coupling can be disrupted when transcranial magnetic stimulation is applied to the frontal eye fields, suggesting a causal relationship between attention and eye movements (Neggers et al., 2007). These outcomes may imply that the process of eye motor planning can have a significant impact on perception. Although the exact mechanisms underpinning this impact are not yet fully known, it is believed that the coordinated activity of multiple brain regions and systems, including the saccadic system, vestibular system, and attentional processes, is at play.
Over the past few decades, the scientific literature has provided compelling evidence as to how perception is biased by the planning of arm movements, such as reaching and grasping (Musseler and Hommel, 1997; Prinz, 1997; Craighero et al., 1999; Wohlschläger, 2000; Hommel et al., 2001; Knoblich and Flach, 2001; Wühr and Müsseler, 2001; Hamilton et al., 2004; Kunde and Wuhr, 2004; Fagioli et al., 2007; Wykowska et al., 2009, 2011; Kirsch et al., 2012; Kirsch and Kunde, 2013; Kirsch, 2015). From the perceiver's point of view, it is intriguing to consider the fact that when planning a reaching or grasping movement toward an object, the perception of the object is somehow influenced. For example, when reaching for a cup of coffee, the perceiver's visual system considers the cup's location and orientation relative to the perceiver's body. The perceived properties of the cup may also be influenced by the planned action, as the perceiver's motor system may need to make adjustments based on these properties in order to successfully grasp the cup. This suggests that the motor system is not only involved in executing actions, but also in shaping perception based on the perceiver's intended actions. In fact, multiple perceptual aspects, such as orientation, size, luminance, location, motion, among many others, have been reported as target features that are directly influenced by action planning (Musseler and Hommel, 1997; Craighero et al., 1999; Wohlschläger, 2000; Zwickel et al., 2007; Lindemann and Bekkering, 2009; Kirsch et al., 2012). For example, studies by Kirsch have shown how planning itself interferes with distance perception and, therefore, with target spatial location (Kirsch et al., 2012; Kirsch and Kunde, 2013; Kirsch, 2015).
This action(planning)-perception interaction is dependent on whether the goal is related or not to the action. When there is a direct relationship between goal and action, perception is facilitated by the planning of the action, whereas when the two are independent, action planning interferes with perception (Hommel et al., 2016).
Several benchmark studies carried out in the 1990s and 2000s demonstrated various scenarios exhibiting facilitation and interference. Based on a set of five experiments, Musseler and Hommel (1997) reported the impact of action planning on the direction perception of a visual stimulus. Direction perception (right or left) was influenced by action planning concurrence (right or left button press). Specifically, identifying the direction of a right-pointing stimulus was more costly after planning a right button press (Musseler and Hommel, 1997). Given the common code (Hommel et al., 2001), action planning toward a concrete direction led to an interference scenario, i.e., the share-code weighting favored action over perception (Musseler and Hommel, 1997; Hommel et al., 2016).
Subsequent studies corroborated the interaction between perception and action planning processes. In Wohlschläger (2000) study, observers had to report the perceived motion direction of projected discs while turning a knob in a designated direction. Hand motion direction biased subjects' motion perception. Under a similar experimental approach to that of Craighero et al. (1999), Lindemann and Bekkering (2009) instructed volunteers to reach, grasp, and subsequently rotate an x-shaped manipulandum following the visual go signal's onset. Here, a tilted bar (-45° or +45°) served as the visual go signal. Volunteers detected the onset of the go signal faster in the congruent conditions, in which the go signal, and action planning presented the same direction (Lindemann and Bekkering, 2009). These findings imply that perception was facilitated in the direction in which the action had been previously planned. In contrast, like Musseler and Hommel (1997), Zwickel et al. (2007) reported action (planning)-perception coupling but in an interference scenario. In their study, reaction times were longer when movement deviations agreed with the action planning direction (Zwickel et al., 2007). Interference situations have also been reported by other authors (Schubö et al., 2001; Hamilton et al., 2004; Zwickel et al., 2010), indicating that the action (planning)-perception coupling is dependent on whether the perceived target is linked or not to the planned action.
Recent research has proven the relevance of the type of action planning in how perception is biased (Bekkering and Neggers, 2002; Fagioli et al., 2007; Symes et al., 2008; Wykowska et al., 2009, 2011; Gutteling et al., 2011). Bekkering and Neggers (2002) instructed observers to point at or grasp an object with a specific orientation and color. The authors found that while color errors were identical in both approaches, the number of orientation errors was lower in the grasping scenario (Bekkering and Neggers, 2002). Gutteling et al. (2011) asked participants to perform a grasping or pointing movement simultaneously with an orientation or luminance discrimination task (see Figure 2). Orientation sensitivity increased when planning a grasping action, as opposed to a pointing action. Size, location, and luminance have also been described as being perceptually dependent attributes of the type of action planning (Fagioli et al., 2007; Wykowska et al., 2009, 2011; Kirsch et al., 2012; Wykowska and Schubö, 2012; Kirsch and Kunde, 2013). Fagioli et al. (2007) revealed that planning a grasping action improved the ability to detect deviations in object size, while planning a reaching action facilitated the detection of location deviations. Studies by Wykowska et al. (2009) and Wykowska and Schubö (2012) corroborated the finding that planning to grasp improves size perception, while planning to reach enhances luminance perception.
Figure 2. Effect of grasping and pointing planning on orientation and luminance detection. (A) Experimental approach. Experiments 1 and 2 used a similar stimulus display, which included a fixation spot and two bars. Participants were instructed to execute an action after a go-cue signaled by the appearance of the first bar. The second bar was either rotated slightly (Experiment 1) or differed in luminance (Experiment 2) from the first bar. (B) In Experiment 1, participants showed better orientation discrimination when planning a grasping action rather than when planning a pointing action. (C) Experiment 2 did not reveal any consistent change in luminance discrimination between grasping and pointing planning. Modified from Gutteling et al. (2011).
All the above-mentioned scientific evidence seems to support the common coupling of action(planning)-perception. Planning an action primes those perceptual dimensions that can enhance one's own action (Hommel et al., 2001; Wykowska et al., 2009).
The majority of studies cited have shown that the motor system dynamically modulates the incoming perceptual information. However, these modulations have been observed when the perceptual information is intermixed with attentional and decisional mechanisms because they are strictly related to the motor response (i.e., Gutteling et al., 2011). Relevant literature was dedicated to understanding the temporal tuning of incoming perceptual information at very early cortical stages. To do this, different studies measured the contrast sensitivity of a brief visual stimulus that was not correlated with the action to be performed, and that was presented at different times during motor planning and execution. These studies used contrast sensitivity because it represents and reflects the activity of the primary visual cortex, since it has been demonstrated that the change of contrast visibility requires a modulation at this early cortical level (Boynton et al., 1999). Furthermore, it has recently been demonstrated that both sensory and motor processes are regulated by a rhythmic process that reflects the oscillations of neuronal excitability (Buzsáki and Draguhn, 2004; Thut et al., 2012). Combining all these pieces of evidence, Tomassini et al. (2015) evaluated whether the rhythmic oscillations of visual contrast sensitivity were also present when synchronizing the perceptual information with the onset of a reaching and grasping movement. They found that the oscillations in contrast sensitivity emerged around 500 ms before movement onset, during action planning, even if perception was not related to the motor task (see Figure 3). These findings were extended in an electroencephalographic (EEG) study, in which the same group demonstrated that motor planning is combined with perceptual neural oscillations (Tomassini et al., 2017). The perceptual “action-locked” oscillations were also observed when the movements were performed with the eyes (Benedetto and Morrone, 2017; Benedetto et al., 2020). In this study, the results showed that saccadic preparation and visual contrast sensitivity oscillations are coupled, suggesting a functional alignment of the saccade onset with the visual suppression (Benedetto and Morrone, 2017).
Figure 3. Rhythmic oscillations of contrast sensitivity synchronized with hand movements. (A) Experimental setup of the motor and visual tasks. (B) Example of trial sequence. Visual noise and fixation point were presented from the beginning of the trial to the end. At a random time from the start of the trial, a Gabor stimulus was displayed to the lower right or to the lower left of fixation. (C) Time course of the orientation discrimination responses for each participant aligned with the onset of the hand movement. Modified from Tomassini et al. (2015).
The above-discussed research focuses on peripersonal space. However, it has been observed that the impact of action planning on perception can extend to the leg effector domain, resulting in facilitation effects on the perception of extrapersonal space. Several studies have shown that, when viewing objects in our extrapersonal space, we scale the perceived distance according to our intended motor action. For example, if we plan to walk a certain distance, we perceive the distance based on the amount of walking effort needed to traverse it, while, if we intend to throw a ball, the perceived distance is based on the amount of throwing effort required (Witt et al., 2004; Proffitt, 2006; Witt and Proffitt, 2008). The way we perceive our environment seems to be influenced by the specific actions we anticipate taking, with perception being adjusted based on an optimal cost-benefit principle (Proffitt, 2006). Recently, Fini et al. (2014, 2015a,b) used a virtual paradigm to investigate the influence of anticipated actions on spatial perception. Participants were asked to judge the location of an object positioned at progressively increasing or decreasing distances from a reference frame. They noticed that participants perceived the target object to be closer to their own body when they intended to move toward it compared to when they had no intention of moving. This effect was not observed when the target object was compared to another static object (Fini et al., 2015a). Additionally, studies have demonstrated that when leg actions such as walking or running are primed, the portion of extrapersonal space judged as near in other-based coordinates is significantly expanded (Fini et al., 2017), together with an extension of peripersonal space during full-body actions such as walking compared to standing (Noel et al., 2015). These findings suggest that visual perception of the physical environment beyond our body is heavily influenced by our actions, intentions, and physical abilities. Apparently, the main way of exploring the extended environment seems to be through locomotion, as it is the only way to cover distances and access information from more distant locations in the extrapersonal space compared to near extrapersonal locations, where information can be extracted from different sources (di Marco et al., 2019).
Human ability to perform actions impacts the visual perception of objects/targets. This represents the framework within which the influence of action execution on perception is typically explained. The action-specific effects indicate all the effects generated from the ability to act on spatial perception (Proffitt, 2006, 2008). The first study suggesting that spatial perception was influenced by the ability to perform an action was carried out by Bhalla and Proffitt (1999). They showed that the perception of hill slant was influenced by the physiological potential. In fact, if the energetic costs required to climb them increased, the hills were estimated to be steeper. Following this work, several researchers have focused and expanded this concept beyond the physiological potential; however, these studies focus on other aspects of action. For example, softball players who were good at hitting the ball estimated it as being bigger compared to others (Witt and Proffitt, 2005; Gray, 2013). Similarly, archers who had a better shot than others estimated the target as bigger (Lee et al., 2012). Parkour athletes judged walls as lower compared to non-parkour athletes (Taylor et al., 2011), and good tennis players judged the net as being lower (Witt and Sugovic, 2010).
Another study examining a different branch of action-specific effects analyzed the affordance of the object, in other words, the possibility to act on or with an object (Gibson, 1979). Typically, the measurement of affordance perception is carried out by assessing the point at which an action is perceived as barely possible. For example, some studies have explored the width of a doorway that is perceived as being just possible to pass through, or the height of a step at which the affordance of stepping up is perceived (Warren, 1984; Mark, 1987; Warren and Whang, 1987). Other examples are studies in which people with broader shoulders perceived doorways to be smaller compared to people with narrower shoulders (Stefanucci and Geuss, 2009), or studies in which a target is presented beyond the distance of the arm's reach: the target is perceived as being closer when the participants use a reach-extending tool to reach the target and more distant when they reach without the tool (Witt et al., 2005; Witt and Proffitt, 2008; Witt, 2011; Davoli et al., 2012; Osiurak et al., 2012; Morgado et al., 2013). Given all these remarkable data, the next section focuses on the action-specific effects on perception as a function of the specific effector used, expanding the panorama to other investigation modes.
In the eye realm, the effect of saccade execution on perception has been investigated through saccadic adaptation and perisaccadic mislocalization mechanisms. Saccadic adaptation allows researchers to study how saccade amplitudes change according to changes in the post-saccadic target shift. This change can be either parallel or orthogonal to the main direction of the saccade. In other words, it is well established that saccade amplitudes adapt when a small target is horizontally shifted during saccade execution to another position in relation to the initial one (McLaughlin, 1967; Miller et al., 1981; Deubel, 1987; Watanabe et al., 2003; Hopp and Fuchs, 2004; Kojima et al., 2005; Ethier et al., 2008; Rahmouni and Madelain, 2019). Further studies investigated the possibility of the saccadic system sharing common coordinates with other domains. In fact, several researchers have demonstrated that the modification of motor variables induced by saccade adaptation leads to a concomitant modification of the perceived location of the target when the localization is executed by a pointing movement or by a perceptual report (Bahcall and Kowler, 1999; Awater et al., 2005; Bruno and Morrone, 2007; Collins et al., 2007; Zimmermann and Lappe, 2010; Garaas and Pomplun, 2011; Gremmler et al., 2014).
A particular application of the saccadic adaptation paradigm was developed using spatially extended targets that, during the saccade, systematically changed their horizontal size (Bosco et al., 2015), and in reading studies (McConkie et al., 1989; Lavergne et al., 2010). In particular, the manipulation used in Bosco et al. (2015) influenced the target visual perception. The modification of size perception occurred according to the direction of saccadic amplitude adaptation: if the saccade was adapted to a smaller amplitude, target size was perceived as being smaller; if the saccade adapted to a larger amplitude, target size was perceived as being larger (Bosco et al., 2015). The scheme of the adaptation phase paradigm and the consequent size perception modification measured by grip aperture of the hand is shown in Figure 4.
Figure 4. Size perception modification induced by saccadic adaptation. (A) Top row, Shortening adaptation condition. The fixation point was presented at the start of the trial. After 1 s, a bar appeared, but participants had to continue to focus on the fixation target. After a randomized time, an acoustic signal indicated the possibility of executing a saccade toward the bar. Then the bar was decreased in size by 30% of its length at the right border as soon as the saccade was detected. Bottom row, Lengthening adaptation phase. This condition was identical to the shortening adaptation condition, with the only difference being that the bar was increased in size by 30% during saccade execution. (B) Mean deviation of grip aperture from baseline in shortening adaptation (white column) and lengthening (black column) adaptation. The data were averaged across subjects and sizes. Error bars indicate SE. *p < 0.05, significant deviations from baseline (modified from Bosco et al., 2015).
However, recent studies have shown that change in perception of visual features is present also without saccadic adaptation (Herwig and Schneider, 2014; Herwig et al., 2015, 2018; Valsecchi and Gegenfurtner, 2016; Paeye et al., 2018; Köller et al., 2020; Valsecchi et al., 2020). This phenomenon occurs with the following features: the perception of spatial frequency (Herwig and Schneider, 2014; Herwig et al., 2018), shape (Herwig et al., 2015; Paeye et al., 2018; Köller et al., 2020), and size (Valsecchi and Gegenfurtner, 2016; Bosco et al., 2020; Valsecchi et al., 2020). For example, Bosco et al. (2020) used a manipulation consisting in the systematic shortening and lengthening of a vertical bar during a horizontal saccade aimed to do not modify the saccade amplitude; by these conditions, they observed a significant difference in perceived size after the saccade execution (see Figure 5A for the scheme of saccadic adaptation paradigm in Bosco et al., 2020). This finding suggested that the modification of size perception does not rely on the modified saccadic amplitude induced by saccadic adaptation mechanisms (see Figure 5B, Bosco et al., 2020). In the study by Valsecchi et al. (2020), it was shown that saccadic adaptation and size recalibration share the same temporal development. However, size recalibration of the visual stimuli was also present in the opposite hemifield, but saccadic adaptation did not suggest that distinct mechanisms were involved. Although the modification of saccadic parameter induced by saccadic adaptation is not the causal mechanism for the modification of stimulus property perception, the shift of the target image from the periphery to the fovea, typically performed by a saccade, remains the potential cause of the observed object perception modification.
Figure 5. Size perception modification not induced by saccadic adaptation. (A) Top row, Shortening adaptation condition. The fixation point was presented at the start of the trial. After 1 s, a bar appeared, but participants had to continue to focus on the fixation target. After a randomized time, an acoustic signal indicated the possibility of executing a saccade toward the bar. Then the bar was symmetrically decreased in size by 30% of its length as soon as the saccade was detected. Bottom row, Lengthening adaptation. This was identical to the shortening adaptation, with the only difference being that the bar was symmetrically increased in size by 30% during the execution of the saccade. (B) Mean deviation of size perception (grip aperture) from baseline for the shortening (white column) and the lengthening (black column) adaptation trials. The data were averaged across participants and sizes. Details as in Figure 4 (modified from Bosco et al., 2020). *p < 0.05, significance level.
A considerable body of literature has shown that the visual stimuli briefly presented just before the onset of a saccade, or during it, are mislocalized and perceived as being closer to the saccade target (Matin and Pearce, 1965; Honda, 1989; Schlag and Schlag-Rey, 1995; Ross et al., 1997). In other terms, this mislocalization consists in a shift of apparent position in the direction of the saccade (Honda, 1989, 1995; Schlag and Schlag-Rey, 1995; Cai et al., 1997; Lappe et al., 2000) and a compression of positions onto the target location of the saccade (Bischof and Kramer, 1968; Ross et al., 1997; Lappe et al., 2000). The shift is attributed to a mismatch between the actual eye position during the saccades and the predicted position originating from an internal corollary discharge (Duhamel et al., 1992; Nakamura and Colby, 2002; Kusunoki and Goldberg, 2003; Morrone et al., 2005).
Interestingly, the compression effect is primarily observed parallel to the saccade direction (Ross et al., 1997), and also in the orthogonal direction (Kaiser and Lappe, 2004; Zimmermann et al., 2014, 2015), suggesting that a linear translation of the internal coordinate system is a reductive explanation. Additionally, non-spatial features such as the shape and colors of perisaccadic stimuli have also been investigated to evaluate the effect of perisaccadic compression. Specifically, the discrimination of shape (Matsumiya and Uchikawa, 2001) and colors (Lappe et al., 2006; Wittenberg et al., 2008) of visual stimuli is preserved, but they are not perceived in separate positions. Although the mechanism of this effect is still an open question, the general view describes the perisaccadic mislocalization as being related to mechanisms aimed at maintaining visual stability (Matin and Pearce, 1965; Honda, 1989; Schlag and Schlag-Rey, 1995; Ross et al., 1997; Lappe et al., 2000; Pola, 2004; Binda and Morrone, 2018).
The execution of different types of hand movements can generate perceptual modifications of object properties relevant for that type of action, such as the perception of size and weight. In 2017, Bosco et al. (2017) investigated the direct effect of reaching and grasping execution on the size perception of a visual target. They found that the change in size perception was larger after a grasping action than after a reaching action and all participants perceived objects to be smaller after the grasping compared to the reaching. These results were consistent in both manual and verbal reports, as is shown in Figure 6 (Bosco et al., 2017). Sanz Diez et al. (2022) evaluated size perception after a grasping movement performed toward a visual target that changed in size during the execution of the movement. Although the perceptual phase before and after grasping execution applied to the same target that, in these two moments of the task, was identical in size, they found that, after the grasping action, reports regarding perceptual size showed significant differences that depended on the type of size change that occurred during movement execution. In fact, as shown in Figure 7, observers reported a smaller size perception when the visual target was lengthened during the grasping execution and no perception modification when the visual target was shortened during the grasping execution (Sanz Diez et al., 2022). In both of the studies described above, the perceptual modification occurred according to the type of movement (i.e., reaching or grasping) and to the unpredictable changes of target size during the movement itself, suggesting that this modification can be considered to be a descriptive parameter of the previous motor action (Bosco et al., 2017; Sanz Diez et al., 2022).
Figure 6. Size perception modification after reaching and grasping actions. (A) Mean deviation of perceptual responses by grip aperture for reaching (white column) and grasping (black column). (B) Mean deviation of verbal perceptual reports for reaching (white column) and grasping (black column). All data are averaged across participants and sizes. Error bars are standard errors of the mean. *p < 0.05, significance level (modified from Bosco et al., 2017).
Figure 7. Size perception modification after size-perturbed grasping execution. (A) Experimental task sequence. (B) Mean Deviation values of grip aperture from the baseline averaged across size perturbation condition. Details as in Figure 5 (modified from Sanz Diez et al., 2022). *p < 0.05, significance level.
An advantage of the effect of action execution on perception is represented by changes to the motor system obtained with skill learning. The formation and retrieval of sensorimotor memories acquired from previous hand-object interactions are fundamental for dexterous object manipulation learning (Westling and Johansson, 1984; Johansson and Westling, 1988). This allows the modulation of digit forces in a fashion that is anticipatory, i.e., before the lifting of the object (Gordon et al., 1993; Burstedt et al., 1999; Salimi et al., 2000). In a task requiring participants to lift an object while minimizing the roll caused by asymmetric mass distribution of an external torque, the implicit learning after action execution led to minimization of object roll by a re-arrangement of digit positions (Lukos et al., 2007, 2008) and a modulation of the force distribution exerted by the fingers (Salimi et al., 2000; Fu et al., 2010).
Within this perspective, it is also useful to describe the size-weight illusion (SWI), for the first time described by Charpentier (Charpentier, 1891). In fact, the SWI is visible when a subject lifts two objects of different size, but of equal weight, and reports the smaller object as being heavier. The SWI illusion is robust (Murray et al., 1999; Flanagan and Beltzner, 2000; Kawai, 2002a,b, 2003a,b; Grandy and Westwood, 2006; Dijker, 2008; Flanagan et al., 2008; Chouinard et al., 2009), and the effect is still present when the lifter knows that both objects are of the same weight (Flanagan and Beltzner, 2000). The SWI illusion has been thoroughly studied to understand the mechanism of signal integration for weight perception, and it is an example of how the sensorimotor system works in a Bayesian manner. According to this view, the nervous system combines prior knowledge regarding object properties learned by previous experience (“the prior”) with current sensory information (“the likelihood”), to appropriately estimate object property (“the posterior”) for action and perception functions (van Beers et al., 2002; Körding and Wolpert, 2006). In most cases, the combination of prior and likelihood generates correct perception and behavior, but perception can be misleading. In the case of SWI, for example, the prior is perceived higher than the likelihood, generating a perception that does not correspond with the actual physical properties of the object. However, the repetition of the lifting action recalibrates the perception of weight, and the force distribution is adjusted according to the real weight of the objects. Although there is still no consensus as to the process that gives rise to the SWI, an objective aspect is that the execution of the manipulation action on the objects has a pragmatic effect on weight and size perception.
Walking interaction leads to a perception-action recalibration, and it is typically investigated by the measurement of perceived size or perceived distance. This is because, according to the size-distance invariance hypothesis (Sedgwick, 1986), size and distance perception are strictly coupled. Brenner and van Damme (1999) found that perceived object size, shape, and distance are largely independent. Although object size, shape, and distance estimations were similarly affected by changes in object distance perception, modifications in perceived shape caused by motion parallax did not affect perceived size or distance. This indicates their independence. Although the direct relationship between size and distance perception has been debated in this study, the judgements of distance and size have been shown to be tightly linked in other studies (Gogel et al., 1985; Hutchison and Loomis, 2006). Results showing an improvement in judgments of distances after the walking interaction were found by Waller & Richardson (Waller and Richardson, 2008). The same authors showed that distance judgments in a virtual environment were unaffected by interactions in which participants viewed only a simulation of visual walking (i.e., optic flow only). This suggested that a body-based movement is necessary. Furthermore, perceptual reports of distance increased in accuracy after participants performed a blind-walking task consisting in the receipt of visual or verbal feedback (Richardson and Waller, 2005; Mohler et al., 2006). The results showed the sufficiency of body-based interaction. Kelly et al. (2013) found that perceptual reports of object size improved after a walking interaction, because an increase in perceived distance was observed. The finding that perceptual reports regarding size improved after the interaction indicates that walking leads to a rescaling of space perception and not only to a simple recalibration of walked distance (Siegel et al., 2017). In open-loop blind walking tasks, calibration and recalibration of locomotion has been observed. In these tasks, an observer views a target on the ground and, after closing his/her eyes, he/she has to walk toward the target without seeing. In normal conditions, blind walking performance is quite accurate and reflects the perception of the target location (Rieser et al., 1990; Loomis and Philbeck, 2008). After manipulation of the rate of environmental optic flow in relation to the biomechanical rate of normal walking, observers undershot the target when the environmental flow was faster, and overshot the target when environmental flow was slower compared to the perception of normal walking speed (Rieser et al., 1995). Additionally, studies investigating the visual perception of egocentric distances showed that perceptual judgments (e.g., verbal reports) showed a systematic underestimation of egocentric distances (Foley, 1977; Li and Giudice, 2013), while blindfolded walking toward a remembered target location was executed more accurately (Loomis et al., 1992; Li and Giudice, 2013). Although the former suggests that a systematic compression of physical distance is visually perceived, visually directed walking is not affected by this perceptual distortion.
A multitude of works have been presented showing the effect of actions performed with the eyes, the hands, and the legs on visual perception of objects and space using different approaches and paradigms. The action influence is present before and after execution of the movement, suggesting that visual perception, when it is integrated with the action, is “ready to act” (before execution) and is transformed by action execution (see Figure 8 for a summary). In both cases, the perceptual responses, collected in different ways, are parameters that describe the subsequent or previous motor responses. This suggests a mechanism which exchanges information between the motor and perceptual system when we are in a specific visuomotor contingency. At a behavioral level, we can take advantage of these aspects because they can be used as action intention predictors when they occur during action planning and, interestingly, as a postdictive component that specifies the previous motor experience when they occur after action execution. In this latter case, the postdictive perceptual component also updates the information that is necessary for a potential subsequent action. The use of the action-based perceptual information can be helpful in all those artificial intelligent (AI) systems that are used with motor assistive devices. In fact, the use of perceptual information during action planning can be implemented with other parameters (e.g., neural signals) to extract action intentions that exploit the residual motor abilities of different effectors that are necessary to give perceptual responses by pressing a button, for example, or extending only certain fingers and not others. The use of perceptual information after action execution can be implemented in AI systems that are able to communicate with humans, with the objective of creating a mutual learning exchange. In fact, the modification of perception following the execution of a particular movement may be used as a feedback signal, in order to correct a subsequent motor response and compensate for the error due to previous AI action decisions. This allows the system to improve the outcome of the action and, consequently, increases the user's trust in the AI system.
Figure 8. Summary of object/space perception modulation by action planning and action execution evoked by movement of different effectors.
AB: conceptualization, visualization, writing original draft, and writing—review and editing. SDP: visualization and writing original draft. MF: writing—review and editing. PF: writing—review and editing and funding acquisition. All authors contributed to the article and approved the submitted version.
(1) MAIA project has received funding from the European Union's Horizon 2020 Research and Innovation Programme under grant agreement No 951910; (2) Ministero dell'Istruzione—Ministero dell'Universita' e della Ricerca (PRIN2017—Prot. 2017KZNZLN). This work has received funding from the European Union's Horizon 2020 Research and Innovation Programme under the Marie-Skodowska-Curie grant agreement No. 734227-Platypus.
SDP is employed by Carl Zeiss Vision International GmbH.
The remaining authors declare that the research was conducted in the absence of any commercial or financial relationships that could be constructed as a potential conflict of interest.
All claims expressed in this article are solely those of the authors and do not necessarily represent those of their affiliated organizations, or those of the publisher, the editors and the reviewers. Any product that may be evaluated in this article, or claim that may be made by its manufacturer, is not guaranteed or endorsed by the publisher.
Angel, R. W., Alston, W., and Garland, H. (1970). Functional relations between the manual and oculomotor control systems. Exp. Neurol. 27, 248–257. doi: 10.1016/0014-4886(70)90218-9
Awater, H., Burr, D., Lappe, M., Morrone, M. C., and Goldberg, M. E. (2005). Effect of saccadic adaptation on localization of visual targets. J. Neurophysiol. 93, 3605–14. doi: 10.1152/jn.01013.2003
Bagesteiro, L. B., Sarlegna, F. R., and Sainburg, R. L. (2006). Differential influence of vision and proprioception on control of movement distance. Exp. Brain Res. 171, 358. doi: 10.1007/s00221-005-0272-y
Bahcall, D. O., and Kowler, E. (1999). Illusory shifts in visual direction accompany adaptation of saccadic eye movements. Nature 400, 864–6. doi: 10.1038/23693
Bekkering, H., Adam, J. J., van den Aarssen, A., Kingma, H., and Whiting, H. T. A. (1995). Interference between saccadic eye and goal-directed hand movements. Exp. Brain. Res. 106, 475–484. doi: 10.1007/BF00231070
Bekkering, H., Adam, Jos, J., Kingma, H., Huson, A., and Whiting, H. T. A. (1994). Reaction time latencies of eye and hand movements in single- and dual-task conditions. Exp. Brain. Res. 97, 12. doi: 10.1007/BF00241541
Bekkering, H., and Neggers, S. F. (2002). Visual search is modulated by action intentions. Psychol. Sci. 13, 370–374. doi: 10.1111/j.0956-7976.2002.00466.x
Benedetto, A., and Morrone, M. C. (2017). Saccadic suppression is embedded within extended oscillatory modulation of sensitivity. J. Neurosci. 37, 3661. doi: 10.1523/JNEUROSCI.2390-16.2016
Benedetto, A., Morrone, M. C., and Tomassini, A. (2020). The common rhythm of action and perception. J. Cogn. Neurosci. 32, 187–200. doi: 10.1162/jocn_a_01436
Bhalla, M., and Proffitt, D. R. (1999). Visual–motor recalibration in geographical slant perception. J. Exp. Psychol. Hum. Percept. Perform. 25, 1076–1096. doi: 10.1037/0096-1523.25.4.1076
Biguer, B., Jeannerod, M., and Prablanc, C. (1982). The coordination of eye, head, and arm movements during reaching at a single visual target. Exp. Brain. Res. 46, 301–304. doi: 10.1007/BF00237188
Binda, P., and Morrone, M. C. (2018). Vision during saccadic eye movements. Annu. Rev. Vis. Sci. 4, 193–213. doi: 10.1146/annurev-vision-091517-034317
Bischof, N., and Kramer, E. (1968). Untersuchungen und berlegungen zur Richtungswahrnehmung bei willkrlichen sakkadischen Augenbewegungen. Psychol. Forsch. 32, 185–218. doi: 10.1007/BF00418660
Bosco, A., Daniele, F., and Fattori, P. (2017). Reaching and grasping actions and their context shape the perception of object size. J. Vis. 17, 10. doi: 10.1167/17.12.10
Bosco, A., Lappe, M., and Fattori, P. (2015). Adaptation of saccades and perceived size after trans-saccadic changes of object size. J. Neurosci. 35, 14448–14456. doi: 10.1523/JNEUROSCI.0129-15.2015
Bosco, A., Rifai, K., Wahl, S., Fattori, P., and Lappe, M. (2020). Trans-saccadic adaptation of perceived size independent of saccadic adaptation. J. Vis. 20, 19. doi: 10.1167/jov.20.7.19
Bourdin, C., Bringoux, L., Gauthier, G. M., and Vercher, J. L. (2006). Vision of the hand prior to movement onset allows full motor adaptation to a multi-force environment. Brain. Res. Bull. 71, 101–110. doi: 10.1016/j.brainresbull.2006.08.007
Boynton, G. M., Demb, J. B., Glover, G. H., and Heeger, D. J. (1999). Neuronal basis of contrast discrimination. Vision Res. 39, 257–269. doi: 10.1016/S0042-6989(98)00113-8
Brenner, E., and van Damme, W. J. M. (1999). Perceived distance, shape and size. Vision Res. 39, 975–986. doi: 10.1016/S0042-6989(98)00162-X
Brouwer, A.-M., Franz, V. H., and Gegenfurtner, K. R. (2009). Differences in fixations between grasping and viewing objects. J. Vis. 9, 1–24. doi: 10.1167/9.1.18
Bruno, A., and Morrone, M. C. (2007). Influence of saccadic adaptation on spatial localization: comparison of verbal and pointing reports. J. Vis. 7, 1–13. doi: 10.1167/7.5.16
Burstedt, M. K. O., Flanagan, J. R., and Johansson, R. S. (1999). Control of grasp stability in humans under different frictional conditions during multidigit manipulation. J. Neurophysiol. 82, 2393–2405. doi: 10.1152/jn.1999.82.5.2393
Buzsáki, G., and Draguhn, A. (2004). Neuronal oscillations in cortical networks. Science 304, 1926–1929. doi: 10.1126/science.1099745
Cai, R. H., Pouget, A., Schlag-Rey, M., and Schlag, J. (1997). Perceived geometrical relationships affected by eye-movement signals. Nature 386, 601–604. doi: 10.1038/386601a0
Charpentier, A. (1891). Analyse experimentale de quelques elements de la sensation de poids. Arch. Physiol. Norm. Pathol. 3, 122–135.
Chouinard, P., Large, M., Chang, E., and Goodale, M. (2009). Dissociable neural mechanisms for determining the perceived heaviness of objects and the predicted weight of objects during lifting: An fMRI investigation of the size–weight illusion. Neuroimage 44, 200–212. doi: 10.1016/j.neuroimage.2008.08.023
Coello, Y., and Grealy, M. A. (1997). Effect of size and frame of visual field on the accuracy of an aiming movement. Perception 26, 287–300. doi: 10.1068/p260287
Collins, T., Doré-Mazars, K., and Lappe, M. (2007). Motor space structures perceptual space: evidence from human saccadic adaptation. Brain Res. 1172, 32–39. doi: 10.1016/j.brainres.2007.07.040
Conti, P., and Beaubaton, D. (1980). Role of structured visual field and visual reafference in accuracy of pointing movements. Percept. Mot. Skills 50, 239–244. doi: 10.2466/pms.1980.50.1.239
Craighero, L., Fadiga, L., Rizzolatti, G., and Umiltà, C. (1999). Action for perception a motor—visual attentional effect. J. Exp. Psychol. Hum. Percept. Perform. 25, 1673–1692. doi: 10.1037/0096-1523.25.6.1673
Davoli, C. C., Brockmole, J. R., and Witt, J. K. (2012). Compressing perceived distance with remote tool-use: Real, imagined, and remembered. J. Exp. Psychol. Hum. Percept. Perform. 38, 80–89. doi: 10.1037/a0024981
de Brouwer, A. J., Flanagan, J. R., and Spering, M. (2021). Functional use of eye movements for an acting system. Trends Cogn. Sci. 25, 252–263. doi: 10.1016/j.tics.2020.12.006
Desmurget, M., Pélisson, D., Rossetti, Y., and Prablanc, C. (1998). “From eye to hand: planning goal-directed movements,” in Neuroscience and Biobehavioral Reviews, p. 761–788. doi: 10.1016/S0149-7634(98)00004-9
Desmurget, M., Rossetti, Y., Jordan, M., Meckler, C., and Prablanc, C. (1997). Viewing the hand prior to movement improves accuracy of pointing performed toward the unseen contralateral hand. Exp. Brain. Res. 115, 180–186. doi: 10.1007/PL00005680
Desmurget, M., Rossetti, Y., Prablanc, C., Jeannerod, M., and Stelmach, G. E. (1995). Representation of hand position prior to movement and motor variability. Can. J. Physiol. Pharmacol. 73, 262–272. doi: 10.1139/y95-037
Deubel, H. (1987). “Adaptivity of gain and direction in oblique saccades.,” in Eye movements from physiology to cognition. (London: Elsevier), p. 181–190. doi: 10.1016/B978-0-444-70113-8.50030-8
Deubel, H., and Schneider, W. X. (1996). Saccade target selection and object recognition: evidence for a common attentional mechanism. Vision. Res. 36, 1827–1837. doi: 10.1016/0042-6989(95)00294-4
di Marco, S., Tosoni, A., Altomare, E. C., Ferretti, G., Perrucci, M. G., and Committeri, G. (2019). Walking-related locomotion is facilitated by the perception of distant targets in the extrapersonal space. Sci. Rep. 9, 9884. doi: 10.1038/s41598-019-46384-5
Dijker, A. J. M. (2008). Why Barbie feels heavier than Ken: the influence of size-based expectancies and social cues on the illusory perception of weight. Cognition 106, 1109–1125. doi: 10.1016/j.cognition.2007.05.009
Duhamel, J.-R., Colby, C. L., and Goldberg, M. E. (1992). The updating of the representation of visual space in parietal cortex by intended eye movements. Science 255, 90–92. doi: 10.1126/science.1553535
Elliott, D., Dutoy, C., Andrew, M., Burkitt, J. J., Grierson, L. E. M., Lyons, J. L., et al. (2014). The influence of visual feedback and prior knowledge about feedback on vertical aiming strategies. J. Mot. Behav. 46, 433–443. doi: 10.1080/00222895.2014.933767
Elliott, D., Garson, R. G., Goodman, D., and Chua, R. (1991). Discrete vs. continuous visual control of manual aiming. Hum. Mov. Sci. 10, 393–418. doi: 10.1016/0167-9457(91)90013-N
Ethier, V., Zee, D. S., and Shadmehr, R. (2008). Changes in control of saccades during gain adaptation. J. Neurosci. 28, 13929 LP–13937. doi: 10.1523/JNEUROSCI.3470-08.2008
Fagioli, S., Hommel, B., and Schubotz, R. I. (2007). Intentional control of attention: action planning primes action-related stimulus dimensions. Psychol. Res. 71, 22–29. doi: 10.1007/s00426-005-0033-3
Fini, C., Bardi, L., Troje, N. F., Committeri, G., and Brass, M. (2017). Priming biological motion changes extrapersonal space categorization. Acta. Psychol. 172, 77–83. doi: 10.1016/j.actpsy.2016.11.006
Fini, C., Brass, M., and Committeri, G. (2015a). Social scaling of extrapersonal space: target objects are judged as closer when the reference frame is a human agent with available movement potentialities. Cognition 134, 50–56. doi: 10.1016/j.cognition.2014.08.014
Fini, C., Committeri, G., Müller, B. C. N., Deschrijver, E., and Brass, M. (2015b). How watching pinocchio movies changes our subjective experience of extrapersonal space. PLoS One 10, e0120306. doi: 10.1371/journal.pone.0120306
Fini, C., Costantini, M., and Committeri, G. (2014). Sharing space: the presence of other bodies extends the space judged as near. PLoS One 9, e114719. doi: 10.1371/journal.pone.0114719
Flanagan, J. R., and Beltzner, M. A. (2000). Independence of perceptual and sensorimotor predictions in the size–weight illusion. Nat. Neurosci. 3, 737–741. doi: 10.1038/76701
Flanagan, J. R., Bittner, J. P., and Johansson, R. S. (2008). Experience can change distinct size-weight priors engaged in lifting objects and judging their weights. Curr. Biol. 18, 1742–1747. doi: 10.1016/j.cub.2008.09.042
Flanagan, J. R., and Rao, A. K. (1995). Trajectory adaptation to a non-linear visuomotor transformation: evidence of motion planning in visually perceived space. J. Neurophysiol. 74, 2174–2178. doi: 10.1152/jn.1995.74.5.2174
Foley, J. M. (1977). Effect of distance information and range on two indices of visually perceived distance. Perception 6, 449–460. doi: 10.1068/p060449
Fu, Q., Zhang, W., and Santello, M. (2010). Anticipatory planning and control of grasp positions and forces for dexterous two-digit manipulation. J. Neurosci. 30, 9117–9126. doi: 10.1523/JNEUROSCI.4159-09.2010
Garaas, T. W., and Pomplun, M. (2011). Distorted object perception following whole-field adaptation of saccadic eye movements. J. Vis. 11, 2. doi: 10.1167/11.1.2
Gogel, W. C., Loomis, J. M., Newman, N. J., and Sharkey, T. J. (1985). Agreement between indirect measures of perceived distance. Percept. Psychophys. 37, 17–27. doi: 10.3758/BF03207134
Gordon, A. M., Westling, G., Cole, K. J., and Johansson, R. S. (1993). Memory representations underlying motor commands used during manipulation of common and novel objects. J. Neurophysiol. 69, 1789–1796. doi: 10.1152/jn.1993.69.6.1789
Grandy, M. S., and Westwood, D. A. (2006). Opposite perceptual and sensorimotor responses to a size-weight illusion. J. Neurophysiol. 95, 3887–3892. doi: 10.1152/jn.00851.2005
Gray, R. (2013). Being selective at the plate: processing dependence between perceptual variables relates to hitting goals and performance. J. Exp. Psychol. Hum. Percept. Perform. 39, 1124–1142. doi: 10.1037/a0030729
Gremmler, S., Bosco, A., Fattori, P., and Lappe, M. (2014). Saccadic adaptation shapes visual space in macaques. J. Neurophysiol. 111, 1846–1851. doi: 10.1152/jn.00709.2013
Gutteling, T. P., Kenemans, J. L., and Neggers, S. F. W. (2011). Grasping preparation enhances orientation change detection. PLoS One 6, 11. doi: 10.1371/journal.pone.0017675
Hamilton, A., Wolpert, D., and Frith, U. (2004). Your own action influences how you perceive another person's action. Curr. Biol. 14, 493–498. doi: 10.1016/j.cub.2004.03.007
Hayhoe, M. M. (2017). Vision and action. Ann. Rev. Vis. Sci. 3, 389–413. doi: 10.1146/annurev-vision-102016-061437
Hayhoe, M. M., Shrivastava, A., Mruczek, R., and Pelz, J. B. (2003). Visual memory and motor planning in a natural task. J. Vis. 3, 6. doi: 10.1167/3.1.6
Herwig, A., and Schneider, W. X. (2014). Predicting object features across saccades: evidence from object recognition and visual search. J. Exp. Psychol. Gen. 143, 1903–1922. doi: 10.1037/a0036781
Herwig, A., Weiß, K., and Schneider, W. X. (2015). When circles become triangular: how transsaccadic predictions shape the perception of shape. Ann. N Y Acad. Sci. 1339, 97–105. doi: 10.1111/nyas.12672
Herwig, A., Weiß, K., and Schneider, W. X. (2018). Feature prediction across eye movements is location specific and based on retinotopic coordinates. J. Vis. 18, 13. doi: 10.1167/18.8.13
Hoffman, J. E., and Subramaniam, B. (1995). The role of visual attention in saccadic eye movements. Percept. Psychophys. 57, 787–795. doi: 10.3758/BF03206794
Hommel, B., Brown, S., and Nattkemper, D. (2016). Human Action Control. Cham: Springer. doi: 10.1007/978-3-319-09244-7
Hommel, B., Müsseler, J., Aschersleben, G., and Prinz, W. (2001). The theory of event coding (TEC): a framework for perception and action planning. Behav. Brain Sci. 24, 849–878. doi: 10.1017/S0140525X01000103
Honda, H. (1989). Perceptual localization of visual stimuli flashed during saccades. Percept. Psychophys. 45, 162–174. doi: 10.3758/BF03208051
Honda, H. (1995). Visual mislocalization produced by a rapid image displacement on the retina: examination by means of dichoptic presentation of a target and its background scene. Vision. Res. 35, 3021–3028. doi: 10.1016/0042-6989(95)00108-C
Hopp, J. J., and Fuchs, A. F. (2004). The characteristics and neuronal substrate of saccadic eye movement plasticity. Prog. Neurobiol. 72, 27–53. doi: 10.1016/j.pneurobio.2003.12.002
Hutchison, J. J., and Loomis, J. M. (2006). Does energy expenditure affect the perception of egocentric distance? a failure to replicate experiment 1 of proffitt, stefanucci, banton, and epstein (2003). Span. J. Psychol. 9, 332–339. doi: 10.1017/S1138741600006235
Jeannerod, M. (1981). Intersegmental coordination during reaching at natural visual objects. Atten. Perform. 11, 153–169.
Johansson, R. S., and Westling, G. (1988). Programmed and triggered actions to rapid load changes during precision grip. Exp. Brain Res. 2, 71. doi: 10.1007/BF00247523
Johansson, R. S., Westling, G., Bäckström, A., and Flanagan, J. R. (2001). Eye–hand coordination in object manipulation. J. Neurosci. 21, 6917–6932. doi: 10.1523/JNEUROSCI.21-17-06917.2001
Kaiser, M., and Lappe, M. (2004). Perisaccadic mislocalization orthogonal to saccade direction. Neuron 41, 293–300. doi: 10.1016/S0896-6273(03)00849-3
Kawai, S. (2002a). Heaviness perception: I. constant involvement of haptically perceived size in weight discrimination. Exp. Brain Res. 147, 16–22. doi: 10.1007/s00221-002-1209-3
Kawai, S. (2002b). Heaviness perception: II. contributions of object weight, haptic size, and density to the accurate perception of heaviness or lightness. Exp. Brain. Res. 147, 23–28. doi: 10.1007/s00221-002-1210-x
Kawai, S. (2003a). Heaviness perception: III. Weight/aperture in the discernment of heaviness in cubes haptically perceived by thumb-index finger grasp. Exp. Brain Res. 153, 289–296. doi: 10.1007/s00221-003-1621-3
Kawai, S. (2003b). Heaviness perception: IV. Weight x aperture−1 as a heaviness model in finger-grasp perception. Exp. Brain Res. 153, 297–301. doi: 10.1007/s00221-003-1622-2
Kelly, J. W., Donaldson, L. S., Sjolund, L. A., and Freiberg, J. B. (2013). More than just perception–action recalibration: walking through a virtual environment causes rescaling of perceived space. Atten. Percept. Psychophys. 75, 1473–1485. doi: 10.3758/s13414-013-0503-4
Kirsch, W. (2015). Impact of action planning on spatial perception: attention matters. Acta. Psychol. (Amst) 156, 22–31. doi: 10.1016/j.actpsy.2015.01.002
Kirsch, W., Herbort, O., Butz, M. v, and Kunde, W. (2012). Influence of motor planning on distance perception within the peripersonal space. PLoS One 7, e34880. doi: 10.1371/journal.pone.0034880
Kirsch, W., and Kunde, W. (2013). Visual near space is scaled to parameters of current action plans. J. Exp. Psychol. Hum. Percept. Perform. 39, 1313–1325. doi: 10.1037/a0031074
Knoblich, G., and Flach, R. (2001). Predicting the effects of actions: interactions of perception and action. Psychol. Sci. 12, 467–472. doi: 10.1111/1467-9280.00387
Kojima, Y., Iwamoto, Y., and Yoshida, K. (2005). Effect of saccadic amplitude adaptation on subsequent adaptation of saccades in different directions. Neurosci. Res. 53, 404–412. doi: 10.1016/j.neures.2005.08.012
Köller, C. P., Poth, C. H., and Herwig, A. (2020). Object discrepancy modulates feature prediction across eye movements. Psychol. Res. 84, 231–244. doi: 10.1007/s00426-018-0988-5
Körding, K. P., and Wolpert, D. M. (2006). Bayesian decision theory in sensorimotor control. Trends Cogn. Sci. 10, 319–326. doi: 10.1016/j.tics.2006.05.003
Kunde, W., and Wuhr, P. (2004). Actions blind to conceptually overlapping stimuli. Psychologic. Res. Psychologische Forschung 4, 68. doi: 10.1007/s00426-003-0156-3
Kusunoki, M., and Goldberg, M. E. (2003). The time course of perisaccadic receptive field shifts in the lateral intraparietal area of the monkey. J. Neurophysiol. 89, 1519–1527. doi: 10.1152/jn.00519.2002
Land, M., Mennie, N., and Rusted, J. (1999). The roles of vision and eye movements in the control of activities of daily living. Perception 28, 1311–1328. doi: 10.1068/p2935
Land, M. F. (1992). Predictable eye-head coordination during driving. Nature 359, 318–320. doi: 10.1038/359318a0
Land, M. F. (2006). Eye movements and the control of actions in everyday life. Prog Retin Eye Res 25, 296–324. doi: 10.1016/j.preteyeres.2006.01.002
Land, M. F. (2009). Vision, eye movements, and natural behavior. Vis. Neurosci. 26, 51–62. doi: 10.1017/S0952523808080899
Land, M. F., and Lee, D. N. (1994). Where we look when we steer. Nature 369, 742–744. doi: 10.1038/369742a0
Lappe, M., Awater, H., and Krekelberg, B. (2000). Postsaccadic visual references generate presaccadic compression of space. Nature 403, 892–895. doi: 10.1038/35002588
Lappe, M., Kuhlmann, S., Oerke, B., and Kaiser, M. (2006). The fate of object features during perisaccadic mislocalization. J. Vis. 6, 11. doi: 10.1167/6.11.11
Lavergne, L., Vergilino-Perez, D., Collins, T., and Dore'-Mazars, K. (2010). Adaptation of within-object saccades can be induced by changing stimulus size. Exp. Brain Res. 203, 773–780. doi: 10.1007/s00221-010-2282-7
Lee, Y., Lee, S., Carello, C., and Turvey, M. T. (2012). An archer's perceived form scales the “hitableness” of archery targets. J. Exp. Psychol. Hum. Percept. Perform. 38, 1125–1131. doi: 10.1037/a0029036
Li, H., and Giudice, N. (2013). “The effects of 2D and 3D maps on learning virtual multi-level indoor environments,” in Proceedings of the 1st ACM SIGSPATIAL International Workshop on Map Interaction (Orlando: ACM, Orlando, p. 7–12.
Lindemann, O., and Bekkering, H. (2009). Object manipulation and motion perception: Evidence of an influence of action planning on visual processing. J. Exp. Psychol. Hum. Percept. Perform. 35, 1062–1071. doi: 10.1037/a0015023
Liversedge, S. P., and Findlay, J. M. (2000). Saccadic eye movements and cognition. Trends Cogn. Sci. 4, 6–14. doi: 10.1016/S1364-6613(99)01418-7
Loomis, J. M., da Silva, J. A., Fujita, N., and Fukusima, S. S. (1992). Visual space perception and visually directed action. J. Exp. Psychol. Hum. Percept. Perform. 18, 906–921. doi: 10.1037/0096-1523.18.4.906
Loomis, J. M., and Philbeck, J. W. (2008). “Measuring perception with spatial updating and action.,” in. Embodiment, ego-space, and action (Mahwah, NJ: Erlbaum.), eds R. L. Klatzky, M. Behrmann, and B. MacWhinney, p. 1–43.
Lukos, J., Ansuini, C., and Santello, M. (2007). Choice of contact points during multidigit grasping: effect of predictability of object center of mass location. J. Neurosci. 27, 3894–3903. doi: 10.1523/JNEUROSCI.4693-06.2007
Lukos, J. R., Ansuini, C., and Santello, M. (2008). Anticipatory control of grasping: independence of sensorimotor memories for kinematics and kinetics. J. Neurosci. 28, 12765–12774. doi: 10.1523/JNEUROSCI.4335-08.2008
Lünenburger, L., Kutz, D. F., and Hoffmann, K.-P. (2000). Influence of arm movements on saccades in humans. Euro. J. Neurosci. 12, 4107–4116. doi: 10.1046/j.1460-9568.2000.00298.x
Mark, L. S. (1987). Eyeheight-scaled information about affordances: a study of sitting and stair climbing. J. Exp. Psychol. Hum. Percept. Perform. 13, 361–370. doi: 10.1037/0096-1523.13.3.361
Matin, L., and Pearce, D. G. (1965). Visual perception of direction for stimuli flashed during voluntary saccadic eye movements. Science 148, 1485–1488. doi: 10.1126/science.148.3676.1485
Matsumiya, K., and Uchikawa, K. (2001). Apparent size of an object remains uncompressed during presaccadic compression of visual space. Vision Res. 41, 3039–3050. doi: 10.1016/S0042-6989(01)00174-2
Mattar, M. G., and Lengyel, M. (2022). Planning in the brain. Neuron. 110, 914–934. doi: 10.1016/j.neuron.2021.12.018
McConkie, G., PW, K., Reddix, M., Zola, D., and Jacobs, A. (1989). Eye movement control during reading: II. frequency of refixating a word. Percept. Psychophys. 46, 245–253. doi: 10.3758/BF03208086
McLaughlin, S. C. (1967). Parametric adjustment in saccadic eye movements. Percept. Psychophys. 2, 359–362. doi: 10.3758/BF03210071
Miller, J., Anstis, T., and Templeton, W. (1981). Saccadic plasticity: parametric adaptive control by retinal feedback. J. Exp. Psychol. Hum. Percept. Perform. 7, 356–66. doi: 10.1037/0096-1523.7.2.356
Mohler, B. J., Creem-Regehr, S. H., and Thompson, W. B. (2006). “The influence of feedback on egocentric distance judgments in real and virtual environments,” in ACM SIGGRAPH Symposium on Applied Perception in Graphics and Visualization (Washington, DC: ACM), p. 9–14. doi: 10.1145/1140491.1140493
Morasso, P. (1981). Spatial control of arm movements. Exp. Brain Res. 2, 42. doi: 10.1007/BF00236911
Morgado, N., Gentaz, É., Guinet, É., Osiurak, F., and Palluel-Germain, R. (2013). Within reach but not so reachable: Obstacles matter in visual perception of distances. Psychon. Bull. Rev. 20, 462–467. doi: 10.3758/s13423-012-0358-z
Morrone, M. C., Ross, J., and Burr, D. (2005). Saccadic eye movements cause compression of time as well as space. Nat. Neurosci. 8, 950–954. doi: 10.1038/nn1488
Murray, D. J., Ellis, R. R., Bandomir, C. A., and Ross, H. E. (1999). Charpentier 1891 on the size—weight illusion. Percept. Psychophys. 61, 1681–1685. doi: 10.3758/BF03213127
Musseler, J., and Hommel, B. (1997). Blindness to response-compatible stimuli. J. Exp. Psychol. Hum. Percept. Perform. 23, 861–872. doi: 10.1037/0096-1523.23.3.861
Nakamura, K., and Colby, C. L. (2002). Updating of the visual representation in monkey striate and extrastriate cortex during saccades. Proceed. Nat. Acad. Sci. 99, 4026–4031. doi: 10.1073/pnas.052379899
Neggers, S., Huijbers, W., Vrijlandt, C., Vlaskamp, B., Schutter, D., and Kenemans, J. (2007). TMS pulses on the frontal eye fields break coupling between visuospatial attention and eye movements. J. Neurophysiol. 98, 2765–2778. doi: 10.1152/jn.00357.2007
Neggers, S. F., and Bekkering, H. (2000). Ocular gaze is anchored to the target of an ongoing pointing movement. J. Neurophysiol. 83, 639–651. doi: 10.1152/jn.2000.83.2.639
Neggers, S. F. W., and Bekkering, H. (1999). Integration of visual and somatosensory target information in goal-directed eye and arm movements. Exp. Brain. Res. 125, 97–107. doi: 10.1007/s002210050663
Neggers, S. F. W., and Bekkering, H. (2001). Gaze anchoring to a pointing target is present during the entire pointing movement and is driven by a non-visual signal. J. Neurophysiol. 86, 961–970. doi: 10.1152/jn.2001.86.2.961
Neggers, S. F. W., and Bekkering, H. (2002). Coordinated control of eye and hand movements in dynamic reaching. Hum. Mov. Sci. 21, 37–64. doi: 10.1016/S0167-9457(02)00120-3
Noel, J.-P., Grivaz, P., Marmaroli, P., Lissek, H., Blanke, O., and Serino, A. (2015). Full body action remapping of peripersonal space: the case of walking. Neuropsychologia 70, 375–384. doi: 10.1016/j.neuropsychologia.2014.08.030
Osiurak, F., Morgado, N., and Palluel-Germain, R. (2012). Tool use and perceived distance: when unreachable becomes spontaneously reachable. Exp. Brain Res. 218, 331–339. doi: 10.1007/s00221-012-3036-5
Paeye, C., Collins, T., Cavanagh, P., and Herwig, A. (2018). Calibration of peripheral perception of shape with and without saccadic eye movements. Atten. Percept. Psychophys. 80, 723–737. doi: 10.3758/s13414-017-1478-3
Patla, A., and Vickers, J. (2003). How far ahead do we look when required to step on specific locations in the travel path during locomotion? Exp. Brain Res. 148, 133–138. doi: 10.1007/s00221-002-1246-y
Pelisson, D., Prablanc, C., Goodale, M., and Jeannerod, M. (1986). Visual control of reaching movements without vision of the limb. Exp. Brain Res. 62, 303–311.
Pelz, J., Hayhoe, M., and Loeber, R. (2001). The coordination of eye, head, and hand movements in a natural task. Exp. Brain Res. 139, 266–277. doi: 10.1007/s002210100745
Pola, J. (2004). Models of the mechanism underlying perceived location of a perisaccadic flash. Vision Res. 44, 2799–2813. doi: 10.1016/j.visres.2004.06.008
Prablanc, C., Echallier, J. F., Komilis, E., and Jeannerod, M. (1979). Optimal response of eye and hand motor systems in pointing at a visual target. Biol. Cybern. 35, 113–124. doi: 10.1007/BF00337436
Prinz, W. (1990). “A Common Coding Approach to Perception and Action,” in Relationships Between Perception and Action: Current Approaches, eds. O. Neumann and W. Prinz (Berlin, Heidelberg: Springer Berlin Heidelberg), p. 167–201. doi: 10.1007/978-3-642-75348-0_7
Prinz, W. (1997). Perception and action planning. Euro. J. Cogn. Psychol. 9, 129–154. doi: 10.1080/713752551
Proffitt, D. (2008). “An action-specific approach to spatial perception,” in R. L. Klatzky, B. MacWhinney and M. Behrmann (Eds.) (New York: Psychology Press.), 179–202.
Proffitt, D. R. (2006). Distance perception. Curr. Dir. Psychol. Sci. 15, 131–135. doi: 10.1111/j.0963-7214.2006.00422.x
Rahmouni, S., and Madelain, L. (2019). Inter-individual variability and consistency of saccade adaptation in oblique saccades: amplitude increase and decrease in the horizontal or vertical saccade component. Vision Res. 160, 82–98. doi: 10.1016/j.visres.2019.05.001
Richardson, A. R., and Waller, D. (2005). The effect of feedback training on distance estimation in virtual environments. Appl. Cogn. Psychol. 19, 1089–1108. doi: 10.1002/acp.1140
Rieser, J. J., Ashmead, D. H., Talor, C. R., and Youngquist, G. A. (1990). Visual perception and the guidance of locomotion without vision to previously seen targets. Perception 19, 675–689. doi: 10.1068/p190675
Rieser, J. J., Pick, H. L., Ashmead, D. H., and Garing, A. E. (1995). Calibration of human locomotion and models of perceptual-motor organization. J. Exp. Psychol. Hum. Percept. Perform. 21, 480–497. doi: 10.1037/0096-1523.21.3.480
Ross, J., Morrone, M. C., and Burr, D. C. (1997). Compression of visual space before saccades. Nature 386, 598–601. doi: 10.1038/386598a0
Rossetti, Y., Stelmach, G., Desmurget, M., Prablanc, C., and Jeannerod, M. (1994). The effect of viewing the static hand prior to movement onset on pointing kinematics and variability. Exp. Brain. Res. 101, 323–330. doi: 10.1007/BF00228753
Salimi, I., Hollender, I., Frazier, W., and Gordon, A. M. (2000). Specificity of internal representations underlying grasping. J. Neurophysiol. 84, 2390–2397. doi: 10.1152/jn.2000.84.5.2390
Sanz Diez, P., Bosco, A., Fattori, P., and Wahl, S. (2022). Horizontal target size perturbations during grasping movements are described by subsequent size perception and saccade amplitude. PLoS One 17, e0264560. doi: 10.1371/journal.pone.0264560
Sarlegna, F. R., and Sainburg, R. L. (2009). The Roles of Vision and Proprioception in the Planning of Reaching Movements, p. 317–335. doi: 10.1007/978-0-387-77064-2_16
Schlag, J., and Schlag-Rey, M. (1995). Illusory localization of stimuli flashed in the dark before saccades. Vision. Res. 35, 2347–2357. doi: 10.1016/0042-6989(95)00021-Q
Schubö, A., Aschersleben, G., and Prinz, W. (2001). Interactions between perception and action in a reaction task with overlapping S-R assignments. Psychol. Res. 65, 145–157. doi: 10.1007/s004260100061
Sedgwick, H. (1986). “Space perception.,” in Handbook of perception and human performance: Vol I. Sensory processes and perception, eds Thomas (New York, NY: Wiley.), p. 21–57.
Siegel, Z. D., Kelly, J. W., and Cherep, L. A. (2017). Rescaling of perceived space transfers across virtual environments. J. Exp. Psychol. Hum. Percept. Perform. 43, 1805–1814. doi: 10.1037/xhp0000401
Stefanucci, J. K., and Geuss, M. N. (2009). Big people, little world: the body influences size perception. Perception 38, 1782–1795. doi: 10.1068/p6437
Sutton, R., and Barto, A. (1998). Reinforcement Learning: An Introduction. London: The MIT Press. doi: 10.1109/TNN.1998.712192
Symes, E., Tucker, M., Ellis, R., Vainio, L., and Ottoboni, G. (2008). Grasp preparation improves change detection for congruent objects. J. Exp. Psychol. Hum. Percept. Perform. 34, 854–871. doi: 10.1037/0096-1523.34.4.854
Taylor, J. E. T., Witt, J. K., and Sugovic, M. (2011). When walls are no longer barriers: perception of wall height in parkour. Perception 40, 757–760. doi: 10.1068/p6855
Thut, G., Miniussi, C., and Gross, J. (2012). The functional importance of rhythmic activity in the brain. Current Biology 22, R658–R663. doi: 10.1016/j.cub.2012.06.061
Tomassini, A., Ambrogioni, L., Medendorp, W. P., and Maris, E. (2017). Theta oscillations locked to intended actions rhythmically modulate perception. Elife 6, e25618. doi: 10.7554/eLife.25618.014
Tomassini, A., Spinelli, D., Jacono, M., Sandini, G., and Morrone, M. C. (2015). Rhythmic oscillations of visual contrast sensitivity synchronized with action. J. Neurosci. 35, 7019. doi: 10.1523/JNEUROSCI.4568-14.2015
Valsecchi, M., Cassanello, C., Herwig, A., Rolfs, M., and Gegenfurtner, K. R. (2020). A comparison of the temporal and spatial properties of trans-saccadic perceptual recalibration and saccadic adaptation. J. Vis. 20, 2. doi: 10.1167/jov.20.4.2
Valsecchi, M., and Gegenfurtner, K. R. (2016). Dynamic re-calibration of perceived size in fovea and periphery through predictable size changes. Curr. Biol. 26, 59–63. doi: 10.1016/j.cub.2015.10.067
van Beers, R. J., Baraduc, P., and Wolpert, D. M. (2002). Role of uncertainty in sensorimotor control. Philos. Trans. R Soc. Lond. B Biol. Sci. 357, 1137–1145. doi: 10.1098/rstb.2002.1101
Velay, J., and Beaubaton, D. (1986). Influence of visual context on pointing movement accuracy. Cahiers de Psychologie Cognitive 6, 447–456.
Vishwanath, D., and Kowler, E. (2003). Localization of shapes: eye movements and perception compared. Vision. Res. 43, 1637–1653. doi: 10.1016/S0042-6989(03)00168-8
Waller, D., and Richardson, A. R. (2008). Correcting distance estimates by interacting with immersive virtual environments: Effects of task and available sensory information. J. Exp. Psychol. Appl. 14, 61–72. doi: 10.1037/1076-898X.14.1.61
Warren, W. H. (1984). Perceiving affordances: visual guidance of stair climbing. J. Exp. Psychol. Hum. Percept. Perform. 10, 683–703. doi: 10.1037/0096-1523.10.5.683
Warren, W. H., and Whang, S. (1987). Visual guidance of walking through apertures: body-scaled information for affordances. J. Exp. Psychol. Hum. Percept. Perform. 13, 371–383. doi: 10.1037/0096-1523.13.3.371
Watanabe, S., Ogino, S., Nakamura, T., and Koizuka, I. (2003). Saccadic adaptation in the horizontal and vertical directions in normal subjects. Auris Nasus Larynx 30, 41–45. doi: 10.1016/S0385-8146(02)00119-0
Westling, G., and Johansson, R. S. (1984). Factors influencing the force control during precision grip. Exp. Brain Res. 53, 6. doi: 10.1007/BF00238156
Witt, J. (2018). “Perception and Action,” in Stevens' Handbook of Experimental Psychology and Cognitive Neuroscience (New York, NY: Wiley), p. 489–523. doi: 10.1002/9781119170174.epcn211
Witt, J. K. (2011). Tool use influences perceived shape and perceived parallelism, which serve as indirect measures of perceived distance. J. Exp. Psychol. Hum. Percept. Perform. 37, 1148–1156. doi: 10.1037/a0021933
Witt, J. K., and Proffitt, D. R. (2005). See the ball, hit the ball: apparent ball size is correlated with batting average. Psychol. Sci. 16, 937–938. doi: 10.1111/j.1467-9280.2005.01640.x
Witt, J. K., and Proffitt, D. R. (2008). Action-specific influences on distance perception: a role for motor simulation. J. Exp. Psychol. Hum. Percept. Perform. 34, 1479–1492. doi: 10.1037/a0010781
Witt, J. K., Proffitt, D. R., and Epstein, W. (2004). Perceiving distance: a role of effort and intent. Perception 33, 577–590. doi: 10.1068/p5090
Witt, J. K., Proffitt, D. R., and Epstein, W. (2005). Tool use affects perceived distance, but only when you intend to use it. J. Exp. Psychol. Hum. Percept. Perform. 31, 880–888. doi: 10.1037/0096-1523.31.5.880
Witt, J. K., and Sugovic, M. (2010). Performance and ease influence perceived speed. Perception 39, 1341–1353. doi: 10.1068/p6699
Wittenberg, M., Bremmer, F., and Wachtler, T. (2008). Perceptual evidence for saccadic updating of color stimuli. J. Vis. 8, 9. doi: 10.1167/8.14.9
Wohlschläger, A. (2000). Visual motion priming by invisible actions. Vision. Res. 40, 925–930. doi: 10.1016/S0042-6989(99)00239-4
Wolpert, D. M., Ghahramani, Z., and Jordan, Michael, I. (1995). Are arm trajectories planned in kinematic or dynamic coordinates? an adaptation study. Exp. Brain. Res. 5, 103. doi: 10.1007/BF00241505
Woodworth, R. S. (1899). Accuracy of voluntary movement. Psychologic. Rev. Monograp. Suppl. 3, i−114. doi: 10.1037/h0092992
Wühr, P., and Müsseler, J. (2001). Time course of the blindness to response-compatible stimuli. J. Exp. Psychol. Hum. Percept. Perform. 27, 1260–1270. doi: 10.1037/0096-1523.27.5.1260
Wykowska, A., Hommel, B., and Schubo, A. (2011). Action-induced effects on perception depend neither on element-level nor on set-level similarity between stimulus and response sets. Atten. Percept. Psychophys. 73, 1034–1041. doi: 10.3758/s13414-011-0122-x
Wykowska, A., and Schubö, A. (2012). Action intentions modulate allocation of visual attention: electrophysiological evidence. Front. Psychol. 3, 12. doi: 10.3389/fpsyg.2012.00379
Wykowska, A., Schubö, A., and Hommel, B. (2009). How you move is what you see: action planning biases selection in visual search. J. Exp. Psychol. Hum. Percept. Perform. 35, 1755–1769. doi: 10.1037/a0016798
Zelinsky, G. J., Rao, R. P. N., Hayhoe, M. M., and Ballard, D. H. (1997). Eye movements reveal the spatiotemporal dynamics of visual search. Psychol. Sci. 8, 448–453. doi: 10.1111/j.1467-9280.1997.tb00459.x
Zimmermann, E., and Lappe, M. (2010). Motor signals in visual localization. J. Vis. 10, 1–11. doi: 10.1167/10.6.2
Zimmermann, E., Morrone, M. C., and Burr, D. (2015). Visual mislocalization during saccade sequences. Exp. Brain. Res. 233, 577–585. doi: 10.1007/s00221-014-4138-z
Zimmermann, E., Morrone, M. C., and Burr, D. C. (2014). The visual component to saccadic compression. J. Vis. 14, 13. doi: 10.1167/14.12.13
Zwickel, J., Grosjean, M., and Prinz, W. (2007). Seeing while moving: measuring the online influence of action on perception. Q. J. Experiment. Psychol. 60, 1063–1071. doi: 10.1080/17470210701288722
Keywords: object properties, reaching, grasping, eye movements, walking
Citation: Bosco A, Sanz Diez P, Filippini M and Fattori P (2023) The influence of action on perception spans different effectors. Front. Syst. Neurosci. 17:1145643. doi: 10.3389/fnsys.2023.1145643
Received: 16 January 2023; Accepted: 10 April 2023;
Published: 02 May 2023.
Edited by:
Preston E. Garraghty, Indiana University Bloomington, United StatesReviewed by:
Yu Liu, University of Minnesota Medical School, United StatesCopyright © 2023 Bosco, Sanz Diez, Filippini and Fattori. This is an open-access article distributed under the terms of the Creative Commons Attribution License (CC BY). The use, distribution or reproduction in other forums is permitted, provided the original author(s) and the copyright owner(s) are credited and that the original publication in this journal is cited, in accordance with accepted academic practice. No use, distribution or reproduction is permitted which does not comply with these terms.
*Correspondence: Annalisa Bosco, YW5uYWxpc2EuYm9zY28yQHVuaWJvLml0
Disclaimer: All claims expressed in this article are solely those of the authors and do not necessarily represent those of their affiliated organizations, or those of the publisher, the editors and the reviewers. Any product that may be evaluated in this article or claim that may be made by its manufacturer is not guaranteed or endorsed by the publisher.
Research integrity at Frontiers
Learn more about the work of our research integrity team to safeguard the quality of each article we publish.