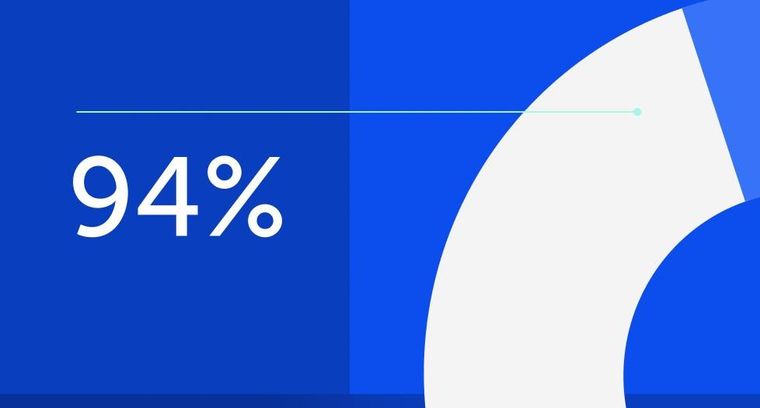
94% of researchers rate our articles as excellent or good
Learn more about the work of our research integrity team to safeguard the quality of each article we publish.
Find out more
REVIEW article
Front. Syst. Neurosci., 14 December 2022
Volume 16 - 2022 | https://doi.org/10.3389/fnsys.2022.1044686
This article is part of the Research TopicEndogenous Opioids in Systems NeuroscienceView all 10 articles
Due to the prevalence of chronic pain worldwide, there is an urgent need to improve pain management strategies. While opioid drugs have long been used to treat chronic pain, their use is severely limited by adverse effects and abuse liability. Neurostimulation techniques have emerged as a promising option for chronic pain that is refractory to other treatments. While different neurostimulation strategies have been applied to many neural structures implicated in pain processing, there is variability in efficacy between patients, underscoring the need to optimize neurostimulation techniques for use in pain management. This optimization requires a deeper understanding of the mechanisms underlying neurostimulation-induced pain relief. Here, we discuss the most commonly used neurostimulation techniques for treating chronic pain. We present evidence that neurostimulation-induced analgesia is in part driven by the release of endogenous opioids and that this endogenous opioid release is a common endpoint between different methods of neurostimulation. Finally, we introduce technological and clinical innovations that are being explored to optimize neurostimulation techniques for the treatment of pain, including multidisciplinary efforts between neuroscience research and clinical treatment that may refine the efficacy of neurostimulation based on its underlying mechanisms.
Over 20% of people worldwide suffer from chronic pain disorders (Goldberg and McGee, 2011). In response to an unmet need for effective pain management, opioid drugs have been widely adopted. Opioid drugs harness the body’s endogenous opioid receptors, which are dispersed throughout the central and peripheral nervous system to modulate pain perception. While prescription opioids often provide effective pain relief, they have undesirable and potentially dangerous side effects including abuse liability and respiratory depression. Their contribution to the ongoing opioid epidemic and the enormous negative impact of chronic pain underscore the need for safe and effective pain therapies (Manchikanti et al., 2012). Neurostimulation therapies are potential alternatives for managing medically refractory pain. However, these therapies are hampered by inconsistent pain relief across patients and diminishing analgesic effects over time (Kumar K. et al., 1998). To optimize these therapies and predict patient responses, we must first understand the mechanisms of action underlying their therapeutic effects. The purpose of this review is to summarize the evidence suggesting current neurostimulation therapies may provide analgesia in part by driving endogenous opioid mechanisms. We conclude by discussing opportunities for multidisciplinary research to shed new light on mechanisms of neurostimulation-induced pain relief.
Chronic pain is a condition often defined by the presence of long-standing pain that persists beyond recovery of the injured tissue. In humans, chronic pain is clinically defined as pain that persists for longer than 6 months (Russo and Brose, 1998), without regard to tissue healing. One type of severe chronic pain for which neurostimulation techniques are often used is neuropathic pain, which is defined by the International Association for the Study of Pain as “pain caused by a lesion or disease of the somatosensory system” (Jensen et al., 2011). In the United States, an estimated 20.5% of adults suffer from a chronic pain condition, with 10% experiencing high-impact chronic pain that limits work and diminishes quality of life (Yong et al., 2022). This figure is mirrored by an estimated global prevalence of chronic pain of 18% (Sá et al., 2019). Many patients experiencing chronic pain are inadequately treated, with estimates ranging from 40 to 77% depending on pain etiology and study parameters (Deandrea et al., 2008; Majedi et al., 2019). Due to its high prevalence worldwide, there is a clear and urgent need for safe and effective therapies for managing chronic pain.
Prescription opioids have major drawbacks that limit their tolerability, effectiveness, and safety. Opioids produce disorienting psychoactive effects which can interfere with daily activities. Opioid use can cause constipation which produces significant discomfort. Repeated opioid use leads to adaptations in opioid receptor signaling, such as receptor desensitization, internalization, and augmented downstream signaling pathways, which are thought to differentially contribute to tolerance and limit effectiveness in treating pain (von Zastrow et al., 2003; Gintzler and Chakrabarti, 2006; Martini and Whistler, 2007). Activation of opioid receptors in circuits that control breathing induces strong respiratory depression that leads to death at high doses, with opioid-related deaths rising steadily over the past 20 years and continuing at epidemic levels (Rudd et al., 2016; Scholl et al., 2019). Coupled with the rewarding aspects of opioid signaling that reinforce drug consumption, respiratory depression is the most dangerous aspect of opioid analgesics, as it is responsible for the large number of opioid overdose deaths. There is thus an urgent demand for novel effective and tolerable treatment paradigms to lessen suffering of chronic pain patients, a mission that has been recently prioritized by the US National Institutes of Health (Collins et al., 2018).
Opioid receptors are expressed throughout the nervous system, including the cortex, midbrain, brainstem, spinal cord, and in the presynaptic terminals of the primary afferents of the dorsal root ganglion (le Merrer et al., 2009). Due to its prominence as the primary target of opioid analgesics, most studies of pain revolve around the μ-opioid receptor (MOR). However, the δ- and κ-opioid receptors (DORs and KORs) are also important in pain modulation (Fields, 2004; Corder et al., 2018). MORs are activated by the endogenous opioid neuropeptides enkephalin, beta-endorphin, and dynorphin. Enkephalins, of which there are two forms that differ in their C-terminal amino acid ([Met5]-enkephalin and [Leu5]-enkephalin), also activate DORs with similar affinity (Toll et al., 1998; Gomes et al., 2020). Beta-endorphin, which includes [Met5]-enkephalin at its N-terminus, is usually considered MOR-selective but can also activate DORs and KORs, with notable signaling bias toward downstream G-protein signaling compared to beta-arrestin signaling at MORs observed in vitro (Gomes et al., 2020). Several opioid peptides that can be described as short, C-terminally extended forms of [Met5]-enkephalin have also been isolated from mammalian brains; one of which (Met-enkephalin-Arg-Phe) has been recently demonstrated to act at MORs when released endogenously (Trieu et al., 2022). Several dynorphin peptides of different length and sequence are prominent in the mammalian nervous system. Although dynorphins are usually considered KOR agonists due their high affinity for KORs (especially the longer forms), they can also activate MORs and DORs at physiologically relevant concentrations (Toll et al., 1998; Gomes et al., 2020).
It is generally assumed that endogenous opioids produce pain relief through MOR activation. The most unequivocal experimental manipulation in humans implicating endogenous opioids in pain is the administration of naloxone, which is a non-specific opioid antagonist that acts on MORs, DORs, and KORs in a similar concentration range. Thus, endogenous opioids may impart some of their antinociceptive effects through activation of DORs and KORs, in addition to MORs.
Pain information is processed by two broad pathways: the ascending nociceptive pathway and the descending pain modulatory system (DPMS). The ascending pathway begins in peripheral nociceptors, which encode painful stimuli and synapse onto projection neurons and interneurons in the spinal cord dorsal horn (DH). Ascending pathways include the spinothalamic, spinomesencephalic, and spinoreticular tracts, which target the thalamus, midbrain areas such as the periaqueductal gray (PAG), and the brainstem reticular formation, respectively. Within the spinothalamic tract, subdivisions that target the lateral thalamus and onto the somatosensory cortices and insula are considered to mediate the sensory-discriminative aspects of pain (i.e., the sensory experience of pain involved in reflexive pain behaviors such as limb withdrawal in response to noxious stimuli). Spinothalamic subdivisions that target the medial thalamus and onto the anterior cingulate cortex (ACC) are thought to contribute to the affective percept of pain (i.e., the emotional-motivational experience of pain which is non-reflexive). The descending pain modulatory pathway begins in the PAG. Canonically, ventrolateral PAG (vlPAG) projects to the rostroventral medulla (RVM), which in turn sends projections to the DH to gate spinal outflow of incoming pain information. A brief overview of key brain areas that encode and modulate pain for the understanding of neurostimulation-induced analgesia follows. Schematics of the location, circuitry, and opioid receptor expression in brain areas within the descending and ascending pathways most relevant for current neurostimulation techniques for the treatment of chronic pain are shown in Figure 1.
Figure 1. Overview of three neural structures that have been targeted by neurostimulation therapies. Schematic of ascending (purple) and descending (blue) pain modulatory pathways (left). Middle: Macro level anatomy of the cortex, brainstem and spinal cord, showing key nodes in the ascending and descending pain modulatory pathways. Connections between the brainstem and spinal cord via the RVM are indicated. Right: Select synaptic connections and microcircuitry of the ACC, vlPAG and DH are shown. Mu-and delta-opioid receptors are expressed on cell bodies and pre-synaptic terminals of neurons throughout the pain neuraxis to modulate ascending and descending pain pathways. ACC, anterior cingulate cortex; RVM, rostroventromedial medulla; vlPAG, ventrolateral periaqueductal gray; LC, locus coeruleus; DH, dorsal horn.
The PAG, a heterogenous midbrain region known for its roles in divergent behaviors such as defensive responses and vocalization (Behbehani, 1995), represents the first major hub in the DPMS. In the context of the pain, PAG receives and consolidates top-down input from numerous cortical and subcortical regions, including the prefrontal cortex (PFC), ACC, anterior insula, and amygdala (Hardy and Leichnetz, 1981; Bingel et al., 2006; Lu et al., 2016; Cheriyan and Sheets, 2018; Li and Sheets, 2018; Rozeske et al., 2018; Huang et al., 2019; Zhu et al., 2021). In addition to the RVM and nearby noradrenergic nuclei, the PAG displays broad ascending efferent projections to brain regions such as the thalamus, hypothalamus, and ventral tegmental area (Cameron et al., 1995a,b; Linnman et al., 2012; Ntamati et al., 2018). Though human tractography studies indicate some differences in PAG cortical connectivity between rodents and humans, midbrain and hindbrain connectivity is conserved, which is critical to our understanding of neurostimulation techniques that may harness descending pain modulatory mechanisms (Ezra et al., 2015; Menant et al., 2016).
In the rodent, the anatomy and function of the PAG opioid system has been extensively studied and recently reviewed by Bagley and Ingram, 2020. The canonical circuit by which opioids signal in the PAG follows a disinhibitory mechanism: MORs are highly expressed on local vlPAG GABAergic interneurons that provide tonic inhibition onto PAG projection neurons. In the presence of endogenous or exogenous opioids, these inhibitory inputs are suppressed by MOR signaling, leading to the disinhibition of glutamatergic PAG-RVM projections (Lau and Vaughan, 2014). The resultant activation of descending GABAergic, opioidergic and serotonergic RVM neurons directly inhibits spinal cord neurons to suppress nociception (Salas et al., 2016; Weiwei et al., 2021).
In line with this hypothesis, vlPAG microinfusion of glutamate receptor agonists and GABA receptor antagonists produces antinociception in rodents (Moreau and Fields, 1986; Jones and Gebhart, 1988; Jensen and Yaksh, 1989). More recently, modern chemogenetic methods in behaving rodents indicate that activation of glutamatergic vlPAG neurons or inhibition of GABAergic neurons is antinociceptive, while inhibition of glutamatergic neurons or activation of GABAergic neurons is pronociceptive, although the opioid dependence of this analgesia was not examined (Samineni et al., 2017). Local opioid infusion in the PAG, especially vlPAG, has long been noted for its strong antinociceptive properties in rodents (Yaksh, 1979; Jones and Gebhart, 1988; Jensen and Yaksh, 1989). MORs can also be found, however, in a subpopulation of PAG projection neurons (Wang and Wessendorf, 2002; Bagley and Ingram, 2020), suggesting that this accepted circuitry may not account for non-canonical or bidirectional signaling from PAG to RVM, which may involve competing facilitation and inhibition. Indeed, about half of RVM-projecting PAG neurons are actually hyperpolarized by MOR agonists (Osborne et al., 1996; Umana et al., 2017).
Using functional imaging in humans, PAG activity has been implicated in a multitude of functions, from pain-and placebo-related conditions to homeostatic bodily processes and the manifestation of negative emotional states in panic and depression (Zhao, 2008; George et al., 2019). For a comprehensive review of human functional imaging of PAG, we recommend the meta-analysis provided by Linnman et al., 2012. In brief, many studies have found pain-induced PAG activation in response to noxious stimuli such as heat, cold, pressure, and light touch on allodynic regions, as well as in chronic pain conditions such as neuropathic pain. PAG fMRI indicates its functional connectivity at rest with ACC and RVM (Kong et al., 2010), and this ACC-PAG interaction correlates with attentional analgesia and can be disrupted by opioid antagonists (Oliva et al., 2022). Placebo conditioning in humans increases PAG activity during the anticipation of a painful stimulus (Wager et al., 2004) and induces coupling of ACC and PAG activity that is sensitive to systemic naloxone (Eippert et al., 2009). Due to the abundance of opioid receptors expressed, PAG is thought to play a key role in pain modulation produced by exogenous and endogenous opioids. In humans, PET imaging of [11C]-carfentanil indicates a decrease in radiotracer binding and therefore an increase in PAG endogenous opioid signaling in response to pain (Zubieta et al., 2005) and placebo analgesia (Scott et al., 2008).
Rostroventral medulla (RVM) receives inputs from PAG and sends projections to the DH to modulate spinal signaling through GABAergic, serotonergic, and opioidergic mechanisms (Millan, 2002; François et al., 2017). RVM neurons are categorized as ON, OFF, and neutral cells based on their electrophysiological responses to noxious stimuli and during nocifensive responses. RVM receives input from the PAG and has recently been shown to receive synaptic connections from the parabrachial nucleus (Chen et al., 2017). RVM outputs relevant for pain modulation include the spinal cord and midbrain and brainstem noradrenergic nuclei (Clark and Proudfit, 1991a).
Like PAG, RVM is a known locus of exogenous and endogenous opioids in pain modulation (Bagley and Ingram, 2020). RVM neurons express opioid receptors in serotonergic and non-serotonergic neurons that project to the spinal cord (Gutstein et al., 1998; Wang and Wessendorf, 1999). Supporting a role for endogenous opioids, all three opioid receptor types are also expressed by terminals in the neuropil around RVM neurons (Kalyuzhny et al., 1996; Gutstein et al., 1998). RVM receives input from enkephalinergic terminals and some RVM neurons are enkephalinergic, including a subset of spinally-projecting GABAergic neurons (Khachaturian et al., 1983; Zhang et al., 2015). In addition to enkephalins, RVM receives dynorphinergic input from PAG and contains KOR-expressing spinally-projecting neurons that inhibit pain and itch via descending mechanisms (Nguyen et al., 2022). RVM may also contain dynorphin-expressing neuronal cell bodies (Menetrey and Basbaum, 1987). Application of opioids to the RVM leads to the increase in activity of antinociceptive OFF-cells and the decrease in spiking of pronociceptive ON-cells (Heinricher et al., 1994) as well as strong antinociception in rodents (Dickenson et al., 1979; Azami et al., 1982).
Rodent intrathecal pharmacological studies have long implicated spinal noradrenergic signaling as a key component in supraspinal influence on pain suppression (Yaksh, 1979; Proudfit and Hammond, 1981; Hammond and Yaksh, 1984; West et al., 1993). The locus coeruleus (LC) (A6), brainstem (A5), and midbrain (A7) noradrenergic cell groups display projections to the spinal cord in parallel with the RVM (Westlund et al., 1983, 1984; Clark and Proudfit, 1991b,c, 1993; Proudfit and Clark, 1991; Bruinstroop et al., 2012; Li et al., 2016; Hirschberg et al., 2017) and receive anatomical input from canonical DPMS nuclei PAG and RVM (Clark and Proudfit, 1991a; Bajic and Proudfit, 1999).
Locus coeruleus (LC) highly expresses opioid receptors (Pert et al., 1976) and LC neuron activity is directly suppressed by both endogenous and exogenous opioids (Williams et al., 1982). Opioid receptor expression in LC, A5, and A7 neurons appears to be limited to MORs (Williams and North, 1984; North et al., 1987; Guajardo et al., 2017), although a subset of presynaptic terminals in these areas have been shown to express DORs (Arvidsson et al., 1995; van Bockstaele et al., 1997; Holden et al., 1999; Erbs et al., 2015). Additionally, LC and the pericoerulear region are densely innervated by enkephalin-expressing terminals (Drolet et al., 1992). Microinfusion of morphine directly into the LC is antinociceptive in rodents (Bodnar et al., 1988).
The spinal cord, especially the DH, is the ultimate target of the DPMS. Release of neuromodulators and neurotransmitters in the DH from descending sources modulates spinal outflow of ascending nociceptive information arriving from the periphery. Aδ and C nociceptive fibers terminate onto DH superficial laminae I projection neurons that respond to high threshold stimulation, as well as onto deeper layer V wide dynamic range projection neurons. Most neurons in the laminae II-III, however, are not supraspinally-projecting, but instead are excitatory or inhibitory interneurons that signal locally in the spinal cord. It is thought that descending fibers from the midbrain and brainstem can terminate onto primary afferent terminals, spinal interneurons, and spinal projection neurons to modulate the spinal circuit response to incoming pain information at multiple levels (Mannion and Woolf, 2000; D’Mello and Dickenson, 2008). In addition to neurotransmitters, spinal pain transmission is also modulated by a complicated combination of other neurochemicals such as neurokinins, CGRP, somatostatin, and opioids (Dickenson, 1995).
Endogenous opioid peptides and receptors play a substantial role in spinal cord pain-related activity. The rat spinal cord predominantly expresses MORs, but also exhibits some DORs and very low KOR expression. Within each of these receptor subtypes, all show predominant expression on presynaptic terminals entering the DH, with a smaller proportion on postsynaptic neurons (Besse et al., 1990; Dickenson, 1995). Recordings from DH neurons during intrathecal morphine application show that C and Aδ fibers that convey noxious information are the most highly inhibited by morphine, while the pain evoked activity of larger Aβ mechanosensory fibers is only mildly opioid-modulated (Dickenson and Sullivan, 1986; Heinke et al., 2011). Intrathecal application of enkephalin is analgesic (Yaksh et al., 1977), presumably due to activation of the same opioid receptors affected by morphine. Enkephalin- and dynorphin-immunoreactive cell bodies and fibers are present in the DH, suggesting that endogenous opioid peptides are released in the DH locally and by descending mechanisms (Seybold and Elde, 1980; Harlan et al., 1987; Marvizón et al., 2009; François et al., 2017). However, parsing the contribution of local and descending opioid release has been experimentally challenging.
The thalamus receives nociceptive information directly from the spinal cord and relays it to the cortex (Ab Aziz and Ahmad, 2006). The spinothalamic tract conveys information about non-noxious and noxious stimuli to the lateral and medial thalamus. The lateral thalamic ventral posterolateral (VPL) and ventral posteromedial (VPM) nuclei project to the somatosensory cortex and relay tactile, proprioceptive, and nociceptive signals from the body and face, respectively (Monconduit et al., 1999; Alitto and Usrey, 2003). Medial thalamic nuclei receive additional nociceptive information from ascending spinal tracts. These nuclei transmit information thought to be related to the affective components of pain to areas involved in emotional processing, such as the ACC and the insular cortices (Friedman and Murray, 1986). A study in rats found a functional correlation between medial thalamus and ACC activity during electrical stimulation, supporting the idea that thalamus conveys information on the affective components of pain through this projection (Shyu et al., 2004). Among the medial thalamic nuclei, the mediodorsal nucleus (MD) is the major source of inputs to the ACC. Also implicated in pain processing is the medial thalamic nucleus submedius (Sm), which projects to the ventrolateral orbital cortex (VLO) and on to the PAG, a pathway that has been shown to mediate antinociception (Zhang et al., 1995; Huang et al., 2021). Imaging and electrophysiology studies in both animals and humans have also found that, like ACC, the MD is hyperactive in chronic pain conditions (Whitt et al., 2013; Meda et al., 2019). In mice with neuropathic pain, optogenetic activation of MD inputs to ACC induces behavioral avoidance and is considered aversive (Meda et al., 2019).
A meta-analysis of published fMRI data in humans with acute, experimentally-induced and chronic pain showed that the thalamus is active in both conditions (Friebel et al., 2011). Chronic pain patients show altered thalamic regional cerebral blood flow (rCBF) and several imaging studies suggest that altered thalamic activity is involved in the development of neuropathic pain (Witting et al., 2001; Casey et al., 2003). Studies in animal models of neuropathic pain have also shown a correlation between chronic pain and changes in biochemistry and immediate early gene expression in the thalamus (Narita, 2003).
Opioid receptors are widely expressed in the thalamus. High levels of MOR mRNA are observed in several thalamic nuclei, including the medial habenula, laterodorsal, paraventricular, centromedial, and reuniens nuclei. DOR mRNA expression is also observed in the thalamus, but KOR mRNA expression is limited to fewer nuclei in the paraventricular and zona incerta (Mansour et al., 1994; Erbs et al., 2015). In rodent brain slices, thalamic output to ACC and dorsal striatum is suppressed in the presence of a MOR agonist, indicating the sensitivity of thalamic output to opioids and suggesting the attenuation of noxious information relay to cortex during opioid treatment (Birdsong et al., 2019). In rodents, pharmacological blockade of MORs in the dorsal midline thalamus induced a fear memory extinction deficit (Bengoetxea et al., 2020), while stimulation of MORs caused increased locomotor activity associated with decreased freezing extinction. These data suggest that targeting dorsal midline thalamus MORs could have therapeutic effects on stress-related and anxiety disorders. Animal research using both electrophysiology and EEG points to the medial thalamus as the primary site of morphine action (Linseman and Grupp, 1980). Indeed, morphine microinfused in the medial or intralaminar thalamic nuclei has been shown in a small number of rodent studies to produce analgesia (Carr and Bak, 1988; Wang et al., 2006; Erfanparast et al., 2015). Consistently, studies in both humans measuring [11C]diprenorphine binding via PET imaging and rodents have found lower opioid receptor availability in chronic pain conditions in the thalamus, ACC, posterior temporal and orbitofrontal cortices, as well as in the posterior midbrain (Thompson et al., 2018).
The ACC refers to a subregion of frontal cortex with heterogenous subdivisions that are differentially involved in the affective, cognitive, and emotional components of pain processing (Bush et al., 2000; Vogt, 2005; Heilbronner and Hayden, 2016). In humans, ACC receives inputs from the anterior insular cortex (aI) (Peltz et al., 2011; Wiech et al., 2014) and amygdala (Sharma et al., 2020). It receives ascending noxious sensory information mainly via the medial thalamic nuclei (Xiao and Zhang, 2018). The ACC pain-aversive response can be increased by inputs from the primary somatosensory cortex on a subset of ACC neurons (Singh et al., 2020). Several pieces of evidence suggest that projections from ACC to the brainstem, specifically through the PAG or by way of the medial thalamic nuclei, are important for the cortical contribution to opioid analgesia and to placebo analgesia (Hardy and Leichnetz, 1981; Royce, 1983; Devinsky et al., 1995). ACC also sends reciprocal projections to the amygdala (Allsop et al., 2018) and insular cortex; while functional connectivity between these regions is associated with negative affective states (Shao et al., 2018), the role of this circuitry in the emotional and affective components of pain remains to be determined.
Early single neuron recordings in cingulotomy patients showed that ACC neurons respond selectively to mechanical and thermal painful stimuli, but not to innocuous stimuli (Hutchison et al., 1999). Likewise, single-unit recordings in rabbits demonstrate that ACC neurons which respond to noxious stimuli have diffuse receptive fields covering the entire body (Sikes and Vogt, 1992). In non-human primates, ACC neurons were reported to encode the integration of nociception, specifically the anticipation of pain following cutaneous electric stimulation (Koyama et al., 1998). Interestingly, ACC activation has also been observed during placebo-induced analgesia (Wager et al., 2004), though this activation may occur in a different substructure than that activated by noxious stimuli. Subsequent human fMRI and PET studies further confirm that ACC is activated by noxious stimuli (Kwan et al., 2000) and the response magnitude correlates with stimulus intensity and changes in the perceived unpleasantness of painful stimuli (Vogt et al., 1996; Rainville et al., 1997; Tölle et al., 1999). Together, these findings confirm that nociceptive stimuli activate ACC across species.
Arguing against a simple role for the ACC in nociception, patients with ACC lesions experience reduced pain-related unpleasantness and reduced avoidance of noxious stimuli, but their ability to identify intensity and location of noxious stimuli remains intact (Foltz and White, 1962; Ballantine et al., 1967; Wayne Hurt et al., 1974). Similarly, microinjection of excitatory amino acids into the ACC in naïve rodents elicits conditioned place aversion without altering pain thresholds (Johansen and Fields, 2004), while ACC lesions eliminate the aversiveness of neuropathic pain but not stimulus-evoked hypersensitivity (Qu et al., 2011). These findings argue against the role of ACC in nociceptive processing per se. Instead, several studies in both humans and rodents have shown that ACC contributes to the unpleasantness of pain (Seminowicz et al., 2009; Fuchs et al., 2014; Bliss et al., 2016). Functional and structural alterations of ACC, such as hyperactivation and reduction of gray matter, have been observed in neuropathic patients and are associated with emotional and psychological pain (Rodriguez-Raecke et al., 2009; Bushnell et al., 2013).
Early human studies reported high [3H]diprenorphine binding in the ACC of healthy subjects but a reduction in patients with central post-stroke pain (Willoch et al., 1999), suggesting that opioids can directly impact aspects of pain processing by binding ACC opioid receptors (Vogt et al., 1995; Jones et al., 1999). Further receptor-imaging studies confirm the involvement of ACC in opioid-dependent analgesia and, intriguingly, suggest a role in placebo analgesia (Petrovic et al., 2002). PET studies performed with [11C]Carfentanil observed endogenous ACC opioid release during placebo analgesia and the consequent endogenous opioid-induced ACC activation correlated with a reduction in pain affect during a sustained painful stimulus (Zubieta et al., 2001). Consistent with this, rodent ACC morphine microinjection selectively suppresses pain affect but not withdrawal responses (LaGraize et al., 2006; Gomtsian et al., 2018).
Opioid receptors are abundantly expressed in the ACC, with MOR expression most prominent in superficial layers (Vogt et al., 1995). MORs are expressed by both cortical neurons and afferent axons from subcortical regions. Presynaptic MORs are predominant on thalamic axonal projections to the ACC (Vogt et al., 1995). This distribution pattern led to the idea that endogenous opioids can regulate nociception by inhibiting the thalamocortical afferents in the ACC or by modulating the activity of interneurons and projection neurons (Navratilova et al., 2015). This model has been recently expanded upon by examining the thalamo-cortico-striatal circuit (Birdsong et al., 2019), whose involvement in pain processing was first described by Rainville et al. (1997). Thalamic inputs to ACC are potently inhibited by MOR agonists, but ACC inputs to dorsomedial striatal neurons are not affected. In contrast, DOR agonists disinhibit ACC pyramidal neurons and allow for the excitation of ACC inputs onto striatal medium spiny neurons. These mechanisms are mediated by different receptors and suggest that opioid-mediated attenuation of nociceptive information transfer to ACC from thalamus may be a primary mechanism by which opioids reduce the negative affective component of pain.
While most frequently studied in the context of executive cognitive function, recent evidence has begun to implicate the PFC in processing acute nociceptive stimuli and in the development of chronic pain. Within the PFC, the dorsolateral PFC (dlPFC) is considered a master regulator of higher order cognitive functions and is also involved in the cognitive and affective modulation of pain (Lorenz et al., 2003), including placebo analgesia (Petrovic et al., 2002). Functional imaging in humans with acute and chronic pain reveal that PFC activity correlates with the activity of pain-implicated regions above, including ACC, insula, and thalamus (Apkarian et al., 2005). Further, it has been posited that PFC-PAG output and reciprocal PFC connections with the amygdala play a role in antinociception, whereas thalamocortical PFC input and PFC output to the basal ganglia may contribute to pain chronicity (Ong et al., 2019). Previous fMRI studies have found that the magnitude of placebo-induced dlPFC activity correlates with an increase in PAG activity, supporting the idea that this circuit is involved in expectancy-based placebo (Wager et al., 2004, 2007). The prelimbic cortex in rodents is often included in definitions of the rodent PFC, and while not considered homologous to dlPFC in primates (Laubach et al., 2018), recent work has revealed a role for this structure in pain processing. Specifically, inflammatory pain decreases both basal firing rate and evoked nociceptive responses in prelimbic neurons (Dale et al., 2018), while inhibition of prelimbic neurons and their outputs to the nucleus accumbens enhances pain responses (Zhou et al., 2018).
The effects of opioids in the PFC are less well-characterized. Rodent PFC neuronal activity has been shown to be opioid sensitive (Williams and Zieglgänsberger, 1981; Giacchino and Henriksen, 1998), while in humans, PET imaging implicates PFC endogenous opioid signaling in placebo-induced analgesia (Wager et al., 2007). Caution is required, however, when attempting to draw parallels between the rodent and human PFC as expansion over the course of evolution has led to more distinct functions and subregions within the human PFC as compared to the rodent (Carlén, 2017; Laubach et al., 2018), with rodents lacking a specific homologue of the dlPFC. Nonetheless, important findings for the implications for PFC in pain signaling may still be gleaned by carefully designing and interpreting experiments and corroborating findings across experimental models.
It is now well-established that the widespread adoption of prescription opioids for the treatment of chronic pain has been instrumental in driving the ongoing opioid epidemic. The continuing burden of untreated chronic pain on patients underscores the need for safe and effective pain therapies. Neurostimulation therapies that target peripheral or central pain mechanisms are promising alternatives for managing medically refractory pain. However, these therapies are hampered by inconsistent pain relief across patients and frequently diminishing analgesic effects over time. Across all neurostimulation therapies, we do not currently understand the physiological mechanisms of action by which these therapies provide pain relief. A clear understanding of the mechanisms of stimulation-induced analgesia is crucial to improve the efficacy of these therapies.
Neurostimulation therapies (Figure 2) are non-addictive, reversible strategies for managing intractable chronic pain. Neurostimulation therapies aim to modulate neural activity through targeted delivery of electrical stimuli to specific regions of the nervous system. In the clinical context, the term “neuromodulation” commonly refers to electrical neurostimulation therapies, but may also refer to targeted drug delivery (e.g., intrathecal pumps), radiofrequency ablation therapies, or modulation of neural activity via ultrasound, which are outside the scope of this review. We use the terms “neuromodulation” and “neurostimulation” interchangeably to describe therapies which use electrical stimulation of the nervous system to treat neurological disorders.
Figure 2. Overview of neurostimulation modalities for the treatment of chronic pain. (Left) Schematic of application of neurostimulation devices for the treatment of chronic pain. (A) DBS electrodes are surgically targeted to specific brain nuclei (i.e., ACC, midline thalamus, PAG) with an external pulse generator. Following optimization of stimulation settings, the pulse generator and leads are internalized under the clavicle to deliver electrical stimulation to the brain. (B) With tDCS, small amounts of electric current are applied externally via electrodes held in place against the scalp. (C) rTMS is applied with an external electromagnetic coil to generate an electromagnetic field in the underlying cortical regions. Both tDCS and rTMS are applied for 20–60 min over repeated sessions without requiring anesthesia. (D) SCS employs implanted electrodes in the epidural space to apply electrical current to the spinal cord. Similar to DBS, SCS patients undergo a trial period to ensure adequate pain relief before the pulse generator and leads are internalized in the posterior flank. (Bottom) For all modalities, several properties of the stimulus waveform can be modulated, including the waveform shape, pulse amplitude, duration, and frequency, as well as whether it is applied continuously, in regular burst patterns or in a closed-loop manner in response to neural activity or patient control. DBS, deep brain stimulation; tDCS, transcranial direct current stimulation; rTMS, repeated transcranial magnetic stimulation; SCS, spinal cord stimulation; IPG, implanted pulse generator.
Neurostimulation therapies range in invasiveness. Non-invasive therapies, such as transcranial direct current stimulation (tDCS), place electrodes on the scalp or magnetic coils proximal to the head. Invasive neurostimulation therapies, such as deep brain stimulation (DBS) or spinal cord stimulation (SCS), involve placing small electrode arrays in the body near the neural structure of interest, which are connected to implantable pulse generators. After electrode placement, a clinician programs the stimulus pulse (i.e., sets the stimulus pulse amplitude, duration, and frequency) to maximize therapeutic effect while minimizing unwanted side effects. These stimulation parameters may be adjusted at follow-up visits to ensure consistent therapeutic benefit.
Neurostimulation has emerged in the past 60 years as an effective therapeutic approach to treating pain and other disorders (Bittar et al., 2005; Moisset et al., 2020). Based on the premise that pain percept is encoded by aberrant patterns of neural activity, the objective of neurostimulation is to alter neural activity in a way that minimizes the experience of pain. Melzack and Wall’s Gate Control Theory of Pain formed the scientific basis for the first modern uses of electrical stimulation-induced pain relief in humans (Melzack and Wall, 1965). This theory suggests that driving the activity of large-diameter afferents may produce pain relief by increasing the activity of inhibitory interneurons in the spinal cord DH. Only 2 years after the publication of the Gate Control Theory, Wall and Sweet demonstrated analgesia via peripheral nerve stimulation (Wall and Sweet, 1967), and Shealy and colleagues demonstrated analgesia via electrical stimulation of the dorsal columns of the spinal cord (Shealy et al., 1967). Conventional neurostimulation theory suggests that extracellular electrical stimulation induces action potentials (APs) in myelinated axons at lower stimulus amplitudes than other neural structures (e.g., non-myelinated axons, cell bodies) (Rattay, 1986, 1999; McIntyre and Grill, 1999). Therefore, electrical stimulation of peripheral nerves and the dorsal columns likely provides analgesia by driving the activity of myelinated tactile afferent axons and feed-forward pain-gating circuitry (Mendell, 2013; Braz et al., 2014; Duan et al., 2018).
The past several decades have produced many innovations in stimulation-induced analgesia. Therapies such as spinal cord stimulation (SCS) are most commonly indicated for neuropathic limb pain conditions, such as failed back surgery syndrome and complex regional pain syndrome. Modern neurostimulation approaches have also been investigated to treat central chronic pain syndromes, such as post-stroke and phantom limb pain (Bittar et al., 2005; Moisset et al., 2020). Furthermore, novel stimulation targets (e.g., deep brain stimulation (DBS) of the ACC (Spooner et al., 2007)) and stimulus pulse paradigms [e.g., burst SCS (de Ridder et al., 2013)] are hypothesized to modulate the neural activity associated with the affective component of pain, rather than affecting circuits associated with the sensory component (e.g., the spinal cord DH). Recent years have seen numerous promising innovations in neurostimulation for pain, and these modalities of exogenous electrical stimulation likely have broad effects across the pain neuraxis, which are not limited to circuits being directly stimulated. This property poses additional challenges to understanding the specific therapeutic mechanisms underlying each neurostimulation technique. Therefore, understanding how different neurostimulation therapies affect specific circuits, such as opioidergic circuits, is crucial to understanding the mechanisms that will ultimately be necessary for optimizing the design and implementation of each therapy.
Spinal cord stimulation (SCS) is the most common neurostimulation therapy, with more than 50,000 SCS systems implanted each year (Sdrulla et al., 2018). SCS is primarily indicated for chronic neuropathic pain of the trunk or limbs which is refractory to conventional medical management (Kumar et al., 2007). SCS is achieved by implanting an electrode array in the dorsal epidural space, either via percutaneous implantation of a cylindrical electrode array, or by implanting a paddle electrode array which requires a laminectomy (Sears et al., 2011). Traditionally, SCS is applied with stimulus pulse frequencies between 40 and 60 Hz, pulse durations between 200 and 600 μs, and pulse amplitudes on the order of several Volts or milliamps for voltage-and current-controlled stimulation, respectively (Kumar R. et al., 1998; Kapural et al., 2015; Malinowski et al., 2020). Recent innovations in SCS technology apply novel stimulus pulse paradigms, particularly with regards to stimulus pulse frequency (Lempka and Patil, 2018). However, few studies have provided evidence regarding the involvement of endogenous opioid mechanisms in analgesia achieved with these novel SCS therapies. Therefore, we will focus our discussion on the possible opioidergic mechanisms of conventional SCS. Furthermore, to the extent that peripherally-targeted neurostimulation therapies such as peripheral nerve stimulation (PNS) (Helm et al., 2021) and dorsal root ganglion stimulation (DRGS) (Deer et al., 2017) engage the CNS, they are hypothesized to directly stimulate similar neural targets as conventional SCS (Lin et al., 2020; Graham et al., 2022). Accordingly, in addition to potentially modulating action potential propagation in nociceptors, these therapies likely engage similar central analgesic mechanisms as with conventional SCS.
Conventional SCS applied with pulse frequencies between ∼40 and 60 Hz evokes paresthesia (i.e., tingling or pins-and-needles sensations) in the area of the body targeted by stimulation. The goal of stimulator programming is to overlap these evoked paresthesias with the patient’s painful region (North et al., 1991). Conventional SCS induces bidirectionally propagating action potentials (APs) in Aβ axons in the dorsal columns (Struijk et al., 1991; Holsheimer, 2002; Zhang et al., 2014; Lempka et al., 2020; Rogers et al., 2022). Antidromically propagating APs enter the dorsal horn caudal to the spinal level where SCS is applied, where they likely provide pain relief by activating feed-forward pain-gating circuitry in the spinal cord. Orthodromically propagating APs are likely responsible for SCS-induced paresthesia (Moffitt et al., 2009) and enter the brain at the brainstem dorsal column nuclei. It is possible that SCS simultaneously engages the endogenous opioid system both via orthodromically propagating APs to the brain and antidromically propagating APs into the DH.
Several brain structures related to the endogenous opioid system have been implicated in the supraspinal mechanisms of action of SCS, such as the PAG, RVM, and thalamic VPL nucleus (Sivanesan et al., 2019). Many studies have examined the role of the DPMS, particularly the GABAergic and serotonergic components, in SCS-induced analgesia (Cui et al., 1996; Song et al., 2009, 2011). Early work in four patients suggested that SCS-induced analgesia is not reversed by naloxone administration, suggesting opioid-independent mechanisms (Freeman et al., 1983). However, this study examined a limited number of patients, and subsequent preclinical work has demonstrated RVM activation during SCS, a structure known to be crucial in endogenous opioid release (Dejongste et al., 1998), leaving the role of opioidergic circuits in SCS-induced analgesia unclear.
In more recent preclinical work, SCS applied to the cervical spinal cord caused dynorphin release in spinal segments caudal to the stimulation site (Ding et al., 2008), suggesting a potential role for segmental opioid release in SCS. In addition, SCS-induced analgesia in rats can be abolished by systemic naloxone, with both SCS-frequency and naloxone-dose dependent effects (Sato et al., 2013). A naloxone dose of 3 mg/kg/h reversed the effects of 4 Hz SCS, but the dose had to be increased to 10 mg/kg/h to reverse the analgesic effects of 60 Hz SCS. Interestingly, administering the DOR antagonist naltrindole abolished analgesia induced by 60 Hz but not by 4 Hz SCS. Finally, a recent preclinical study simultaneously applied SCS and the cholecystokinin (CCK) receptor antagonist proglumide (Inoue et al., 2017). While CCK receptor antagonists typically enhance opioid-dependent analgesia, co-application of SCS and proglumide did not provide enhanced analgesia compared to a single therapy alone. Taken together, these data present a murky picture regarding opioid-dependent analgesia during SCS, warranting continued study into both the involvement of endogenous opioids in SCS-induced analgesia and how SCS pulse parameters influence the engagement of these mechanisms.
Deep brain stimulation (DBS) is a surgical therapy whereby electrode arrays are implanted in discrete nuclei in the brain. Current is then passed through these electrode contacts through a fully implanted pulse generator to manipulate brain activity. Due to its invasiveness, DBS is typically reserved as a late-stage intervention after pharmacological and behavioral treatments have proven ineffective. Brain regions targeted for DBS are often historically identified as sites at which surgical lesions provide some relief for a disorder. Relative to ablative surgery, DBS is reversible and individually programmable, enabling stimulation parameters to be titrated for each patient. Although most commonly used for treatment of movement disorders, indications for DBS have recently expanded to include major depressive disorder, obsessive compulsive disorder, Tourette syndrome, cluster headache, and chronic pain. We focus our discussion on three brain sites that have been targeted clinically for pain relief and highlight evidence for involvement of opioidergic mechanisms in the therapeutic effects of DBS applied to these brain targets.
When targeting PAG, DBS electrodes are placed bilaterally or contralaterally to the site of pain. Some studies indicate that even unilateral electrode placement provides a largely generalized pain relief described as a feeling of warmth and analgesia (Hosobuchi et al., 1977; Boccard et al., 2015). Across multiple case studies, PAG-DBS has proven effective in patients with “nociceptive pain” (Kumar and Wyant, 1985; Levy et al., 1987; Gybels and Kupers, 1990; Kumar et al., 1990), referring to pain generated through ascending dorsal horn input, such as peripheral neuropathic pain, spinal cord injury, plexopathy or phantom limb pain (Prévinaire et al., 2009; Subedi and Grossberg, 2011). Conversely, PAG-DBS exhibits much lower efficacy in centrally generated pain (e.g., post-stroke pain or headache) (Levy et al., 1987; Kumar et al., 1990; Gray et al., 2014; Kashanian et al., 2020). PAG-DBS was largely abandoned in 2000 after two large scale clinical trials (206 total patients) failed to meet clinical endpoints (Coffey, 2001). However, several design and interpretation issues have been raised concerning these studies, including the absence of randomization or placebo control, heterogeneity of the initial pain condition, and attrition of patients from the study which reduced statistical power to detect treatment differences (Shirvalkar et al., 2020). Critically, most data on PAG-DBS has been collected in case series or small clinical trials, without proper randomization or double blinding, the latter of which is arguably unfeasible due to PAG-DBS-induced paresthesia. Though its popularity has decreased, PAG-DBS is still used clinically to treat patients who are treatment refractory with good overall outcomes (Boccard et al., 2013). In the future, patient selection will be a key focus point for refinement to optimize treatment efficacy (Farrell et al., 2018; Frizon et al., 2020).
The therapeutic effects of PAG-DBS are frequency-dependent, with frequencies between 5 and 25 Hz being more efficacious than frequencies above 50 Hz (Nandi et al., 2002; Hentall et al., 2016). Interestingly, patients tended to prefer stimulation frequencies as low as 0.67 Hz (Jermakowicz et al., 2017) and between 5 and 35 Hz (Nandi and Aziz, 2004) when given the opportunity to blindly tune the parameters of their own DBS. It is interesting to note that pain-relieving stimulation in the 5 to 25 Hz range is within the physiological firing frequency of PAG neurons (Yu et al., 2021) and stands in sharp contrast to frequencies classically used to treat movement disorders, which are typically above 100 Hz (Creed, 2018). This supports the interpretation that intermittent activation of PAG descending projections with DBS applied at a physiological firing rate could induce its effects through downstream opioid release.
In rodents with nerve injury, electrical stimulation of vlPAG was effective in reducing spontaneous pain behaviors and mechanical allodynia even 30–40 min after stimulation (Lee et al., 2012). A similar study using acute noxious stimuli found that unilateral vlPAG stimulation produces significant bilateral analgesia in rodents (Wang N. et al., 2016). Both studies state that the mechanism of this analgesia is still unclear, although opioids have been identified as a probable factor due to the concentration of MORs in PAG (Wang and Wessendorf, 2002; Loyd et al., 2008) and the finding that naloxone reverses some of the PAG stimulation-induced analgesia (Mayer et al., 1971; Akil et al., 1976; Morgan et al., 1991). Further downstream, the role of endogenous opioid release in the RVM for antinociception achieved by pharmacological and electrical activation of PAG has been assayed in preclinical models. PAG microinjection of GABA receptor antagonists (to cause PAG disinhibition), morphine, and non-opioid painkillers leads to antinociception that can be blocked by RVM microinfusion of naloxone (Llewelyn et al., 1984; Aimone and Gebhart, 1986; Kiefel et al., 1993; Roychowdhury and Fields, 1996; Vasquez and Vanegas, 2000). The role of endogenous opioid activity in the spinal cord with activation of DPMS by PAG and RVM electrical stimulation is still unclear; these stimulation interventions produce antinociception that can be blocked by intrathecal naloxone in some studies, while others have found a lack of an effect on antinociception by spinal opioid antagonism (Aimone et al., 1987; Miller and Proudfit, 1990; Morgan et al., 1991).
Clinical studies also suggest a role of endogenous opioids in PAG-DBS-induced analgesia. Early studies found that treatment with systemic naloxone blocks the analgesic effects of PAG-DBS in humans (Adams, 1976; Hosobuchi et al., 1977). A more recent study investigating dlPAG DBS-produced local field potentials also found that naloxone reversed the analgesia while increasing the 30–60 Hz band power measured at the same site, but this experiment was restricted to only two human subjects (Pereira et al., 2013). However, in a study of 45 patients with electrodes implanted in the PAG or periventricular gray (PVG), the attenuation of PAG-DBS pain relief by naloxone was similar in magnitude in both active and sham DBS conditions, suggesting the effect of naloxone may not specifically block PAG-DBS, but may instead enhance subjective pain ratings independent of stimulation (Young and Chambi, 1987). A study utilizing PET imaging to observe PAG opioid release found an increase in endogenous release during DBS, but it was not correlated with subjective analgesia (Sims-Williams et al., 2017). Furthermore, upon naloxone treatment, analgesia was still observed, with no significant effect to ongoing pain scores.
Additionally, it has been reported that patients may develop tolerance to chronic PAG-DBS stimulation and cross tolerance to opioids such that morphine becomes less effective after chronic PAG stimulation, suggesting occlusion of descending pain modulatory pathways and endogenous opioid release (Hosobuchi, 1986). However, other studies of PAG-DBS in humans have found tolerance to stimulation in other brain regions that are not presumed to function through endogenous opioid signaling and a lack of cross tolerance to morphine in chronic PAG-DBS (Young et al., 1985; Young and Chambi, 1987; Duncan et al., 1991). Finally, initial reports of endogenous opioid release driven by PAG stimulation in humans found increased enkephalin and beta-endorphin in cerebrospinal fluid of patients that had a positive, pain-relieving response to stimulation (Akil et al., 1978; Hosobuchi et al., 1979). Follow-up studies, however, found that this effect may be due to artifacts in immunoreactivity assays caused by contrast media (Dionne et al., 1984; Fessler et al., 1984). As a result of these collective studies, involvement of endogenous opioid peptides in PAG-DBS-driven analgesia remains unresolved.
Compared to PAG-DBS, DBS in the sensory thalamus is thought to be more effective for deafferentation pain (Bittar et al., 2005), which is caused by damage to the peripheral or central nervous system that causes the loss of normal incoming pain signals. Examples of this type of pain include post-stroke pain, spinal cord injury, and facial anesthesia dolorosa (Hosobuchi et al., 1973; Adams et al., 1974). The theory behind the effectiveness of sensory thalamus DBS for this type of pain is that deafferentation pain is caused by a lack of normal proprioceptive information reaching the thalamus, which is combated by direct stimulation of VPL and VPM (Duncan et al., 1991). Additionally, stimulation may modulate the altered firing patterns in the sensory thalamus that are found in chronic pain patients (Dostrovsky, 2000; Moisset et al., 2020). When targeting sensory thalamus, stimulating electrodes are typically placed contralaterally and somatotopically according to the location of the painful area, and stimulation produces paresthesia in that area that masks pain (Hosobuchi et al., 1973; Boccard et al., 2015; Moisset et al., 2020). Comparatively, studies of sensory thalamic-DBS often use higher stimulus pulse frequencies than PAG-DBS, with frequencies falling between 50 and 100 Hz (Bittar et al., 2005; Moisset et al., 2020).
Deep brain stimulation (DBS) of medial thalamic centromedian-parafascicular nuclear complex (CM-Pf) has been attempted in humans under the assumption that this stimulation may activate descending pain modulatory opioidergic or non-opioidergic mechanisms, as well as drive a sensory feedforward loop with cortical targets (Andy, 1980; Duncan et al., 1991). While this manipulation appeared to be effective in a small cohort of patients with painful dyskinesia (Andy, 1980), other studies have produced variable results on reported painfulness and report a variety of potentially unpleasant side effects (Thoden et al., 1979; Hollingworth et al., 2017). Interestingly, a recent case study in 3 patients refractory to conventional neuromodulatory therapies found potential therapeutic benefits of dual stimulation of CM-Pf and PAG/PVG using a single electrode at different frequencies (Hollingworth et al., 2017).
The different electrical stimulation parameters of successful PAG-and thalamic-DBS strongly suggest that these two therapies exert their effects through distinct neural mechanisms. Early neurostimulation trials provide further evidence for this distinction. Specifically, responsiveness to morphine is used throughout the literature to select patients for PAG-or thalamic-DBS. Patients that respond moderately well to morphine are selected for PAG-DBS, while those that do not respond well to high doses of morphine are still able to find pain relief via thalamic-DBS whereas PAG-DBS would be ineffective (Hosobuchi, 1986). Along these lines, centrally generated pain is attenuated by thalamic-DBS, whereas PAG-DBS is not effective. These findings, coupled with the observation of low-threshold spontaneous discharge patterns in midline thalamic nuclei associated with pain states (Andy, 1983), lead to the hypothesis that thalamic-DBS produces a “functional lesion” by inducing depolarization block and inactivating low threshold discharging neurons surrounding the stimulation electrode. This “functional lesion” mechanism has also been proposed to account for the anti-dyskinetic effects of subthalamic nucleus-DBS applied for Parkinson’s disease, which shows pathological burst activity that correlates with onset of motor symptoms (Lobb, 2014). If an analogous mechanism of thalamic-DBS were confirmed, it presents the opportunity to trigger thalamic-DBS in response to nociceptive-related spontaneous discharge patterns of thalamic nuclei. Such closed-loop stimulation protocols have been increasingly adopted with STN-DBS for Parkinson’s disease and have the advantage of reduced off-target effects and extended battery life by requiring only intermittent stimulation.
In contrast to PAG and thalamus which have been targeted with electrical stimulation for pain relief for over 30 years, DBS of dorsal ACC (dACC) has only recently emerged as treatment for neuropathic pain. In an initial case report (Spooner et al., 2007), a single patient with neuropathic pain resulting from a spinal cord injury received bilateral dACC-DBS electrodes and a unilateral electrode in the PVG. In this patient, DBS applied to the dACC at 130 Hz provided superior pain relief, mood improvement, and reduction in medication usage compared to PVG-DBS applied at 20 Hz. This treatment resulted in reduced pain as assessed via visual analog scale (VAS) pain ratings and pain medication usage. This patient also showed improved mood in terms of reduction of fear, anxiety, and depression, suggesting that dACC stimulation works at least in part by targeting pain affect.
Anterior cingulate cortex (ACC) stimulation in rodents can produce diverse behavioral effects depending on stimulus pulse frequency and which neuronal subtypes are stimulated. Unilateral electrical stimulation of the rodent ACC with intermittent trains of 100 Hz pulses (200 ms inter-train interval) induced fear-like freezing responses (Tang et al., 2005). Optogenetically activating ACC Thy1 + neurons at 20 Hz induced anxiodepressive behaviors, but did not increase the hindpaw withdraw threshold to mechanical stimuli (Barthas et al., 2015). Optogenetic activation at 10 Hz of CaMKII + excitatory ACC neurons (which partially overlap with the Thy1 + population) increased paw withdrawal thresholds in naïve mice, while inhibition reversed inflammatory pain-induced behavior (Kang et al., 2015). Further, nociceptive responses have been demonstrated to be attenuated in rodents following optogenetic and chemogenetic activation of subsets of ACC interneurons (Gu et al., 2015; Kang et al., 2015; Shao et al., 2021). These findings suggest that heterogeneity in both function, topography, and cellular architecture contribute to the diverse behavioral responses produced by ACC stimulation.
Clinical applications of ACC-DBS are typically applied at stimulation frequencies of approximately 130 Hz and stimulus pulse widths around 450 μs (Boccard et al., 2014, 2017). The efficacy of ACC-DBS has been shown for patients suffering from failed back surgery syndrome, poststroke pain, brachial plexus injury, cervical spinal cord injury, head injury, and pain of unknown origin (Boccard et al., 2014). Interestingly, some patients receiving ACC-DBS do not report significant reductions in pain as measured by numerical rating scales. However, many ACC-DBS patients report improvements in metrics related to the affective component of pain as well as overall improvements in quality of life and describe their pain as being “separate from them” or “not distressing” (Boccard et al., 2017).
Due to its novelty, there are few published studies on ACC-DBS mechanisms of action. However, the ACC projects to many pain matrix structures, such as amygdala and PAG (Shi et al., 2022). Therefore, it is possible that the analgesic effects of ACC-DBS are due to postsynaptic DPMS engagement. MORs are present both on local ACC cells and afferents (particularly from the thalamus) terminating in the ACC (Vogt et al., 1995). Furthermore, it is understood that terminating afferents are highly excitable near DBS electrodes (Bower and McIntyre, 2020). This suggests that local opioid release could occur during ACC-DBS to either engage the DPMS or suppress thalamocortical relay of noxious sensory information. Preclinical and clinical data are needed to test these hypotheses.
For more superficial brain targets, some researchers and physicians have opted for intracortical or epidural stimulation. Using this method, a craniotomy is performed, and electrodes are placed on the surface of the brain in the epidural space. Intracortical stimulation (ICS) is used for patients with chronic neuropathic pain that cannot be treated by medication and does not respond to other forms of stimulation, such as post-stroke pain (Moisset et al., 2020). For chronic pain patients, ICS is mostly performed on the surface of the motor cortex in a procedure called intracortical motor cortex stimulation (iMCS). iMCS is typically applied at stimulus frequencies between 30 and 90 Hz and requires constant, continuous stimulation via an implanted device for patients to continue the therapy at all times (Fontaine et al., 2009; Lefaucheur et al., 2009). The stimulus pulse amplitude is set at 80% of the amplitude necessary to elicit a motor response, but is generally imperceptible to the patient (Moisset et al., 2020).
Primary motor cortex (M1) is not particularly rich in endogenous opioid peptides or receptors. Rat M1 exhibits radiolabeled ligand binding at MORs at intermediate levels in layers I and VI, but the level of MOR expression is much less than in nearby limbic cortical areas. Ligand binding to DORs is also very low (Lewis et al., 1983). Similarly, M1 dynorphin and enkephalin immunoreactivity reveals extremely sparse expression of these endogenous opioids (Fallon and Leslie, 1986). However, because M1 stimulation is thought to activate the DPMS, endogenous opioid signaling in downstream circuits could still be an important mechanism of action. In the rat, iMCS has been shown to effectively activate M1 layer V output neurons via transsynaptic mechanisms, underscoring a mechanism by which superficial electrodes can affect motor cortex output (Hussin et al., 2015). In rodents, iMCS activates PAG and decreases activity in the DH, as assessed by recordings of neuronal activity and immunohistochemistry for immediate early genes, such as cFOS (Pagano et al., 2012; França et al., 2013). Some of the strongest evidence implicating endogenous opioid signaling in M1 stimulation-driven analgesia arises from the finding in rats that the resulting analgesia is consistently blocked by systemic naloxone (Fonoff et al., 2009). Further, PAG naloxone pretreatment in rats blocked the inhibition of sensory evoked potentials in the somatosensory cortex induced by M1 stimulation (Chiou et al., 2013). These preclinical data suggest that release of endogenous opioids may be a key component of iMCS-induced analgesia.
Exactly how M1 stimulation activates the DPMS remains unclear. In rats, iMCS activates striatum, cerebellum and some thalamic areas, while responses to noxious stimuli in VPL, S1, and PFC are inhibited (Jiang et al., 2014; Kim et al., 2016). In humans, functional imaging and electrophysiological studies have revealed that iMCS rapidly activates lateral thalamus. Hours later, activation of medial thalamus, ACC, orbitofrontal cortex (OFC), and PAG is observed. The PAG receives input from ACC and OFC, and functional connectivity between ACC and PAG in particular is associated with pain suppression in the contexts of opioid analgesia, placebo analgesia, and attentional analgesia (García-Larrea et al., 1999; Peyron et al., 2007). It is plausible to hypothesize that the prefrontal pain modulatory network engages the PAG, yet it remains unclear precisely how M1 stimulation recruits the prefrontal cortex and how this unfolds on such a slow timescale. The precentral gyrus in the macaque, which contains M1, additionally sends projections to PAG, suggesting a possible direct route for DPMS activation via iMCS (von Monakow et al., 1979).
In a meta-analysis of 14 studies that used iMCS in 210 chronic pain patients, subjective classification of outcomes yielded a positive response to iMCS in ∼55% of patients, which dropped to 45% in patients that were able to be assessed more than 1 year later. For the patients that provided visual analog scale scores of pain, their pain ratings improved by 56% after receiving the intracortical stimulation. Importantly, however, in the two studies that had internal controls for stimulation by cycling through “on” and “off” stimulation periods, patients did not show significant differences in pain outcomes between the two (Fontaine et al., 2009), suggesting the possibility that at least some aspects of iMCS pain relief result from placebo effects. Alternatively, “wash-out” effects of stimulation or induction of plasticity may also contribute to persistently reduced pain outcomes during the “off” stimulation periods. Future experiments are required to parse the contribution of these factors.
As assessed by PET imaging using [11C]diprenorphine, iMCS leads to endogenous opioid release in patients with refractory neuropathic pain in anterior midcingulate cortex (aMCC), PAG, PFC, and cerebellum, with aMCC and PAG changes correlating with pain relief (Maarrawi et al., 2007). Additionally, high opioid receptor availability in insula, thalamus, PAG, ACC, and OFC were positively correlated with later MCS pain relief efficacy (Maarrawi et al., 2013). However, another study appears to challenge the evidence pointing to endogenous opioid recruitment of the DPMS by iMCS. Although M1 stimulation increased discharge rates in LC neurons in rats experiencing neuropathic pain, lidocaine block of LC or intrathecal alpha2-adrenergic antagonists did not attenuate M1 stimulation-induced antinociception in neuropathic pain or control rats (Viisanen and Pertovaara, 2010). Continued study is needed to elucidate the exact mechanisms of endogenous opioid release during iMCS, and how it may correlate with resultant analgesia.
Repetitive transcranial magnetic stimulation (rTMS) is a non-invasive neurostimulation method during which an electromagnetic coil is placed against the scalp in alignment with a target brain region. A current is passed through the coil to produce pulsatile changes in the magnetic field surrounding the coil. This magnetic field passes through the skull and into the brain, where it induces electrical currents which modulate the activity of neurons in target regions. rTMS is most commonly used to treat depression in patients who are unresponsive to or unable to tolerate medications (Speer et al., 2000). However, a systematic review of the literature concluded that rTMS is effective for central pain, peripheral nerve disorders, fibromyalgia, and migraine, and that studies using rTMS for orofacial pain, phantom limb pain, lower back pain, and complex regional pain syndrome were promising but inconclusive (Yang and Chang, 2020). Importantly, when targeted to the appropriate brain regions, the reported rTMS effects are pain-specific (Nahmias et al., 2009).
Repetitive transcranial magnetic stimulation (rTMS) treatment paradigms are widely used in the clinic and are therefore highly standardized. Typically, a patient receives rTMS for several min per session, undergoing 10s of sessions over several months. rTMS frequencies for pain treatment range between ∼0.5 and 10 Hz, with the consensus being that frequencies greater than 5 Hz are most effective (Lefaucheur et al., 2006; Moisset et al., 2015). rTMS has been extensively studied at two sites: the dlPFC, based on its accessibility and role in pain processing, and primary motor cortex (M1). M1 rTMS has been consistently reported to provide pain relief in both chronic pain patients and experimental models of pain (Lefaucheur et al., 2006; Nahmias et al., 2009; de Andrade et al., 2011; Moisset et al., 2015). Although there is some disagreement in the literature (Yoo et al., 2006), there is a general consensus that dlPFC rTMS also provides pain relief in models of experimental pain in healthy subjects (Graff-Guerrero et al., 2005; Borckardt et al., 2007; Nahmias et al., 2009; Valmunen et al., 2009; de Andrade et al., 2011; Taylor et al., 2012). While rTMS is performed contralateral to the painful site, bilateral analgesia can be evoked in humans (Nahmias et al., 2009). M1 rTMS produces bilateral analgesia in healthy patients that does not affect thermal detection thresholds, which points toward a role for diffuse descending pain modulation (Nahmias et al., 2009). rTMS provides both short-term pain relief immediately after the stimulation session, which may take 2–3 days to reach its peak, as well as long term relief that lasts for weeks to months after the end of session in contrast with the previously introduced stimulation techniques (Lefaucheur et al., 2001, 2006). Interestingly, the impact on pain affect lasts longer than on the sensory component of pain (Passard et al., 2007).
In humans, evidence for the involvement of endogenous opioids in M1 rTMS-induced analgesia has emerged from studies in healthy subjects in which naloxone blocked the rTMS-induced short-term analgesia. However, dlPFC studies by different groups reached different conclusions. A landmark study found that naloxone attenuated the analgesic effect of M1 stimulation but not dlPFC or sham rTMS (de Andrade et al., 2011), whereas another study found that naloxone blocked the analgesic effect of dlPFC rTMS (Taylor et al., 2012). A PET study using the radioligand [11C]carfentanil administered several hours after rTMS treatment of a diffuse area containing M1 and primary somatosensory cortex in healthy subjects revealed endogenous opioid release in the ipsilateral ventral striatum, mOFC, PFC, ACC, contralateral insula, superior temporal gyrus, dlPFC, and precentral gyrus, without impacting striatal D2 receptor availability (Lamusuo et al., 2017).
Transcranial direct current stimulation (tDCS) applies low levels of electrical current via small battery powered electrodes placed on the head. Although it is not currently approved by the Federal Drug Administration in the United States as its regulatory status is only “investigational,” studies on small cohorts have shown promising results for the use of tDCS in patients with fibromyalgia, spinal cord injury, and migraine (Fregni et al., 2006a,b; Dasilva et al., 2012). In other studies, however, tDCS was not effective for chronic low back pain or in spinal cord injury (O’Connell et al., 2013; Wrigley et al., 2013). Similar to iMCS and rTMS, tDCS appears most effective when applied over the motor cortex. Interestingly, PET imaging for radiolabeled opioids revealed motor cortex tDCS-driven endogenous opioid release, which reveals a possible mechanism for the measured improvements in thermal pain thresholds (DosSantos et al., 2014). Although both tDCS and placebo stimulation caused endogenous opioid release in PAG and precuneus, tDCS alone produced analgesia and additional opioid release in left PFC. Though naloxone was not administered to determine the causality of opioid signaling in the observed analgesia, these studies suggest opioidergic signaling is responsible at least in part for the tDCS-induced pain relief.
In recent years, there have been several innovations regarding the electrical stimulus waveforms applied by neurostimulation therapies for chronic pain. With SCS, many of these innovations apply tonic SCS at frequencies not typically utilized by conventional (i.e., 40 to 60 Hz) SCS. Kilohertz frequency SCS (KHFSCS), ultra-low frequency SCS (ULFSCS), and burst SCS all provide pain relief without producing paresthesias. KHFSCS utilizes frequencies greater than 1,000 Hz (Kapural et al., 2015), while ULFSCS applies frequencies below 0.1 Hz (Jones et al., 2021). Burst SCS employs bursts of SCS pulses at ∼40 Hz with an intraburst frequency of 500 Hz (de Ridder et al., 2013). Similar to conventional SCS, the physiological mechanisms of analgesia for each of these novel forms of SCS are unknown, presenting the same challenges to improving their design and implementation. However, these paresthesia-free SCS waveforms allow for placebo-controlled clinical studies, providing exciting new opportunities to systematically examine the effects of these new therapies in the patient’s pain experience.
In addition to new tonic SCS waveforms, new stimulus paradigms are emerging in clinical neuromodulation. Differential targeted multiplexed SCS (DTMSCS) applies two simultaneous SCS waveforms: a lower frequency 50 Hz waveform, and a higher frequency 1,200 Hz waveform (Vallejo et al., 2020). It is hypothesized that in addition to inducing conventional segmental pain inhibition, DTMSCS also affects properties of spinal glial cells (Vallejo et al., 2020). A recent innovation in DBS, Coordinated Reset DBS (CRDBS), applies precisely timed, spatially distributed stimuli to desynchronize pathological brain activity, possibly by rectifying aberrant synapses which were remodeled by disease conditions (Tass, 2003). Interestingly, CRDBS may produce long-lasting therapeutic benefit, even after the stimulus pulse is switched off (Wang J. et al., 2016). These stimulus paradigms suggest that it is critical to consider the effects of neuromodulation therapies on pre-and post-synaptic terminals and on non-neuronal cells, and that improving our scientific understanding of how the timing of exogenous electrical stimuli is integrated by neurons and synapses may allow for the evidence-based design of novel stimulus protocols which directly target the synaptic basis of pathological neural activity.
A major challenge in diagnosis and treatment of chronic pain conditions is that there are no objective biomarkers of the pain experience. Most existing neurostimulation therapies apply stimulation in an “open-loop” fashion, where electrical stimuli are delivered at a constant frequency with no variation in intensity or rate. Given temporal fluctuations in severity of pain symptoms in chronic pain patients, modulating stimulation in response to changes in neural activity or behavioral biomarkers would represent an important treatment advance and may prevent tolerance by delivering stimulation only when needed and limiting unwanted side effects. Closed-loop approaches are beginning to be adopted in neurostimulation for pain, such as monitoring the amplitude of evoked compound action potentials recorded from the dorsal columns to modulate SCS pulse amplitudes. This approach was recently demonstrated to provide superior pain relief compared to open-loop SCS (Mekhail et al., 2020). Improving our understanding of how chronic pain pathogenesis and neurostimulation therapies affect the characteristics and behavior of opioidergic (and other) circuits could reveal new biomarkers with which to design closed-loop stimulation algorithms.
Continued study of the complicated matrix of brain areas involved in pain processing has revealed other targets that may provide therapeutic benefit by neurostimulation, including the insular cortex (IC). The IC can be divided along the anterior-posterior axis, with the posterior insula (pI) participating in somatosensory features of pain, whereas the anterior portion (aI) is implicated in encoding pain unpleasantness (Craig, 2002).
Low frequency electrical stimulation of the right pI elicits nociception in humans and primates with some somatotopy (Ostrowsky et al., 2002; Mazzola et al., 2009), while high frequency stimulation of pI and aI reduces pain thresholds with no obvious side effects, consistent with insular inactivation (Denis et al., 2016; Liu et al., 2021). A form of rTMS in IC has been shown to produce bilateral thermal analgesia in humans without affecting the ability to perceive innocuous thermal or vibrotactile sensations (Lenoir et al., 2018). Similarly, pI-rTMS increases thermal pain thresholds in patients with central neuropathic pain, but this did not translate to differences in relief from chronic pain and quality of life (Galhardoni et al., 2019). Although studies have not yet extended ICS to the human insula, one preclinical study in rodents suggests a potential role for low frequency intracortical pI stimulation in relief from chronic neuropathic pain. Importantly for this review, all forms of analgesia examined in this study were blocked by naloxone, clearly implicating endogenous opioid release (Komboz et al., 2022). Although opioid peptides and receptors are prominent in pI, it remains to be determined whether local opioid signaling, activation of afferents from other structures, or projections to the DPMS are involved. Innovation in the brain areas targeted by neurostimulation techniques may elucidate stimulation paradigms that provide pain relief in the absence of adverse side effects.
A key challenge with electrical stimulation of any neural structure is the cellular heterogeneity of the target. Electrical stimulation is inherently non-specific; all neurons in the vicinity of the electrode are subject to modulation, which presents a challenge when the target structure is comprised of diverse neuronal subtypes which may play distinct or even opposing functional roles in neural circuits. In some cases, it may be advantageous to preferentially modulate specific subpopulations of neurons within a target structure. For example, the PAG can be subdivided into populations of glutamatergic and GABAergic neurons with subpopulations of each type projecting to the RVM to drive descending pain modulation. We hypothesize that MOR-expressing PAG-RVM projection neurons may facilitate pain, since they are inhibited by opioid analgesics. Thus, selective recruitment of the MOR-lacking PAG projection neurons using electrical stimulation may produce the most effective pain relief.
We recently demonstrated that pharmacological adjuvants can be combined with DBS to enhance its specificity (Creed et al., 2015; Creed, 2017). Pharmacological adjuvants have also been applied in preclinical (Cui et al., 1996) and clinical (Lind et al., 2004, 2007) studies of SCS, suggesting that co-application of SCS and the GABAB receptor agonist baclofen may increase analgesia compared to the application of a single therapy alone. Currently, the combined approach of simultaneous electrical and chemical neuromodulation is not widely adopted in the clinical neuromodulation field. However, characterizing differences in ion channel or receptor expression between functional subpopulations of a target structure could identify pharmacological targets to be chemically manipulated during concurrent electrical stimulation. A dual electrical and chemical modulatory approach may allow for greater symptom control in cases where symptoms of a given disease are governed by biochemically distinct neuronal subpopulations. This approach may improve the specificity of a therapy, and thus increase efficacy while limiting off-target effects.
The advent of non-invasive, region-specific drug delivery and devices capable of delivering simultaneous electrical and chemical stimulation (Capogrosso et al., 2018) makes this an even more exciting and tractable possibility. Recently, focused ultrasound has been used to target drug release to specific sites in the brain in a non-invasive manner (Airan and Butts Pauly, 2018; Wang et al., 2018; McMahon et al., 2021). We anticipate that light-driven activation of drugs and neurotransmitters (i.e., photopharmacology) will also emerge as a viable approach that offers improved spatial and temporal precision for in vivo drug delivery (Banghart and Sabatini, 2012; Font et al., 2017; Hüll et al., 2018; López-Cano et al., 2021). Photopharmacology may interface particularly well with DBS and iCS, as light sources can be readily incorporated into stimulating electrodes (Royer et al., 2010; Lechasseur et al., 2011).
Neurostimulation therapies are usually reserved for patients who are treatment refractory to every other standard of care in chronic pain conditions and for other neurological and psychiatric disorders. However, chronic pain, like other neurological and psychiatric disorders, is a disease of neural plasticity, with reorganization of neural pathways involved in pain and affective processing contributing to the persistence of pain symptoms. Recently, it has been proposed that patients receiving stocktickerSCS to manage their chronic pain would benefit from implementing the therapy earlier in disease pathogenesis (Kumar et al., 2014; Taylor et al., 2014; Lad et al., 2016; Campos et al., 2019). Along the same lines, novel DBS protocols have been shown to effectively reverse maladaptive plasticity associated with behavioral symptoms in Parkinson’s disease (Wang J. et al., 2016; Mastro et al., 2017; Spix et al., 2021) and addiction (Creed et al., 2015; Lüscher et al., 2015). Because these protocols alter plasticity in neural circuits, their therapeutic effects outlast the duration of stimulation, which is in stark contrast to classically applied tonic ∼100 Hz DBS in which motor or psychiatric symptoms reappear nearly immediately after DBS offset (Lüscher et al., 2015). An intriguing prospect would be to apply DBS in patients with pain disorders before nociceptive and affective circuitry undergo pain-induced plasticity that contributes to affective comorbidities or cognitive symptoms of chronic pain (Andrade et al., 2013). Alternatively, designing DBS protocols capable of normalizing chronic pain-induced synaptic adaptations in nociceptive processing pathways would hold enormous therapeutic promise.
Accurate assessment of treatment efficacy is crucial for any therapy. The success of neurostimulation therapies for chronic pain is typically defined as a ≥ 50% reduction in a patient’s overall pain, measured by the visual analog scale (VAS), verbal rating scale (VRS), or numeric rating scale (NRS). However, subjective measurements made with different scales are not always comparable (Ohnhaus and Adler, 1975; Lund et al., 2005) and may suffer from low reproducibility (van Tubergen et al., 2002). Furthermore, some have shown that the percentage of a patient cohort satisfied with SCS is disproportionately greater than the percentage of the cohort which met the ≥ 50% reduction in VAS (Sears et al., 2011). Taken together, these findings suggest that novel, holistic assessments of a patient’s pain experience may more accurately capture the efficacy of a neurostimulation therapy than a single pain rating alone. Some have suggested that dynamic pain measures, such as temporal summation and conditioned pain modulation, which are proxy measures for central sensitization and descending inhibitory tone respectively, may hold clinical value in both patient selection and assessing the efficacy of SCS (Yarnitsky et al., 2010; Campbell et al., 2015; Sankarasubramanian et al., 2019, 2021). Others have demonstrated that composite metrics which incorporate measurements of pain intensity, physical functioning, quality of life, and affect more closely represent the patient’s impression of therapeutic benefit (Pilitsis et al., 2021). These measures could provide a more accurate and reliable readout of a patient’s experience with a therapy for use during stimulator programming and as primary endpoints in clinical trials of neurostimulation therapies.
A key limitation facing all neurostimulation therapies is that we do not understand their therapeutic mechanisms of action. Uncovering these mechanisms may allow for the evidence-based design of targeted therapies which produce robust therapeutic benefit with minimal side effects. The study of neurostimulation therapies highlights several key knowledge gaps pertaining to understanding the neural substrates of symptom management in neurological disorders.
The rationale for selecting an implant location for a neurostimulation therapy, such as ACC-DBS, is often based on historical lesioning studies (Boccard et al., 2017). However, the selection of stimulus pulse parameters is largely initially arbitrary and empirically adjusted based on subjective patient feedback. The stimulus pulse frequencies which produce therapeutic benefit in the many therapies discussed in this review are quite variable, with some therapies using pulse frequencies greater than 100 Hz, while others use pulse frequencies closer to 20 Hz. A notable finding of the involvement of endogenous opioids in preclinical SCS studies is that opioidergic analgesia during SCS may be dependent on stimulus pulse frequency. SCS applied at 60 Hz required higher doses of naloxone to abolish SCS-induced analgesia and was sensitive to a DOR antagonist, while SCS applied at 4 Hz required lower doses of naloxone to abolish analgesia and was not sensitive to a DOR antagonist (Sato et al., 2013). These data imply that the stimulus pulse frequency, putatively the rate at which axons near the stimulating electrode are conducting artificially generated APs (McIntyre et al., 2004), may affect the characteristics of neurotransmitter release from the presynaptic terminals of stimulated neurons. Future studies should examine how varying stimulus pulse frequency affects neurotransmitter release and pre-and post-synaptic receptor activation.
Many studies of neurostimulation therapies focus on the effects of stimulation on the neurons which are directly responding to the stimulus pulse. However, the resulting effects on postsynaptic networks are likely complex and intricately involved in symptom relief. Novel experimental techniques to study the activity of large networks such as in vivo calcium imaging (Göbel and Helmchen, 2007) and high-density electrical recordings (Jun et al., 2017; Juavinett et al., 2019; Steinmetz et al., 2021) provide the opportunity to monitor the behavior and properties of neural networks over time. These techniques could be used to observe the network response to neurostimulation therapies (Trevathan et al., 2021). Crucially, these methods also allow for the characterization of network properties across different behavioral states (Sweeney et al., 2021). Comparing network properties during both pain pathogenesis and intervention could give key insights into the development of neurological disease and reveal novel methods for targeted intervention.
Neurostimulation therapies are important tools in managing intractable chronic pain. Our incomplete understanding of the mechanisms of action of such therapies precludes their improvement to maximize pain relief. In this review, we summarized the evidence that many neurostimulation therapies for pain may provide analgesia in part by modulating opioidergic circuits throughout the neuraxis. Further study is needed to understand the mechanisms by, and extent to which, neurostimulation therapies modulate these circuits. Continued study of the interactions between exogenous electric fields and neuronal and synaptic dynamics will be critical to the evidence-based design of neurostimulation therapies which specifically target mechanisms underlying neurological disease. We believe that a multidisciplinary approach combining basic neurobiological studies, innovation in clinical paradigms, and novel technology development will be key to engineering the next generation of safe and effective therapies for chronic pain.
SL, RG, GL, RS, MB, and MC wrote and edited the manuscript. All authors listed have made a substantial, direct, and intellectual contribution to the work, and approved it for publication.
This work was supported by the National Institute of Neurological Disease and Stroke (U01NS113295 to MB), the National Institute for General Medical Sciences (R35GM133802 to MB), the National Institute for Drug Abuse (R01DA49924 and R21DA047127 to MC and T32DA007261 to RG), the Rita Allen Foundation (MB and MC), and the Hetzler Stiftung Foundation (MC).
We thank Emily Hromi of Hromi Biomedical for illustrations.
The authors declare that the research was conducted in the absence of any commercial or financial relationships that could be construed as a potential conflict of interest.
All claims expressed in this article are solely those of the authors and do not necessarily represent those of their affiliated organizations, or those of the publisher, the editors and the reviewers. Any product that may be evaluated in this article, or claim that may be made by its manufacturer, is not guaranteed or endorsed by the publisher.
Ab Aziz, C. B., and Ahmad, A. H. (2006). The role of the thalamus in modulating pain. Malays. J. Med. Sci. 13, 11–18.
Adams, J. E. (1976). Naloxone reversal of analgesia produced by brain stimulation in the human. Pain 2, 161–166. doi: 10.1016/0304-3959(76)90111-1
Adams, J. E., Hosobuchi, Y., and Fields, H. L. (1974). Stimulation of internal capsule for relief of chronic pain. J. Neurosurg. 41, 740–744.
Aimone, L. D., and Gebhart, G. F. (1986). Stimulation-produced spinal inhibition from the midbrain in the rat is mediated by an excitatory amino acid neurotransmitter in the medial medulla. J. Neurosci. 6, 1803–1813. doi: 10.1523/JNEUROSCI.06-06-01803.1986
Aimone, L. D., Jones, S. L., and Gebhart, G. F. (1987). Stimulation-produced descending inhibition from the periaqueductal Gray and nucleus raphe Magnus in the rat: Mediation by spinal monoamines but not opioids. Pain 31, 123–136. doi: 10.1016/0304-3959(87)90012-1
Airan, R. D., and Butts Pauly, K. (2018). Hearing out ultrasound neuromodulation. Neuron 98, 875–877. doi: 10.1016/J.NEURON.2018.05.031
Akil, H., Mayer, D. J., and Liebeskind, J. C. (1976). Antagonism of stimulation-produced analgesia by naloxone, a narcotic antagonist. Science 191, 961–962. doi: 10.1126/science.1251210
Akil, H., Richardson, D. E., Hughes, J., and Barchas, J. D. (1978). Enkephalin-like material elevated in ventricular cerebrospinal fluid of pain patients after analgetic focal stimulation. Science 201, 463–465. doi: 10.1126/science.663668
Alitto, H. J., and Usrey, W. M. (2003). Corticothalamic feedback and sensory processing. Curr. Opin. Neurobiol. 13, 440–445. doi: 10.1016/S0959-4388(03)00096-5
Allsop, S. A., Wichmann, R., Mills, F., Burgos-Robles, A., Chang, C. J., Felix-Ortiz, A. C., et al. (2018). Corticoamygdala transfer of socially derived information gates observational learning. Cell 173, 1329–1342.e18. doi: 10.1016/j.cell.2018.04.004
Andrade, D. C., Borges, I., Bravo, G. L., Bolognini, N., and Fregni, F. (2013). Therapeutic time window of noninvasive brain stimulation for pain treatment: Inhibition of maladaptive plasticity with early intervention. Exp. Rev. Med. Devices 10, 339–352. doi: 10.1586/ERD.12.90
Andy, O. J. (1980). Parafascicular-center median nuclei stimulation for intractable pain and dyskinesia (painful-dyskinesia). Appl. Neurophysiol. 43, 133–144. doi: 10.1159/000102247
Andy, O. J. (1983). Thalamic stimulation for chronic pain. Appl. Neurophysiol. 46, 116–123. doi: 10.1159/000101250
Apkarian, A. V., Bushnell, M. C., Treede, R. D., and Zubieta, J. K. (2005). Human brain mechanisms of pain perception and regulation in health and disease. Eur. J. Pain 9:463. doi: 10.1016/J.EJPAIN.2004.11.001
Arvidsson, U., Dado, R. J., Riedl, M., Lee, J. H., Law, P. Y., Loh, H. H., et al. (1995). δ-Opioid receptor immunoreactivity: Distribution in brainstem and spinal cord, and relationship to biogenic amines and enkephalin. J. Neurosci. 15, 1215–1235. doi: 10.1523/jneurosci.15-02-01215.1995
Azami, J., Llewelyn, M. B., and Roberts, M. H. T. (1982). The contribution of nucleus reticularis paragigantocellularis and nucleus raphe Magnus to the analgesia produced by systemically administered morphine, investigated with the microinjection technique. Pain 12, 229–246. doi: 10.1016/0304-3959(82)90155-5
Bagley, E. E., and Ingram, S. L. (2020). Endogenous opioid peptides in the descending pain modulatory circuit. Neuropharmacology 173:108131. doi: 10.1016/j.neuropharm.2020.108131
Bajic, D., and Proudfit, H. K. (1999). Projections of neurons in the periaqueductal gray to pontine and medullary catecholamine cell groups involved in the modulation of nociception. J. Comp. Neurol. 405, 359–379. doi: 10.1002/(SICI)1096-9861(19990315)405:3<359::AID-CNE6<3.0.CO;2-W
Ballantine, H. T., Cassidy, W. L., Flanagan, N. B., and Marino, R. (1967). Stereotaxic anterior cingulotomy for neuropsychiatric illness and intractable pain. J. Neurosurg. 26, 488–495. doi: 10.3171/JNS.1967.26.5.0488
Banghart, M. R., and Sabatini, B. L. (2012). Photoactivatable neuropeptides for spatiotemporally precise delivery of opioids in neural tissue. Neuron 73, 249–259. doi: 10.1016/j.neuron.2011.11.016
Barthas, F., Sellmeijer, J., Hugel, S., Waltisperger, E., Barrot, M., and Yalcin, I. (2015). The anterior cingulate cortex is a critical hub for pain-induced depression. Biol. Psychiatry 77, 236–245. doi: 10.1016/J.BIOPSYCH.2014.08.004
Behbehani, M. M. (1995). Functional characteristics of the midbrain periaqueductal gray. Prog. Neurobiol. 46, 575–605. doi: 10.1016/0301-0082(95)00009-K
Bengoetxea, X., Goedecke, L., Blaesse, P., Pape, H. C., and Jüngling, K. (2020). The μ-opioid system in midline thalamic nuclei modulates defence strategies towards a conditioned fear stimulus in male mice. J. Psychopharmacol. 34, 1280–1288. doi: 10.1177/0269881120940919
Besse, D., Lombard, M. C., Zajac, J. M., Roques, B. P., and Besson, J. M. (1990). Pre- and postsynaptic distribution of μ, δ and κ opioid receptors in the superficial layers of the cervical dorsal horn of the rat spinal cord. Brain Res. 521, 15–22. doi: 10.1016/0006-8993(90)91519-M
Bingel, U., Lorenz, J., Schoell, E., Weiller, C., and Büchel, C. (2006). Mechanisms of placebo analgesia: rACC recruitment of a subcortical antinociceptive network. Pain 120, 8–15. doi: 10.1016/j.pain.2005.08.027
Birdsong, W. T., Jongbloets, B. C., Engeln, K. A., Wang, D., Scherrer, G., and Mao, T. (2019). Synapse-specific opioid modulation of thalamo-cortico-striatal circuits. Elife 8:e45146. doi: 10.7554/eLife.45146
Bittar, R. G., Kar-Purkayastha, I., Owen, S. L., Bear, R. E., Green, A., Wang, S., et al. (2005). Deep brain stimulation for pain relief: A meta-analysis. J. Clin. Neurosci. 12, 515–519. doi: 10.1016/j.jocn.2004.10.005
Bliss, T. V. P., Collingridge, G. L., Kaang, B. K., and Zhuo, M. (2016). Synaptic plasticity in the anterior cingulate cortex in acute and chronic pain. Nat. Rev. Neurosci. 17, 485–496. doi: 10.1038/nrn.2016.68
Boccard, S. G. J., Fitzgerald, J. J., Pereira, E. A. C., Moir, L., van Hartevelt, T. J., Kringelbach, M. L., et al. (2014). Targeting the affective component of chronic pain: A case series of deep brain stimulation of the anterior cingulate cortex. Neurosurgery 74, 628–635. doi: 10.1227/NEU.0000000000000321
Boccard, S. G. J., Pereira, E. A. C., and Aziz, T. Z. (2015). Deep brain stimulation for chronic pain. J. Clin. Neurosci. 22, 1537–1543. doi: 10.1016/J.JOCN.2015.04.005
Boccard, S. G. J., Pereira, E. A. C., Moir, L., Aziz, T. Z., and Green, A. L. (2013). Long-term outcomes of deep brain stimulation for neuropathic pain. Neurosurgery 72, 221–230. doi: 10.1227/NEU.0B013E31827B97D6
Boccard, S. G. J., Prangnell, S. J., Pycroft, L., Cheeran, B., Moir, L., Pereira, E. A. C., et al. (2017). Long-term results of deep brain stimulation of the anterior cingulate cortex for neuropathic pain. World Neurosurg. 106, 625–637. doi: 10.1016/j.wneu.2017.06.173
Bodnar, R. J., Williams, C. L., Lee, S. J., and Pasternak, G. W. (1988). Role of μ1-opiate receptors in supraspinal opiate analgesia: A microinjection study. Brain Res. 447, 25–34. doi: 10.1016/0006-8993(88)90962-6
Borckardt, J. J., Smith, A. R., Reeves, S. T., Weinstein, M., Kozel, F. A., Nahas, Z., et al. (2007). Fifteen minutes of left prefrontal repetitive transcranial magnetic stimulation acutely increases thermal pain thresholds in healthy adults. Pain Res. Manag. 12, 287–290. doi: 10.1155/2007/741897
Bower, K. L., and McIntyre, C. C. (2020). Deep brain stimulation of terminating axons. Brain Stimul. 13, 1863–1870. doi: 10.1016/j.brs.2020.09.001
Braz, J., Solorzano, C., Wang, X., and Basbaum, A. I. (2014). Transmitting pain and itch messages: A contemporary view of the spinal cord circuits that generate gate control. Neuron 82, 522–536. doi: 10.1016/J.NEURON.2014.01.018
Bruinstroop, E., Cano, G., Vanderhorst, V. G. J. M., Cavalcante, J. C., Wirth, J., Sena-Esteves, M., et al. (2012). Spinal projections of the A5, A6 (locus coeruleus), and A7 noradrenergic cell groups in rats. J. Comp. Neurol. 520, 1985–2001. doi: 10.1002/cne.23024
Bush, G., Luu, P., Posner, M. I., and Posner, M. (2000). Cognitive and emotional influences in anterior cingulate cortex. Trends Cogn. Sci. 4, 215–222.
Bushnell, M. C., Čeko, M., and Low, L. A. (2013). Cognitive and emotional control of pain and its disruption in chronic pain. Nat. Rev. Neurosci. 14, 502–511. doi: 10.1038/nrn3516
Cameron, A. A., Khan, I. A., Westlund, K. N., and Willis, W. D. (1995b). The efferent projections of the periaqueductal gray in the rat: A Phaseolus vulgaris-leucoagglutinin study. II. Descending projections. J. Comp. Neurol. 351, 585–601.
Cameron, A. A., Khan, I. A., Westlund, K. N., Cliffer, K. D., and Willis, W. D. (1995a). The efferent projections of the periaqueductal gray in the rat: A Phaseolus vulgaris-leucoagglutinin study. I. Ascending projections. J. Comp. Neurol. 351, 568–584.
Campbell, C. M., Buenaver, L. F., Raja, S. N., Kiley, K. B., Swedberg, L. J., Wacnik, P. W., et al. (2015). Dynamic pain phenotypes are associated with spinal cord stimulation-induced reduction in pain: A repeated measures observational pilot study. Pain Med. 16, 1349–1360. doi: 10.1111/pme.12732
Campos, W. K., Linhares, M. N., Sarda, J., Santos, A. R. S., Licinio, J., Quevedo, J., et al. (2019). Determinants for meaningful clinical improvement of pain and health-related quality of life after spinal cord stimulation for chronic intractable pain. Neuromodulation 22, 280–289. doi: 10.1111/ner.12891
Capogrosso, M., Gandar, J., Greiner, N., Moraud, E. M., Wenger, N., Shkorbatova, P., et al. (2018). Advantages of soft subdural implants for the delivery of electrochemical neuromodulation therapies to the spinal cord. J. Neural. Eng. 15:026024. doi: 10.1088/1741-2552/aaa87a
Carlén, M. (2017). What constitutes the prefrontal cortex? Science 358, 478–482. doi: 10.1126/SCIENCE.AAN8868
Carr, K. D., and Bak, T. H. (1988). Medial thalamic injection of opioid agonists: μ-agonist increases while κ-agonist decreases stimulus thresholds for pain and reward. Brain Res. 441, 173–184. doi: 10.1016/0006-8993(88)91396-0
Casey, K. L., Lorenz, J., and Minoshima, S. (2003). Insights into the pathophysiology of neuropathic pain through functional brain imaging. Exp. Neurol. 184, 80–88. doi: 10.1016/j.expneurol.2003.07.006
Chen, Q. L., Roeder, Z., Li, M. H., Zhang, Y. M., Ingram, S. L., and Heinricher, M. M. (2017). Optogenetic evidence for a direct circuit linking nociceptive transmission through the parabrachial complex with pain-modulating neurons of the rostral ventromedial medulla (RVM. eNeuro 4, 202–219. doi: 10.1523/ENEURO.0202-17.2017
Cheriyan, J., and Sheets, P. L. (2018). Altered excitability and local connectivity of mPFC-PAG neurons in a mouse model of neuropathic pain. J. Neurosci. 38, 4829–4839. doi: 10.1523/JNEUROSCI.2731-17.2018
Chiou, R. J., Chang, C. W., and Kuo, C. C. (2013). Involvement of the periaqueductal gray in the effect of motor cortexstimulation. Brain Res. 1500, 28–35. doi: 10.1016/j.brainres.2013.01.022
Clark, F. M., and Proudfit, H. K. (1991a). Projections of neurons in the ventromedial medulla to pontine catecholamine cell groups involved in the modulation of nociception. Brain Res. 540, 105–115. doi: 10.1016/0006-8993(91)90496-I
Clark, F. M., and Proudfit, H. K. (1991b). The projection of locus coeruleus neurons to the spinal cord in the rat determined by anterograde tracing combined with immunocytochemistry. Brain Res. 538, 231–245. doi: 10.1016/0006-8993(91)90435-X
Clark, F. M., and Proudfit, H. K. (1991c). The projection of noradrenergic neurons in the A7 catecholamine cell group to the spinal cord in the rat demonstrated by anterograde tracing combined with immunocytochemistry. Brain Res. 547, 279–288. doi: 10.1016/0006-8993(91)90972-X
Clark, F. M., and Proudfit, H. K. (1993). The projections of noradrenergic neurons in the A5 catecholamine cell group to the spinal cord in the rat: Anatomical evidence that A5 neurons modulate nociception. Brain Res. 616, 200–210. doi: 10.1016/0006-8993(93)90210-E
Coffey, R. J. (2001). Deep brain stimulation for chronic pain: Results of two multicenter trials and a structured review. Pain Med. 2, 183–192. doi: 10.1046/j.1526-4637.2001.01029.x
Collins, F. S., Koroshetz, W. J., and Volkow, N. D. (2018). Helping to end addiction over the long-term the research plan for the NIH HEAL initiative. JAMA 320, 129–130. doi: 10.1001/jama.2018.8826
Corder, G., Castro, D. C., Bruchas, M. R., and Scherrer, G. (2018). Endogenous and exogenous opioids in pain. Annu. Rev. Neurosci. 41, 453–473. doi: 10.1146/annurev-neuro-080317-061522
Craig, A. D. (2002). How do you feel? Interoception: The sense of the physiological condition of the body. Nat. Rev. 3, 655–666. doi: 10.1038/nrn894
Creed, M. (2018). Current and emerging neuromodulation therapies for addiction: Insight from pre-clinical studies. Curr. Opin. Neurobiol. 49, 168–174. doi: 10.1016/j.conb.2018.02.015
Creed, M. C. (2017). Toward a targeted treatment for addiction. Science 357, 464–465. doi: 10.1126/SCIENCE.AAO1197
Creed, M., Pascoli, V. J., and Lüscher, C. (2015). Addiction therapy. Refining deep brain stimulation to emulate optogenetic treatment of synaptic pathology. Science 347, 659–664. doi: 10.1126/SCIENCE.1260776
Cui, J. G., Linderoth, B., and Meyerson, B. A. (1996). Effects of spinal cord stimulation on touch-evoked allodynia involve GABAergic mechanisms. An experimental study in the mononeuropathic rat. Pain 66, 287–295. doi: 10.1016/0304-3959(96)03069-2
D’Mello, R., and Dickenson, A. H. (2008). Spinal cord mechanisms of pain. Br. J. Anaesth. 101, 8–16. doi: 10.1093/bja/aen088
Dale, J., Zhou, H., Zhang, Q., Martinez, E., Hu, S., Liu, K., et al. (2018). Scaling up cortical control inhibits pain. Cell Rep. 23, 1301–1313. doi: 10.1016/j.celrep.2018.03.139
Dasilva, A. F., Mendonca, M. E., Zaghi, S., Lopes, M., Dossantos, M. F., Spierings, E. L., et al. (2012). tDCS-induced analgesia and electrical fields in pain-related neural networks in chronic migraine. Headache 52, 1283–1295. doi: 10.1111/J.1526-4610.2012.02141.X
de Andrade, D. C., Mhalla, A., Adam, F., Texeira, M. J., and Bouhassira, D. (2011). Neuropharmacological basis of rTMS-induced analgesia: The role of endogenous opioids. Pain 152, 320–326. doi: 10.1016/J.PAIN.2010.10.032
de Ridder, D., Plazier, M., Kamerling, N., Menovsky, T., and Vanneste, S. (2013). Burst spinal cord stimulation for limb and back pain. World Neurosurg. 80, 642–649.e1. doi: 10.1016/J.WNEU.2013.01.040
Deandrea, S., Montanari, M., Moja, L., and Apolone, G. (2008). Prevalence of undertreatment in cancer pain. A review of published literature. Ann. Oncol. 19, 1985–1991. doi: 10.1093/annonc/mdn419
Deer, T. R., Levy, R. M., Kramer, J., Poree, L., Amirdelfan, K., Grigsby, E., et al. (2017). Dorsal root ganglion stimulation yielded higher treatment success rate for complex regional pain syndrome and causalgia at 3 and 12 months: A randomized comparative trial. Pain 158, 669–681. doi: 10.1097/j.pain.0000000000000814
Dejongste, M. J., Hautvast, R. W., Ruiters, M. H., and ter Horst, G. J. (1998). Spinal cord stimulation and the induction of c-fos and heat shock protein 72 in the central nervous system of rats. Neuromodulation 1, 73–84. doi: 10.1111/J.1525-1403.1998.TB00020.X
Denis, D. J., Marouf, R., Rainville, P., Bouthillier, A., and Nguyen, D. K. (2016). Effects of insular stimulation on thermal nociception. Eur. J. Pain 20, 800–810. doi: 10.1002/ejp.806
Devinsky, O., Morrell, M. J., and Vogt, B. A. (1995). Contributions of anterior cingulate cortex to behaviour. Brain 118, 279–306.
Dickenson, A. H. (1995). Spinal cord pharmacology of pain. Br. J. Anaesth. 75, 193–200. doi: 10.1093/bja/75.2.193
Dickenson, A. H., and Sullivan, A. F. (1986). Electrophysiological studies on the effects of intrathecal morphine on nociceptive neurones in the rat dorsal horn. Pain 24, 211–222.
Dickenson, A. H., Oliveras, J. L., and Besson, J. M. (1979). Role of the nucleus raphe Magnus in opiate analgesia as studied by the microinjection technique in the rat. Brain Res. 170, 95–111. doi: 10.1016/0006-8993(79)90943-0
Ding, X. H., Hua, F., Sutherly, K., Ardell, J. L., and Williams, C. A. (2008). C2 spinal cord stimulation induces dynorphin release from rat T4 spinal cord: Potential modulation of myocardial ischemia-sensitive neurons. Am. J. Physiol. Regul. Integr. Comp. Physiol. 295, R1519–R1528. doi: 10.1152/AJPREGU.00899.2007
Dionne, R. A., Mueller, G. P., Young, R. F., Greenberg, R. P., Hargreaves, K. M., Gracely, R., et al. (1984). Contrast medium causes the apparent increase in β-endorphin levels in human cerebrospinal fluid following brain stimulation. Pain 20, 313–321. doi: 10.1016/0304-3959(84)90109-X
DosSantos, M. F., Martikainen, I. K., Nascimento, T. D., Love, T. M., DeBoer, M. D., Schambra, H. M., et al. (2014). Building up analgesia in humans via the endogenous μ-opioid system by combining placebo and active tDCS: A preliminary report. PLoS One 9:e102350. doi: 10.1371/journal.pone.0102350
Dostrovsky, J. O. (2000). Role of thalamus in pain. Prog. Brain Res. 129, 245–257. doi: 10.1016/S0079-6123(00)29018-3
Drolet, G., van Bockstaele, E. J., and Aston-Jones, G. (1992). Robust enkephalin innervation of the locus coeruleus from the rostral medulla. J. Neurosci. 12, 3162–3174. doi: 10.1523/jneurosci.12-08-03162.1992
Duan, B., Cheng, L., and Ma, Q. (2018). Spinal circuits transmitting mechanical pain and itch. Neurosci. Bull. 34, 186–193. doi: 10.1007/s12264-017-0136-z
Duncan, G. H., Bushnell, M. C., and Marchand, S. (1991). Deep brain stimulation: A review of basic research and clinical studies. Pain 45, 49–59. doi: 10.1016/0304-3959(91)90164-S
Eippert, F., Bingel, U., Schoell, E. D., Yacubian, J., Klinger, R., Lorenz, J., et al. (2009). Activation of the opioidergic descending pain control system underlies placebo analgesia. Neuron 63, 533–543. doi: 10.1016/j.neuron.2009.07.014
Erbs, E., Faget, L., Scherrer, G., Matifas, A., Filliol, D., Vonesch, J. L., et al. (2015). A mu–delta opioid receptor brain atlas reveals neuronal co-occurrence in subcortical networks. Brain Struct. Funct. 220, 677–702. doi: 10.1007/s00429-014-0717-9
Erfanparast, A., Tamaddonfard, E., Taati, M., and Dabaghi, M. (2015). Role of the thalamic submedius nucleus histamine H1 and H2 and opioid receptors in modulation of formalin-induced orofacial pain in rats. Naunyn Schmiedebergs Arch. Pharmacol. 388, 1089–1096. doi: 10.1007/s00210-015-1143-0
Ezra, M., Faull, O. K., Jbabdi, S., and Pattinson, K. T. (2015). Connectivity-based segmentation of the periaqueductal gray matter in human with brainstem optimized diffusion MRI. Hum. Brain Mapp. 36, 3459–3471. doi: 10.1002/hbm.22855
Fallon, J. H., and Leslie, F. M. (1986). Distribution of dynorphin and enkephalin peptides in the rat brain. J. Comp. Neurol. 249, 293–336. doi: 10.1002/CNE.902490302
Farrell, S. M., Green, A., and Aziz, T. (2018). The current state of deep brain stimulation for chronic pain and its context in other forms of neuromodulation. Brain Sci. 8:158. doi: 10.3390/BRAINSCI8080158
Fessler, R. G., Brown, F. D., Rachlin, J. R., Mullan, S., and Fang, V. S. (1984). Elevated β-endorphin in cerebrospinal fluid after electrical brain stimulation: Artifact of contrast infusion? Science 224, 1017–1019. doi: 10.1126/SCIENCE.6326266
Fields, H. (2004). State-dependent opioid control of pain. Nat. Rev. Neurosci. 5, 565–575. doi: 10.1038/nrn1431
Foltz, E. L., and White, L. E. (1962). Pain “Relief” by frontal cingulumotomy. J. Neurosurg. 19, 89–100. doi: 10.3171/JNS.1962.19.2.0089
Fonoff, E. T., Dale, C. S., Pagano, R. L., Paccola, C. C., Ballester, G., Teixeira, M. J., et al. (2009). Antinociception induced by epidural motor cortex stimulation in naive conscious rats is mediated by the opioid system. Behav. Brain Res. 196, 63–70. doi: 10.1016/j.bbr.2008.07.027
Font, J., López-Cano, M., Notartomaso, S., Scarselli, P., di Pietro, P., Bresolí-Obach, R., et al. (2017). Optical control of pain in vivo with a photoactive mGlu5 receptor negative allosteric modulator. Elife 6:e23545. doi: 10.7554/eLife.23545
Fontaine, D., Hamani, C., and Lozano, A. (2009). Efficacy and safety of motor cortex stimulation for chronic neuropathic pain: Critical review of the literature. J. Neurosurg. 110, 251–256. doi: 10.3171/2008.6.17602
França, N. R. M., Toniolo, E. F., Franciosi, A. C., Alves, A. S., de Andrade, D. C., Fonoff, E. T., et al. (2013). Antinociception induced by motor cortex stimulation: Somatotopy of behavioral response and profile of neuronal activation. Behav. Brain Res. 250, 211–221. doi: 10.1016/j.bbr.2013.05.019
François, A., Low, S. A., Sypek, E. I., Christensen, A. J., Sotoudeh, C., Beier, K. T., et al. (2017). A brainstem-spinal cord inhibitory circuit for mechanical pain modulation by GABA and enkephalins. Neuron 93, 822–839.e6. doi: 10.1016/J.NEURON.2017.01.008
Freeman, T. B., Campbell, J. N., and Long, D. M. (1983). Naloxone does not affect pain relief induced by electrical stimulation in man. Pain 17, 189–195. doi: 10.1016/0304-3959(83)90142-2
Fregni, F., Gimenes, R., Valle, A. C., Ferreira, M. J. L., Rocha, R. R., Natalle, L., et al. (2006b). A randomized, sham-controlled, proof of principle study of transcranial direct current stimulation for the treatment of pain in fibromyalgia. Arthritis Rheum. 54, 3988–3998. doi: 10.1002/art.22195
Fregni, F., Boggio, P. S., Lima, M. C., Ferreira, M. J. L., Wagner, T., Rigonatti, S. P., et al. (2006a). A sham-controlled, phase II trial of transcranial direct current stimulation for the treatment of central pain in traumatic spinal cord injury. Pain 122, 197–209. doi: 10.1016/j.pain.2006.02.023
Friebel, U., Eickhoff, S. B., and Lotze, M. (2011). Coordinate-based meta-analysis of experimentally induced and chronic persistent neuropathic pain. Neuroimage 58, 1070–1080. doi: 10.1016/j.neuroimage.2011.07.022
Friedman, D. P., and Murray, E. A. (1986). Thalamic connectivity of the second somatosensory area and neighboring somatosensory fields of the lateral sulcus of the macaque. J. Comp. Neurol. 252, 348–373. doi: 10.1002/cne.902520305
Frizon, L. A., Yamamoto, E. A., Nagel, S. J., Simonson, M. T., Hogue, O., and Machado, A. G. (2020). Deep brain stimulation for pain in the modern era: A systematic review. Clin. Neurosurg. 86, 191–202. doi: 10.1093/neuros/nyy552
Fuchs, P. N., Peng, Y. B., Boyette-Davis, J. A., and Uhelski, M. L. (2014). The anterior cingulate cortex and pain processing. Front. Integr. Neurosci. 8:35. doi: 10.3389/fnint.2014.00035
Galhardoni, R., da Silva, V. A., García-Larrea, L., Dale, C., Baptista, A. F., Barbosa, L. M., et al. (2019). Insular and anterior cingulate cortex deep stimulation for central neuropathic pain disassembling the percept of pain. Neurology 92, E2165–E2175. doi: 10.1212/WNL.0000000000007396
García-Larrea, L., Peyron, R., Mertens, P., Gregoire, M. C., Lavenne, F., le Bars, D., et al. (1999). Electrical stimulation of motor cortex for pain control: A combined PET-scan and electrophysiological study. Pain 83, 259–273. doi: 10.1016/S0304-3959(99)00114-1
George, D. T., Ameli, R., and Koob, G. F. (2019). Periaqueductal GRAY sheds light on dark areas of psychopathology. Trends Neurosci. 42, 349–360. doi: 10.1016/j.tins.2019.03.004
Giacchino, J. L., and Henriksen, S. J. (1998). Opioid effects on activation of neurons in the medial prefrontal cortex. Prog. Neuropsychopharmacol. Biol. Psychiatry 22, 1157–1178. doi: 10.1016/S0278-5846(98)00053-0
Gintzler, A. R., and Chakrabarti, S. (2006). Post-opioid receptor adaptations to chronic morphine; altered functionality and associations of signaling molecules. Life Sci. 79, 717–722. doi: 10.1016/j.lfs.2006.02.016
Göbel, W., and Helmchen, F. (2007). In vivo calcium imaging of neural network function. Physiology 22, 358–365. doi: 10.1152/physiol.00032.2007
Goldberg, D. S., and McGee, S. J. (2011). Pain as a global public health priority. BMC Public Health 11:770. doi: 10.1186/1471-2458-11-770
Gomes, I., Sierra, S., Lueptow, L., Gupta, A., Gouty, S., Margolis, E. B., et al. (2020). Biased signaling by endogenous opioid peptides. Proc. Natl. Acad. Sci. U.S.A. 117, 11820–11828. doi: 10.1073/pnas.2000712117
Gomtsian, L., Bannister, K., Eyde, N., Robles, D., Dickenson, A. H., Porreca, F., et al. (2018). Morphine effects within the rodent anterior cingulate cortex and rostral ventromedial medulla reveal separable modulation of affective and sensory qualities of acute or chronic pain. Pain 159, 2512–2521. doi: 10.1097/j.pain.0000000000001355
Graff-Guerrero, A., González-Olvera, J., Fresán, A., Gómez-Martín, D., Carlos Méndez-Núñez, J., and Pellicer, F. (2005). Repetitive transcranial magnetic stimulation of dorsolateral prefrontal cortex increases tolerance to human experimental pain. Cogn. Brain Res. 25, 153–160. doi: 10.1016/j.cogbrainres.2005.05.002
Graham, R. D., Sankarasubramanian, V., and Lempka, S. F. (2022). Dorsal root ganglion stimulation for chronic pain: Hypothesized mechanisms of action. J. Pain 23, 196–211. doi: 10.1016/j.jpain.2021.07.008
Gray, A. M., Pounds-Cornish, E., Eccles, F. J. R., Aziz, T. Z., Green, A. L., and Scott, R. B. (2014). Deep brain stimulation as a treatment for neuropathic pain: A longitudinal study addressing neuropsychological outcomes. J. Pain 15, 283–292. doi: 10.1016/J.JPAIN.2013.11.003
Gu, L., Uhelski, M. L., Anand, S., Romero-Ortega, M., Kim, Y., Fuchs, P. N., et al. (2015). Pain inhibition by optogenetic activation of specific anterior cingulate cortical neurons. PLoS One 10:e0117746. doi: 10.1371/journal.pone.0117746
Guajardo, H. M., Snyder, K., Ho, A., and Valentino, R. J. (2017). Sex differences in μ-opioid receptor regulation of the rat locus coeruleus and their cognitive consequences. Neuropsychopharmacology 42, 1295–1304. doi: 10.1038/npp.2016.252
Gutstein, H. B., Mansour, A., Watson, S. J., Akil, H., and Fields, H. L. (1998). Mu and kappa opioid receptors in periaqueductal gray and rostral ventromedial medulla. Neuroreport 9, 1777–1781. doi: 10.1097/00001756-199806010-00019
Gybels, J., and Kupers, R. (1990). Deep brain stimulation in the treatment of chronic pain in man: Where and why? Neurophysiol. Clin. 20, 389–398. doi: 10.1016/S0987-7053(05)80206-0
Hammond, D. L., and Yaksh, T. L. (1984). Antagonism of stimulation-produced antinociception by intrathecal administration of methysergide or phentolamine. Brain Res. 298, 329–337. doi: 10.1016/0006-8993(84)91432-X
Hardy, S. G. P., and Leichnetz, G. R. (1981). Frontal cortical projections to the periaqueductal gray in the rat: A retrograde and orthograde horseradish peroxidase study. Neurosci. Lett. 23, 13–17. doi: 10.1016/0304-3940(81)90183-X
Harlan, R. E., Shivers, B. D., Romano, G. J., Pfaff, D. W., and Howells, R. D. (1987). Localization of preproenkephalin mRNA in the rat brain and spinal cord by in situ hybridization. J. Comp. Neurol. 258, 159–184. doi: 10.1002/cne.902580202
Heilbronner, S. R., and Hayden, B. Y. (2016). Dorsal anterior cingulate cortex: A bottom-up view. Annu. Rev. Neurosci. 39:149. doi: 10.1146/ANNUREV-NEURO-070815-013952
Heinke, B., Gingl, E., and Sandkühler, J. (2011). Multiple targets of μ-opioid receptor-mediated presynaptic inhibition at primary afferent Aδ- and C-fibers. J. Neurosci. 31, 1313–1322. doi: 10.1523/JNEUROSCI.4060-10.2011
Heinricher, M. M., Morgan, M. M., Tortorici, V., and Fields, H. L. (1994). Disinhibition of off-cells and antinociception produced by an opioid action within the rostral ventromedial medulla. Neuroscience 63, 279–288. doi: 10.1016/0306-4522(94)90022-1
Helm, S., Shirsat, N., Calodney, A., Abd-Elsayed, A., Kloth, D., Soin, A., et al. (2021). Peripheral nerve stimulation for chronic pain: A systematic review of effectiveness and safety. Pain Ther. 10, 985–1002. doi: 10.1007/s40122-021-00306-4
Hentall, I. D., Luca, C. C., Widerstrom-Noga, E., Vitores, A., Fisher, L. D., Martinez-Arizala, A., et al. (2016). The midbrain central gray best suppresses chronic pain with electrical stimulation at very low pulse rates in two human cases. Brain Res. 1632, 119–126. doi: 10.1016/j.brainres.2015.12.021
Hirschberg, S., Li, Y., Randall, A., Kremer, E. J., and Pickering, A. E. (2017). Functional dichotomy in spinal- vs prefrontal-projecting locus coeruleus modules splits descending noradrenergic analgesia from ascending aversion and anxiety in rats. Elife 6, 1–26. doi: 10.7554/elife.29808
Holden, J. E., Schwartz, E. J., and Proudfit, H. K. (1999). Microinjection of morphine in the A7 catecholamine cell group produces opposing effects on nociception that are mediated by α1- and α2- adrenoceptors. Neuroscience 91, 979–990. doi: 10.1016/S0306-4522(98)00673-3
Hollingworth, M., Sims-Williams, H. P., Pickering, A. E., Barua, N., and Patel, N. K. (2017). Single electrode deep brain stimulation with dual targeting at dual frequency for the treatment of chronic pain: A case series and review of the literature. Brain Sci. 7:9. doi: 10.3390/BRAINSCI7010009
Holsheimer, J. (2002). Which neuronal elements are activated directly by spinal cord stimulation. Neuromodulation 5, 25–31. doi: 10.1046/j.1525-1403.2002._2005.x
Hosobuchi, Y. (1986). Subcortical electrical stimulation for control of intractable pain in humans. Report of 122 cases (1970-1984). J. Neurosurg. 64, 543–553. doi: 10.3171/jns.1986.64.4.0543
Hosobuchi, Y., Adams, J. E., and Linchitz, R. (1977). Pain relief by electrical stimulation of the central gray matter in humans and its reversal by naloxone. Science 197, 183–186. doi: 10.1126/SCIENCE.301658
Hosobuchi, Y., Adams, J. E., and Rutkin, B. (1973). Chronic thalamic stimulation for the control of facial anesthesia dolorosa. Arch. Neurol. 29, 158–161. doi: 10.1001/ARCHNEUR.1973.00490270040005
Hosobuchi, Y., Rossier, J., Bloom, F. E., and Guillemin, R. (1979). Stimulation of human periaqueductal gray for pain relief increases immunoreactive β-endorphin in ventricular fluid. Science 203, 279–281. doi: 10.1126/science.83674
Huang, J., Gadotti, V. M., Chen, L., Souza, I. A., Huang, S., Wang, D., et al. (2019). A neuronal circuit for activating descending modulation of neuropathic pain. Nat. Neurosci. 22, 1659–1668. doi: 10.1038/s41593-019-0481-5
Huang, J., Zhang, Z., Gambeta, E., Chen, L., and Zamponi, G. W. (2021). An orbitofrontal cortex to midbrain projection modulates hypersensitivity after peripheral nerve injury. Cell Rep. 35:109033. doi: 10.1016/j.celrep.2021.109033
Hüll, K., Morstein, J., and Trauner, D. (2018). In vivo photopharmacology. Chem. Rev. 118, 10710–10747. doi: 10.1021/acs.chemrev.8b00037
Hussin, A. T., Boychuk, J. A., Brown, A. R., Pittman, Q. J., and Campbell Teskey, G. (2015). Intracortical microstimulation (ICMS) activates motor cortex layer 5 pyramidal neurons mainly transsynaptically. Brain Stimul. 8, 742–750. doi: 10.1016/J.BRS.2015.03.003
Hutchison, W. D., Davis, K. D., Lozano, A. M., Tasker, R. R., and Dostrovsky, J. O. (1999). Pain-related neurons in the human cingulate cortex. Nat. Neurosci. 5, 403–405. doi: 10.1038/8065
Inoue, S., Johanek, L. M., and Sluka, K. A. (2017). Lack of analgesic synergy of the cholecystokinin receptor antagonist proglumide and spinal cord stimulation for the treatment of neuropathic pain in rats. Neuromodulation 20, 534–542. doi: 10.1111/NER.12601
Jensen, T. S., and Yaksh, T. L. (1989). Comparison of the antinociceptive effect of morphine and glutamate at coincidental sites in the periaqueductal gray and medial medulla in rats. Brain Res. 476, 1–9. doi: 10.1016/0006-8993(89)91529-1
Jensen, T. S., Baron, R., Haanpää, M., Kalso, E., Loeser, J. D., Rice, A. S. C., et al. (2011). A new definition of neuropathic pain. Pain 152, 2204–2205. doi: 10.1016/J.PAIN.2011.06.017
Jermakowicz, W. J., Hentall, I. D., Jagid, J. R., Luca, C. C., Adcock, J., Martinez-Arizala, A., et al. (2017). Deep brain stimulation improves the symptoms and sensory signs of persistent central neuropathic pain from spinal cord injury: A case report. Front. Hum. Neurosci. 11:177. doi: 10.3389/FNHUM.2017.00177
Jiang, L., Ji, Y., Voulalas, P. J., Keaser, M., Xu, S., Gullapalli, R. P., et al. (2014). Motor cortex stimulation suppresses cortical responses to noxious hindpaw stimulation after spinal cord lesion in rats. Brain Stimul. 7, 182–189. doi: 10.1016/J.BRS.2013.12.013
Johansen, J. P., and Fields, H. L. (2004). Glutamatergic activation of anterior cingulate cortex produces an aversive teaching signal. Nat. Neurosci. 7, 398–403. doi: 10.1038/nn1207
Jones, A. K. P., Kitchen, N. D., Watabe, T., Cunningham, J., Jones, T., Luthra, K., et al. (1999). Measurement of changes in opioid receptor binding in vivo during trigeminal neuralgic pain using e 1 C]diprenorphine and positron emission tomography. J. Cereb. Blood Flow Metab. 19, 803–808. doi: 10.1097/00004647-199907000-00011
Jones, M. G., Rogers, E. R., Harris, J. P., Sullivan, A., Ackermann, D. M., Russo, M., et al. (2021). Neuromodulation using ultra low frequency current waveform reversibly blocks axonal conduction and chronic pain. Sci. Transl. Med. 13:eabg9890. doi: 10.1126/scitranslmed.abg9890
Jones, S. L., and Gebhart, G. F. (1988). Inhibition of spinal nociceptive transmission from the midbrain, pons and medulla in the rat: Activation of descending inhibition by morphine, glutamate and electrical stimulation. Brain Res. 460, 281–296. doi: 10.1016/0006-8993(88)90373-3
Juavinett, A. L., Bekheet, G., and Churchland, A. K. (2019). Chronically implanted neuropixels probes enable high-yield recordings in freely moving mice. Elife 8:e47188. doi: 10.7554/eLife.47188
Jun, J. J., Steinmetz, N. A., Siegle, J. H., Denman, D. J., Bauza, M., Barbarits, B., et al. (2017). Fully integrated silicon probes for high-density recording of neural activity. Nature 551, 232–236. doi: 10.1038/nature24636
Kalyuzhny, A. E., Arvidsson, U., Wu, W., and Wessendorf, M. W. (1996). μ-opioid and δ-opioid receptors are expressed in brainstem antinociceptive circuits: Studies using immunocytochemistry and retrograde tract-tracing. J. Neurosci. 16, 6490–6503. doi: 10.1523/jneurosci.16-20-06490.1996
Kang, S. J., Kwak, C., Lee, J., Sim, S. E., Shim, J., Choi, T., et al. (2015). Bidirectional modulation of hyperalgesia via the specific control of excitatory and inhibitory neuronal activity in the ACC. Mol. Brain 8, 1–11. doi: 10.1186/s13041-015-0170-6
Kapural, L., Yu, C., Doust, M. W., Gliner, B. E., Vallejo, R., Todd Sitzman, B., et al. (2015). Novel 10-kHz high-frequency therapy (HF10 Therapy) is superior to traditional low-frequency spinal cord stimulation for the treatment of chronic back and leg pain: The SENZA-rct randomized controlled trial. Anesthesiology 123, 851–860. doi: 10.1097/ALN.0000000000000774
Kashanian, A., DiCesare, J. A. T., Rohatgi, P., Albano, L., Krahl, S. E., Bari, A., et al. (2020). Case series: Deep brain stimulation for facial pain. Oper. Neurosurg. (Hagerstown) 19, 510–517. doi: 10.1093/ONS/OPAA170
Khachaturian, H., Lewis, M. E., and Watson, S. J. (1983). Enkephalin systems in diencephalon and brainstem of the rat. J. Comp. Neurol. 220, 310–320. doi: 10.1002/cne.902200305
Kiefel, J. M., Rossi, G. C., and Bodnar, R. J. (1993). Medullary μ and δ opioid receptors modulate mesencephalic morphine analgesia in rats. Brain Res. 624, 151–161. doi: 10.1016/0006-8993(93)90073-V
Kim, J., Ryu, S. B., Lee, S. E., Shin, J., Jung, H. H., Kim, S. J., et al. (2016). Motor cortex stimulation and neuropathic pain: How does motor cortex stimulation affect pain-signaling pathways? J. Neurosurg. 124, 866–876. doi: 10.3171/2015.1.JNS14891
Komboz, F., Mehsein, Z., Kobaïter-Maarrawi, S., Chehade, H. D., and Maarrawi, J. (2022). Epidural posterior insular stimulation alleviates neuropathic pain manifestations in rats with spared nerve injury through endogenous opioid system. Neuromodulation doi: 10.1016/j.neurom.2022.01.002
Kong, J., Tu, P. C., Zyloney, C., and Su, T. P. (2010). Intrinsic functional connectivity of the periaqueductal gray, a resting fMRI study. Behav. Brain Res. 211, 215–219. doi: 10.1016/j.bbr.2010.03.042
Koyama, T., Tanaka, Y. Z., and Mikami, A. (1998). Nociceptive neurons in the macaque anterior cingulate activate during anticipation of pain. Neuroreport 9, 2663–2667. doi: 10.1097/00001756-199808030-00044
Kumar, K., and Wyant, G. M. (1985). Deep brain stimulation for alleviating chronic intractable pain. Can. J. Surg. 28, 20–22.
Kumar, K., Rizvi, S., Nguyen, R., Abbas, M., Bishop, S., and Murthy, V. (2014). Impact of wait times on spinal cord stimulation therapy outcomes. Pain Pract. 14, 709–720. doi: 10.1111/PAPR.12126
Kumar, K., Taylor, R. S., Jacques, L., Eldabe, S., Meglio, M., Molet, J., et al. (2007). Spinal cord stimulation versus conventional medical management for neuropathic pain: A multicentre randomised controlled trial in patients with failed back surgery syndrome. Pain 132, 179–188. doi: 10.1016/J.PAIN.2007.07.028
Kumar, K., Toth, C., Nath, R., and Laing, P. (1998). Epidural spinal cord stimulation for treatment of chronic pain–some predictors of success. A 15-year experience. Surg. Neurol. 50, 110–121. doi: 10.1016/S0090-3019(98)00012-3
Kumar, R., Lozano, A. M., Kim, Y. J., Hutchison, W. D., Sime, E., Halket, E., et al. (1998). Double-blind evaluation of subthalamic nucleus deep brain stimulation in advanced Parkinson’s disease. Neurology 51, 850–855. doi: 10.1212/WNL.51.3.850
Kumar, K., Wyant, G. M., and Nath, R. (1990). Deep brain stimulation for control of intractable pain in humans, present and future: A ten-year follow-up. Neurosurgery 26:774. doi: 10.1097/00006123-199005000-00007
Kwan, C. L., Crawley, A. P., Mikulis, D. J., and Davis, K. D. (2000). An fMRI study of the anterior cingulate cortex and surrounding medial wall activations evoked by noxious cutaneous heat and cold stimuli. Pain 85, 359–374. doi: 10.1016/S0304-3959(99)00287-0
Lad, S. P., Petraglia, F. W., Kent, A. R., Cook, S., Murphy, K. R., Dalal, N., et al. (2016). Longer delay from chronic pain to spinal cord stimulation results in higher healthcare resource utilization. Neuromodulation 19, 469–476. doi: 10.1111/NER.12389
LaGraize, S. C., Borzan, J., Peng, Y. B., and Fuchs, P. N. (2006). Selective regulation of pain affect following activation of the opioid anterior cingulate cortex system. Exp. Neurol. 197, 22–30. doi: 10.1016/J.EXPNEUROL.2005.05.008
Lamusuo, S., Hirvonen, J., Lindholm, P., Martikainen, I. K., Hagelberg, N., Parkkola, R., et al. (2017). Neurotransmitters behind pain relief with transcranial magnetic stimulation–positron emission tomography evidence for release of endogenous opioids. Eur. J. Pain 21, 1505–1515. doi: 10.1002/ejp.1052
Lau, B. K., and Vaughan, C. W. (2014). Descending modulation of pain: The GABA disinhibition hypothesis of analgesia. Curr. Opin. Neurobiol. 29, 159–164. doi: 10.1016/j.conb.2014.07.010
Laubach, M., Amarante, L. M., Swanson, K., and White, S. R. (2018). What, if anything, is rodent prefrontal cortex? eNeuro 5. doi: 10.1523/ENEURO.0315-18.2018
le Merrer, J., Becker, J. A. J., Befort, K., and Kieffer, B. L. (2009). Reward processing by the opioid system in the brain. Physiol. Rev. 89, 1379–1412. doi: 10.1152/physrev.00005.2009
Lechasseur, Y., Dufour, S., Lavertu, G., Bories, C., Deschênes, M., Vallée, R., et al. (2011). A microprobe for parallel optical and electrical recordings from single neurons in vivo. Nat. Methods 8, 319–325. doi: 10.1038/nmeth.1572
Lee, K. S., Huang, Y. H., and Yen, C. T. (2012). Periaqueductal gray stimulation suppresses spontaneous pain behavior in rats. Neurosci. Lett. 514, 42–45. doi: 10.1016/J.NEULET.2012.02.053
Lefaucheur, J. P., Drouot, X., and Nguyen, J. P. (2001). Interventional neurophysiology for pain control: Duration of pain relief following repetitive transcranial magnetic stimulation of the motor cortex. Neurophysiol. Clin. 31, 247–252. doi: 10.1016/S0987-7053(01)00260-X
Lefaucheur, J. P., Drouot, X., Cunin, P., Bruckert, R., Lepetit, H., Crange, A., et al. (2009). Motor cortex stimulation for the treatment of refractory peripheral neuropathic pain. Brain 132, 1463–1471. doi: 10.1093/BRAIN/AWP035
Lefaucheur, J. P., Drouot, X., Menard-Lefaucheur, I., Keravel, Y., and Nguyen, J. P. (2006). Motor cortex rTMS restores defective intracortical inhibition in chronic neuropathic pain. Neurology 67, 1568–1574. doi: 10.1212/01.wnl.0000242731.10074.3c
Lempka, S. F., and Patil, P. G. (2018). Innovations in spinal cord stimulation for pain. Curr. Opin. Biomed. Eng. 8, 51–60. doi: 10.1016/J.COBME.2018.10.005
Lempka, S. F., Zander, H. J., Anaya, C. J., Wyant, A., Ozinga, J. G. IV, and Machado, A. G. (2020). Patient-specific analysis of neural activation during spinal cord stimulation for pain. Neuromodulation 23, 572–581. doi: 10.1111/ner.13037
Lenoir, C., Algoet, M., and Mouraux, A. (2018). Deep continuous theta burst stimulation of the operculo-insular cortex selectively affects Aδ-fibre heat pain. J. Physiol. 596, 4767–4787. doi: 10.1113/JP276359
Levy, R. M., Lamb, S., and Adams, J. E. (1987). Treatment of chronic pain by deep brain stimulation: Long term follow-up and review of the literature. Neurosurgery 21, 885–893. doi: 10.1227/00006123-198712000-00017
Lewis, M. E., Pert, A., Pert, C. B., and Herkenham, M. (1983). Opiate receptor localization in rat cerebral cortex. J. Comp. Neurol. 216, 339–358. doi: 10.1002/CNE.902160310
Li, J., and Sheets, P. L. (2018). The central amygdala to periaqueductal gray pathway comprises intrinsically distinct neurons differentially affected in a model of inflammatory pain. J. Physiol. 596, 6289–6305. doi: 10.1113/JP276935
Li, Y., Hickey, L., Perrins, R., Werlen, E., Patel, A. A., Hirschberg, S., et al. (2016). Retrograde optogenetic characterization of the pontospinal module of the locus coeruleus with a canine adenoviral vector. Brain Res. 1641, 274–290. doi: 10.1016/j.brainres.2016.02.023
Lin, T., Gargya, A., Singh, H., Sivanesan, E., and Gulati, A. (2020). Mechanism of peripheral nerve stimulation in chronic pain. Pain Med. 21, S6–S12. doi: 10.1093/pm/pnaa164
Lind, G., Meyerson, B. A., Winter, J., and Linderoth, B. (2004). Intrathecal baclofen as adjuvant therapy to enhance the effect of spinal cord stimulation in neuropathic pain: A pilot study. Eur. J. Pain 8, 377–383. doi: 10.1016/j.ejpain.2003.11.002
Lind, G., Schechtmann, G., Winter, J., and Linderoth, B. (2007). “Drug-enhanced spinal stimulation for pain: A new strategy,” in Operative neuromodulation, eds D. E. Sakas, B. A. Simpson, and E. S. Krames (Vienna: Springer), 57–63. doi: 10.1007/978-3-211-33079-1_7
Linnman, C., Moulton, E. A., Barmettler, G., Becerra, L., and Borsook, D. (2012). Neuroimaging of the periaqueductal gray: State of the field. Neuroimage 60, 505–522. doi: 10.1016/j.neuroimage.2011.11.095
Linseman, M. A., and Grupp, L. A. (1980). Acute and chronic opiate effects on single units and EEG of medial thalamus and hippocampus: A latency analysis. Psychopharmacology (Berl) 71, 11–20. doi: 10.1007/BF00433246
Liu, C. C., Moosa, S., Quigg, M., and Elias, W. J. (2021). Anterior insula stimulation increases pain threshold in humans: A pilot study. J. Neurosurg. 135, 1487–1492. doi: 10.3171/2020.10.JNS203323
Llewelyn, H. B., Azami, J., and Roberts, M. H. T. (1984). Antinociception produced by stimulation of the periaqueductal grey matter: Effects of antagonists microinjected into the nucleus raphe Magnus. Pain 18:S220. doi: 10.1016/0304-3959(84)90459-7
Lobb, C. J. (2014). Abnormal bursting as a pathophysiological mechanism in Parkinson’s disease. Basal Ganglia 3, 187–195. doi: 10.1016/j.baga.2013.11.002
López-Cano, M., Font, J., Aso, E., Sahlholm, K., Cabré, G., Giraldo, J., et al. (2021). Remote local photoactivation of morphine produces analgesia without opioid-related adverse effects. Br. J. Pharmacol. 1–17. doi: 10.1111/bph.15645
Lorenz, J., Minoshima, S., and Casey, K. L. (2003). Keeping pain out of mind: The role of the dorsolateral prefrontal cortex in pain modulation. Brain 126, 1079–1091. doi: 10.1093/brain/awg102
Loyd, D. R., Wang, X., and Murphy, A. Z. (2008). Sex differences in μ-opioid receptor expression in the rat midbrain periaqueductal gray are essential for eliciting sex differences in morphine analgesia. J. Neurosci. 28:14007. doi: 10.1523/JNEUROSCI.4123-08.2008
Lu, C., Yang, T., Zhao, H., Zhang, M., Meng, F., Fu, H., et al. (2016). Insular cortex is critical for the perception, modulation, and chronification of pain. Neurosci. Bull. 32, 191–201. doi: 10.1007/s12264-016-0016-y
Lund, I., Lundeberg, T., Sandberg, L., Budh, C. N., Kowalski, J., and Svensson, E. (2005). Lack of interchangeability between visual analogue and verbal rating pain scales: A cross sectional description of pain etiology groups. BMC Med. Res. Methodol. 5:31. doi: 10.1186/1471-2288-5-31
Lüscher, C., Pascoli, V., and Creed, M. (2015). Optogenetic dissection of neural circuitry: From synaptic causalities to blue prints for novel treatments of behavioral diseases. Curr. Opin. Neurobiol. 35, 95–100. doi: 10.1016/J.CONB.2015.07.005
Maarrawi, J., Peyron, R., Mertens, P., Costes, N., Magnin, M., Sindou, M., et al. (2007). Motor cortex stimulation for pain control induces changes in the endogenous opioid system. Neurology 69, 827–834. doi: 10.1212/01.wnl.0000269783.86997.37
Maarrawi, J., Peyron, R., Mertens, P., Costes, N., Magnin, M., Sindou, M., et al. (2013). Brain opioid receptor density predicts motor cortex stimulation efficacy for chronic pain. Pain 154, 2563–2568. doi: 10.1016/j.pain.2013.07.042
Majedi, H., Dehghani, S. S., Soleyman-Jahi, S., Tafakhori, A., Emami, S. A., Mireskandari, M., et al. (2019). Assessment of factors predicting inadequate pain management in chronic pain patients. Anesth. Pain Med. 9:97229. doi: 10.5812/AAPM.97229
Malinowski, M. N., Jain, S., Jassal, N., and Deer, T. (2020). Spinal cord stimulation for the treatment of neuropathic pain: Expert opinion and 5-year outlook. Exp. Rev. Med. Devices 17, 1293–1302. doi: 10.1080/17434440.2020.1801411
Manchikanti, L., Helm, S. II, Fellows, B., Janata, J. W., Pampati, V., Grider, J. S., et al. (2012). Opioid epidemic in the United States. Pain Physician 15, ES9–ES38.
Mannion, R. J., and Woolf, C. J. (2000). Pain mechanisms and management: A central perspective. Clin. J. Pain 16, S144–S156. doi: 10.1097/00002508-200009001-00006
Mansour, A., Fox, C. A., Burke, S., Meng, F., Thompson, R. C., Akil, H., et al. (1994). Mu, delta, and kappa opioid receptor mRNA expression in the rat CNS: An in situ hybridization study. J. Comp. Neurol. 350, 412–438. doi: 10.1002/cne.903500307
Martini, L., and Whistler, J. L. (2007). The role of mu opioid receptor desensitization and endocytosis in morphine tolerance and dependence. Curr. Opin. Neurobiol. 17, 556–564. doi: 10.1016/j.conb.2007.10.004
Marvizón, J. C. G., Chen, W., and Murphy, N. (2009). Enkephalins, dynorphins, and β-endorphin in the rat dorsal horn: An immunofluorescence colocalization study. J. Comp. Neurol. 517, 51–68. doi: 10.1002/cne.22130
Mastro, K. J., Zitelli, K. T., Willard, A. M., Leblanc, K. H., Kravitz, A. V., and Gittis, A. H. (2017). Cell-specific pallidal intervention induces long-lasting motor recovery in dopamine-depleted mice. Nat. Neurosci. 20, 815–823. doi: 10.1038/NN.4559
Mayer, D. J., Wolfle, T. L., Akil, H., Carder, B., and Liebeskind, J. C. (1971). Analgesia from electrical stimulation in the brainstem of the rat. Science 174, 1351–1354.
Mazzola, L., Isnard, J., Peyron, R., Guénot, M., and Mauguière, F. (2009). Somatotopic organization of pain responses to direct electrical stimulation of the human insular cortex. Pain 146, 99–104. doi: 10.1016/J.PAIN.2009.07.014
McIntyre, C. C., and Grill, W. M. (1999). Excitation of central nervous system neurons by nonuniform electric fields. Biophys. J. 76, 878–888. doi: 10.1016/S0006-3495(99)77251-6
McIntyre, C. C., Grill, W. M., Sherman, D. L., and Thakor, N. V. (2004). Cellular effects of deep brain stimulation: Model-based analysis of activation and inhibition. J. Neurophysiol. 91, 1457–1469. doi: 10.1152/JN.00989.2003
McMahon, D., O’Reilly, M. A., and Hynynen, K. (2021). Therapeutic agent delivery across the blood-brain barrier using focused ultrasound. Annu. Rev. Biomed. Eng. 23, 89–113. doi: 10.1146/ANNUREV-BIOENG-062117-121238
Meda, K. S., Patel, T., Braz, J. M., Malik, R., Turner, M. L., Seifikar, H., et al. (2019). Microcircuit mechanisms through which mediodorsal thalamic input to anterior cingulate cortex exacerbates pain-related aversion. Neuron 102, 944–959.e3. doi: 10.1016/j.neuron.2019.03.042
Mekhail, N., Levy, R. M., Deer, T. R., Kapural, L., Li, S., Amirdelfan, K., et al. (2020). Long-term safety and efficacy of closed-loop spinal cord stimulation to treat chronic back and leg pain (Evoke): A double-blind, randomised, controlled trial. Lancet Neurol. 19, 123–134. doi: 10.1016/S1474-4422(19)30414-4
Melzack, R., and Wall, P. D. (1965). Pain mechanisms: A new theory. Science 150, 971–979. doi: 10.1126/SCIENCE.150.3699.971
Menant, O., Andersson, F., Zelena, D., and Chaillou, E. (2016). The benefits of magnetic resonance imaging methods to extend the knowledge of the anatomical organisation of the periaqueductal gray in mammals. J. Chem. Neuroanat. 77, 110–120. doi: 10.1016/j.jchemneu.2016.06.003
Mendell, L. M. (2013). Constructing and deconstructing the gate theory of pain. Pain 155, 210–216. doi: 10.1016/J.PAIN.2013.12.010
Menetrey, D., and Basbaum, A. I. (1987). The distribution of substance P-, enkephalin-and dynorphin-immunoreactive neurons in the medulla of the rat and their contribution to bulbospinal pathways. Neuroscience 23, 173–187. doi: 10.1016/0306-4522(87)90281-8
Miller, J. F., and Proudfit, H. K. (1990). Antagonism of stimulation-produced antinociception from ventrolateral pontine sites by intrathecal administration of α-adrenergic antagonists and naloxone. Brain Res. 530, 20–34. doi: 10.1016/0006-8993(90)90653-S
Moffitt, M. A., Lee, D. C., and Bradley, K. (2009). “Spinal cord stimulation: Engineering approaches to clinical and physiological challenges,” in Implantable neural prostheses 1. Biological and medical physics, biomedical engineering, eds E. Greenbaum and D. Zhou (New York, NY: Springer), 155–194. doi: 10.1007/978-0-387-77261-5_5
Moisset, X., Goudeau, S., Poindessous-Jazat, F., Baudic, S., Clavelou, P., and Bouhassira, D. (2015). Prolonged continuous theta-burst stimulation is more analgesic than “classical” high frequency repetitive transcranial magnetic stimulation. Brain Stimul. 8, 135–141. doi: 10.1016/j.brs.2014.10.006
Moisset, X., Lanteri-Minet, M., and Fontaine, D. (2020). Neurostimulation methods in the treatment of chronic pain. J. Neural Transm. 127, 673–686. doi: 10.1007/s00702-019-02092-y
Monconduit, L., Bourgeais, L., Bernard, J.-F., le Bars, D., and Villanueva, L. (1999). Ventromedial thalamic neurons convey nociceptive signals from the whole body surface to the dorsolateral neocortex. J. Neurosci. 19, 9063–9072. doi: 10.1523/JNEUROSCI.19-20-09063.1999
Moreau, J. L., and Fields, H. L. (1986). Evidence for GABA involvement in midbrain control of medullary neurons that modulate nociceptive transmission. Brain Res. 397, 37–46. doi: 10.1016/0006-8993(86)91367-3
Morgan, M. M., Gold, M. S., Liebeskind, J. C., and Stein, C. (1991). Periaqueductal gray stimulation produces a spinally mediated, opioid antinociception for the inflamed hindpaw of the rat. Brain Res. 545, 17–23. doi: 10.1016/0006-8993(91)91264-2
Nahmias, F., Debes, C., de Andrade, D. C., Mhalla, A., and Bouhassira, D. (2009). Diffuse analgesic effects of unilateral repetitive transcranial magnetic stimulation (rTMS) in healthy volunteers. Pain 147, 224–232. doi: 10.1016/J.PAIN.2009.09.016
Nandi, D., and Aziz, T. Z. (2004). Deep brain stimulation in the management of neuropathic pain and multiple sclerosis tremor. J. Clin. Neurophysiol. 21, 31–39. doi: 10.1097/00004691-200401000-00005
Nandi, D., Liu, X., Joint, C., Stein, J., and Aziz, T. (2002). Thalamic field potentials during deep brain stimulation of periventricular gray in chronic pain. Pain 97, 47–51. doi: 10.1016/S0304-3959(01)00486-9
Narita, M. (2003). Change in the expression of c-fos in the rat brain following sciatic nerve ligation. Neurosci. Lett. 352, 231–233. doi: 10.1016/s0304-3940(03)01045-0
Navratilova, E., Xie, J. Y., Meske, D., Qu, C., Morimura, K., Okun, A., et al. (2015). Endogenous opioid activity in the anterior cingulate cortex is required for relief of pain. J. Neurosci. 35, 7264–7271. doi: 10.1523/JNEUROSCI.3862-14.2015
Nguyen, E., Smith, K. M., Cramer, N., Holland, R. A., Bleimeister, I. H., Flores-Felix, K., et al. (2022). Medullary kappa-opioid receptor neurons inhibit pain and itch through a descending circuit. Brain 145, 2586–2601. doi: 10.1093/brain/awac189
North, R. A., Williams, J. T., Surprenant, A., and Christie, M. J. (1987). Mu and delta receptors belong to a family of receptors that are coupled to potassium channels. Proc. Natl. Acad. Sci. U.S.A. 84, 5487–5491. doi: 10.1073/pnas.84.15.5487
North, R. B., Ewend, M. G., Lawton, M. T., and Piantadosi, S. (1991). Spinal cord stimulation for chronic, intractable pain: Superiority of “multi-channel” devices. Pain 44, 119–130. doi: 10.1016/0304-3959(91)90125-H
Ntamati, N. R., Creed, M., Achargui, R., and Lüscher, C. (2018). Periaqueductal efferents to dopamine and GABA neurons of the VTA. PLoS One 13:e190297. doi: 10.1371/journal.pone.0190297
O’Connell, N. E., Cossar, J., Marston, L., Wand, B. M., Bunce, D., de Souza, L. H., et al. (2013). Transcranial direct current stimulation of the motor cortex in the treatment of chronic nonspecific low back pain: A randomized, double-blind exploratory study. Clin. J. Pain 29, 26–34. doi: 10.1097/AJP.0B013E318247EC09
Ohnhaus, E. E., and Adler, R. (1975). Methodological problems in the measurement of pain: A comparison between the verbal rating scale and the visual analogue scale. Pain 1, 379–384. doi: 10.1016/0304-3959(75)90075-5
Oliva, V., Hartley-davies, R., Moran, R., Pickering, A. E., and Brooks, C. W. (2022). Simultaneous brain, brainstem and spinal cord pharmacological-fMRI reveals involvement of an endogenous opioid network in attentional analgesia. Elife 11:e71877. doi: 10.7554/eLife.71877
Ong, W. Y., Stohler, C. S., and Herr, D. R. (2019). Role of the prefrontal cortex in pain processing. Mol. Neurobiol. 56, 1137–1166. doi: 10.1007/S12035-018-1130-9
Osborne, P. B., Vaughan, C. W., Wilson, H. I., and Christie, M. J. (1996). Opioid inhibition of rat periaqueductal grey neurones with identified projections to rostral ventromedial medulla in vitro. J. Physiol. 490(Pt 2), 383–389. doi: 10.1113/jphysiol.1996.sp021152
Ostrowsky, K., Magnin, M., Ryvlin, P., Isnard, J., Guenot, M., and Mauguière, F. (2002). Representation of pain and somatic sensation in the human insula: A study of responses to direct electrical cortical stimulation. Cereb. Cortex 12, 376–385. doi: 10.1093/CERCOR/12.4.376
Pagano, R. L., Fonoff, E. T., Dale, C. S., Ballester, G., Teixeira, M. J., and Britto, L. R. G. (2012). Motor cortex stimulation inhibits thalamic sensory neurons and enhances activity of PAG neurons: Possible pathways for antinociception. Pain 153, 2359–2369. doi: 10.1016/j.pain.2012.08.002
Passard, A., Attal, N., Benadhira, R., Brasseur, L., Saba, G., Sichere, P., et al. (2007). Effects of unilateral repetitive transcranial magnetic stimulation of the motor cortex on chronic widespread pain in fibromyalgia. Brain 130, 2661–2670. doi: 10.1093/brain/awm189
Peltz, E., Seifert, F., DeCol, R., Dörfler, A., Schwab, S., and Maihöfner, C. (2011). Functional connectivity of the human insular cortex during noxious and innocuous thermal stimulation. Neuroimage 54, 1324–1335. doi: 10.1016/j.neuroimage.2010.09.012
Pereira, E. A. C., Wang, S., Peachey, T., Lu, G., Shlugman, D., Stein, J. F., et al. (2013). Elevated gamma band power in humans receiving naloxone suggests dorsal periaqueductal and periventricular gray deep brain stimulation produced analgesia is opioid mediated. Exp. Neurol. 239, 248–255. doi: 10.1016/j.expneurol.2012.10.017
Pert, C. B., Kuhar, M. J., and Snyder, S. H. (1976). Opiate receptor: Autoradiographic localization in rat brain. Proc. Natl. Acad. Sci. U.S.A. 73, 3729–3733.
Petrovic, P., Kalso, E., Petersson, K. M., and Ingvar, M. (2002). Placebo and opioid analgesia–imaging a shared neuronal network. Science 295, 1737–1740. doi: 10.1126/SCIENCE.1067176
Peyron, R., Faillenot, I., Mertens, P., Laurent, B., and Garcia-Larrea, L. (2007). Motor cortex stimulation in neuropathic pain. Correlations between analgesic effect and hemodynamic changes in the brain. A pet study. Neuroimage 34, 310–321. doi: 10.1016/j.neuroimage.2006.08.037
Pilitsis, J. G., Fahey, M., Custozzo, A., Chakravarthy, K., and Capobianco, R. (2021). Composite score is a better reflection of patient response to chronic pain therapy compared with pain intensity alone. Neuromodulation 24, 68–75. doi: 10.1111/ner.13212
Prévinaire, J. G., Nguyen, J. P., Perrouin-Verbe, B., and Fattal, C. (2009). Chronic neuropathic pain in spinal cord injury: Efficiency of deep brain and motor cortex stimulation therapies for neuropathic pain in spinal cord injury patients. Ann. Phys. Rehabil. Med. 52, 188–193. doi: 10.1016/J.REHAB.2008.12.002
Proudfit, H. K., and Clark, F. M. (1991). The projections of locus coeruleus neurons to the spinal cord. Amsterdam: Elsevier. doi: 10.1016/S0079-6123(08)63803-0
Proudfit, H. K., and Hammond, D. L. (1981). Alterations in nociceptive threshold and morphine-induced analgesia produced by intrathecally administered amine antagonists. Brain Res. 218, 393–399. doi: 10.1016/0006-8993(81)91318-4
Qu, C., King, T., Okun, A., Lai, J., Fields, H. L., and Porreca, F. (2011). Lesion of the rostral anterior cingulate cortex eliminates the aversiveness of spontaneous neuropathic pain following partial or complete axotomy. Pain 152, 1641–1648. doi: 10.1016/J.PAIN.2011.03.002
Rainville, P., Duncan, G. H., Price, D. D., Carrier, B., and Bushnell, M. C. (1997). Pain affect encoded in human anterior cingulate but not somatosensory cortex. Science 277, 968–971.
Rattay, F. (1986). Analysis of models for external stimulation of axons. IEEE Trans. Biomed. Eng. 33, 974–977. doi: 10.1109/TBME.1986.325670
Rattay, F. (1999). The basic mechanism for the electrical stimulation of the nervous system. Neuroscience 89, 335–346. doi: 10.1016/S0306-4522(98)00330-3
Rodriguez-Raecke, R., Niemeier, A., Ihle, K., Ruether, W., and May, A. (2009). Brain gray matter decrease in chronic pain is the consequence and not the cause of pain. J. Neurosci. 29, 13746–13750. doi: 10.1523/JNEUROSCI.3687-09.2009
Rogers, E. R., Zander, H. J., and Lempka, S. F. (2022). Neural recruitment during conventional, burst, and 10-kHz spinal cord stimulation for pain. J. Pain 23, 434–449. doi: 10.1016/j.jpain.2021.09.005
Royce, G. J. (1983). Cortical neurons with collateral projections to both the caudate nucleus and the centromedian-parafascicular thalamic complex: A fluorescent retrograde double labeling study in the cat. Exp. Brain Res. 50, 157–165. doi: 10.1007/BF00239179
Roychowdhury, S. M., and Fields, H. L. (1996). Endogenous opioids acting at a medullary mu-opioid receptor contribute to the behavioral antinociception produced by GABA antagonism in the midbrain periaqueductal gray. Neuroscience 74, 863–872. doi: 10.1016/0306-4522(96)00180-7
Royer, S., Zemelman, B. V., Barbic, M., Losonczy, A., Buzsáki, G., and Magee, J. C. (2010). Multi-array silicon probes with integrated optical fibers: Light-assisted perturbation and recording of local neural circuits in the behaving animal. Eur. J. Neurosci. 31, 2279–2291. doi: 10.1111/j.1460-9568.2010.07250.x
Rozeske, R. R., Jercog, D., Karalis, N., Chaudun, F., Khoder, S., Girard, D., et al. (2018). Prefrontal-periaqueductal gray-projecting neurons mediate context fear discrimination. Neuron 97, 898–910.e6. doi: 10.1016/j.neuron.2017.12.044
Rudd, R. A., Aleshire, N., Zibbell, J. E., and Gladden, R. M. (2016). Increases in drug and opioid overdose deaths–United States, 2000-2014. Morb. Mortal. Wkly. Rep. 64, 1378–1382. doi: 10.15585/mmwr.mm6450a3
Sá, K. N., Moreira, L., Baptista, A. F., Yeng, L. T., Teixeira, M. J., Galhardoni, R., et al. (2019). Prevalence of chronic pain in developing countries: Systematic review and meta-analysis. Pain Rep. 4:e779. doi: 10.1097/PR9.0000000000000779
Salas, R., Ramirez, K., Vanegas, H., and Vazquez, E. (2016). Activity correlations between on-like and off-like cells of the rostral ventromedial medulla and simultaneously recorded wide-dynamic-range neurons of the spinal dorsal horn in rats. Brain Res. 1652, 103–110. doi: 10.1016/J.BRAINRES.2016.10.001
Samineni, V. K., Grajales-Reyes, J. G., Copits, B. A., O’Brien, D. E., Trigg, S. L., Gomez, A. M., et al. (2017). Divergent modulation of nociception by glutamatergic and GABAergic neuronal subpopulations in the periaqueductal gray. eNeuro 4. doi: 10.1523/ENEURO.0129-16.2017
Sankarasubramanian, V., Chiravuri, S., Mirzakhalili, E., Anaya, C. J., Scott, J. R., Brummett, C. M., et al. (2021). Quantitative sensory testing of spinal cord and dorsal root ganglion stimulation in chronic pain patients. Neuromodulation 24, 672–684. doi: 10.1111/NER.13329
Sankarasubramanian, V., Harte, S. E., Chiravuri, S., Harris, R. E., Brummett, C. M., Patil, P. G., et al. (2019). Objective measures to characterize the physiological effects of spinal cord stimulation in neuropathic pain: A literature review. Neuromodulation 22, 227–248. doi: 10.1111/ner.12804
Sato, K. L., King, E. W., Johanek, L. M., and Sluka, K. A. (2013). Spinal cord stimulation reduces hypersensitivity through activation of opioid receptors in a frequency-dependent manner. Eur. J. Pain 17, 551–561. doi: 10.1002/J.1532-2149.2012.00220.X
Scholl, L., Seth, P., Kariisa, M., Wilson, N., and Baldwin, G. (2019). Drug and opioid-involved overdose deaths–United States, 2013-2017. Morb. Mort. Wkly. Rep. 67, 1419–1427. doi: 10.15585/mmwr.mm675152e1
Scott, D. J., Stohler, C. S., Egnatuk, C. M., Wang, H., Koeppe, R. A., and Zubieta, J. K. (2008). Placebo and nocebo effects are defined by opposite opioid and dopaminergic responses. Arch. Gen. Psychiatry 65, 220–231. doi: 10.1001/archgenpsychiatry.2007.34
Sdrulla, A. D., Guan, Y., and Raja, S. N. (2018). Spinal cord stimulation: Clinical efficacy and potential mechanisms. Pain Pract. 18, 1048–1067. doi: 10.1111/papr.12692
Sears, N. C., MacHado, A. G., Nagel, S. J., Deogaonkar, M., Stanton-Hicks, M., Rezai, A. R., et al. (2011). Long-term outcomes of spinal cord stimulation with paddle leads in the treatment of complex regional pain syndrome and failed back surgery syndrome. Neuromodulation 14, 312–318. doi: 10.1111/J.1525-1403.2011.00372.X
Seminowicz, D. A., Laferriere, A. L., Millecamps, M., Yu, J. S. C., Coderre, T. J., and Bushnell, M. C. (2009). MRI structural brain changes associated with sensory and emotional function in a rat model of long-term neuropathic pain. Neuroimage 47, 1007–1014. doi: 10.1016/j.neuroimage.2009.05.068
Seybold, V., and Elde, R. (1980). Immunohistochemical studies of peptidergic neurons in the dorsal horn of the spinal cord. J. Histochem. Cytochem. 28, 367–370. doi: 10.1177/28.4.6154731
Shao, F. B., Fang, J. F., Wang, S. S., Qiu, M. T., Xi, D. N., Jin, X. M., et al. (2021). Anxiolytic effect of GABAergic neurons in the anterior cingulate cortex in a rat model of chronic inflammatory pain. Mol. Brain 14:139. doi: 10.1186/S13041-021-00849-9
Shao, R., Lau, W. K. W., Leung, M. K., and Lee, T. M. C. (2018). Subgenual anterior cingulate-insula resting-state connectivity as a neural correlate to trait and state stress resilience. Brain Cogn. 124, 73–81. doi: 10.1016/j.bandc.2018.05.001
Sharma, K. K., Kelly, E. A., Pfeifer, C. W., and Fudge, J. L. (2020). Translating fear circuitry: Amygdala projections to subgenual and perigenual anterior cingulate in the macaque. Cereb. Cortex 30, 550–562. doi: 10.1093/cercor/bhz106
Shealy, C. N., Mortimer, J. T., and Reswick, J. B. (1967). Electrical inhibition of pain by stimulation of the dorsal columns: Preliminary clinical report. Anesth. Analg. 46, 489–491.
Shi, W., Xue, M., Wu, F., Fan, K., Chen, Q. Y., Xu, F., et al. (2022). Whole-brain mapping of efferent projections of the anterior cingulate cortex in adult male mice. Mol. Pain 18, 1–12. doi: 10.1177/17448069221094529
Shirvalkar, P., Sellers, K. K., Schmitgen, A., Prosky, J., Joseph, I., Starr, P. A., et al. (2020). A deep brain stimulation trial period for treating chronic pain. J. Clin. Med. 9, 1–15. doi: 10.3390/jcm9103155
Shyu, B. C., Lin, C. Y., Sun, J. J., Chen, S. L., and Chang, C. (2004). Bold response to direct thalamic stimulation reveals a functional connection between the medial thalamus and the anterior cingulate cortex in the rat. Magn. Reson. Med. 52, 47–55. doi: 10.1002/mrm.20111
Sikes, R. W., and Vogt, B. A. (1992). Nociceptive neurons in area 24 of rabbit cingulate cortex. J. Neurophysiol. 68, 1720–1732. doi: 10.1152/JN.1992.68.5.1720
Sims-Williams, H., Matthews, J. C., Talbot, P. S., Love-Jones, S., Brooks, J. C., Patel, N. K., et al. (2017). Deep brain stimulation of the periaqueductal gray releases endogenous opioids in humans. Neuroimage 146, 833–842. doi: 10.1016/j.neuroimage.2016.08.038
Singh, A., Patel, D., Li, A., Hu, L., Zhang, Q., Liu, Y., et al. (2020). Mapping cortical integration of sensory and affective pain pathways. Curr. Biol. 30, 1703–1715.e5. doi: 10.1016/j.cub.2020.02.091
Sivanesan, E., Maher, D. P., Raja, S. N., Linderoth, B., and Guan, Y. (2019). Supraspinal mechanisms of spinal cord stimulation for modulation of pain: Five decades of research and prospects for the future. Anesthesiology 130, 651–665. doi: 10.1097/ALN.0000000000002353
Song, Z., Meyerson, B. A., and Linderoth, B. (2011). Spinal 5-HT receptors that contribute to the pain-relieving effects of spinal cord stimulation in a rat model of neuropathy. Pain 152, 1666–1673. doi: 10.1016/J.PAIN.2011.03.012
Song, Z., Ultenius, C., Meyerson, B. A., and Linderoth, B. (2009). Pain relief by spinal cord stimulation involves serotonergic mechanisms: An experimental study in a rat model of mononeuropathy. Pain 147, 241–248. doi: 10.1016/J.PAIN.2009.09.020
Speer, A. M., Kimbrell, T. A., Wassermann, E. M., Repella, J. D., Willis, M. W., Herscovitch, P., et al. (2000). Opposite effects of high and low frequency rTMS on regional brain activity in depressed patients. Biol. Psychiatry 48, 1133–1141. doi: 10.1016/S0006-3223(00)01065-9
Spix, T. A., Nanivadekar, S., Toong, N., Kaplow, I. M., Isett, B. R., Goksen, Y., et al. (2021). Population-specific neuromodulation prolongs therapeutic benefits of deep brain stimulation. Science 374, 201–206. doi: 10.1126/SCIENCE.ABI7852
Spooner, J., Yu, H., Kao, C., Sillay, K., and Konrad, P. (2007). Neuromodulation of the cingulum for neuropathic pain after spinal cord injury. Case report. J. Neurosurg. 107, 169–172. doi: 10.3171/JNS-07/07/0169
Steinmetz, N. A., Aydin, C., Lebedeva, A., Okun, M., Pachitariu, M., Bauza, M., et al. (2021). Neuropixels 2.0: A miniaturized high-density probe for stable, long-term brain recordings. Science 372:eabf4588. doi: 10.1126/science.abf4588
Struijk, J. J., Holsheimer, J., van Veen, B. K., and Boom, H. B. K. (1991). Epidural spinal cord stimulation: Calculation of field potentials with special reference to dorsal column nerve fibers. IEEE Trans. Biomed. Eng. 38, 104–110. doi: 10.1109/10.68217
Subedi, B., and Grossberg, G. T. (2011). Phantom limb pain: Mechanisms and treatment approaches. Pain Res. Treat. 2011:864605. doi: 10.1155/2011/864605
Sweeney, P., Chen, C., Rajapakse, I., and Cone, R. D. (2021). Network dynamics of hypothalamic feeding neurons. Proc. Natl. Acad. Sci. U.S.A. 118:e2011140118. doi: 10.1073/PNAS.2011140118/SUPPL_FILE/PNAS.2011140118.SM02.MP4
Tang, J., Ko, S., Ding, H.-K., Qiu, C.-S., Calejesan, A. A., and Zhuo, M. (2005). Pavlovian fear memory induced by activation in the anterior cingulate cortex. Mol. Pain 1:6. doi: 10.1186/1744-8069-1-6
Tass, P. A. (2003). A model of desynchronizing deep brain stimulation with a demand-controlled coordinated reset of neural subpopulations. Biol. Cybern. 89, 81–88. doi: 10.1007/S00422-003-0425-7
Taylor, J. J., Borckardt, J. J., and George, M. S. (2012). Endogenous opioids mediate left dorsolateral prefrontal cortex rTMS-induced analgesia. Pain 153, 1219–1225. doi: 10.1016/j.pain.2012.02.030
Taylor, R. S., Desai, M. J., Rigoard, P., and Taylor, R. J. (2014). Predictors of pain relief following spinal cord stimulation in chronic back and leg pain and failed back surgery syndrome: A systematic review and meta-regression analysis. Pain Pract. 14:489. doi: 10.1111/PAPR.12095
Thoden, U., Doerr, M., Dieckmann, G., and Krainick, J.-U. (1979). Medial thalamic permanent electrodes for pain control in man: An electrophysiological and clinical study. Electroencephalogr. Clin. Neurophysiol. 47, 582–591. doi: 10.1016/0013-4694(79)90259-1
Thompson, S. J., Pitcher, M. H., Stone, L. S., Tarum, F., Niu, G., Chen, X., et al. (2018). Chronic neuropathic pain reduces opioid receptor availability with associated anhedonia in rat. Pain 159, 1856–1866. doi: 10.1097/j.pain.0000000000001282
Toll, L., Berzetei-Gurske, I. P., Polgar, W. E., Brandt, S. R., Adapa, I. D., Rodriguez, L., et al. (1998). Standard binding and functional assays related to medications development division testing for potential cocaine and opiate narcotic treatment medications. NIDA Res. Monogr. 178, 440–466.
Tölle, T. R., Kaufmann, T., Siessmeier, T., Lautenbacher, S., Berthele, A., Munz, F., et al. (1999). Region-specific encoding of sensory and affective components of pain in the human brain: A positron emission tomography correlation analysis. Ann. Neurol. 45, 40–47. doi: 10.1002/1531-8249
Trevathan, J. K., Asp, A. J., Nicolai, E. N., Trevathan, J. M., Kremer, N. A., Kozai, T. D. Y., et al. (2021). Calcium imaging in freely moving mice during electrical stimulation of deep brain structures. J. Neural Eng. 18:026008. doi: 10.1088/1741-2552/ABB7A4
Trieu, B. H., Remmers, B. C., Toddes, C., Brandner, D. D., Lefevre, E. M., Kocharian, A., et al. (2022). Angiotensin-converting enzyme gates brain circuit–specific plasticity via an endogenous opioid. Science 375, 1177–1182. doi: 10.1126/science.abl5130
Umana, I. C., Daniele, C. A., Miller, B. A., Abburi, C., Gallagher, K., Brown, M. A., et al. (2017). Nicotinic modulation of descending pain control circuitry. Pain 158, 1938–1950. doi: 10.1097/j.pain.0000000000000993
Vallejo, R., Kelley, C. A., Gupta, A., Smith, W. J., Vallejo, A., and Cedeño, D. L. (2020). Modulation of neuroglial interactions using differential target multiplexed spinal cord stimulation in an animal model of neuropathic pain. Mol. Pain 16:1744806920918057. doi: 10.1177/1744806920918057
Valmunen, T., Pertovaara, A., Taiminen, T., Virtanen, A., Parkkola, R., and Jääskeläinen, S. K. (2009). Modulation of facial sensitivity by navigated rTMS in healthy subjects. Pain 142, 149–158. doi: 10.1016/j.pain.2008.12.031
van Bockstaele, E. J., Commons, K., and Pickel, V. M. (1997). δ-Opioid receptor is present in presynaptic axon terminals in the rat nucleus locus coeruleus: Relationships with methionine5-enkephalin. J. Comp. Neurol. 388, 575–586. doi: 10.1002/(sici)1096-9861(19971201)388:4<575::aid-cne6<3.0.co;2-#
van Tubergen, A., Debats, I., Ryser, L., Londoño, J., Burgos-Vargas, R., Cardiel, M. H., et al. (2002). Use of a numerical rating scale as an answer modality in ankylosing spondylitis-specific questionnaires. Arthritis Rheum. 47, 242–248. doi: 10.1002/ART.10397
Vasquez, E., and Vanegas, H. (2000). The antinociceptive effect of PAG-microinjected dipyrone in rats is mediated by endogenous opioids of the rostral ventromedical medulla. Brain Res. 854, 249–252. doi: 10.1016/S0006-8993(99)02303-3
Viisanen, H., and Pertovaara, A. (2010). Antinociception by motor cortex stimulation in the neuropathic rat: Does the locus coeruleus play a role? Exp. Brain Res. 201, 283–296. doi: 10.1007/S00221-009-2038-4
Vogt, B. A. (2005). Pain and emotion interactions in subregions of the cingulate gyrus. Nat. Rev. Neurosci. 6, 533–544. doi: 10.1038/nrn1704
Vogt, B. A., Derbyshire’, S., and Jones’, A. K. P. (1996). Pain processing in four regions of human cingulate cortex localized with co-registered PET and MR imaging. Eur. J. Neurosci. 8, 1461–1473. doi: 10.1111/j.1460-9568.1996.tb01608.x
Vogt, B. A., Watanabe, H., Grootoonk, S., and Jones, A. K. P. (1995). Topography of diprenorphine binding in human cingulate gyrus and adjacent cortex derived from coregistered PET and MR images. Hum. Brain Mapp. 3, 1–12.
von Monakow, K. H., Akert, K., and Künzle, H. (1979). Projections of precentral and premotor cortex to the red nucleus and other midbrain areas in Macaca fascicularis. Exp. Brain Res. 34, 91–105. doi: 10.1007/BF00238343
von Zastrow, M., Svingos, A., Haberstock-Debic, H., and Evans, C. (2003). Regulated endocytosis of opioid receptors: Cellular mechanisms and proposed roles in physiological adaptation to opiate drugs. Curr. Opin. Neurobiol. 13, 348–353. doi: 10.1016/S0959-4388(03)00069-2
Wager, T. D., Rilling, J. K., Smith, E. E., Sokolik, A., Casey, K. L., Davidson, R. J., et al. (2004). Placebo-induced changes in fMRI in the anticipation and experience of pain. Science 303, 1162–1167. doi: 10.1126/science.1093065
Wager, T. D., Scott, D. J., and Zubieta, J. K. (2007). Placebo effects on human μ-opioid activity during pain. Proc. Natl. Acad. Sci. U.S.A. 104, 11056–11061. doi: 10.1073/pnas.0702413104
Wall, P. D., and Swert, W. H. (1967). Temporary abolition of pain in man. Science 155, 108–109. doi: 10.1126/SCIENCE.155.3758.108
Wang, H., and Wessendorf, M. W. (1999). μ- and δ-opioid receptor mRNAs are expressed in spinally projecting serotonergic and nonserotonergic neurons of the rostral ventromedial medulla. J. Comp. Neurol. 404, 183–196. doi: 10.1002/(sici)1096-9861(19990208)404:2<183::aid-cne4>3.0.co;2-n
Wang, H., and Wessendorf, M. W. (2002). μ- and δ-opioid receptor mRNAs are expressed in periaqueductal gray neurons projecting to the rostral ventromedial medulla. Neuroscience 109, 619–634. doi: 10.1016/S0306-4522(01)00328-1
Wang, J. B., Aryal, M., Zhong, Q., Vyas, D. B., and Airan, R. D. (2018). Noninvasive ultrasonic drug uncaging maps whole-brain functional networks. Neuron 100, 728–738.e7. doi: 10.1016/J.NEURON.2018.10.042
Wang, J. Y., Zhao, M., Yuan, Y. K., Fan, G. X., Jia, H., and Tang, J.-S. (2006). The roles of different subtypes of opioid receptors in mediating the nucleus submedius opioid-evoked antiallodynia in a neuropathic pain model of rats. Neuroscience 138, 1319–1327. doi: 10.1016/j.neuroscience.2005.11.071
Wang, N., Zhang, T., Su, Y.-L., Wang, J.-Y., and Luo, F. (2016). Differential modulation of electrical stimulation of periaqueductal gray and thalamus on nociceptive behaviors of rats. Sheng Li Xue Bao 68, 115–125.
Wang, J., Nebeck, S., Muralidharan, A., Johnson, M. D., Vitek, J. L., and Baker, K. B. (2016). Coordinated reset deep brain stimulation of subthalamic nucleus produces long-lasting, dose-dependent motor improvements in the 1-Methyl-4-phenyl-1,2,3,6-tetrahydropyridine Non-human primate model of parkinsonism. Brain Stimul. 9, 609–617. doi: 10.1016/J.BRS.2016.03.014
Wayne Hurt, R., Thomas Ballantine, H., THOl, H., and Ballantine, I. (1974). Stereotactic anterior cingulate lesions for persistent pain: A report on 68 cases. Neurosurgery 21, 334–351. doi: 10.1093/NEUROSURGERY/21.CN_SUPPL_1.334
Weiwei, Y., Wendi, F., Mengru, C., Tuo, Y., and Chen, G. (2021). The cellular mechanism by which the rostral ventromedial medulla acts on the spinal cord during chronic pain. Rev. Neurosci. 32, 545–558. doi: 10.1515/REVNEURO-2020-0121
West, W. L., Yeomans, D. C., and Proudfit, H. K. (1993). The function of noradrenergic neurons in mediating antinociception induced by electrical stimulation of the locus coeruleus in two different sources of Sprague-Dawley rats. Brain Res. 626, 127–135. doi: 10.1016/0006-8993(93)90571-4
Westlund, K. N., Bowker, R. M., Ziegler, M. G., and Coulter, J. D. (1983). Noradrenergic projections to the spinal cord of the rat. Brain Res. 263, 15–31. doi: 10.1016/0006-8993(83)91196-4
Westlund, K. N., Bowker, R. M., Ziegler, M. G., and Coulter, J. D. (1984). Origins and terminations of descending noradrenergic projections to the spinal cord of monkey. Brain Res. 292, 1–16. doi: 10.1016/0006-8993(84)90884-9
Whitt, J. L., Masri, R., Pulimood, N. S., and Keller, A. (2013). Pathological activity in mediodorsal thalamus of rats with spinal cord injury pain. J. Neurosci. 33:3915. doi: 10.1523/JNEUROSCI.2639-12.2013
Wiech, K., Jbabdi, S., Lin, C. S., Andersson, J., and Tracey, I. (2014). Differential structural and resting state connectivity between insular subdivisions and other pain-related brain regions. Pain 155, 2047–2055. doi: 10.1016/j.pain.2014.07.009
Williams, J. T., and North, R. A. (1984). Opiate-receptor interactions on single locus coeruleus neurones. Mol. Pharmacol. 26, 489–497.
Williams, J. T., and Zieglgänsberger, W. (1981). Neurons in the frontal cortex of the rat carry multiple opiate receptors. Brain Res. 226, 304–308. doi: 10.1016/0006-8993(81)91103-3
Williams, J. T., Egan, T. M., and North, R. A. (1982). Enkephalin opens potassium channels on mammalian central neurones. Nature 299, 74–77.
Willoch, F., Tölle, T. R., Wester, H. J., Munz, F., Petzold, A., Schwaiger, M., et al. (1999). Central pain after pontine infarction is associated with changes in opioid receptor binding: A pet study with 11 C-diprenorphine. AJNR Am. J. Neuroradiol. 20, 686–690.
Witting, N., Kuper, R. C., Svensson, D. D. S., Arendt-Nielsen, L., Gjedde, A., and Jensen, T. S. (2001). Experimental brush-evoked allodynia activates posterior parietal cortex. Neurology 57, 1817–1824.
Wrigley, P. J., Gustin, S. M., McIndoe, L. N., Chakiath, R. J., Henderson, L. A., and Siddall, P. J. (2013). Longstanding neuropathic pain after spinal cord injury is refractory to transcranial direct current stimulation: A randomized controlled trial. Pain 154, 2178–2184. doi: 10.1016/J.PAIN.2013.06.045
Xiao, X., and Zhang, Y. Q. (2018). A new perspective on the anterior cingulate cortex and affective pain. Neurosci. Biobehav. Rev. 90, 200–211. doi: 10.1016/J.NEUBIOREV.2018.03.022
Yaksh, T. L. (1979). Direct evidence that spinal serotonin and noradrenaline terminals mediate the spinal antinociceptive effects of morphine in the periaqueductal gray. Brain Res. 160, 180–185. doi: 10.1016/0006-8993(79)90616-4
Yaksh, T. L., Huang, S. P., Rudy, T. A., and Frederickson, R. C. A. (1977). The direct and specific opiate-like effect of Met5-enkephalin and analogues on the spinal cord. Neuroscience 2, 593–596. doi: 10.1016/0306-4522(77)90055-0
Yang, S., and Chang, M. C. (2020). Effect of repetitive transcranial magnetic stimulation on pain management: A systematic narrative review. Front. Neurol. 11:114. doi: 10.3389/fneur.2020.00114
Yarnitsky, D., Arendt-Nielsen, L., Bouhassira, D., Edwards, R. R., Fillingim, R. B., Granot, M., et al. (2010). Recommendations on terminology and practice of psychophysical DNIC testing. Eur. J. Pain 14, 339–339. doi: 10.1016/j.ejpain.2010.02.004
Yong, R. J., Mullins, P. M., and Bhattacharyya, N. (2022). Prevalence of chronic pain among adults in the United States. Pain 163, E328–E332. doi: 10.1097/J.PAIN.0000000000002291
Yoo, W.-K., Kim, Y.-H., Doh, W.-S., Lee, J.-H., Jung, K.-I., Park, D.-S., et al. (2006). Dissociable modulating effect of repetitive transcranial magnetic stimulation on sensory and pain perception. Neuroreport 17, 141–144. doi: 10.1097/01.wnr.0000198438.37012.d6
Young, R. F., and Chambi, V. I. (1987). Pain relief by electrical stimulation of the periaqueductal and periventricular gray matter. Evidence for a non-opioid mechanism. J. Neurosurg. 66, 364–371. doi: 10.3171/jns.1987.66.3.0364
Young, R. F., Kroening, R., Fulton, W., Feldman, R. A., and Chambi, I. (1985). Electrical stimulation of the brain in treatment of chronic pain. Experience over 5 years. J. Neurosurg. 62, 389–396. doi: 10.3171/JNS.1985.62.3.0389
Yu, H., Xiang, X., Chen, Z., Wang, X., Dai, J., Wang, X., et al. (2021). Periaqueductal gray neurons encode the sequential motor program in hunting behavior of mice. Nat. Commun. 12, 1–15. doi: 10.1038/S41467-021-26852-1
Zhang, T. C., Janik, J. J., and Grill, W. M. (2014). Mechanisms and models of spinal cord stimulation for the treatment of neuropathic pain. Brain Res. 1569, 19–31. doi: 10.1016/j.brainres.2014.04.039
Zhang, Y., Zhao, S., Rodriguez, E., Takatoh, J., Han, B. X., Zhou, X., et al. (2015). Identifying local and descending inputs for primary sensory neurons. J. Clin. Invest. 125, 3782–3794. doi: 10.1172/JCI81156
Zhang, Y. Q., Tang, J. S., Yuan, B., and Jia, H. (1995). Effects of thalamic nucleus submedius lesions on the tail flick reflex inhibition evoked by hindlimb electrical stimulation in the rat. Neuroreport 6, 1237–1240. doi: 10.1097/00001756-199506090-00002
Zhao, Z. Q. (2008). Neural mechanism underlying acupuncture analgesia. Prog. Neurobiol. 85, 355–375. doi: 10.1016/j.pneurobio.2008.05.004
Zhou, H., Martinez, E., Lin, H. H., Yang, R., Dale, J. A., Liu, K., et al. (2018). Inhibition of the prefrontal projection to the nucleus accumbens enhances pain sensitivity and affect. Front. Cell. Neurosci. 12:240. doi: 10.3389/fncel.2018.00240
Zhu, X., Xu, Y., Shen, Z., Zhang, H., Xiao, S., Zhu, Y., et al. (2021). Rostral anterior cingulate cortex–ventrolateral periaqueductal gray circuit underlies electroacupuncture to alleviate hyperalgesia but not anxiety-like behaviors in mice with spared nerve injury. Front. Neurosci. 15:757628. doi: 10.3389/FNINS.2021.757628
Zubieta, J. K., Bueller, J. A., Jackson, L. R., Scott, D. J., Xu, Y., Koeppe, R. A., et al. (2005). Placebo effects mediated by endogenous opioid activity on μ-opioid receptors. J. Neurosci. 25, 7754–7762. doi: 10.1523/JNEUROSCI.0439-05.2005
Keywords: pain, analgesia, opioid, μ-opioid receptor, neurostimulation, neuromodulation, deep brain stimulation (DBS), spinal cord stimulation (SCS)
Citation: Lubejko ST, Graham RD, Livrizzi G, Schaefer R, Banghart MR and Creed MC (2022) The role of endogenous opioid neuropeptides in neurostimulation-driven analgesia. Front. Syst. Neurosci. 16:1044686. doi: 10.3389/fnsys.2022.1044686
Received: 14 September 2022; Accepted: 18 November 2022;
Published: 14 December 2022.
Edited by:
Gregory Corder, University of Pennsylvania, United StatesReviewed by:
Haocheng Zhou, Central South University, ChinaCopyright © 2022 Lubejko, Graham, Livrizzi, Schaefer, Banghart and Creed. This is an open-access article distributed under the terms of the Creative Commons Attribution License (CC BY). The use, distribution or reproduction in other forums is permitted, provided the original author(s) and the copyright owner(s) are credited and that the original publication in this journal is cited, in accordance with accepted academic practice. No use, distribution or reproduction is permitted which does not comply with these terms.
*Correspondence: Matthew R. Banghart, bWJhbmdoYXJ0QHVjc2QuZWR1; Meaghan C. Creed, bWVhZ2hhbi5jcmVlZEB3dXN0bC5lZHU=
†These authors have contributed equally to this work
Disclaimer: All claims expressed in this article are solely those of the authors and do not necessarily represent those of their affiliated organizations, or those of the publisher, the editors and the reviewers. Any product that may be evaluated in this article or claim that may be made by its manufacturer is not guaranteed or endorsed by the publisher.
Research integrity at Frontiers
Learn more about the work of our research integrity team to safeguard the quality of each article we publish.