- Área de Psicobiología, Universitat Jaume I, Castellón de la Plana, Spain
The traditional cerebellum’s role has been linked to the high computational demands for sensorimotor control. However, several findings have pointed to its involvement in executive and emotional functions in the last decades. First in 2009 and then, in 2016, we raised why we should consider the cerebellum when thinking about drug addiction. A decade later, mounting evidence strongly suggests the cerebellar involvement in this disorder. Nevertheless, direct evidence is still partial and related mainly to drug-induced reward memory, but recent results about cerebellar functions may provide new insights into its role in addiction. The present review does not intend to be a compelling revision on available findings, as we did in the two previous reviews. This minireview focuses on specific findings of the cerebellum’s role in drug-related reward memories and the way ahead for future research. The results discussed here provide grounds for involving the cerebellar cortex’s apical region in regulating behavior driven by drug-cue associations. They also suggest that the cerebellar cortex dysfunction may facilitate drug-induced learning by increasing glutamatergic output from the deep cerebellar nucleus (DCN) to the ventral tegmental area (VTA) and neural activity in its projecting areas.
Introduction
It is remarkable that during evolution there has been a coordinated scaling in the number of cortical and cerebellar neurons across species (Herculano-Houzel, 2010). However, in terms of the number of neurons contained in the brain mass, the cerebellum is the winner with 80% of neurons, the majority granular cells, packed in 10% of brain volume (Azevedo et al., 2009). It is still uncertain why so many neurons are needed for cerebellar functions as silencing 75% of granule cells does not impact motor adaptation and performance (Galliano et al., 2013). It has been suggested as one possible explanation that the huge number of granule cells enable to make sparse coding available to increase memory storage capacity in the cerebellum (Schweighofer et al., 2001). In evolutionary terms, it would have allowed great apes to improve the learning of motor skills, tool-making and in the end, verbal communication (Barton, 2012).
It is now clear that reciprocal connectivity between the cerebellum and the rest of the brain is crucial to understanding cerebellar function (Galliano and De Zeeuw, 2014; Lackey et al., 2018). A key principle of the anatomical organization in the cerebellum is the segmentation of inputs and outputs in several longitudinal oriented modules (Figure 1). Each module includes descending and ascending afferents from subdivisions in the pontine nuclei and inferior olive, respectively. These inputs reach specific areas in the cerebellar cortex as well as regions of deep cerebellar and vestibular nuclei to which Purkinje cells project their axons (see for a recent review, Watson and Apps, 2019). Cerebellar modules integrate information from differentiated brain areas with incoming peripheral signals (Figure 1). Accordingly, sensorimotor coordination depends on the convergence at a cellular level of somatosensory and motor cortical inputs into specific regions of the cerebellar cortex that, in turn, send ascending projections to the motor cortex through differentiated thalamic areas (Proville et al., 2014). Moreover, the functional loop formed by the anterior lateral motor cortex and fastigial nucleus in the cerebellum is required to maintain the representation of the information in the frontal cortex during motor planning, as Gao et al. (2018) recently demonstrated.
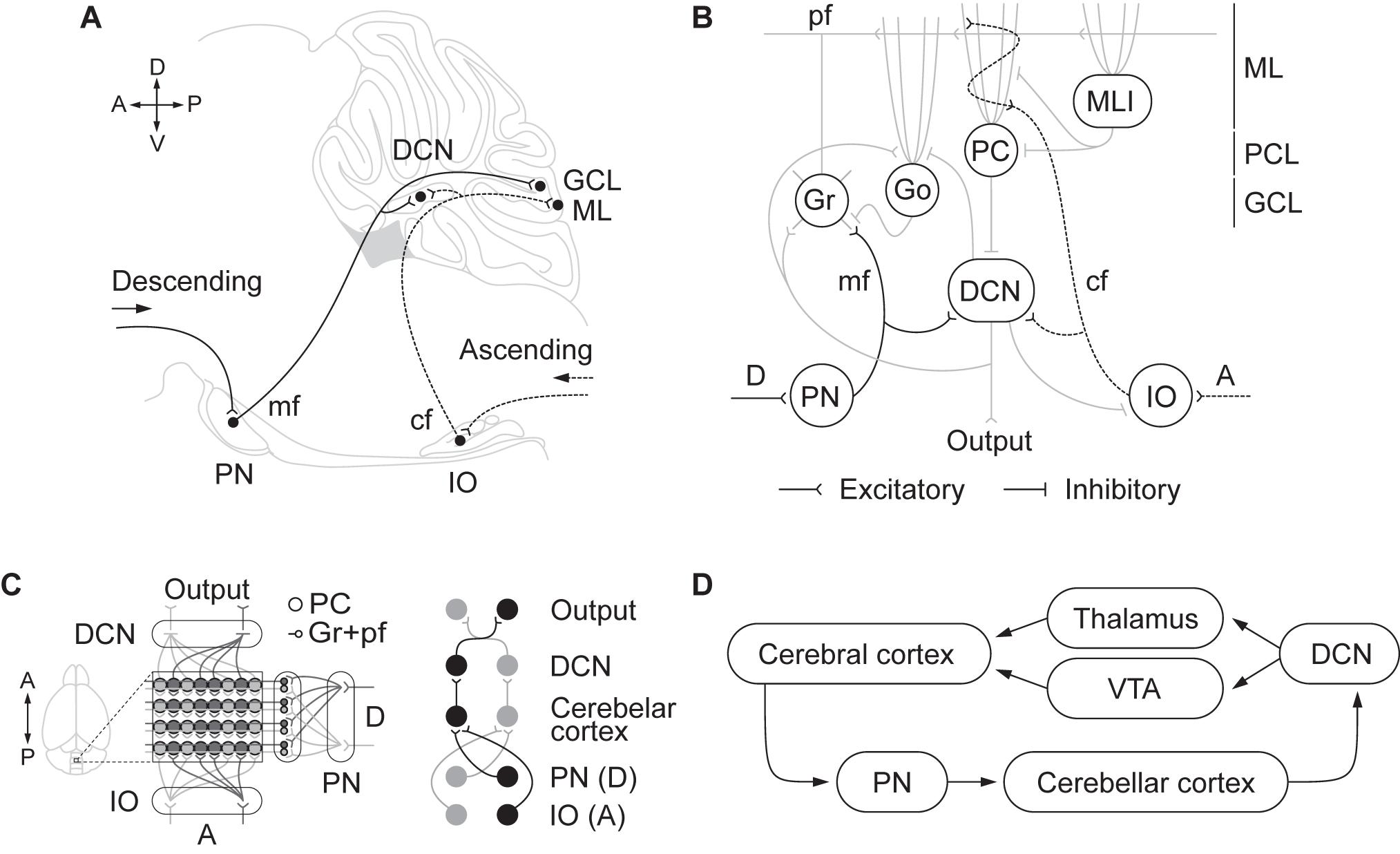
Figure 1. (A) Descending and ascending afferents to the cerebellum, cerebro-pontinocerebellar and spino-olivocerebellar pathways, respectively. Descending cortical efferents arrive to the pontine nuclei (PN) that provides mossy fiber (mf) projections to the cerebellar cortex ending in the granule cell layer (GCL). The ascending pathway from to the inferior olive (IO) projects climbing fibers (cf) to the cerebellar cortex contacting Purkinje cell (PC) dendrites in the molecular layer (ML). Both pathways leave collaterals in the deep cerebellar nuclei (DCN) on their direction to the cerebellar cortex. (B) Schematic representation of the cerebellar circuitry. The descending pathway through mf contacts granule (Gr) and Golgi (Go) cells in the GCL and leaves collaterals in the DCN. Gr send their ascending axon to the ML that bifurcates forming the parallel fibers (pf). In the ML, pf make contact with the dendrites of Go, PC, and molecular layer interneurons (MLI). The ascending pathway through cf contacts PC dendrites. PC sends inhibitory projections to the DCN that only sends output signal when PC are inhibited by MLI. DCN not only send the main cerebellar output but also send inhibitory feedback to IO and Go, and excitatory feedback to Gr and Go. The cerebellar circuit is organized as a feedforward excitatory network with inhibitory loops. (C) Modular organization of the cerebellum. The anatomical organization of the cerebellum is distributed in longitudinal modules, where PC are organized perpendicular to the cortical folds. Moreover, differential microzones can be observed forming striped zones of PC. Each module is organized by parasagittal bands of PC and the cf emerging from the contralateral IO that contact them. Mf projecting to a certain group of PC through the GCL also contact with the same DCN those PC project to. In that manner there is somatotopy between the deseeding and ascending pathways, the PN and IO regions, the cerebellar cortical zones where mf and cf terminate in, and the specific DCN region where PC project to. (D) Cortico-cerebellar loops.
Beyond the well-accepted role of the cerebellum in the high computational demands for sensorimotor control, several findings have pointed to its involvement in executive and emotional functions in the last decades (Schmahmann and Sherman, 1998; Blackwood et al., 2004; Bastian, 2006; Callu et al., 2007; Cardoso et al., 2014; Nguyen et al., 2017; Deverett et al., 2019; Hull, 2020). Direct and indirect reciprocal connectivity between the cerebellum and other brain regions as the amygdala, basal ganglia, and mPFC can explain cerebellar roles in fear memory, cognitive flexibility, behavioral control, and goal-directed behavior (Sacchetti et al., 2002; Bostan et al., 2013; Wagner et al., 2017; Xiao et al., 2018; Schmahmann, 2019). Likewise, it can also explain why cerebellar dysfunction is associated with neuropsychiatric disorders in which impairment of behavioral and cognitive inhibitory control is a central part of the disease pattern as to happen in autism, obsessive-compulsive (OCD), attention deficit/hyperactivity (ADHD) and addiction (Miquel et al., 2019; Figure 2).
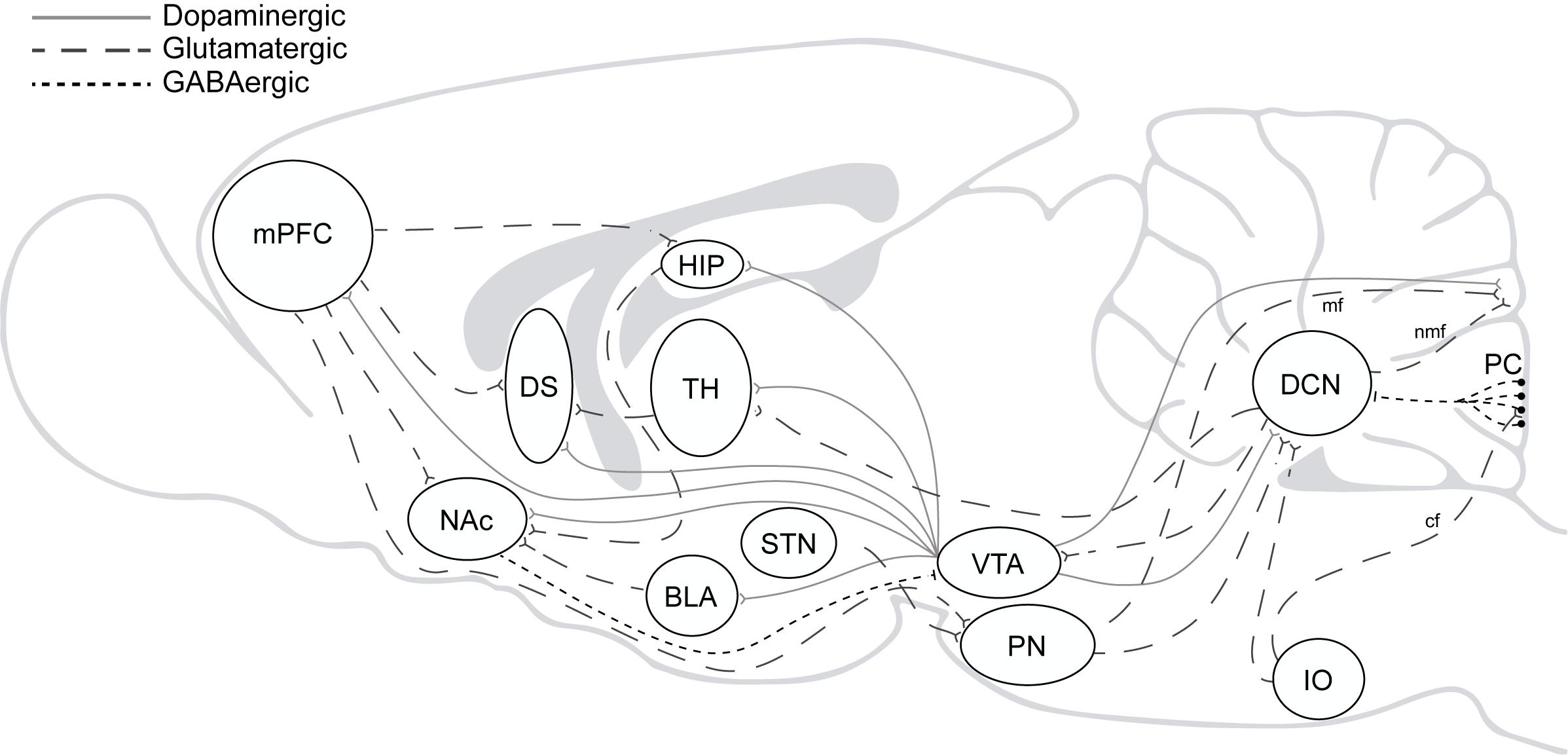
Figure 2. The addiction circuitry. mPFC, medial prefrontal cortex; DS, dorsal striatum; NAc, nucleus accumbens; HIP, hippocampus; BLA, basolateral amygdala; TH, thalamus; STN, subthalamic nucleus; VTA, ventral tegmental area; PN, pontine nucleus; IO, inferior olive; DCN, deep cerebellar nucleus; PC, purkinje cells; mf, mossy fibers; nmf, nuclear mossy fibers; cf, climbing fibers. Direct and indirect reciprocal connectivity between the cerebellum and other brain regions as the VTA, amygdala, basal ganglia, and mPFC.
Accordingly, in 2009 and then in 2016, we raised the question to why we should consider the cerebellum when thinking about drug addiction and drew attention to the cerebellum’s roles in several of the brain functions affected in drug addicts. A decade later, mounting evidence strongly suggest the cerebellar involvement in this disorder. It is worth noting that the cerebellum connects bidirectionally to functional loops involved in drug addiction. In particular, the cerebellum modulates cortical influences on striatal activity (Chen et al., 2014; Watson et al., 2014) and exerts an indirect (Forster and Blaha, 2003; Rogers et al., 2011) but also a direct control over VTA dopaminergic neurons (Watabe-Uchida et al., 2012; Carta et al., 2019; Gil-Miravet et al., 2019b; Figure 2). Nevertheless, evidence is still partial and related mainly to drug-induced reward memory, but recent results may provide with a wider perspective of cerebellar roles in drug addiction. For the purpose of this minireview, we will focus on specific findings about the role of the cerebellum in cocaine-induced learning, suggesting the way forward for future research.
A Brief Historical View of Addictive Drug Effects on Cerebellar Function
First evidences of drug effects on the cerebellum came from the toxicology of alcohol and cannabis. For many years, it was thought that the main role of the cerebellum was limited to tolerance to motor incoordination and some of the withdrawal symptoms induced by drug misuse (Killicorn, 1955; Decker et al., 1959; Allsop and Turner, 1966; Ho et al., 1972; Dewey et al., 1973; Vivian et al., 1973; Rogers et al., 1980; Liljequist and Tabakoff, 1985; Rodríguez de Fonseca et al., 1994; Blednov et al., 2017). It is undeniable that alcohol and cannabis induce molecular changes in the cerebellum that correlate with homeostatic adaptations. Reduction of inhibitory binding sites and downstream pathways (Dewey et al., 1973; Liljequist and Tabakoff, 1985; Rodríguez de Fonseca et al., 1994; Casu et al., 2005) or decreases in cerebellar responses to these drugs (Volkow et al., 1993; Blednov et al., 2017) are both examples of homeostatic adaptations with chronic drug use. Nevertheless, drug impact on the cerebellum goes beyond homeostatic alterations and entails similar plasticity changes that have been described in the basal ganglia and mPFC to underly incentive sensitization (Couceyro et al., 1994; Klitenick et al., 1995; Bhargava and Kumar, 1999; Fumagalli et al., 2007; Palomino et al., 2014) and cocaine-induced associative memory (Carbo-Gas et al., 2014a, b, 2017).
A critical part of aberrant drug-induced plasticity in several brain regions is linked to Brain-Derived Neurotrophic Factor (BDNF) mechanisms (Li and Wolf, 2015). Indeed, relapse risk correlates with serum BDNF levels in abstinent cocaine addicts (Grimm et al., 2003; D’Sa et al., 2011; Corominas-Roso et al., 2015). Likewise, time-dependent increases in BDNF in the mPFC and VTA, but not in the nucleus accumbens (Bobadilla et al., 2019), have been associated with the incubation of craving under cocaine abstinence (Grimm et al., 2003). We have explored the effects of cocaine reinstatement on BDNF-related plasticity in the cerebellum of sensitized mice after short (Vazquez-Sanroman et al., 2015a) and protracted abstinence (Vazquez-Sanroman et al., 2015b). The direction of cerebellar plasticity after reinstatement depends entirely on the length of abstinence. Cocaine reinstatement after short abstinence (1 week) promotes transcriptional activity through the overexpression of exon VI in the cerebellar cortex that resulted in an accumulation of proBDNF to the detriment of matureBDNF isoform. Accordingly, p75NGF receptor levels also increased. ProBDNF expression enhanced in Purkinje neurons and Bergman glia, and was associated with structural changes such as pruning of Purkinje dendritic spines and a reduction in the size and density of their synaptic terminals. Moreover, GluR2 subunits of AMPA receptors in Purkinje neurons appeared internalized (Vazquez-Sanroman et al., 2015a). Therefore, shortly after drug cessation, cocaine reinstatement reduces the inhibitory tonic control of Purkinje neurons on DCN output neurons.
In contrast, cocaine reinstatement after protracted abstinence (1 month) increased mature-BDNF mechanisms in the cerebellar cortex of cocaine sensitized mice. The increase was a post-transcriptional effect plausibly fueled through the enhancement of tissue plasminogen activator (tpA) levels responsible for proBDNF cleavage (Vazquez-Sanroman et al., 2015b). In consequence, we observed higher levels of mature-BDNF isoform and TrkB receptors, as well as increased cellular BDNF expression in Purkinje neurons. BDNF-related plasticity was accompanied this time by dendritic sprouting and a larger size of synaptic boutons in Purkinje neurons. Moreover, GluR2 expression enhanced selectively in the soma and dendrites of these cells in lobules VIII and IX of the vermis, suggesting GluR2 trafficking toward the cell surface (Vazquez-Sanroman et al., 2015b). It has been shown that the mature-BDNF isoform may promote activity-dependent dendritic sprouting and axonal re-modeling in striatal-cortico-limbic neurons through TrkB receptor activation (Kafitz et al., 1999; Kovalchuk et al., 2002). Similar role has been demonstrated for BDNF and TrkB receptors in parallel fiber-Purkinje cell synapses (Lu and Figurov, 1997). Thus, although it remains to be tested, these results suggest that cocaine reinstatement after protracted abstinence would promote enhanced Purkinje inhibitory functions decreasing the cerebellar output. Altogether, these findings indicate a time-dependent regulation of molecular and structural plasticity in Purkinje neurons during withdrawal that might contribute to promote cocaine seeking and relapse.
Cerebellar Role in Cocaine-Induced Associative Memory
The central role of the cerebellum in associative motor and non-motor learning has been well established (Kim and Thompson, 1997; De Zeeuw and Yeo, 2005; Sacchetti et al., 2005). It has been shown that both electrical (Steinmetz et al., 1989) and optogenetic stimulation (Albergaria et al., 2018) of mossy fiber inputs from the pontine nuclei projecting to the granular cell layer mimic the conditioned stimulus to produce conditioned eyeblink responses. Acquisition and short-term expression of the conditioned motor response depend on the specific regions in the cerebellar cortex (Galliano et al., 2013). However, long-term consolidation seems to occur in the interposed nucleus (Carulli et al., 2020).
In drug addiction, learned reinforcing properties make drug-associated cues strong motivational triggers for drug seeking. The consolidation and persistence of these associative memories (Everitt and Robbins, 2005; Hyman et al., 2006) together with deficits in executive inhibitory control (Koob and Volkow, 2010) result in an elevated risk of relapse even after long periods of protracted abstinence. Long-lasting brain changes that underlie drug-related memories make addiction a chronic disease.
A strong support for a cerebellar role in long-lasting drug-cue associations comes from human and animal research (Miquel et al., 2009, 2016; Moulton et al., 2014; Moreno-Rius and Miquel, 2017). Neuroimaging studies of cue-reactivity have consistently shown cerebellar activations when drug-related cues are presented to drug addicts (Grant et al., 1996; Martin-Sölch et al., 2001; Schneider et al., 2001; Anderson et al., 2006; Smolka et al., 2006; Filbey et al., 2009). The cerebellar activation has been described regardless the sensory modality and the type of drug used. Several findings make unlikely cerebellar activity to be a craving correlate (Moreno-Rius and Miquel, 2017). First, overlapping patterns of cerebellar activation have been described when drug abusers are presented with food- and drug-related cues (Tomasi et al., 2015). Second, the presentation of anger-associated cues also triggers increased activity in the cerebellum (Kilts et al., 2001). Last but not least, a positive correlation between cerebellar activations and craving self-reports is far from being a consistent result (Grant et al., 1996; Bonson et al., 2002; Risinger et al., 2005).
We have been investigating the cerebellum’s contribution to cocaine-cue associative memory during the last decade (Carbo-Gas et al., 2014a, b, 2017; Gil-Miravet et al., 2019a, b). Using an animal model of cocaine-induced odor preference conditioning, we have shown that the conditioned preference for cocaine-associated cues correlates with a selective increase in neural activity (cFos expression) at the apical part of the granular cell layer in the posterior vermis (lobules 8 and 9). The increased activity was observed in granule cells mainly, and in about half of Golgi interneurons (Carbo-Gas et al., 2014a, b, 2017). It has been described that the number of activated granule cells rises as learning progresses (Giovannucci et al., 2017). It is worthy of mention that no activity changes were detected in the cerebellum of pseudo-conditioning animals or those that did not express the conditioned response. These findings rule out the possibility that the cerebellar activation results exclusively from unconditioned stimulating properties of cocaine or from movements performed during the test, since all treated groups received the same drug regimen and no differences were found in motor activity (Carbo-Gas et al., 2017). Furthermore, they suggest that granule cell activation would represent some aspects of the drug-cue associative engram or, at least, use this engram to regulate goal-directed behavior. According to the last suggestion, increased granule cell activity was not seen when mice showing preference were confined in the presence of CS+ (cocaine-related cue), but with no chance of selecting between CS+ and CS− (saline-related cue) (Carbo-Gas et al., 2017). Thus, granule cell activity did not result from the cue presentation merely. It seems to be more related to response selection driven by the reward-predictive cue. In addition, the main cerebellar task during the action selection might involve the generation of intentions to reach the goal as it has been recently suggested (Caligiore et al., 2017). In other words, the cerebellum would contribute to bias behavioral selection toward the context that predicts drug availability (Carbo-Gas et al., 2014b). The internal state (drug abstinence) might modulate these cerebellar predictions, increasing the probability of selecting the drug-associated context when the drug is absent from the body. Nevertheless, although the representation and estimation of the goal value appears to be a role of the cortico-basal ganglia loop (Houk and Wise, 1995; Doya, 2000), we cannot rule out that the cerebellar cortex encodes the reward value of the cocaine associated cue. Granule cell activity in the apical region has been demonstrated to encode reward, the expectation of reward, reward omission and the conditioned response using natural rewards (Wagner et al., 2017). In functional terms, the expected consequence of increasing activity in granule cells is an enhanced inhibitory effect of molecular interneurons over Purkinje activity as parallel fibers contact and can stimulate dendrites of molecular interneurons (Gao et al., 2016; Albergaria et al., 2018). Then, one should expect granule cell activation to facilitate cerebellar output by reducing Purkinje inhibitory control onto DCN neurons. However, we have not been able to demonstrate consistent Purkinje activity changes being linked to cocaine-induced preference memory by using cFos expression analysis (Carbo-Gas et al., 2014a, b).
A significant issue in the addiction field is to explain why drug-induced memories are so persistent and long-lasting. In this respect, we have been interested in the function of perineuronal nets (PNNs) in the cerebellum and their regulation by cocaine-related behavior. PNNs are extracellular matrix lattice cartilage-like structures that wrap the soma and proximal neurites of several neuronal populations, the majority fast-spiking GABAergic interneurons (Grimpe and Silver, 2002; Fawcett et al., 2019). These structures restrict neuronal plasticity and actively contribute to synaptic stabilization in the adult brain (Dityatev and Schachner, 2003; Corvetti and Rossi, 2005). In the cerebellum, only a few neuronal populations are surrounded by PNNs, and unlike other regions, PNN-bearing neurons do not express parvalbumin (Carulli et al., 2006). In a recent study, we have investigated the regulation of cerebellar PNNs by cocaine-induced memory (Carbo-Gas et al., 2017). The acquisition of conditioned preference for cocaine-related cues increases PNN expression surrounding Golgi interneurons in the apical region of the cerebellar cortex. Stronger PNNs correlate with a higher preference for cocaine-related cues. Again, the effect was found in the apical region of the cerebellar cortex and was not reproduced in pseudo-conditioned groups or when conditioned response was not evident. However, PNNs expressed by inhibitory and excitatory neuronal populations in the DCN decreased in all cocaine-treated groups irrespective of the establishment of a conditioned preference. Importantly, previous research has shown that degradation of PNNs in the prelimbic cortex and anterior hypothalamus is able to prevent the formation of drug-related memory (Slaker et al., 2015; Blacktop et al., 2017).
Taken together, our findings indicate that drug-related memory may be represented in the cerebellum through two hallmarks signatures localized in the apical region of the granule cell layer: increased granule cell activity and strong fully condensed PNNs around Golgi interneurons. We have not been able to reproduce either of these effects in ventral regions of the posterior vermis though these regions expressed high granule cell activity and a large number of Golgi cells bearing a PNN (Carbo-Gas et al., 2014a, 2017). These results raise the question of which functional characteristics and connectivity patterns make the apical region relevant to drug associative memory (Gil-Miravet et al., 2019a, b). The apical cerebellar cortex receives mossy fiber inputs from sensorimotor and exteroceptive cortices through specialized regions in the pontine nuclei (Voogd and Ruigrok, 2004). In addition, a prominent excitatory projection of mossy fibers to the apical region of the granule cell layer arises from the DCN and has demonstrated to optimize the conditioned response in motor associative learning (Gao et al., 2016). Finally, granule cell activity in this area is present during unconditioned and conditioned stimuli, as well as during the conditioned response (Giovannucci et al., 2017; Wagner et al., 2017).
In a pursuit of an explanation for the relevance of this cerebellar region, we investigated the effects of a neurotoxic lesion restricted to the apical part of the posterior vermis (lobule 8) on cocaine-related memory (Gil-Miravet et al., 2019a, b). Unexpectedly, the apical lesion dramatically raises the probability of learning/expressing the cocaine-cue Pavlovian association (Gil-Miravet et al., 2019a, b). More important, the cerebellar lesion increased neuronal activity in the mPFC, NAC, and all striatal subdivisions except the ventrolateral striatum, backing the idea of the VTA as a plausible hotspot for a modulatory action of the cerebellum on drug-related memory effects (Carta et al., 2019; Gil-Miravet et al., 2019b). Indeed, using both retrograde and anterograde tracing, we demonstrated that neurons from the apical region of lobule 8 in the vermis reach both the interposed and lateral nuclei (DCN) in the deep cerebellum running laterally to the middle line. In turn, the DCN send direct glutamatergic projections to the contralateral VTA making contacts with dopaminergic and non-dopaminergic neurons. The role of the cerebellum in regulating VTA neuronal activity and conditioned place preference (CPP) has been nicely demonstrated in a recent paper (Carta et al., 2019). Optogenetic stimulation of DCN-VTA glutamatergic projections activated VTA neurons and was sufficient to induce a strong CPP. In agreement, the apical lesion of lobule 8 increased neural activity in the dentate nucleus, likely by reducing the tonic inhibition exerts by Purkinje onto DCN neurons (Gil-Miravet et al., 2019b). We were also interested in the role of the functional loops between the cerebellum and medial prefrontal cortex as well. Our results showed that the deactivation of the infralimbic (IL) but not the prelimbic (PL) cortex during conditioning also facilitates the acquisition of cocaine-induced conditioned preference. Interestingly, simultaneous cerebellum-IL deactivations abolished the facilitative effect of separate deactivations on drug-related learning, indicating a compensatory close IL-cerebellar loop (Gil-Miravet et al., 2019a; Figure 2).
Model-Free vs. Model-Based Systems in Drug Reward Learning. What Would the Cerebellum’s Role Be?
Reinforcement learning involves two distinct and parallel processes with dissociated neural substrates (Balleine and Dickinson, 1998). During instrumental actions, a flexible and planned goal-directed behavior can compete but also cooperate with the stimulus-response automatic process (habit). When habitual behavior is established, the probability of responding for devalued outcomes increases because the mental representation of the relationship between the stimulus (S) and response (R) does not incorporate information about the current value of the outcome (Adams and Dickinson, 1981; Dayan and Balleine, 2002; Balleine and O’Doherty, 2010). From a theory of decision perspective, these two different systems guide behavior during decision making. A model-based system (goal-directed) that includes an explicit knowledge about the reward context competes with a model-free system (habit) that learn about the reward value and emits habit-like responses with repetition (Lee et al., 2014). The first one operates under uncertainty while the second predominates when outcomes become more predictable. The ability to resolve and monitor the competition between habit and goal-directed processes entails a coordinated work of cortico-basal ganglia-cerebellar loops (Caligiore et al., 2017) and depends on the engagement of executive control (Lee et al., 2014; Watson et al., 2018). It has been proposed that the executive control is under an “arbitrator” that would mediate between planning systems that use predictions of action-outcomes and habitual action selection systems that learn to automate by repeating previously rewarded actions (Lee et al., 2014). The model-free seems to be the default system (Wang et al., 2018). Namely, the arbitrator appears to modulate the activity of brain regions involved in model-free control thereby gating the shift to a flexible behavioral approach. Although it is far to be clear which brain regions may arbitrate this action selection, findings point to inferior medial and lateral prefrontal regions including the rostral cingulate cortex (Lee et al., 2014). Moreover, unquestionable evidence indicates that ventral regions of the basal ganglia receiving dopamine projections from the VTA and SNc are responsible for goal-directed behaviors (de Wit et al., 2009; Balleine and O’Doherty, 2010). Likewise, habit-like responses require the integrity of sensorimotor cortices and dorsolateral regions within the striatum (caudate/putamen) (Yin and Knowlton, 2006; Lee et al., 2014).
Recent findings provide compelling support for the role of the cerebellum in reward-based reinforcement learning and goal-directed behavior (Wagner et al., 2017; Carta et al., 2019; Hull, 2020), but also in model-free learning (Callu et al., 2007; De Bartolo et al., 2009; Liljeholm et al., 2015; Watson et al., 2018). It is clear that cf and Gr cells (Figure 1) generate responses in the cerebellar cortex to events that predict upcoming reward, but they also encode reward omissions (Wagner et al., 2017; Heffley et al., 2018). These signals will enable Purkinje cells to learn responses and establish “forward models” to make effective predictions that regulate behavioral decisions.
One of the most relevant insights into the role of the cerebellum in goal-directed behavior has been provided by the recent study by Carta et al. (2019). In this work, optogenetic stimulation of glutamatergic projections from the DCN to VTA induces consistent short and long-term place preference for the location in which the optogenetic stimulation was applied. Optogenetic stimulation was release upon entry in the reward location. Mice expressing channelrhodopsin2 (ChR2) actively approached this location to obtain the stimulation of the cerebellum-VTA pathway. Moreover, the stimulation was able to increase firing in one third of VTA cells in vivo, eliciting excitatory synaptic currents in DA and non-DA neurons. Optogenetic inhibition of cerebellar terminals in the VTA did not induce aversion but prevented the establishment of social preferences. Altogether, these findings reveal that the cerebellum not only is able to encode the goal and the context but also to regulate behavior to reach the goal.
Evidence about the contribution of the cerebellum to habits has come from animal and human research. A bilateral lesion in the interposed nucleus or hemicerebellectomy (Callu et al., 2007; De Bartolo et al., 2009) prevent the establishment of habits with overtraining. In these experiments, animals with the cerebellar alteration maintained the action-outcome features despite overtraining, and expression of an automatic cue-response stage is not created. In humans, the cerebellum and other regions in the sensorimotor network show greater activation when subjects respond to previously devalued outcomes, suggesting their involvement in the expression of S-R habits (Watson et al., 2018; Liljeholm et al., 2015). Indeed, activity in the tail of the caudate/thalamus, the cerebellum and the lingual gyrus predicts insensitivity to outcome devaluation. The greater the activity the higher the probability to respond to devaluated outcomes (Liljeholm et al., 2015). In this case, the cerebellar activity did not result from repetition, since the task did not involve overtraining. On the contrary, it predicted the formation of strong S-R associations.
Drug addiction like other compulsive disorders might result from an excessive dominance of model-free control (Everitt and Robbins, 2005; Lüscher et al., 2020). With extended drug experience, cue-action-reward associations become stronger and drug-associated cues come to be powerful motivational triggers for craving and relapse even after long periods of protracted abstinence. Addictive behavior has been identified as a compulsive habit (Belin et al., 2013). First, drug seeking is automatically triggered by the presence of drug-related cues or their mental representation. Second, behavior becomes insensitive to outcome devaluation (Everitt and Robbins, 2005; Zapata et al., 2010; Belin et al., 2013; Ersche et al., 2016). Compulsive disorders imply a failure in cortical top-down control and over-activity in dorsal regions of the basal ganglia which cause behavioral disinhibition and stereotyped behavioral repetition (Fineberg et al., 2014; Figee et al., 2016).
Structural neuroimage findings also point to a role for the cerebellum in compulsive behavior. The most common finding of patients with compulsive disorders has been decreased gray matter (GM) volume in several regions of the cerebellum and increased basal ganglia-cerebellar connectivity (see Miquel et al., 2019 for a review). Moreover, drug addicts and heavy drug users exhibit a dysfunctional prefrontal-cerebellar pattern in which the cerebellum appears to be overactive during cognitive tasks that should recruit prefrontal resources (Hester and Garavan, 2004; Bolla et al., 2005; Goldstein et al., 2007). Another brain region that has been demonstrated to be crucial for the formation and crystallization of habits is the infralimbic cortex (IL) (Killcross and Coutureau, 2003; Smith and Graybiel, 2013; Barker et al., 2018). Activity in the IL during habit formation is necessary for full habit acquisition. In fact, repeated optogenetic inhibition during overtraining disrupts the formation of habits in rats and mice (Smith and Graybiel, 2013; Barker et al., 2018).
Overall, our recent findings reveal that the cerebellum may modulate cocaine-induced learning, but also neural activity and synaptic stabilization mechanisms in the IL (Gil-Miravet et al., 2019b). Impairment of the posterior vermis increased activity and perineuronal expression in the IL, but only in those rats that expressed the conditioned response of preference for drug associated cues. We have proposed that under stimulation of the cerebellar cortex the prevailing behavioral pattern of drug seeking will be flexible and sensitive to reward devaluation (goal-directed). On the contrary, cerebellar cortex deactivations will encourage the formation of strong cue-drug associations and insensitivity to reward devaluation (habit) (Gil-Miravet et al., 2019b; Miquel et al., 2019).
Concluding Remarks
Overall, findings point to a modulatory function of the cerebellum on the establishment of drug-induced associative memory through the regulation of VTA activity. Nonetheless, research about the cerebellum’s role in drug-related memory is still in its infancy and there are open questions that remain unanswered. First, it is unknown through which neural pathways drug-related cue and unconditioned signals are conveyed to the cerebellum. Second, it is also ignored how cerebellar cortex encodes the conditioned cue and unconditioned properties of the drug. Third, the role of Purkinje neurons in drug-cue associative memory needs to be further explored. Finally, it is crucial to test the hypotheses including addictive drugs with distinct neuropharmacological actions. It is expected the modulatory role of the cerebellum to affect not only the expression of the conditioned response but also non-motor aspects of drug-induced reward. To address all these questions more precisely cell-specific tools such as DREADDs (designer receptor exclusively activated by designer drugs), optogenetics, electrophysiology and viral tracing will be required.
Our findings also suggest that pathological conditions impairing the cerebellar cortex may increase the likelihood of acquiring drug-induced associative memory and promote drug relapse through disinhibition of VTA and its projecting regions. Moreover, they may explain why both cerebellar disfunction and prefrontal impairment enhance susceptibility to compulsive and impulsive disorders including drug addiction, eating disorders, attention deficit/hyperactivity (ADHD), and obsessive-compulsive disorder (OCD) (Miquel et al., 2019). Accordingly, it has been described that in humans, the impairment of the vermis results in impulsivity and disinhibition (Silveri et al., 1994; Schmahmann and Sherman, 1998; Kim et al., 2013; Tessier et al., 2015), inducing what has been called the Cerebellar Cognitive Affective Syndrome (CAS) (Schmahmann and Sherman, 1998). It would be of the utmost importance to test whether similar cerebellar disfunctions that lead to CAS increase the risk for drug addiction or are sufficient for inducing a compulsive addictive phenotype.
Author Contributions
Findings discussed in this work were obtained by IG-M and JG-C as a part of their doctoral theses and also critically reviewed the manuscript. MM was responsible for writing and critically edited the review. All the authors approved the final version for publication.
Funding
This work was supported by Ministerio de Ciencia e Innovación, Grant/Award No. PGC2018-095980-B-I00; Ministerio de Economía y Competitividad (MINECO), Grant/Award Number: PSI2015-68600-P; Plan Nacional de Drogas 2017, Grant/Award No. PND-132400; Universitat Jaume I (UJI), Grant/Award No. 14I307.01/1; Universitat Jaume I, PREDOC2014/11.
Conflict of Interest
The authors declare that the research was conducted in the absence of any commercial or financial relationships that could be construed as a potential conflict of interest.
References
Adams, C. D., and Dickinson, A. (1981). Instrumental responding following reinforcer devaluation. Q. J. Exp. Psychol. Sect. B 33, 109–121. doi: 10.1080/14640748108400816
Albergaria, C., Silva, N. T., Pritchett, D. L., and Carey, M. R. (2018). Locomotor activity modulates associative learning in mouse cerebellum. Nat. Neurosci. 21, 725–735. doi: 10.1038/s41593-018-0129-x
Allsop, J., and Turner, B. (1966). Cerebellar degeneration associated with chronic alcoholism. J. Neurol. Sci. 3, 238–258. doi: 10.1016/0022-510x(66)90024-4
Anderson, C. M., Maas, L. C., Frederick, B. D. B., Bendor, J. T., Spencer, T. J., Livni, E., et al. (2006). Cerebellar vermis involvement in cocaine-related behaviors. Neuropsychopharmacology 31, 1318–1326. doi: 10.1038/sj.npp.1300937
Azevedo, F. A. C., Carvalho, L. R. B., Grinberg, L. T., Farfel, J. M., Ferretti, R. E. L., Leite, R. E. P., et al. (2009). Equal numbers of neuronal and nonneuronal cells make the human brain an isometrically scaled-up primate brain. J. Comp. Neurol. 513, 532–541. doi: 10.1002/cne.21974
Balleine, B. W., and Dickinson, A. (1998). Goal-directed instrumental action: contingency and incentive learning and their cortical substrates. Neuropharmacology. 37, 407–419. doi: 10.1016/s0028-3908(98)00033-1
Balleine, B. W., and O’Doherty, J. P. (2010). Human and rodent homologies in action control: corticostriatal determinants of goal-directed and habitual action. Neuropsychopharmacology 35, 48–69. doi: 10.1038/npp.2009.131
Barker, J. M., Glen, W. B., Linsenbardt, D. N., Lapish, C. C., and Chandler, L. J. (2018). Habitual behavior is mediated by a shift in response-outcome encoding by infralimbic cortex. eNeuro 4, 2–22. doi: 10.1523/ENEURO.0337-17.2017
Barton, R. A. (2012). Embodied cognitive evolution and the cerebellum. Philos. Trans. R. Soc. Lond. B Biol. Sci. 367, 2097–2107. doi: 10.1098/rstb.2012.0112
Bastian, A. J. (2006). Learning to predict the future: the cerebellum adapts feedforward movement control. Curr. Opin. Neurobiol. 16, 645–649. doi: 10.1016/j.conb.2006.08.016
Belin, D., Belin-Rauscent, A., Murray, J. E., and Everitt, B. J. (2013). Addiction: failure of control over maladaptive incentive habits. Curr. Opin. Neurobiol. 23, 564–572. doi: 10.1016/j.conb.2013.01.025
Bhargava, H. N., and Kumar, S. (1999). Sensitization to the locomotor stimulant effect of cocaine modifies the binding of [3H]MK-801 to brain regions and spinal cord of the mouse. Gen. Pharmacol. 3, 359–363. doi: 10.1016/s0306-3623(98)00107-4
Blacktop, J. M., Todd, R. P., and Sorg, B. A. (2017). Role of perineuronal nets in the anterior dorsal lateral hypothalamic area in the acquisition of cocaine-induced conditioned place preference and self-administration. Neuropharmacology 118, 124–136. doi: 10.1016/j.neuropharm.2017.03.018
Blackwood, N., Ffytche, D., Simmons, A., Bentall, R., Murray, R., and Howard, R. (2004). The cerebellum and decision making under uncertainty. Cogn. Brain Res. 20, 46–53. doi: 10.1016/j.cogbrainres.2003.12.009
Blednov, Y. A., Borghese, C. M., Ruiz, C. I., Cullins, M. A., Da Costa, A., Osterndorff-Kahanek, E. A., et al. (2017). Mutation of the inhibitory ethanol site in GABAA ρ1 receptors promotes tolerance to ethanol-induced motor incoordination. Neuropharmacology 123, 201–209. doi: 10.1016/j.neuropharm.2017.06.013
Bobadilla, A. C., Garcia-Keller, C., Chareunsouk, V., Hyde, J., Medina Camacho, D., Heinsbroek, J. A., et al. (2019). Accumbens brain-derived neurotrophic factor (BDNF) transmission inhibits cocaine seeking. Addict. Biol. 24, 860–873. doi: 10.1111/adb.12638
Bolla, K. I., Eldreth, D. A., Matochik, J. A., and Cadet, J. L. (2005). Neural substrates of faulty decision-making in abstinent marijuana users. Neuroimage 26, 480–492. doi: 10.1016/j.neuroimage.2005.02.012
Bonson, K. R., Grant, S. J., Contoreggi, C. S., Links, J. M., Metcalfe, J., Weyl, H. L., et al. (2002). Neural systems and cue-induced cocaine craving. Neuropsychopharmacology 26, 376–386. doi: 10.1016/S0893-133X(01)00371-2
Bostan, A. C., Dum, R. P., and Strick, P. L. (2013). Cerebellar networks with the cerebral cortex and basal ganglia. Trends Cogn. Sci. 17, 241–254. doi: 10.1016/j.tics.2013.03.003
Caligiore, D., Pezzulo, G., Baldassarre, G., Bostan, A. C., Strick, P. L., Doya, K., et al. (2017). Consensus paper: towards a systems-level view of cerebellar function: the interplay between cerebellum, basal ganglia, and cortex. Cerebellum 16, 203–229. doi: 10.1007/s12311-016-0763-3
Callu, D., Puget, S., Faure, A., Guegan, M., and El Massioui, N. (2007). Habit learning dissociation in rats with lesions to the vermis and the interpositus of the cerebellum. Neurobiol. Dis. 27, 228–237. doi: 10.1016/j.nbd.2007.04.007
Carbo-Gas, M., Moreno-Rius, J., Guarque-Chabrera, J., Vazquez-Sanroman, D., Gil-Miravet, I., Carulli, D., et al. (2017). Cerebellar perineuronal nets in cocaine-induced pavlovian memory: site matters. Neuropharmacology 125, 166–180. doi: 10.1016/j.neuropharm.2017.07.009
Carbo-Gas, M., Vazquez-Sanroman, D., Aguirre-Manzo, L., Coria-Avila, G. A., Manzo, J., Sanchis-Segura, C., et al. (2014a). Involving the cerebellum in cocaine-induced memory: pattern of cFos expression in mice trained to acquire conditioned preference for cocaine. Addict. Biol. 19, 61–76. doi: 10.1111/adb.12042
Carbo-Gas, M., Vazquez-Sanroman, D., Gil-Miravet, I., De Las Heras-Chanes, J., Coria-Avila, G. A., Manzo, J., et al. (2014b). Cerebellar hallmarks of conditioned preference for cocaine. Physiol. Behav. 132, 24–35. doi: 10.1016/j.physbeh.2014.04.044
Cardoso, C. O., Branco, L. D., Cotrena, C., Kristensen, C. H., Bakos, D. D. G. S., and Fonseca, R. P. (2014). The impact of frontal and cerebellar lesions on decision making: evidence from the Iowa Gambling task. Front. Neurosci. 8:61. doi: 10.3389/fnins.2014.00061
Carta, I., Chen, C. H., Schott, A. L., Dorizan, S., and Khodakhah, K. (2019). Cerebellar modulation of the reward circuitry and social behavior. Science 363:eaav0581. doi: 10.1126/science.aav0581
Carulli, D., Broersen, R., de Winter, F., Muir, E. M., Mešković, M., de Waal, M., et al. (2020). Cerebellar plasticity and associative memories are controlled by perineuronal nets. Proc. Natl. Acad. Sci. U.S.A. 117, 6855–6865. doi: 10.1073/pnas.1916163117
Carulli, D., Rhodes, K. E., Brown, D. J., Bonnert, T. P., Pollack, S. J., Oliver, K., et al. (2006). Composition of perineuronal nets in the adult rat cerebellum and the cellular origin of their components. J. Comp. Neurol. 494, 559–577. doi: 10.1002/cne.20822
Casu, M. A., Pisu, C., Sanna, A., Tambaro, S., Spada, G. P., Mongeau, R., et al. (2005). Effect of delta9-tetrahydrocannabinol on phosphorylated CREB in rat cerebellum: an immunohistochemical study. Brain Res. 1048, 41–47. doi: 10.1016/j.brainres.2005.04.053
Chen, C. H., Fremont, R., Arteaga-Bracho, E. E., and Khodakhah, K. (2014). Short latency cerebellar modulation of the basal ganglia. Nat. Neurosci. 17, 1767–1775. doi: 10.1038/nn.3868
Corominas-Roso, M., Roncero, C., Daigre, C., Grau-Lopez, L., Ros-Cucurull, E., Rodríguez-Cintas, L., et al. (2015). Changes in brain-derived neurotrophic factor (BDNF) during abstinence could be associated with relapse in cocaine-dependent patients. Psychiatry Res. 225, 309–314. doi: 10.1016/j.psychres.2014.12.019
Corvetti, L., and Rossi, F. (2005). Degradation of chondroitin sulfate proteoglycans induces sprouting of intact purkinje axons in the cerebellum of the adult rat. J. Neurosci. 25, 7150–7158. doi: 10.1523/JNEUROSCI.0683-05.2005
Couceyro, P., Pollock, K. M., Drews, K., and Douglass, J. (1994). Cocaine differentially regulates activator protein-1 mRNA levels and DNA-binding complexes in the rat striatum and cerebellum. Mol. Pharmacol. 4, 667–676.
Dayan, P., and Balleine, B. W. (2002). Reward, motivation, and reinforcement learning. Neuron 36, 285–298. doi: 10.1016/s0896-6273(02)00963-7
De Bartolo, P., Mandolesi, L., Federico, F., Foti, F., Cutuli, D., Gelfo, F., et al. (2009). Cerebellar involvement in cognitive flexibility. Neurobiol. Learn. Mem. 92, 310–317.
de Wit, S., Corlett, P. R., Aitken, M. R., Dickinson, A., and Fletcher, P. C. (2009). Differential engagement of the ventromedial prefrontal cortex by goal-directed and habitual behavior toward food pictures in humans. J. Neurosci. 29, 11330–11338. doi: 10.1523/JNEUROSCI.1639-09.2009
De Zeeuw, C. I., and Yeo, C. H. (2005). Time and tide in cerebellar memory formation. Curr. Opin. Neurobiol. 15, 667–674. doi: 10.1016/j.conb.2005.10.008
Decker, J. B., Wells, C. E., and Mcdowell, F. (1959). Cerebellar dysfunction associated with chronic alcoholism. Neurology 9, 361–366. doi: 10.1212/wnl.9.5.361
Deverett, B., Kislin, M., Tank, D. W., and Wang, S. S. (2019). Cerebellar disruption impairs working memory during evidence accumulation. Nat. Commun. 10:3128. doi: 10.1038/s41467-019-11050-x
Dewey, W. L., McMillan, D. E., Harris, L. S., and Turk, R. F. (1973). Distribution of radioactivity in brain of tolerant and nontolerant pigeons treated with 3 H- 9 -tetrahydrocannabinol. Biochem. Pharmacol. 22, 399–405. doi: 10.1016/0006-2952(73)90420-6
Dityatev, A., and Schachner, M. (2003). Extracellular matrix molecules and synaptic plasticity. Nat. Rev. Neurosci. 4, 456–468. doi: 10.1038/nrn1115
Doya, K. (2000). Complementary roles of basal ganglia and cerebellum in learning and motor control. Curr. Opin. Neurobiol. 10, 732–739. doi: 10.1016/s0959-4388(00)00153-7
D’Sa, C., Fox, H. C., Hong, A. K., Dileone, R. J., and Sinha, R. (2011). Increased serum brain-derived neurotrophic factor is predictive of cocaine relapse outcomes: a prospective study. Biol. Psychiatry. 70, 706–711. doi: 10.1016/j.biopsych.2011.05.013
Ersche, K. D., Gillan, C. M., Jones, P. S., Williams, G. B., Ward, L. H., Luijten, M., et al. (2016). Carrots and sticks fail to change behavior in cocaine addiction. Science 352, 1468–1471.
Everitt, B. J., and Robbins, T. W. (2005). Neural systems of reinforcement for drug addiction: from actions to habits to compulsion. Nat. Neurosci. 8, 1481–1489. doi: 10.1038/nn1579
Fawcett, J. W., Oohashi, T., and Pizzorusso, T. (2019). The roles of perineuronal nets and the perinodal extracellular matrix in neuronal function. Nat. Rev. Neurosci. 20, 451–465. doi: 10.1038/s41583-019-0196-3
Figee, M., Pattij, T., Willuhn, I., Luigjes, J., van den Brink, W., Goudriaan, A., et al. (2016). Compulsivity in obsessive-compulsive disorder and addictions. Eur. Neuropsychopharmacol. 26, 856–868.
Filbey, F. M., Schacht, J. P., Myers, U. S., Chavez, R. S., and Hutchison, K. E. (2009). Marijuana craving in the brain. Proc. Natl. Acad. Sci. U.S.A. 106, 13016–13021. doi: 10.1073/pnas.0903863106
Fineberg, N. A., Chamberlain, S. R., Goudriaan, A. E., Stein, D. J., Vanderschuren, L. J. M. J., Gillan, C. M., et al. (2014). New developments in human neurocognition: clinical, genetic, and brain imaging correlates of impulsivity and compulsivity. CNS Spectr. 19, 69–89. doi: 10.1017/S1092852913000801
Forster, G. L., and Blaha, C. D. (2003). Pedunculopontine tegmental stimulation evokes striatal dopamine efflux by activation of acetylcholine and glutamate receptors in the midbrain and pons of the rat. Eur. J. Neurosci. 17, 751–762. doi: 10.1046/j.1460-9568.2003.02511.x
Fumagalli, F., Di Pasquale, L., Caffino, L., Racagni, G., and Riva, M. A. (2007). Repeated exposure to cocaine differently modulates BDNF mRNA and protein levels in rat striatum and prefrontal cortex. Eur. J. Neurosci. 26, 2756–2763. doi: 10.1111/j.1460-9568.2007.05918.x
Galliano, E., and De Zeeuw, C. (2014). Questioning the cerebellar doctrine. Prog. Brain Res. 210, 59–77. doi: 10.1016/B978-0-444-63356-9.00003-0
Galliano, E., Gao, Z., Schonewille, M., Todorov, B., Simons, E., Pop, A. S., et al. (2013). Silencing the majority of cerebellar granule cells uncovers their essential role in motor learning and consolidation. Cell Rep. 3, 1239–1251. doi: 10.1016/j.celrep.2013.03.023
Gao, Z., Davis, C., Thomas, A. M., Economo, M. N., Abrego, A. M., Svoboda, K., et al. (2018). A cortico-cerebellar loop for motor planning. Nature 563, 113–116. doi: 10.1038/s41586-018-0633-x
Gao, Z., Proietti-Onori, M., Lin, Z., Ten Brinke, M. M., Boele, H. J., Potters, J. W., et al. (2016). Excitatory cerebellar nucleocortical circuit provides internal amplification during associative conditioning. Neuron 89, 645–657. doi: 10.1016/j.neuron.2016.01.008
Gil-Miravet, I., Guarque-Chabrera, J., Carbo-Gas, M., Olucha-Bordonau, F., and Miquel, M. (2019a). The role of the cerebellum in drug-cue associative memory: functional interactions with the medial prefrontal cortex. Eur. J. Neurosci. 50, 2613–2622. doi: 10.1111/ejn.14187
Gil-Miravet, I., Melchor-Eixea, I., Arias-Sandoval, E., Vasquez-Celaya, L., Guarque-Chabrera, J., Olucha-Bordonau, F., et al. (2019b). From back to front: a functional model for the cerebellar modulation in the establishment of conditioned preferences for cocaine-related cues. Addict. Biol. doi: 10.1111/adb.12834 [Epub ahead of print],
Giovannucci, A., Badura, A., Deverett, B., Najafi, F., Pereira, T. D., Gao, Z., et al. (2017). Cerebellar granule cells acquire a widespread predictive feedback signal during motor learning. Nat. Neurosci. 20, 727–734. doi: 10.1038/nn.4531
Goldstein, R. Z., Tomasi, D., Alia-Klein, N., Zhang, L., Telang, F., and Volkow, N. D. (2007). The effect of practice on a sustained attention task in cocaine abusers. Neuroimage 35, 194–206. doi: 10.1016/j.neuroimage.2006.12.004
Grant, S., London, E. D., Newlin, D. B., Villemagne, V. L., Liu, X., Contoreggi, C., et al. (1996). Activation of memory circuits during cue elicited cocaine craving. Proc. Natl. Acad. Sci. U.S.A. 93, 12040–12045. doi: 10.1073/pnas.93.21.12040
Grimm, J. W., Lu, L., Hayashi, T., Hope, B. T., Su, T. P., and Shaham, Y. (2003). Time-dependent increases in brain-derived neurotrophic factor protein levels within the mesolimbic dopamine system after withdrawal from cocaine: implications for incubation of cocaine craving. J. Neurosci. 23m, 742–747. doi: 10.1523/JNEUROSCI.23-03-00742.2003
Grimpe, B., and Silver, J. (2002). The extracellular matrix in axon regeneration. Prog. Brain Res. 137, 333–349. doi: 10.1016/s0079-6123(02)37025-0
Heffley, W., Song, E. Y., Xu, Z., Taylor, B. N., Hughes, M. A., McKinney, A., et al. (2018). Coordinated cerebellar climbing fiber activity signals learned sensorimotor predictions. Nat. Neurosci. 21, 1431–1441. doi: 10.1038/s41593-018-0228-8
Herculano-Houzel, S. (2010). Coordinated scaling of cortical and cerebellar numbers of neurons. Front. Neuroanat. 4:12. doi: 10.3389/fnana.2010.00012
Hester, R., and Garavan, H. (2004). Executive dysfunction in cocaine addiction: evidence for discordant frontal, cingulate, and cerebellar activity. J. Neurosci. 24, 11017–11022. doi: 10.1523/JNEUROSCI.3321-04.2004
Ho, B. T., Taylor, D., Fritchie, G. E., Englert, L. F., and McIsaac, W. M. (1972). Neuropharmacological study of 9 - and 8 -L-tetrahydrocannabinols in monkeys and mice. Brain Res. 38, 163–170. doi: 10.1016/0006-8993(72)90597-5
Houk, J. C., and Wise, S. P. (1995). Distributed modular architectures linking basal ganglia, cerebellum, and cerebral cortex: their role in planning and controlling action. Cereb. Cortex 5, 95–110. doi: 10.1093/cercor/5.2.95
Hull, C. (2020). Prediction signals in the cerebellum: beyond supervised motor learning. eLife 9:e54073. doi: 10.7554/eLife.54073
Hyman, S. E., Malenka, R. C., and Nestler, E. J. (2006). Neural mechanisms of addiction: the role of reward-related learning and memory. Annu. Rev. Neurosci. 29, 565–598. doi: 10.1146/annurev.neuro.29.051605.113009
Kafitz, K. W., Rose, C. R., Thoenen, H., and Konnerth, A. (1999). Neurotrophin-evoked rapid excitation through TrkB receptors. Nature 401, 918–921. doi: 10.1038/44847
Killcross, S., and Coutureau, E. (2003). Coordination of actions and habits in the medial prefrontal cortex of rats. Cereb. Cortex 13, 400–408.
Killicorn, S. A. (1955). Presenile cerebellar ataxia in chronic alcoholics. Neurology 5, 527–534. doi: 10.1212/wnl.5.8.527
Kilts, C. D., Schweitzer, J. B., Quinn, C. K., Gross, R. E., Faber, T. L., Muhammad, F., et al. (2001). Neural activity related to drug craving in cocaine addiction. Arch. Gen. Psychiatry 58, 334–341. doi: 10.1001/archpsyc.58.4.334
Kim, J. H., Kim, T. H., Choi, Y. C., Chung, S. C., and Moon, S. W. (2013). Impulsive behavior and recurrent major depression associated with dandy-walker variant. Psychiatry Investig. 10, 303–305. doi: 10.4306/pi.2013.10.3.303
Kim, J. J., and Thompson, R. F. (1997). Cerebellar circuits and synaptic mechanisms involved in classical eyeblink conditioning. Trends Neurosci. 20, 177–181. doi: 10.1016/s0166-2236(96)10081-3
Klitenick, M. A., Tham, C. S., and Fibiger, H. C. (1995). Cocaine and d-amphetamine increase c-fos expression in the rat cerebellum. Synapse 19, 29–36. doi: 10.1002/syn.890190105
Koob, G. F., and Volkow, N. D. (2010). Neurocircuitry of addiction. Neuropsychopharmacology 35, 217–238. doi: 10.1038/npp.2009.110
Kovalchuk, Y., Hanse, E., Kafitz, K. W., and Konnerth, A. (2002). Postsynaptic induction of BDNF-mediated long-term potentiation. Science 295, 1729–1734. doi: 10.1126/science.1067766
Lackey, E. P., Heck, D. H., and Sillitoe, R. V. (2018). Recent advances in understanding the mechanisms of cerebellar granule cell development and function and their contribution to behavior. F1000Res. 7, 1–12. doi: 10.12688/f1000research.15021.1
Lee, S. W., Shimojo, S., and O’Doherty, J. P. (2014). Neural computations underlying arbitration between model-based and model-free learning. Neuron 81, 687–699. doi: 10.1016/j.neuron.2013.11.028
Li, X., and Wolf, M. E. (2015). Multiple faces of BDNF in cocaine addiction. Behav. Brain Res. 279, 240–254. doi: 10.1016/j.bbr.2014.11.018
Liljeholm, M., Dunne, S., and O’Doherty, J. P. (2015). Differentiating neural systems mediating the acquisition vs. expression of goal-directed and habitual behavioral control. Eur. J. Neurosci. 41, 1358–1371. doi: 10.1111/ejn.12897
Liljequist, S., and Tabakoff, B. (1985). Binding characteristics of 3H-flunitrazepam and CL-218,872 in cerebellum and cortex of C57B1 mice made tolerant to and dependent on phenobarbital or ethanol. Alcohol 2, 215–220. doi: 10.1016/0741-8329(85)90048-5
Lu, B., and Figurov, A. (1997). Role of neurotrophins in synapse development and plasticity. Rev. Neurosci. 8, 1–12. doi: 10.1515/revneuro.1997.8.1.1
Lüscher, C., Robbins, T. W., and Everitt, B. J. (2020). The transition to compulsion in addiction. Nat. Rev. Neurosci. 21, 247–263. doi: 10.1038/s41583-020-0289-z
Martin-Sölch, C., Magyar, S., Künig, G., Missimer, J., Schultz, W., and Leendersw, K. L. (2001). Changes in brain activation associated with reward processing in smoker and nonsmokers. A positron emission tomography study. Exp. Brain Res. 139, 278–286. doi: 10.1007/s002210100751
Miquel, M., Nicola, S. M., Gil-Miravet, I., Guarque-Chabrera, J., and Sanchez-Hernandez, A. (2019). A working hypothesis for the role of the cerebellum in impulsivity and compulsivity. Front. Behav. Neurosci. 13:99. doi: 10.3389/fnbeh.2019.00099
Miquel, M., Toledo, R., García, L. I., Coria-Avila, G. A., and Manzo, J. (2009). Why should we keep the cerebellum in mind when thinking about addiction? Curr. Drug Abuse Rev. 2, 26–40. doi: 10.2174/1874473710902010026
Miquel, M., Vazquez-Sanroman, D., Carbo-Gas, M., Gil-Miravet, I., Sanchis-Segura, C., Carulli, D., et al. (2016). Have we been ignoring the elephant in the room? Seven arguments for considering the cerebellum as part of addiction circuitry. Neurosci. Biobehav. Rev. 2016, 1–11. doi: 10.1016/j.neubiorev.2015.11.005
Moreno-Rius, J., and Miquel, M. (2017). The cerebellum in drug craving. Drug Alcohol Depend. 173, 151–158. doi: 10.1016/j.drugalcdep.2016.12.028
Moulton, E. A., Elman, I., Becerra, L. R., Goldstein, R. Z., and Borsook, D. (2014). The cerebellum and addiction: insights gained from neuroimaging research. Addict. Biol. 3, 317–331. doi: 10.1111/adb.12101
Nguyen, V. T., Sonkusare, S., Stadler, J., Hu, X., Breakspear, M., and Guo, C. C. (2017). Distinct cerebellar contributions to cognitive-perceptual dynamics during natural viewing. Cereb. Cortex 27, 5652–5662. doi: 10.1093/cercor/bhw334
Palomino, A., Pavón, F. J., Blanco-Calvo, E., Serrano, A., Arrabal, S., Rivera, P., et al. (2014). Effects of acute versus repeated cocaine exposure on the expression of endocannabinoid signaling related proteins in the mouse cerebellum. Front. Integr. Neurosci. 8:22. doi: 10.3389/fnint.2014.00022
Proville, R. D., Spolidoro, M., Guyon, N., Dugué, G. P., Selimi, F., Isope, P., et al. (2014). Cerebellum involvement in cortical sensorimotor circuits for the control of voluntary movements. Nat. Neurosci. 17, 1233–1239. doi: 10.1038/nn.3773
Risinger, R. C., Salmeron, B. J., Ross, T. J., Amen, S. L., Sanfilipo, M., Hoffmann, R. G., et al. (2005). Neural correlates of high and craving during cocaine self-administration using BOLD fMRI. Neuroimage 26, 1097–1108. doi: 10.1016/j.neuroimage.2005.03.030
Rodríguez de Fonseca, F., Gorriti, M. A., Fernández-Ruiz, J. J., Palomo, T., and Ramos, J. A. (1994). Downregulation of rat brain cannabinoid binding sites after chronic delta 9-tetrahydrocannabinol treatment. Pharmacol. Biochem. Behav. 47, 33–40. doi: 10.1016/0091-3057(94)90108-2
Rogers, J., Siggins, G. R., Schulman, J. A., and Bloom, F. E. (1980). Physiological correlates of ethanol intoxication tolerance, and dependence in rat cerebellar Purkinje cells. Brain Res. 196, 183–198. doi: 10.1016/0006-8993(80)90725-8
Rogers, T. D., Dickson, P. E., Heck, D. H., Goldowitz, D., Mittleman, G., and Blaha, C. D. (2011). Connecting the dots of the cerebro-cerebellar role in cognitive function: neuronal pathways for cerebellar modulation of dopamine release in the prefrontal cortex. Synapse 65, 1204–1212. doi: 10.1002/syn.20960
Sacchetti, B., Baldi, E., Lorenzini, C. A., and Bucherelli, C. (2002). Differential contribution of some cortical sites to the formation of memory traces supporting fear conditioning. Exp. Brain Res. 146, 223–232. doi: 10.1007/s00221-002-1165-y
Sacchetti, B., Scelfo, B., and Strata, P. (2005). The cerebellum: synaptic changes and fear conditioning. Neuroscientist 11, 217–227. doi: 10.1177/1073858405276428
Schmahmann, J. D. (2019). The cerebellum and cognition. Neurosci. Lett. 688, 62–75. doi: 10.1016/j.neulet.2018.07.005
Schmahmann, J. D., and Sherman, J. C. (1998). The cerebellar cognitive affective syndrome. Brain 121, 561–579. doi: 10.1093/brain/121.4.561
Schneider, F., Habel, U., Wagner, M., Franke, P., Salloum, J. B., Shah, N. J., et al. (2001). Subcortical correlates of craving in recently abstinent alcoholic patients. Am. J. Psychiatry 158, 1075–1083. doi: 10.1176/appi.ajp.158.7.1075
Schweighofer, N., Doya, K., and Lay, F. (2001). Unsupervised learning of granule cell sparse codes enhances cerebellar adaptive control. Neuroscience 103, 35–50. doi: 10.1016/s0306-4522(00)00548-0
Silveri, M. C., Leggio, M. G., and Molinari, M. (1994). The cerebellum contributes to linguistic production: a case of agrammatic speech following a right cerebellar lesion. Neurology 44, 2047–2047. doi: 10.1212/WNL.44.11.2047
Slaker, M., Churchill, L., Todd, R. P., Blacktop, J. M., Zuloaga, D. G., Raber, J., et al. (2015). Removal of perineuronal nets in the medial prefrontal cortex impairs the acquisition and reconsolidation of a cocaine-induced conditioned place preference memory. J. Neurosci. 35, 4190–4202. doi: 10.1523/JNEUROSCI.3592-14.2015
Smith, K. S., and Graybiel, A. M. (2013). A dual operator view of habitual behavior reflecting cortical and striatal dynamics. Neuron 79, 361–374. doi: 10.1016/j.neuron.2013.05.038
Smolka, M. N., Buhler, M., Klein, S., Zimmermann, U., Mann, K., and Heinz, A. (2006). Severity of nicotine dependence modulates cue-induced brain activity in regions involved in motor preparation and imagery. Psychopharmacology 184, 577–588. doi: 10.1007/s00213-005-0080-x
Steinmetz, J. E., Lavond, D. G., and Thompson, R. F. (1989). Classical conditioning in rabbits using pontine nucleus stimulation as a conditioned stimulus and inferior olive stimulation as an unconditioned stimulus. Synapse 13, 225–233. doi: 10.1002/syn.890030308
Tessier, A., Cosin, C., Mayo, W., Pfeuty, M., Misdrahi, D., and Sibon, I. (2015). Impulsive aggressive obsessions following cerebellar strokes: a case study. J. Neurol. 262, 1775–1776. doi: 10.1007/s00415-015-7804-6
Tomasi, D., Wang, G. J., Wang, R., Caparelli, E. C., Logan, J., and Volkow, N. D. (2015). Overlapping patterns of brain activation to food and cocaine cues in cocaine abusers: association to striatal D2/D3 receptors. Hum. Brain Mapp. 36, 120–136. doi: 10.1002/hbm.22617
Vazquez-Sanroman, D., Carbo-Gas, M., Leto, K., Cerezo-Garcia, M., Gil-Miravet, I., Sanchis-Segura, C., et al. (2015a). Cocaine-induced plasticity in the cerebellum of sensitised mice. Psychopharmacology 232, 4455–4467. doi: 10.1007/s00213-015-4072-1
Vazquez-Sanroman, D., Leto, K., Cerezo-Garcia, M., Carbo-Gas, M., Sanchis-Segura, C., Carulli, D., et al. (2015b). The cerebellum on cocaine: plasticity and metaplasticity. Addict. Biol. 20, 941–955. doi: 10.1111/adb.12223
Vivian, T. N., Goldstein, G., and Shelly, C. (1973). Reaction time and motor speed in chronic alcoholics. Percept. Mot. Skills. 36, 136–138. doi: 10.2466/pms.1973.36.1.136
Volkow, N. D., Wang, G. J., Hitzemann, R., Fowler, J. S., Wolf, A. P., Pappas, N., et al. (1993). Decreased cerebral response to inhibitory neurotransmission in alcoholics. Am. J. Psychiatry. 150, 417–422. doi: 10.1176/ajp.150.3.417
Voogd, J., and Ruigrok, T. J. H. (2004). The organization of the corticonuclear and olivocerebellar climbing fiber projections to the rat cerebellar vermis: the congruence of projection zones and the zebrin pattern. J. Neurocytol. 33, 5–21. doi: 10.1023/B:NEUR.0000029645.72074.2b
Wagner, M. J., Kim, T. H., Savall, J., Schnitzer, M. J., and Luo, L. (2017). Cerebellar granule cells encode the expectation of reward. Nature 544, 96–100. doi: 10.1038/nature21726
Wang, O., Lee, S. W., O’Doherty, J., and Seymur, B. (2018). Model-based and model-free pain avoidance learning. Brain Neurosci. Adv. 2, 1–8. doi: 10.1177/2398212818772964
Watabe-Uchida, M., Zhu, L., Ogawa, S. K., Vamanrao, A., and Uchida, N. (2012). Whole-brain mapping of direct inputs to midbrain dopamine neurons. Neuron 74, 858–873. doi: 10.1016/j.neuron.2012.03.017
Watson, P., van Wingen, G., and de Wit, S. (2018). Conflicted between goal-directed and habitual control – an fMRI investigation. eNeuro 5, 1–11. doi: 10.1523/ENEURO.0240-18.2018
Watson, T. C., and Apps, R. (2019). “Cerebro-cerebellar connections,” in Handbook of the Cerebellum and Cerebellar Disorders, eds M. Manto, D. Gruol, J. Schmahmann, N. Koibuchi, and R. Sillitoe (Cham: Springer), doi: 10.1007/978-3-319-97911-3_48-3
Watson, T. C., Becker, N., Apps, R., and Jones, M. W. (2014). Back to front: cerebellar connections and interactions with the prefrontal cortex. Front. Syst. Neurosci. 8:4. doi: 10.3389/fnsys.2014.00004
Xiao, L., Bornmann, C., Hatstatt-Burklé, L., and Scheiffele, P. (2018). Regulation of striatal cells and goal-directed behavior by cerebellar outputs. Nat Commun. 9:3133. doi: 10.1038/s41467-018-05565-y
Yin, H. H., and Knowlton, B. J. (2006). The role of the basal ganglia in habit formation. Nat. Rev. Neurosci. 7, 464–476. doi: 10.1038/nrn1919
Keywords: cerebellum, drug addiction, prefrontal cortex, ventral tegmental area, striatum, goal-directed behavior, habit
Citation: Miquel M, Gil-Miravet I and Guarque-Chabrera J (2020) The Cerebellum on Cocaine. Front. Syst. Neurosci. 14:586574. doi: 10.3389/fnsys.2020.586574
Received: 23 July 2020; Accepted: 29 September 2020;
Published: 21 October 2020.
Edited by:
Natalie M. Zahr, Stanford University, United StatesReviewed by:
Sandra Chanraud, Université de Sciences Lettres de Paris, FranceJorge Medina, University of Buenos Aires, Argentina
Copyright © 2020 Miquel, Gil-Miravet and Guarque-Chabrera. This is an open-access article distributed under the terms of the Creative Commons Attribution License (CC BY). The use, distribution or reproduction in other forums is permitted, provided the original author(s) and the copyright owner(s) are credited and that the original publication in this journal is cited, in accordance with accepted academic practice. No use, distribution or reproduction is permitted which does not comply with these terms.
*Correspondence: Marta Miquel, bWlxdWVsQHVqaS5lcw==; orcid.org/0000-0001-9670-4210