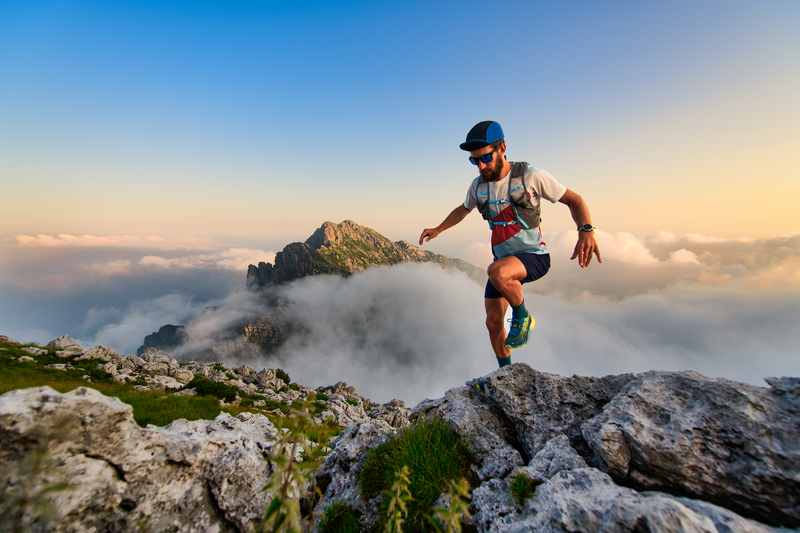
95% of researchers rate our articles as excellent or good
Learn more about the work of our research integrity team to safeguard the quality of each article we publish.
Find out more
REVIEW article
Front. Syst. Neurosci. , 20 October 2020
Volume 14 - 2020 | https://doi.org/10.3389/fnsys.2020.582985
This article is part of the Research Topic Brain Modifications in Response to Stress: From Cellular to Circuit Reorganization View all 5 articles
Parts of this article's content have been modified or rectified in:
Erratum: Stress Varies Along the Social Density Continuum
Social stress is ubiquitous in the lives of social animals. While significant research has aimed to understand the specific forms of stress imparted by particular social interactions, less attention has been paid to understanding the behavioral effects and neural underpinnings of stress produced by the presence and magnitude of social interactions. However, in humans and rodents alike, chronically low and chronically high rates of social interaction are associated with a suite of mental health issues, suggesting the need for further research. Here, we review literature examining the behavioral and neurobiological findings associated with changing social density, focusing on research on chronic social isolation and chronic social crowding in rodent models, and synthesize findings in the context of the continuum of social density that can be experienced by social animals. Through this synthesis, we aim to both summarize the state of the field and describe promising avenues for future research that would more clearly define the broad effects of social interaction on the brain and behavior in mammals.
Stress is a fascinating topic of research, largely due to the variable and enigmatic manner in which stress manifests itself across species and between individual animals. Stress can have context- and experience-dependent effects, and it is both essential and detrimental. Drawing from a rich history of stress research, there has been a theoretical formulation of stress as existing on a continuum, with an optimum level of stress positioned between under- and overstimulation (Selye, 1976; Sapolsky, 2015). In line with this, it can be considered that a single stress-inducing stimulus may exist on a continuum, producing a roughly bell-shaped curve, wherein an optimum magnitude of the stimulus could provide a benefit to the individual while either extremely low or high levels impart costs. Here, we apply this theoretical model to the understanding of psychogenic stress in response to social density (Figures 1, 2).
Figure 1. The social density continuum. As the magnitude of social density rises, different forms of stress occur in a population. (A) Through the action of social density-related stress, a curve-shaped upper bound for possible fitness states exists within a typical population. At species-typical levels of social density, individuals in a population may experience fitness states ranging from low (red) to high (green), while at extreme levels of social density, only low fitness is possible. (B) The shape of the bounding curve for possible states in social density-fitness space can be expected to vary based on taxon-specific features of the social system such as degree of social cohesion and mating system, as in these hypothetical examples. Hash marks show species-typical social density levels. Top panel shows an example for a species that achieves maximum fitness at comparatively low social density (i.e., social organization tends toward small social groups); bottom panel shows an example for a species that achieves maximum fitness at comparatively high social density (e.g., taxa that benefit from large social groups). Species, including model taxa, which differ in social organization can be expected to be comparatively more- or less- tolerant of extremes in social density.
Figure 2. Proportional prevalence of social stress varies with social density. Curves show the proposed general effect of SIS and CS on the proportional prevalence of stress that is induced by the social environment and social activities in a population. At species-typical levels of social density, SIS and CS occur infrequently. At levels of social density far from species-typical values, greater proportions of the population will experience social stress via the action of SIS and CS. Individuals within a population will vary in their resilience to extreme levels of social density, thus social stress may not reach complete saturation.
“Social density” as we use it here refers to the average conspecific encounter rate in a population of animals. While social density could be defined through numerous approaches that variably include measures of physical space, we advocate for a framework that emphasizes the social environment by quantifying the average number of unique social encounters in a given population that occur per unit of time, a concept equivalent to the average degree of a network (Figure 3). In this way, physical area and resource availability of the habitat are indirectly related to social density.
Figure 3. Network illustrations depicting hypothetical social density across housing schemes evaluated over 1 day. (A) In a typical laboratory scheme, mice are housed 4 per cage, and each mouse encounters every other mouse every day. Here, 12 unique social encounters occur per day among 4 animals, equating to a social density of 3.0 encounters per individual per day. (B) In a more densely populated housing scheme of 8 mice per cage, social density would be calculated as 56 encounters per day among 8 animals, equating to a social density of 7.0 encounters per individual per day. (C) In more complex environments or with higher population numbers, all members of a population are less likely to come into social contact with all other members every day, and so accurately calculating actual average social density requires more detailed data (e.g., 70 unique encounters among 10 individuals, social density again equals 7.0, as shown).
Under species-typical levels of social density, social stress occurs. However, this stress is likely to be well-tolerated, perhaps even an integral part of the social system which has evolved alongside the taxon in question. In such situations, neural regulation of the stress response typically operates under conditions to which it is well-equipped to handle. In humans, social stress can be triggered by interpersonal encounters, argument, and fighting. Similar patterns exist in rodent models, where social stress is often studied in the context of mating, aggression, and the establishment of social hierarchy. Each of these behaviors can be enhanced at the individual level by regulation of the HPA axis (Korte et al., 2005). Stressors in this category are often regular occurrences in the lives of animals, and they have the ability to adaptively guide behavioral development. However, when allostatic load, the strain on the body due to the physiological response to stress (McEwen and Stellar, 1993), is chronically high, such stressors can inflict severe and persistent abnormal behavioral syndromes (McEwen, 2000; McEwen and Wingfield, 2003; Tamashiro et al., 2005; Beery et al., 2020). Through allostasis, such social stressors together contribute to an overall increase in fitness, keeping an individual on track for success in its social environment (McEwen, 2000; Korte et al., 2005).
In contrast to moderate forms of social density and stress, mammals respond poorly to extremes in social abundance and density. In comparison to moderate levels, extremely low or high social density may constitute a situation for which the nervous system is not tuned to efficiently handle, especially when administered at chronic levels. Stress associated with such extremes, namely social isolation stress (SIS) and social crowding stress (CS), are well-known to induce chronic stress in animals (Gamallo et al., 1986; Hilakivi et al., 1989; Djordjević et al., 2003; Weiss et al., 2004; Lin et al., 2015). Indeed, these manipulations are some of the few that are truly chronic stressors, in that there is no reprieve from the stressor at any point (Zelikowsky et al., 2018a). In contrast, other models of chronic stress administer acute forms of stress repeatedly over time (e.g., social defeat; Berton et al., 2006; Keeney et al., 2006; Golden et al., 2011). Extended periods of continuous social isolation are known to induce an array of negative behaviors in humans and animal models, and the neural mechanisms that facilitate this change in behavior have recently been exciting topics of research. Chronic social crowding has been observed to induce other changes in behavior in several model systems, but less attention has been paid to understanding the neurobiological underpinnings of this form of stress. Importantly, both forms of extreme social density stress occur across the animal kingdom.
In society today, understanding the effects of variation in social density on the brain and behavior has become increasingly important. During the COVID-19 pandemic, many around the world are (at time of writing) confined to their own spaces for extended periods of time in order to reduce viral transmission (Anderson et al., 2020; Armitage and Nellums, 2020; He et al., 2020). In juxtaposition, major population centers have experienced historically high population growth rates that have led to dense living conditions for many others (Taylor, 2006; Buhaug and Urdal, 2013). To understand the way in which variation in the abundance and density of social interactions, irrespective of their specifics, directly influences the workings of the brain is an important goal for future research. Here, we review research on social stress, focusing on extremes in social abundance and density in rodent models.
An Allee effect is any mechanism that leads to a positive relationship between some individual fitness measure and the number or density of conspecifics in a population (Odum and Allee, 1954; Stephens and Sutherland, 1999; Stephens et al., 1999; Angulo et al., 2018). In this review, we describe how social-density-induced stress constitutes a mechanism through which individual fitness is contingent on population size and social density. Individual fitness is reduced by SIS and CS at extremely low and extremely high population densities relative to fitness at moderate population densities. Thus, this describes behaviorally-mediated positive- and negative-density dependence, including a de-facto Allee effect mechanism. With this acknowledgment, ecology may be able to inform future work in neurobiology and vice-versa.
Social species are defined by their conspecific interactions, which are often multidimensional, complex, and pervasive across lifetimes. Denied exposure to conspecific peers, social animals will behave abnormally. Research on social isolation can be divided into two broad categories based upon whether it focuses on the effect of isolation during development or during adulthood.
Social isolation during early development has been shown to impart an anxiety-like phenotype and other behavioral changes in adult rodents (for review see Lapiz et al., 2003; Fone and Porkess, 2008; Mumtaz et al., 2018). Among the behaviors that are altered, many are social in nature. For example, one study found that developmentally-isolated male mice showed a decrease in adult social competence characterized by altered ultrasonic vocalizations (USVs) and increased male-male mounting behavior (Keesom et al., 2017). Another study found that developmentally isolated adult female mice take longer than socially-housed mice to learn an operant task, though after training they did not show reduced performance on a USV discrimination task (Screven and Dent, 2019). Notably, one study suggests that the effects of adolescent social isolation may be ameliorated by partial indirect social housing, holding promise for future study (Pais et al., 2019).
Focused investigations have been made to determine the neural mechanisms underlying these behavioral changes. In adolescent mice that have been socially isolated, differences arise in the network organization of the structural connectome relative to controls, such that measures of network structure such as modularity (the strength of the division of a network into modules) and small-worldness (the degree to which a network is organized into clusters) decrease, indicating greater homogeneity of connections (Liu et al., 2016). In particular, these alterations hinged on disruption of inter-hemispheric and inter-modular connections of the dorsolateral orbitofrontal cortex (Liu et al., 2016). In adulthood, studies have found developmental social isolation to be associated with reduced myelination, altered dendritic spine development, and reduced plasticity in the pre-frontal cortex [PFC; (Makinodan et al., 2012; Medendorp et al., 2018)], and changes in PFC connectivity (Hermes et al., 2011), especially regarding the circuit between the PFC and amygdala (Castillo-Gómez et al., 2017). Serotonergic fiber density in the inferior colliculus has also been shown to be reduced by developmental isolation (Keesom et al., 2018).
Social isolation also has detrimental effects when experienced during adulthood. This is especially abundant in the elderly and disabled populations, but can occur at any phase of adulthood (Hawkley and Cacioppo, 2010). Adult chronic social isolation is associated with increased risk of death (House et al., 1988) and a suite of mental health issues in humans (Cacioppo et al., 2011). For these reasons, studies of chronic social isolation in model systems are apt to provide relevance for translation to humans.
Adult chronic SIS has been shown to induce a phenotype sharing characteristics with anxiety, depression, and social withdrawal in rats and mice (Scaccianoce et al., 2006; Liu et al., 2012; Ieraci et al., 2016), especially characterized by increased aggression (Allee, 1942; Valzelli, 1973). Given these findings, attempts have been made to dissect the neurobiological correlates of SIS-induced behavioral changes.
SIS is notable in that, despite strongly impacting behavior, it does not appear to increase plasma corticosterone (CORT) or brain monamines such as noradrenaline and dopamine, which are induced by other stressors (Hilakivi et al., 1989; Scaccianoce et al., 2006). For example, one study found reductions in allopregnanolone and 5a-dihydroprogesterne in SIS mice (Dong et al., 2001), and another found significant reductions in plasma corticosterone (Ieraci et al., 2016). Notably, this effect seems to differ across sexes: females exhibit higher CORT in isolation, while males undergoing the same conditions exhibit a mean reduction in plasma CORT (Brown and Grunberg, 1995).
Rather than showing more typical signs of acute stress, SIS has been associated with several neural changes. Included among these changes is decreased myelination in the PFC (Liu et al., 2012), a reduction of BDNF, mGluR1, and mGluR2 in the PFC, and lower immediate early gene expression in the PFC and hippocampus (Ieraci et al., 2016). Importantly, we recently discovered that SIS is accompanied by a brain-wide upregulation of the neuropeptide Tachykinin 2 (Tac2) (Zelikowsky et al., 2018b). Moreover, our data reveal dissociable roles for Tac2 signaling in the anterior dorsal bed nucleus of the stria terminalis (dBNSTa), dorsomedial hypothalamus (DMH), and the central amygdala (CeA) in the control of isolation-induced persistent fear, enhanced aggression, and acute fear, respectively. These data suggest that Tac2 exerts parallel action in multiple brain structures to control SIS and identify a key molecule in the control of social isolation (Figure 4).
Figure 4. Tac2 exerts parallel action in multiple brain structures to influence behaviors associated with SIS. Adapted from Zelikowsky et al. (2018b).
Another key factor determining the extent to which social isolation is detrimental is the degree and duration in which an animal is isolated. For example, one study found that isolation for 2–5 days was associated with a performance increase in the forced swim test characterized by reduced immobility time, but isolation for 10–20 days was not (Hilakivi et al., 1989). In the same vein, a study of the effects of 24 h social isolation found that mice prefer to interact with a novel mouse after this short period of isolation (Matthews et al., 2016), which contrasts with the reduction in social interaction seen following longer, chronic forms of social isolation (Zelikowsky et al., 2018b).
The relationship between chronicity of social isolation and negative effects on the brain and behavior may also be evident in humans. While the negative feelings associated with chronic loneliness are well-studied (Hawkley and Cacioppo, 2010), research that investigates potential benefits of time spent alone is less common. Still, reports suggest that time spent alone can be emotionally beneficial, bringing feelings of freedom, creativity, and intimacy (for review see Long and Averill, 2003). One study found that humans experiencing brief social isolation felt increased high arousal negative affect and heightened salivary cortisol, as expected if isolation is detrimental, but also that these changes in high arousal affect were associated with a simultaneous increase in low arousal positive affect (Pauly et al., 2017). Importantly, this relationship differed with age: adults over 68 years old did not show increased cortisol with brief social isolation while younger groups did (Pauly et al., 2017). Similarly, another study found social isolation to have a detrimental effect on high-arousal affects but a restorative influence on low-arousal positive affect (Nguyen et al., 2018). That same study found that choosing to be alone in an unrestricted environment dramatically decreased measures of experienced stress, increased feelings of relaxation, and heightened high-arousal positive affect (Nguyen et al., 2018). With these findings in mind, defining the context- and chronicity- dependent role of social isolation on human health can be expected to be an important line of future research.
When a population's size increases, changes in individuals' behaviors will arise as a result. For example, crowding produces a stressful increase in competition for limited resources, and increased aggression and territoriality are maintained in the offspring of crowded populations (Agrell et al., 1995). Early research has focused on determining the existence of individual-level population control mechanisms which might be manifested in behavioral change. Such mechanisms were hypothesized to be resource-independent and triggered by high social density. One line of study found that male mouse adrenal gland weight increased with increasing population density, presumably indicating an increase in adrenal function, and that the weight of the pituitary gland, seminal vesicles, and testes declines with increasing social density, suggesting decreased secretion of testicular androgens (Christian, 1961). Importantly, these findings also suggest that a greater absolute number of individuals in a population will trigger the observed changes regardless of the amount of space that population occupies (Christian, 1961).
Another line of early research used mice in semi-natural environments to evaluate the effects of density on behavior (Marsden, 1972). This research showed that a number of abnormal behavioral phenotypes emerged with increased population density among both males and females and that the emergence of these phenotypes was associated with a decline in typical behaviors found under less crowded conditions. At the highest population densities, phenotypes were characterized by extreme social withdrawal. This phenotype continued even after reintroduction of such individuals to non-crowded populations of mice raised under typical densities (Marsden, 1972).
Whether or not an adaptive explanation exists for the mechanisms at work, there appear to be significant behavioral and endocrine effects of social crowding occurring both during development and in adulthood. One study found that crowding during early development does not immediately increase CORT, but that corticoadrenal responses to acute stress (startle and forced swim) were increased compared to non-crowded mice (Ortiz et al., 1985). Another study found effects of crowding on nociception regulation in developmentally-crowded mice, but their control condition was social isolation, rendering interpretation of their results difficult in the current context (Reiss et al., 2007). Interestingly, it appears that limited developmental crowding can be beneficial—not harmful—to mice, since one study found that nighttime crowding of adolescent mice reduces anxiety-associated behaviors (Ago et al., 2014). This finding that a degree of crowding is net-positive for the individual again bolsters the conceptualization of social density stress as a continuum and highlights the importance of chronicity in mediating the negative effects of crowding or isolation.
In adulthood, the results of studies of social crowding stress sometimes conflict. In rats, crowding has been shown to induce increased CORT (Gamallo et al., 1986; Djordjević et al., 2003), but another study found that effects of crowding are sex-specific: females show no change in CORT between group-housed and crowded conditions, while males show a marked increase in CORT with crowding (Brown and Grunberg, 1995). In contrast to the above studies that find increased response to acute stress by developmentally-crowded mice, a series of studies found that crowding in adult rats significantly reduces HPA responsiveness to noradrenaline (Bugajski et al., 1994). Much less work on crowding has been done in mice than in rats, but one study found that crowding induced increased adiposity (but not weight gain) and mild anxiety-like behavior (but no increase in depression-like tendencies). Importantly, this study also found gene expression changes in the hypothalamus (Lin et al., 2015).
Fear less work (or research) has been conducted on the neural effects of CS than on other forms of social stress, with past research being primarily focused on behavior and the neuroendocrine response. Future research in this arena is encouraged. Such studies would help refine our understanding of the behavioral and neural impacts of social crowding stress and seek unifying explanations for these findings.
In comparison to extremes in social density, species-typical social densities facilitate an increase in fitness (Lacey and Sherman, 2007; Silk, 2007). Indeed, empirical and theoretical work portrays social stressors at typical social density as being net-positive for the individual when they are administered acutely, contributing positively to adaptive social dynamics (McEwen, 2000; McEwen and Wingfield, 2003; Korte et al., 2005). Social stress at typical densities is a well-studied subject that has been reviewed thoroughly and recently (Tamashiro et al., 2005; Beery et al., 2020). Briefly, animals in conspecific groups experience social stress in the context of interactions such as fighting, courtship, and territory defense. These stressors are often fleeting or avoidable and vary in their abundance and magnitude across time. When administered chronically, these forms of stress can induce behavioral alterations [see literature on chronic social defeat (Berton et al., 2006; Keeney et al., 2006; Golden et al., 2011) and allostatic overload (McEwen and Wingfield, 2003)], but chronic loads are infrequent in average natural populations. Rather, a suite of theoretical and empirical studies have established that such social stressors facilitate the establishment of taxon-specific social structures and social dynamics that together exert a positive influence on individual fitness (for review see Korte et al., 2005).
Social taxa differ in their typical social density. Mus musculus and Rattus norvegicus, the two species from which laboratory strains of the common mouse and rat models are derived, have highly flexible social systems which allow living in up to 1,000 times higher densities when resource availability is high than when resources are sparsely distributed (Berdoy and Drickamer, 2007). In these taxa, the tendency to live in social groups varies along with population density (Berdoy and Drickamer, 2007), thus, realized individual-level social density of wild populations varies dramatically. Humans, on the other hand, have been described as having evolved to live in tightly-knit social and kin groups (Hawkes et al., 1998; Kaplan et al., 2009). Though a degree of variation occurs, deviation from the group-living habit amongst our own species is infrequent (Boyd et al., 2012). The social density thresholds and phenotypic effects associated with the onset of SIS and CS are likely to show interspecific variation that scales with both typical social density and the flexibility of the taxon-specific social organization (Figure 1B). Accounting for this variation in taxon-specific typical social density should be a priority for future research, as should identifying the possible existence of rules that govern the relationship between SIS and CS social density thresholds and species-typical social density. Indeed, the alignment between individual-level social density stress thresholds in humans and those in model taxa could hold relevance for the direction of research in the field.
Understanding social stress at typical densities may partially explain findings of SIS research. For example, individual differences in hierarchy position could introduce individual variation in the response to SIS. When group housed, both male and female mice form social hierarchies (Van Den Berg et al., 2015; Varholick et al., 2019; Williamson et al., 2019), and top-ranking positions are associated with reduced plasma CORT, decreased initiation of aggression, and increased reception of aggression (Louch and Higginbotham, 1967; Williamson et al., 2019; but see cases where CORT in top-ranking positions does not differ from that of lower ranks: Schoech et al., 1997; Pravosudov et al., 2003; Poisbleau et al., 2005). Hierarchy formation is typically associated with increased stress for all members, which can be reduced after a hierarchy becomes established (Beery et al., 2020). Results of studies that find changes in stress under social isolation may be clarified by accounting for social rank of individuals. In other words, short-term social isolation may be a relief for individuals that had a high-stress rank in group housing, while individuals from low-stress ranks may experience an increase in stress when moved from group to isolation housing.
In addition, the increased aggression widely observed after social reintroduction of SIS-treated mice (Valzelli, 1973) may be explained by the innate territoriality of male mice. Mice and rats have flexible social systems. In most wild populations, male mice hold territories and defend them from other males (Berdoy and Drickamer, 2007). This territorial aggression is reduced in forced group housing, where social hierarchies form, but subordinate males increase aggression when more space is made available in apparent attempts to establish territory (Beery et al., 2020). When a male is moved into isolation, it may be that that animal establishes itself as the dominant territory holder. When it is moved back into group housing, increased aggression may reflect the animal's newfound de-facto territorial dominance. In this way, a behavioral change widely reported as being atypical or maladaptive may be readily explained by the workings of the social system of mice. Understanding the effects of SIS on the brain and behavior in the context of the social system of the model organism should inform future research in the field.
While more work is necessary to form strong conclusions from the body of work on CS, the combined results may be explained by the possibility that crowding amplifies or exaggerates individual-level social stresses that occur under typical group-housed conditions. It has been suggested that disrupting social hierarchy can cause reduced neurogenesis in the hippocampus and altered social preferences via a preference for known over novel conspecifics (Opendak et al., 2016). Chronic social disruption has also been shown to induce anxiety and abnormal social behavior, which can be vertically inherited (Saavedra-Rodríguez and Feig, 2013). It is possible that social crowding could disrupt typical hierarchical organization by overloading the social mechanisms by which it forms, thereby inducing atypical social behavior and associated effects on the brain of group members. While this possibility deserves further testing, some support is garnered from past work. For example, one study which found that reducing group size increased overall hierarchy stability and decreased the time to hierarchy establishment in rats (Becker and Flaherty, 1968). Another found that angelfish hierarchies are less stable at higher group sizes (Ang and Manica, 2010). In addition, theoretical work has shown that increased group sizes decreases the likelihood of linear hierarchies (Mesterton-Gibbons and Dugatkin, 1995). It could also be argued that SIS constitutes de-facto hierarchy disruption, so some of the observations of socially isolated animals could be products of an active disruption, rather than of passive hierarchy absence.
Further behavioral examination of stress under typical social densities to explore these and other possibilities should prove very fruitful toward building a more complete understanding of the way that the brain and behavior respond to variation in social density. Additionally, focusing research efforts on common evolutionarily and ecologically important behaviors will likely be informative for stress research in model systems, as has been witnessed with the influential formulation and use of the predatory imminence scale in research on memory and fear (Fanselow and Lester, 1988; Perusini and Fanselow, 2015). For example, clarifying the behavioral algorithms (Hein et al., 2020) and neural mechanisms that guide such common behaviors as mating and foraging (Kolling et al., 2012) will be likely to contextualize findings of research on SIS and CS, stressful conditions under which these common important behaviors are disrupted.
In social animals, there is a relationship between social density and behavior such that moderate levels of density are beneficial and extreme levels of density are detrimental to an animal's behavior (Figure 1). While some individuals may experience heightened stress and reduced fitness under species-typical social density, the population average fitness will be higher under moderate than under extreme social density. In this framework, social density functions as a stressor, with extremely high and low levels of social density eliciting stress of different forms. Defining this curve for different social species will be an important goal of future research with interest in translation to human health—the shape of this curve for taxa with social systems most similar to our own may provide interesting application to our own lives and to the development of treatments for injuries inflicted by social density-associated stress.
As with any discussion of stress, it is important to recognize that “stress” has had many definitions over the years. Here, we have considered the potential effects of social density-related stress not only as any HPA-axis regulated neuroendocrine stress response and its dysregulation, but also as the behavioral and neural changes that could be either primary or secondary responses to psychogenic stress. Furthermore, we discuss SIS and CS as imparting limits on fitness in a population. To what degree these limits are the result of direct or indirect effects of stress is an important consideration, and disentangling cause from effect regarding the stress-phenotype components and population-level effects described in this review will be an interesting direction for future research.
While we use “social density” to refer to population-level average social encounter rate (Figure 3), social density also manifests at the individual level. In typical laboratory settings, social density is strictly controlled, and each individual is likely to experience the same realized social density. In natural populations, however, individuals have the potential to experience radically different social density, and this variation can be amplified by increased habitat complexity and the social system. Heterogeneity in the environment is likely to reduce the encounter rate among population members and increase individual variation in realized social density. Varying degrees of social group cohesion and other features of the social system can similarly contribute to increased variation in realized social density of individuals in a population. It follows that with this increased variation comes altered population-average social density and an increased likelihood for individuals to experience extremes in social density, along with SIS and CS.
We suggest a definition of social density that should provide utility to the field, but which relies on quantifying social “encounters,” the definition of which is also subject pitfalls. We propose considering an encounter to be any detection of a conspecific by an individual. While identification of encounters is made more difficult in taxa that interact via communicative modes that are relatively difficult to track (e.g., ultrasonic vocalizations, odors in rodents, etc.), opportunities abound for research into the neural and behavioral effects of social density experienced at different levels, in different contexts, and through different modes. Work that incorporates network science approaches to understand the effects of social density and social connectivity on the brain and behavior are likely to significantly advance the field (e.g., So et al., 2015; Williamson et al., 2016).
It should be recognized that effects of social isolation and social crowding have also been recorded in non-mammalian social species, including in insects (Wang et al., 2008; Lihoreau et al., 2009; Ueda and Wu, 2009; Stevenson and Rillich, 2013), fish (Halperin and Dunham, 1993; Brandão et al., 2015; Shams et al., 2015), and birds (Apfelbeck and Raess, 2008; Banerjee and Adkins-Regan, 2011). Notably, a recent study found immediate effects of social isolation in the expression of several immediate early genes in the forebrain of an avian species (George et al., 2019), a finding which has implications for the field of vocal learning, which historically relies on the isolation of animals (Love et al., 2019). It is difficult to determine at present how much of the human response to social density stressors is unique to humans; do we find similar responses among distantly-related taxa that show similarity in social systems, or does genetic relatedness play a more important role in determining how the CNS modulates behavior in response to social density? If the latter, then how evolutionarily conserved are the neural mechanisms that affect behavior in response to social density stress? Detailed investigations into these questions using powerful techniques under a behavior-first paradigm are likely to yield important insights into the specific neural mechanisms that are involved with extreme social density stress. Taking inspiration from the comparative study of the neural bases for other behaviors (Bullock, 1984; O'Connell and Hofmann, 2011a,b, 2012; Dulac et al., 2014; Barkan et al., 2017; Nieder, 2018; Paolino et al., 2020) and leveraging the abundant variation in social structure in diverse animal clades, future evolutionary comparative behavioral neuroscience research would likely be particularly fruitful to that end.
If SIS- and CS-induced behavioral changes are deeply evolutionarily conserved, then the abundant research on positive and negative density dependence could be informative for understanding these altered behavioral states and formulating hypotheses for future research. The combined behavioral changes related to SIS and CS are manifestations of the Allee effect and negative density dependence (Stephens and Sutherland, 1999; Stephens et al., 1999; Angulo et al., 2018). It is critical to point out that both SIS and CS differentiate themselves from many other forms of stress in that their effects on behavior are maintained for the remaining lifetime of the animal that experiences them and are thought to decrease fitness by altering courtship and mating behavior (Christian, 1961; Marsden, 1972). In this way, SIS and CS combine to create substantial density dependence, which would not exist if the effects of these stressors were more fleeting. While this recognition may appear to some as simple semantics, it is important to indicate the potential for neuroscience to investigate the neural substrates for evolutionarily and ecologically important behaviors (Krakauer et al., 2017). Likewise, research on the behavioral modification undergone during extremes in population density will be informative for building more accurate predictive models of population dynamics.
There is some evidence that short periods of social isolation may well be important for human mental health. Seeking solitude is a common experience for many (Long and Averill, 2003), and it has been proposed that a balance between aloneness and relatedness is pivotal to human health (Detrixhe et al., 2014). These observations may seem at odds with the often negative social connotations of being alone. However, such conflicting results regarding the emotional response to being alone may be explained by conditionality and context-dependency. Humans can be alone but not feel lonely, and they can feel lonely even though they are with others (Hawkley and Cacioppo, 2010). The difference between these mental states—uncomfortable loneliness vs. comforting solitude—is profound, given that they occur under the same social environment. The well-studied effects of social isolation on mental health in humans hinges on the prominence of chronic loneliness, not chronic solitude. In order to draw more accurate translation to humans, findings from model systems must be contextualized with these important results in mind. Indeed, we currently know very little about how periodic crowding and isolation may affect the brain and behavior of social animals. Future work on SIS that could characterize the emotional context- and dose-dependency of social isolation in terms of perceived solitude and perceived loneliness would constitute a meaningful addition to the field.
It may not be surprising that extremes of social density—those to which a given social species is not well-adapted—elicit abnormal behavior and neural activity respective to that typical of the species under normal social circumstances. Nonetheless, these models do allow for a simple manipulation to be used during powerful experimental investigation of the neural correlates of relevant behavior, and therefore may provide a fruitful system for understanding the organization of the brain. Furthermore, it seems remarkable that these forms of stress have the potential to induce lasting injury, their effects lingering long after an animal is returned to typical conditions. With an interest in human health, we must ask “why?” and “how?” these behavioral disorders arise. Further integrative study of social density stress resulting in a definition of the shape of the stress curve will allow for the identification of the levels at which these injuries become more likely to occur. With that information, we may be closer to being able to recognize the specific mechanisms by which these stress-induced disorders arise and, perhaps, prevent them.
All authors listed have made a substantial, direct and intellectual contribution to the work, and approved it for publication.
This work was supported by an NIMH award R00MH108734 (MZ), a Sloan Research Fellowship (MZ), and a Klingenstein-Simons Fellowship (MZ).
The authors declare that the research was conducted in the absence of any commercial or financial relationships that could be construed as a potential conflict of interest.
Ago, Y., Tanaka, T., Ota, Y., Kitamoto, M., Imoto, E., Takuma, K., et al. (2014). Social crowding in the night-time reduces an anxiety-like behavior and increases social interaction in adolescent mice. Behav. Brain Res. 270, 37–46. doi: 10.1016/j.bbr.2014.04.047
Agrell, J., Erlinge, S., Nelson, J., Nilsson, C., and Persson, I. (1995). Delayed density-dependence in a small-rodent population. Proc. R. Soc. B 262, 65–70. doi: 10.1098/rspb.1995.0177
Allee, W. (1942). Group organization among vertebrates. Science 95, 289–293. doi: 10.1126/science.95.2464.289
Anderson, R. M., Heesterbeek, H., Klinkenberg, D., and Hollingsworth, T. D. (2020). How will country-based mitigation measures influence the course of the COVID-19 epidemic? Lancet 395, 931–934. doi: 10.1016/S0140-6736(20)30567-5
Ang, T. Z., and Manica, A. (2010). Unavoidable limits on group size in a body size-based linear hierarchy. Behav. Ecol. 21, 819–825. doi: 10.1093/beheco/arq062
Angulo, E., Luque, G. M., Gregory, S. D., Wenzel, J. W., Bessa-Gomes, C., Berec, L., et al. (2018). Review: allee effects in social species. J. Anim. Ecol. 87, 47–58. doi: 10.1111/1365-2656.12759
Apfelbeck, B., and Raess, M. (2008). Behavioural and hormonal effects of social isolation and neophobia in a gregarious bird species, the European starling (Sturnus vulgaris). Horm. Behav. 54, 435–441. doi: 10.1016/j.yhbeh.2008.04.003
Armitage, R., and Nellums, L. B. (2020). COVID-19 and the consequences of isolating the elderly. Lancet Public Healh 5:e256. doi: 10.1016/S2468-2667(20)30061-X
Banerjee, S. B., and Adkins-Regan, E. (2011). Effect of isolation and conspecific presence in a novel environment on corticosterone concentrations in a social avian species, the zebra finch (Taeniopygia guttata). Horm. Behav. 60, 233–238. doi: 10.1016/j.yhbeh.2011.05.011
Barkan, C. L., Zornik, E., and Kelley, D. B. (2017). Evolution of vocal patterns: tuning hindbrain circuits during species divergence. J. Exp. Biol. 220, 856–867. doi: 10.1242/jeb.146845
Becker, G., and Flaherty, T. B. (1968). Group size as a determinant of dominance-hierarchy stability in the rat. J. Comp. Physiol. Psychol. 66, 473–476. doi: 10.1037/h0026371
Beery, A. K., Holmes, M. M., Lee, W., and Curley, J. P. (2020). Stress in groups: lessons from non-traditional rodent species and housing models. Neurosci. Biobehav. Rev. 113, 354–372. doi: 10.1016/j.neubiorev.2020.03.033
Berdoy, M., and Drickamer, L. C. (2007). “Comparative social organization and life history of rattus and mus,” in Rodent societies: An Ecological & Evolutionary Perspective, eds P. W. Sherman and J. O. Wolff (Chicago, IL: The University of Chicago Press), 380–392.
Berton, O., McClung, C. A., DiLeone, R. J., Krishnan, V., Renthal, W., Russo, S. J., et al. (2006). Essential role of BDNF in the mesolimbic dopamine pathway in social defeat stress. Science 311, 864–868. doi: 10.1126/science.1120972
Boyd, I., Rubin, G. J., and Wessely, S. (2012). Taking refuge from modernity: 21st century hermits. J. R. Soc. Med. Suppl. 105, 523–529. doi: 10.1258/jrsm.2012.120060
Brandão, M. L., Braithwaite, V. A., and Gonçalves-de-Freitas, E. (2015). Isolation impairs cognition in a social fish. Appl. Anim. Behav. Sci. 171, 204–210. doi: 10.1016/j.applanim.2015.08.026
Brown, K. J., and Grunberg, N. E. (1995). Effects of housing on male and female rats: crowding stresses males but calms females. Physiol. Behav. 58, 1085–1089. doi: 10.1016/0031-9384(95)02043-8
Bugajski, J., Gadek-Michalska, A., Borycz, J., and Wieczorek, E. (1994). Effect of crowding on corticosterone responses to central adrenergic stimulation. Agents Actions 41, 73–74. doi: 10.1007/BF02007773
Buhaug, H., and Urdal, H. (2013). An urbanization bomb? Population growth and social disorder in cities. Glob. Environ. Chang. 23, 1–10. doi: 10.1016/j.gloenvcha.2012.10.016
Bullock, T. H. (1984). Comparative neuroscience holds promise for quiet revolutions. Science 225, 473–478. doi: 10.1126/science.6740319
Cacioppo, J. T., Hawkley, L. C., Norman, G. J., and Berntson, G. G. (2011). Social isolation. Ann. N. Y. Acad. Sci. 1231, 17–22. doi: 10.1111/j.1749-6632.2011.06028.x
Castillo-Gómez, E., Pérez-Rando, M., Bellés, M., Gilabert-Juan, J., Llorens, J. V., Carceller, H., et al. (2017). Early social isolation stress and perinatal nmda receptor antagonist treatment induce changes in the structure and neurochemistry of inhibitory neurons of the adult amygdala and prefrontal cortex. eNeuro 4:ENEURO.0034-17.2017. doi: 10.1523/ENEURO.0034-17.2017
Christian, J. J. (1961). Phenomena associated with population density. Proc. Natl. Acad. Sci. U.S.A. 47, 428–449. doi: 10.1073/pnas.47.4.428
Detrixhe, J. J., Samstag, L. W., Penn, L. S., and Wong, P. S. (2014). A lonely idea: solitude's separation from psychological research and theory. Contemp. Psychoanal. 50, 310–331. doi: 10.1080/00107530.2014.897853
Djordjević, J., Cvijić, G., and Davidović, V. (2003). Different activation of ACTH and corticosterone release in response to various stressors in rats. Physiol. Res. 52, 67–72.
Dong, E., Matsumoto, K., Uzunova, V., Sugaya, I., Takahata, H., Nomura, H., et al. (2001). Brain 5a-dihydroprogesterone and allopregnanolone synthesis in a mouse model of protracted social isolation. Proc. Natl. Acad. Sci. U.S.A. 98, 2849–2854. doi: 10.1073/pnas.051628598
Dulac, C., O'Connell, L. A., and Wu, Z. (2014). Neural control of maternal and paternal behaviors. Science 345, 765–770. doi: 10.1126/science.1253291
Fanselow, M. S., and Lester, L. S. (1988). “A functional behavioristic approach to aversively motivated behavior: predatory imminence as a determinant of the topography of defensive behavior”, in Evolution and Learning, eds R. C. Bolles and M. D. Beecher (Lawrence Erlbaum Associates, Inc).
Fone, K. C. F., and Porkess, M. V. (2008). Behavioural and neurochemical effects of post-weaning social isolation in rodents-relevance to developmental neuropsychiatric disorders. Neurosci. Biobehav. Rev. 32, 1087–1102. doi: 10.1016/j.neubiorev.2008.03.003
Gamallo, A., Villanua, A., Trancho, G., and Fraile, A. (1986). Stress adaptation and adrenal activity in isolated and crowded rats. Physiol. Behav. 36, 217–221. doi: 10.1016/0031-9384(86)90006-5
George, J. M., Bell, Z. W., Condliffe, D., Dohrer, K., Abaurrea, T., Spencer, K., et al. (2019). Acute social isolation alters neurogenomic state in songbird forebrain. Proc. Natl. Acad. Sci. U.S.A. 201820841. doi: 10.1073/pnas.1820841116
Golden, S. A., Covington, H. E., Berton, O., and Russo, S. J. (2011). A standardized protocol for repeated social defeat stress in mice. Nat. Protoc. 6, 1183–1191. doi: 10.1038/nprot.2011.361
Halperin, J. R. P., and Dunham, D. W. (1993). Increased aggressiveness after brief social isolation of adult fish: a connectionist model which organizes this literature. Behav. Processes. 28, 123–144. doi: 10.1016/0376-6357(93)90087-8
Hawkes, K., O'Connell, J. F., Blurton Jones, N. G., Alvarez, H., and Charnov, E. L. (1998). Grandmothering, menopause, and the evolution of human life histories. Proc. Natl. Acad. Sci. U.S.A. 95, 1336–1339. doi: 10.1073/pnas.95.3.1336
Hawkley, L. C., and Cacioppo, J. T. (2010). Loneliness matters: a theoretical and empirical review of consequences and mechanisms. Ann. Behav. Med. 40, 218–227. doi: 10.1007/s12160-010-9210-8
He, X., Lau, E. H. Y., Wu, P., Deng, X., Wang, J., Hao, X., et al. (2020). Temporal dynamics in viral shedding and transmissibility of COVID-19. Nat. Med. 26, 672–675. doi: 10.1101/2020.03.15.20036707
Hein, A. M., Altshuler, D. L., Cade, D. E., Liao, J. C., Martin, B. T., and Taylor, G. K. (2020). An algorithmic approach to natural behavior. Curr. Biol. 30, 9–11. doi: 10.1016/j.cub.2020.04.018
Hermes, G., Li, N., Duman, C., and Duman, R. (2011). Post-weaning chronic social isolation produces profound behavioral dysregulation with decreases in prefrontal cortex synaptic-associated protein expression in female rats. Physiol. Behav. 104, 354–359. doi: 10.1016/j.physbeh.2010.12.019
Hilakivi, L. A., Ota, M., and Lister, R. (1989). Effect of isolation on brain monoamines and the behavior of mice in tests of exploration, locomotion, anxiety and behavioral “despair.” Pharmacol. Biochem. Behav. 33, 371–374. doi: 10.1016/0091-3057(89)90516-9
House, J. S., Landis, K. R., Umberson, D., House, J. S., Landis, I. A. R. L. R., and Umberson, D. (1988). Social relationships and health. Science 241, 540–545. doi: 10.1126/science.3399889
Ieraci, A., Mallei, A., and Popoli, M. (2016). Social isolation stress induces anxious-depressive-like behavior and alterations of neuroplasticity-related genes in adult male mice. Neural Plast. 2016:6212983. doi: 10.1155/2016/6212983
Kaplan, H. S., Hooper, P. L., and Gurven, M. (2009). The evolutionary and ecological roots of human social organization. Philos. Trans. R. Soc. B Biol. Sci. 364, 3289–3299. doi: 10.1098/rstb.2009.0115
Keeney, A., Jessop, D. S., Harbuz, M. S., Marsden, C. A., Hogg, S., and Blackburn-Munro, R. E. (2006). Differential effects of acute and chronic social defeat stress on hypothalamic-pituitary-adrenal axis function and hippocampal serotonin release in mice. J. Neuroendocrinol. 18, 330–338. doi: 10.1111/j.1365-2826.2006.01422.x
Keesom, S. M., Finton, C. J., Sell, G. L., and Hurley, L. M. (2017). Early-life social isolation influences mouse ultrasonic vocalizations during male-male social encounters. PLoS ONE 12:e0169705. doi: 10.1371/journal.pone.0169705
Keesom, S. M., Morningstar, M. D., Sandlain, R., Wise, B. M., and Hurley, L. M. (2018). Social isolation reduces serotonergic fiber density in the inferior colliculus of female, but not male, mice. Brain Res. 1694, 94–103. doi: 10.1016/j.brainres.2018.05.010
Kolling, N., Behrens, T. E. J., Mars, R. B., and Rushworth, M. F. S. (2012). Neural mechanisms of foraging. Science 335, 95–98. doi: 10.1126/science.1216930
Korte, S. M., Koolhaas, J. M., Wingfield, J. C., and McEwen, B. S. (2005). The Darwinian concept of stress: benefits of allostasis and costs of allostatic load and the trade-offs in health and disease. Neurosci. Biobehav. Rev. 29, 3–38. doi: 10.1016/j.neubiorev.2004.08.009
Krakauer, J. W., Ghazanfar, A. A., Gomez-Marin, A., MacIver, M. A., and Poeppel, D. (2017). Neuroscience needs behavior: correcting a reductionist bias. Neuron 93, 480–490. doi: 10.1016/j.neuron.2016.12.041
Lacey, E. A., and Sherman, P. W. (2007). The Ecology of Sociality in Rodents. Chicago, IL: University of Chicago Press.
Lapiz, M. D. S., Fulford, A., Muchimapura, S., Mason, R., Parker, T., and Marsden, C. A. (2003). Influence of postweaning social isolation in the rat on brain development, contioned behavior, and neurotransmission. Neurosci. Behav. Physiol. 33, 13–29. doi: 10.1023/A:1021171129766
Lihoreau, M., Brepson, L., and Rivault, C. (2009). The weight of the clan: even in insects, social isolation can induce a behavioural syndrome. Behav. Processes 82, 81–84. doi: 10.1016/j.beproc.2009.03.008
Lin, E. J. D., Sun, M., Choi, E. Y., Magee, D., Stets, C. W., and During, M. J. (2015). Social overcrowding as a chronic stress model that increases adiposity in mice. Psychoneuroendocrinology 51, 318–330. doi: 10.1016/j.psyneuen.2014.10.007
Liu, C., Li, Y., Edwards, T. J., Kurniawan, N. D., Richards, L. J., and Jiang, T. (2016). Altered structural connectome in adolescent socially isolated mice. Neuroimage 139, 259–270. doi: 10.1016/j.neuroimage.2016.06.037
Liu, J., Dietz, K., Deloyht, J. M., Pedre, X., Kelkar, D., Kaur, J., et al. (2012). Impaired adult myelination in the prefrontal cortex of socially isolated mice. Nat. Neurosci. 15, 1621–1623. doi: 10.1038/nn.3263
Long, C. R., and Averill, J. R. (2003). Solitude: an exploration of benefits of being alone. J. Theory Soc. Behav. 33, 21–44. doi: 10.1111/1468-5914.00204
Louch, C. D., and Higginbotham, M. (1967). The reation between social rank and plasma corticosterone levels in mice. Gen. Comp. Endocrinol. 8, 441–444. doi: 10.1016/S0016-6480(67)80006-6
Love, J., Hoepfner, A., and Goller, F. (2019). Song feature specific analysis of isolate song reveals interspecific variation in learned components. Dev. Neurobiol. 79, 350–369. doi: 10.1002/dneu.22682
Makinodan, M., Rosen, K. M., Ito, S., and Corfas, G. (2012). A critical period for social experience-dependent oligodendrocyte maturation and myelination. Science 337, 1357–1360. doi: 10.1126/science.1220845
Marsden, H. M. (1972). “Crowding and animal behavior,” in Environment and the Social Sciences: Perspectives and Applications, eds J. F. Wohlwill and D. H. Carson (Washington, DC: American Psychological Association), 5–14. doi: 10.1037/10045-001
Matthews, G. A., Nieh, E. H., Vander Weele, C. M., Halbert, S. A., Pradhan, R. V., Yosafat, A. S., et al. (2016). Dorsal raphe dopamine neurons represent the experience of social isolation. Cell 164, 617–631. doi: 10.1016/j.cell.2015.12.040
McEwen, B. S. (2000). Allostasis and allostatic load: implications for neuropsychopharmacology. Neuropsychopharmacology 22, 108–124. doi: 10.1016/S0893-133X(99)00129-3
McEwen, B. S., and Stellar, E. (1993). Stress and the individual. Arch. Intern. Med. 153, 2093–2101. doi: 10.1001/archinte.1993.00410180039004
McEwen, B. S., and Wingfield, J. C. (2003). The concept of allostasis in biology and biomedicine. Horm. Behav. 43, 2–15. doi: 10.1016/S0018-506X(02)00024-7
Medendorp, W. E., Petersen, E. D., Pal, A., Wagner, L. M., Myers, A. R., Hochgeschwender, U., et al. (2018). Altered behavior in mice socially isolated during adolescence corresponds with immature dendritic spine morphology and impaired plasticity in the prefrontal cortex. Front. Behav. Neurosci. 12:87. doi: 10.3389/fnbeh.2018.00087
Mesterton-Gibbons, M., and Dugatkin, L. A. (1995). Toward a theory of dominance hierarchies: effects of assessment, group size, and variation in fighting ability. Behav. Ecol. 6, 416–423. doi: 10.1093/beheco/6.4.416
Mumtaz, F., Khan, M. I., Zubair, M., and Dehpour, A. R. (2018). Neurobiology and consequences of social isolation stress in animal model—a comprehensive review. Biomed. Pharmacother. 105, 1205–1222. doi: 10.1016/j.biopha.2018.05.086
Nguyen, T., vy, T., Ryan, R. M., and Deci, E. L. (2018). Solitude as an approach to affective self-regulation. Personal Soc. Psychol. Bull. 44, 92–106. doi: 10.1177/0146167217733073
Nieder, A. (2018). Evolution of cognitive and neural solutions enabling numerosity judgements: lessons from primates and corvids. Philos. Trans. R. Soc. B Biol. Sci. 373:20160514. doi: 10.1098/rstb.2016.0514
O'Connell, L. A., and Hofmann, H. A. (2011a). Genes, hormones, and circuits: an integrative approach to study the evolution of social behavior. Front. Neuroendocrinol. 32:4. doi: 10.1016/j.yfrne.2010.12.004
O'Connell, L. A., and Hofmann, H. A. (2011b). The Vertebrate mesolimbic reward system and social behavior network: a comparative synthesis. J. Comp. Neurol. 519, 3599–3639. doi: 10.1002/cne.22735
O'Connell, L. A., and Hofmann, H. A. (2012). Evolution of a vertebrate social decision-making network. Science 336, 1154–1157. doi: 10.1126/science.1218889
Odum, H. T., and Allee, W. C. (1954). A note on the stable point of populations showing both intraspecific cooperation and disoperation. Ecology 35, 95–97. doi: 10.2307/1931412
Opendak, M., Offit, L., Monari, P., Schoenfeld, T. J., Sonti, A. N., Cameron, H. A., et al. (2016). Lasting adaptations in social behavior produced by social disruption and inhibition of adult neurogenesis. J. Neurosci. 36, 7027–7038. doi: 10.1523/JNEUROSCI.4435-15.2016
Ortiz, R., Armario, A., Castellanos, J. M., and Balasch, J. (1985). Post-weaning crowding induces corticoadrenal hyperreactivity in male mice. Physiol. Behav. 34, 857–860. doi: 10.1016/0031-9384(85)90003-4
Pais, A. B., Pais, A. C., Elmisurati, G., Park, S. H., Miles, M. F., and Wolstenholme, J. T. (2019). A novel neighbor housing environment enhances social interaction and rescues cognitive deficits from social isolation in adolescence. Brain Sci. 9:336. doi: 10.3390/brainsci9120336
Paolino, A., Fenlon, L. R., Kozulin, P., Haines, E., Lim, J. W. C., Richards, L. J., et al. (2020). Differential timing of a conserved transcriptional network underlies divergent cortical projection routes across mammalian brain evolution. Proc. Natl. Acad. Sci. U.S.A. 117, 10554–10564. doi: 10.1073/pnas.1922422117
Pauly, T., Lay, J. C., Nater, U. M., Scott, S. B., and Hoppmann, C. A. (2017). How we experience being alone: age differences in affective and biological correlates of momentary solitude. Gerontology 63, 55–66. doi: 10.1159/000450608
Perusini, J. N., and Fanselow, M. S. (2015). Neurobehavioral perspectives on the distinction between fear and anxiety. Learn. Mem. 22, 417–425. doi: 10.1101/lm.039180.115
Poisbleau, M., Fritz, H., Guillon, N., and Chastel, O. (2005). Linear social dominance hierarchy and corticosterone responses in male mallards and pintails. Horm. Behav. 47, 485–492. doi: 10.1016/j.yhbeh.2005.01.001
Pravosudov, V. V., Mendoza, S. P., and Clayton, N. S. (2003). The relationship between dominance, corticosterone, memory, and food caching in mountain chickadees (Poecile gambeli). Horm. Behav. 44, 93–102. doi: 10.1016/S0018-506X(03)00119-3
Reiss, D., Wolter-Sutter, A., Krezel, W., and Ouagazzal, A. M. (2007). Effects of social crowding on emotionality and expression of hippocampal nociceptin/orphanin FQ system transcripts in mice. Behav. Brain Res. 184, 167–173. doi: 10.1016/j.bbr.2007.07.010
Saavedra-Rodríguez, L., and Feig, L. A. (2013). Chronic social instability induces anxiety and defective social interactions across generations. Biol. Psychiatry 73, 44–53. doi: 10.1016/j.biopsych.2012.06.035
Sapolsky, R. M. (2015). Stress and the brain: individual variability and the inverted-U. Nat. Neurosci. 18, 1344–1346. doi: 10.1038/nn.4109
Scaccianoce, S., Del Bianco, P., Paolone, G., Caprioli, D., Modafferi, A. M. E., Nencini, P., et al. (2006). Social isolation selectively reduces hippocampal brain-derived neurotrophic factor without altering plasma corticosterone. Behav. Brain Res. 168, 323–325. doi: 10.1016/j.bbr.2005.04.024
Schoech, S. J., Mumme, R. L., and Wingfield, J. C. (1997). Corticosterone, reproductive status, and body mass in a cooperative breeder. Physiol. Zool. 70, 68–73. doi: 10.1086/639545
Screven, L. A., and Dent, M. L. (2019). Perception of ultrasonic vocalizations by socially housed and isolated mice. Eneuro 6:ENEURO.0049-19.2019. doi: 10.1523/ENEURO.0049-19.2019
Selye, H. (1976). “Stress without distress,” in Psychopathology of Human Adaptation, ed G. Serban (Boston, MA: Springer), 137–146. doi: 10.1007/978-1-4684-2238-2_9
Shams, S., Chatterjee, D., and Gerlai, R. (2015). Chronic social isolation affects thigmotaxis and whole-brain serotonin levels in adult zebrafish. Behav. Brain Res. 292, 283–287. doi: 10.1016/j.bbr.2015.05.061
Silk, J. B. (2007). The adaptive value of sociality in mammalian groups. Philos. Trans. R. Soc. B Biol. Sci. 362, 539–559. doi: 10.1098/rstb.2006.1994
So, N., Franks, B., Lim, S., and Curley, J. P. (2015) A Social Network Approach Reveals Associations between Mouse Social Dominance Brain Gene Expression. PLoS ONE. 10:e0134509. doi: 10.1371/journal.pone.0134509
Stephens, P., Sutherland, W., and Freckleton, R. (1999). What is the Allee effect. Oikos 87, 185–190. doi: 10.2307/3547011
Stephens, P. A., and Sutherland, W. J. (1999). Consequences of the Allee effect for behaviour, ecology and conservation. Trends Ecol. Evol. 14, 401–405. doi: 10.1016/S0169-5347(99)01684-5
Stevenson, P. A., and Rillich, J. (2013). Isolation associated aggression - a consequence of recovery from defeat in a territorial animal. PLoS ONE 8:e0074965. doi: 10.1371/journal.pone.0074965
Tamashiro, K. L. K., Nguyen, M. M. N., and Sakai, R. R. (2005). Social stress: from rodents to primates. Front. Neuroendocrinol. 26:1. doi: 10.1016/j.yfrne.2005.03.001
Ueda, A., and Wu, C.-F. (2009). Effects of social isolation on neuromuscular excitability and aggressive behaviors in Drosophila: altered responses by Hk and gsts1, two mutations implicated in redox regulation. J. Neurogenet. 23, 378–394. doi: 10.3109/01677060903063026
Valzelli, L. (1973). The “isolation syndrome” in mice. Psychopharmacologia 31, 305–320. doi: 10.1007/BF00421275
Van Den Berg, W. E., Lamballais, S., and Kushner, S. A. (2015). Sex-specific mechanism of social hierarchy in mice. Neuropsychopharmacology 40, 1364–1372. doi: 10.1038/npp.2014.319
Varholick, J. A., Pontiggia, A., Murphy, E., Daniele, V., Palme, R., Voelkl, B., et al. (2019). Social dominance hierarchy type and rank contribute to phenotypic variation within cages of laboratory mice. Sci. Rep. 9, 1–11. doi: 10.1038/s41598-019-49612-0
Wang, L., Dankert, H., Perona, P., and Anderson, D. J. (2008). A common genetic target for environmental and heritable influences on aggressiveness in Drosophila. Proc. Natl. Acad. Sci. U.S.A. 105, 5657–5663. doi: 10.1073/pnas.0801327105
Weiss, I. C., Pryce, C. R., Jongen-Rêlo, A. L., Nanz-Bahr, N. I., and Feldon, J. (2004). Effect of social isolation on stress-related behavioural and neuroendocrine state in the rat. Behav. Brain Res. 152, 279–295. doi: 10.1016/j.bbr.2003.10.015
Williamson, C. M., Franks, B., and Curley, J. P. (2016). Mouse Social Network Dynamics and Community Structure are Associated with Plasticity-Related Brain Gene Expression. Front. Behav. Neurosci. 10:152. doi: 10.3389/fnbeh.2016.00152
Williamson, C. M., Lee, W., DeCasien, A. R., Lanham, A., Romeo, R. D., and Curley, J. P. (2019). Social hierarchy position in female mice is associated with plasma corticosterone levels and hypothalamic gene expression. Sci. Rep. 9, 1–14. doi: 10.1038/s41598-019-43747-w
Zelikowsky, M., Ding, K., and Anderson, D. J. (2018a). Neuropeptidergic control of an internal brain state produced by prolonged social isolation stress. Cold Spring Harb. Symp. Quant. Biol. 83, 97–103. doi: 10.1101/sqb.2018.83.038109
Keywords: social isolation, stress, social density, crowding, brain modifications
Citation: Love J and Zelikowsky M (2020) Stress Varies Along the Social Density Continuum. Front. Syst. Neurosci. 14:582985. doi: 10.3389/fnsys.2020.582985
Received: 13 July 2020; Accepted: 22 September 2020;
Published: 20 October 2020.
Edited by:
Arun Asok, Columbia University, United StatesReviewed by:
Annegret L. Falkner, Princeton University, United StatesCopyright © 2020 Love and Zelikowsky. This is an open-access article distributed under the terms of the Creative Commons Attribution License (CC BY). The use, distribution or reproduction in other forums is permitted, provided the original author(s) and the copyright owner(s) are credited and that the original publication in this journal is cited, in accordance with accepted academic practice. No use, distribution or reproduction is permitted which does not comply with these terms.
*Correspondence: Jay Love, amF5LmxvdmVAdXRhaC5lZHU=
Editor's Note: Arun Asok edited the article in collaboration with Eric R. Kandel, Columbia University, United States.
Disclaimer: All claims expressed in this article are solely those of the authors and do not necessarily represent those of their affiliated organizations, or those of the publisher, the editors and the reviewers. Any product that may be evaluated in this article or claim that may be made by its manufacturer is not guaranteed or endorsed by the publisher.
Research integrity at Frontiers
Learn more about the work of our research integrity team to safeguard the quality of each article we publish.