- 1Department of Biomedical Sciences, School of Medicine Greenville, University of South Carolina, Greenville, SC, United States
- 2Department of Psychiatry and Behavioral Sciences, University of Louisville, Louisville, KY, United States
- 3Department of Pediatrics, Greenville Health System, Greenville, SC, United States
- 4Miller School of Medicine, University of Miami, Miami, FL, United States
There is no accepted pathology to autism spectrum disorders (ASD) but research suggests the presence of an altered excitatory/inhibitory (E/I) bias in the cerebral cortex. Repetitive transcranial magnetic stimulation (rTMS) offers a non-invasive means of modulating the E/I cortical bias with little in terms of side effects. In this study, 124 high functioning ASD children (IQ > 80, <18 years of age) were recruited and assigned using randomization to either a waitlist group or one of three different number of weekly rTMS sessions (i.e., 6, 12, and 18). TMS consisted of trains of 1.0 Hz frequency pulses applied over the dorsolateral prefrontal cortex (DLPFC). The experimental task was a visual oddball with illusory Kanizsa figures. Behavioral response variables included reaction time and error rate along with such neurophysiological indices such as stimulus and response-locked event-related potentials (ERP). One hundred and twelve patients completed the assigned number of TMS sessions. Results showed significant changes from baseline to posttest period in the following measures: motor responses accuracy [lower percentage of committed errors, slower latency of commission errors and restored normative post-error reaction time slowing in both early and later-stage ERP indices, enhanced magnitude of error-related negativity (ERN), improved error monitoring and post-error correction functions]. In addition, screening surveys showed significant reductions in aberrant behavior ratings and in both repetitive and stereotypic behaviors. These differences increased with the total number of treatment sessions. Our results suggest that rTMS, particularly after 18 sessions, facilitates cognitive control, attention and target stimuli recognition by improving discrimination between task-relevant and task-irrelevant illusory figures in an oddball test. The noted improvement in executive functions of behavioral performance monitoring further suggests that TMS has the potential to target core features of ASD.
Introduction
Autism is defined as a spectrum of behavioral disorders that share in-common impairments in social interaction and communication skills, language deficits, and a restricted repertoire of interests and stereotyped activities (American Psychiatric Association [APA], 2013). Currently, the diagnosis of autism spectrum disorder (ASD) relies on behavioral evaluations. Since there are no neuropathological findings nor laboratory tests to confirm the diagnosis, research in ASD has been guided by various conceptual models or theoretical constructs, such as executive functioning deficits (Ozonoff et al., 1991), cortical coherence weakness (Frith and Happé, 1994), information processing abnormalities (Minshew et al., 1997), theory-of-mind, empathy (Baron-Cohen, 1989, 2004), abnormal neuroplasticity (Oberman et al., 2010),“broken” mirror neuron system (Dapretto et al., 2006), altered neural connectivity (Belmonte et al., 2004; Just et al., 2004), and an alteration in the excitation-to-inhibition (E/I) bias of the cerebral cortex (Casanova et al., 2003; Rubenstein and Merzenich, 2003; Uzunova et al., 2016).
An inhibitory dysfunction model for ASD is in-keeping with reported neuropathological findings of a minicolumnopathy (Casanova, 2005, 2007; Casanova et al., 2006a,b). It also serves to explain some of the atypicality in cognitive processing, deficits in emotional reactivity, and seizures observed in autistic patients (Casanova et al., 2002a,b,c, 2014a). If this minicolumnopathy is one of the core neuropathological characteristics of ASD it would be amenable to treatment when taking into consideration the location, orientation, and nature of the neuroanatomical elements within this modular structure (Casanova et al., 2006b).
Neuromodulation and, more specifically, non-invasive brain stimulation is an intervention aimed at normalizing the putative cortical inhibitory deficit of autism. Among neuromodulation techniques, transcranial magnetic stimulation (TMS) has provided promising results as a possible therapeutic modality in many psychiatric disorders (Wassermann and Lisanby, 2001; Pascual-Leone et al., 2002; George et al., 2003; Rossi et al., 2009; Enticott et al., 2014; Rotenberg et al., 2014; Oberman and Enticott, 2015; Ni et al., 2017). Magnetic pulses generated by a current passing through a coil stimulate targeted cortical regions, usually within 2–3 cm of the skull surface (Rudiak and Marg, 1994; George et al., 1999; Hoffman and Cavus, 2002). By convention, rTMS of 1.0 Hz frequency or lower is referred to as “slow” or inhibitory, while “fast” frequencies (>1.0 Hz) as excitatory. One of the models of inhibitory rTMS effects considers long-term depression and long-term depotentiation as probable mechanisms of action (Hoffman and Cavus, 2002). It is proposed that TMS-induced alterations of cortical excitability accumulate additively with increasing number of sessions (Casanova et al., 2015). More detailed description of the biophysical aspects of TMS action can be found in Wagner et al. (2009), while basic principles of rTMS are reviewed by Klomjai et al. (2015).
Currently, TMS is used to: (1) explore stimulation-induced alterations in functional connectivity measures within and between brain regions; (2) investigate the behavioral, cognitive, and emotional relevance of these changes; and, most importantly, (3) promote changes in cortical function (Daskalakis et al., 2002). Researchers believe that these changes are achieved by inducing a temporal functional reorganization of the cerebral cortex. The rTMS-induced neuroplasticity is dependent on the state of the stimulated cortical area and on the intensity, frequency, and total number of administered magnetic pulses. Effects induced by rTMS are not limited to the stimulated cortical region, as changes are noted in other functionally interconnected areas. This makes rTMS a valuable instrument in the investigation of neuroplasticity related phenomena of the cerebral cortex (Ziemann, 2004).
The use of rTMS in autism, especially in children with ASD, has never been systematically assessed, e.g., dose, duration, type of rTMS stimulation and other variables, thus there is a need for more rigorous analysis. However, the studies of rTMS in children have not reported any notable adverse side effects. In this regard, Gilbert et al. (2004) article offered a useful reference to Institutional Review Boards (IRBs) when making a determination as to whether TMS-device based intervention poses minimal risk for treating children. The study was based on a MEDLINE review that attempted to establish any evidence of risk from TMS administration. The meta-analysis identified 28 studies involving over 850 children who underwent single or paired pulse TMS. Mild transient side effects (e.g., scalp discomfort, headaches) resolved by the day following stimulation. The authors concluded that the experimental designs of the reviewed studies conveyed no more than minimal risk to children. Similarly, the safety of TMS was confirmed in another review of more than a thousand children treated for different neuropsychiatric conditions (e.g., epilepsy, multiple sclerosis, myoclonus, ADHD, Tourette’s, and depression) (Quintana, 2005; see also Walter et al., 2001; Garvey and Gilbert, 2004; Garvey and Mall, 2008; Croarkin et al., 2011; Le et al., 2013; Hong et al., 2015; Krishnan et al., 2015).
Oberman et al. (2015) reviewed the use of TMS specifically in ASDs. Her studies covered a search in PubMed until May 2013 using TMS, autism and Asperger as keywords. The search identified 17 studies matching these keywords. From these studies, rTMS was used in 8 of them as a therapeutic tool. These 8 studies involved 104 ASD individuals. Five of these studies used the dorsolateral prefrontal cortex (DLPFC) as the site of stimulation. Side effects were minor. The authors called for more carefully designed and properly controlled studies to assess the therapeutic potential of TMS in ASD. Similar advice was provided by a consensus group on TMS application in autism research and treatment (Oberman et al., 2016).
In our own prior studies, we investigated the effects of low frequency (inhibitory) rTMS in children and adolescents with high-functioning autism and Asperger syndrome diagnosis (Sokhadze et al., 2009a,b, 2010b, 2012a,b, 2013, 2014a,b, 2016, 2017; Baruth et al., 2010a,b,c; Casanova et al., 2012, 2014b, 2015; Wang et al., 2016). Outcome measures in these studies included behavioral ratings as well as subjects’ performance (pre- and post-treatment) in a visual oddball task using reaction time measures and event-related potential (ERP) methodology. The behavioral performance findings of these studies can be summarized as group differences in response accuracy and in post-error reaction time slowing rather than in reaction time measures. We reported significant group differences in ERP measures, mostly in terms of excessive responsiveness of early ERP components (100–200 ms post-stimulus) to frequent standard and rare task-irrelevant distracter stimuli resulting in delayed late ERP potentials (200–400 ms post-stimulus) to these non-target items.
In our studies, the group of children with autism typically showed prolonged latencies of ERPs to rare novel distracters but not to targets, with this effect being observed both at the frontal and parietal topographies. In general, signs of excessive activation in the parietal cortex at the early stages of processing of task-irrelevant stimuli, along with under-activation of prefrontal cortex at the late phases of task-relevant target processing were common in children with ASD. In these studies, we could not find any abnormalities of the parietal and/or centro-parietal P3b ERP component in response to target stimuli in children with ASD as compared to typically developing children. We interpreted the results as indicating reduced discriminatory ability during performance on an oddball task in children with autism (Sokhadze et al., 2017).
The present clinical research study was designed to address some technical, feasibility, acceptability, safety and conceptual issues that were not resolved in prior published studies. Among the most important aims of the study was the comparison of three groups of total number of sessions (6 vs. 12 vs. 18), with the prediction that the higher number of TMS sessions was necessary to detect robust changes in aberrant and repetitive behaviors, targeted ERP indices, and executive functions measures manifested during performance on reaction time tests.
Materials and Methods
Subjects
Participants with ASD were recruited through referrals from several pediatric clinics. All patients were diagnosed according to the Diagnostic and Statistical Manual of Mental Disorders (DSM-IVTR) or DSM-5 (American Psychiatric Association [APA], 2000, 2013). Diagnosis of autism was ascertained with the Autism Diagnostic Interview – Revised (ADI-R) (Le Couteur et al., 2003). Participants were evaluated also by a developmental pediatrician. Patients had normal hearing. Patients were excluded from participation if they had a history of seizures, impairment of vision, genetic disorders, and/or brain abnormalities based on neuroimaging studies. Enrolled subjects were high-functioning children or adolescents with a full-scale Intelligence Quotient (IQ) of more than 80 according to evaluations using the Wechsler Intelligence Scale for Children, Fourth Edition [WISC-IV, (Wechsler, 2003)] or the Wechsler Abbreviated Scale of Intelligence [WASI, (Wechsler, 1999)].
The study enrolled a total of 124 children with an ASD diagnosis with 112 of them completing the assigned number of rTMS sessions. Mean age in the waitlist (WTL) group was 13.3 ± 1.78 years [N = 26, 4 Females (F)]; in the group of 6 sessions the mean age was 12.5 ± 1.47 years (N = 25, 4 F); in the group of 12 sessions the mean age was 12.8 ± 1.57 years (N = 30, 6 F); and in the group of 18 sessions the mean age was 13.5 ± 2.30 years (N = 31, 5 F). The mean age for all enrolled subjects was 13.1 ± 1.78 years (N = 112) without significant between-group differences. We had to exclude three participants from the 12 session TMS group and another three subjects from the 18 session TMS group (all of them boys) due to excessive artifacts and/or missing parental evaluation questionnaires, thus reducing the total number of subjects analyzed to 106 (WTL, N = 26; 6 TMS, N = 25; 12 TMS, N = 27, 12.9 ± 1.62 years; and 18 TMS, N = 28, 13.5 ± 2.31 years). Only 19 of completers were females (i.e., boys/girls ratio was ∼4.5:1). This proportion closely approximates that typically reported for gender bias in high-functioning ASD children.
Randomization at the early stages of this project followed recommendations of the local Institutional Review Board (IRB) as to assignments to either TMS or waitlist. Only after initial pilot data analysis on 9 ASD in the rTMS (6 sessions of left DLPFC) and 5 in the waitlist group and publication of results (Sokhadze et al., 2009b), we added amendment to extend session number to 12 and 18, and started randomizing after that subjects either to waitlist, 6 TMS, 12 TMS or 18 TMS.
The study was conducted in accordance with relevant national regulations and institutional policies and complied with the Helsinki Declaration. The protocol of the study including informed consent and assent forms were reviewed and received approval of the university IRB. Children and their family representatives (either parents or legal guardians) received detailed information about this research study specifics, including purpose of research, responsibilities, reimbursement rate, risk vs. benefits evaluation, etc. The participants were reimbursed only for ERP tests ($25 for each procedure), and did not receive any reimbursement for the TMS treatment. Investigators provided consent and assent forms to all families who expressed interest in participation in this treatment research study, allowed them to review these documents and answered all questions. If the child and his family member agreed to be a part of the study and confirmed their commitment, both child and parent were requested to sign and date the consent and assent forms and then received a copy co-signed by the study investigator.
The schematic representation of the sequence of evaluations is provided on Figure 1.
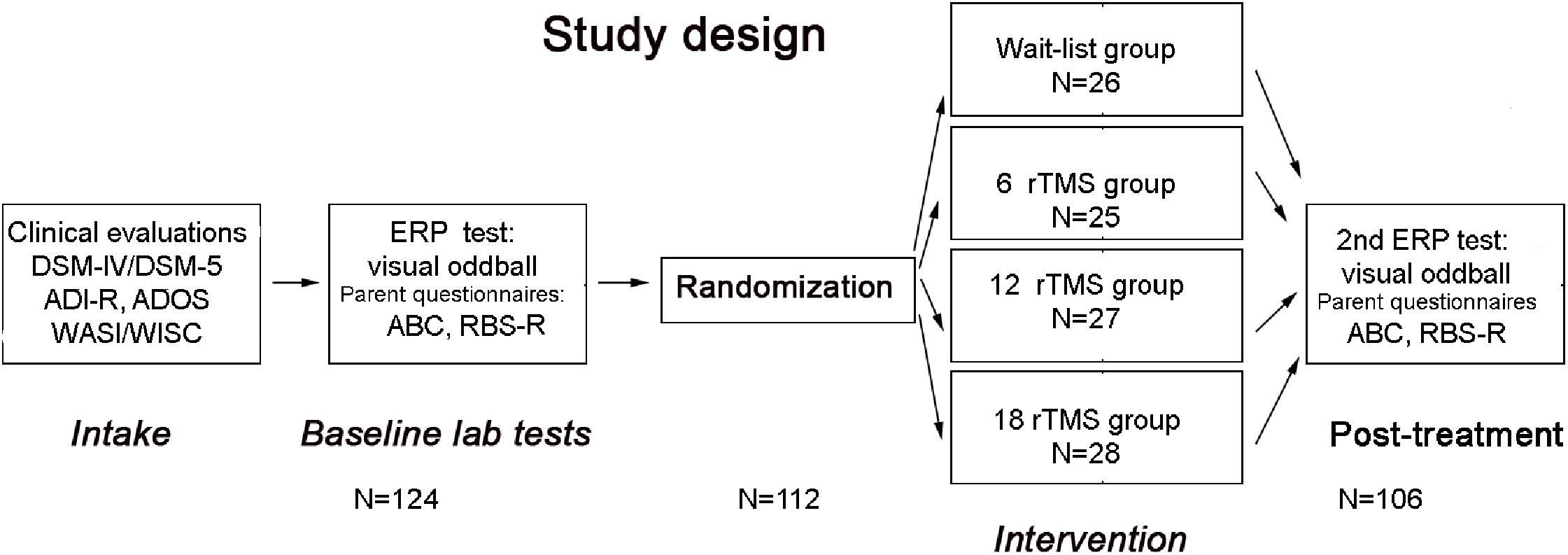
FIGURE 1. Flowchart of the study. After clinical evaluation at intake stage, subjects were tested in the lab using visual oddball task, while parents rated behavioral symptoms using ABC and RBS-R questionnaires. Then participants were randomized either to waitlist, 6 rTMS, 12 rTMS, or 18 rTMS treatment sessions and after completion of the assigned course of treatment were tested again in the lab using the same ERP test, whereas parents completed post-treatment behavioral ratings using ABC and RBS-R questionnaires.
Experimental Task: Visual Oddball With Illusory Kanizsa Figures
The test used in the study was a three-stimuli oddball task with rare illusory Kanizsa (Kanizsa, 1976) squares (target, 25%), rare Kanizsa triangle (novel distracter, also 25%) and frequent non-Kanizsa stimuli (standards, 50%). Visual stimuli were presented for 250 ms with inter-trial interval in 1100–1300 ms range. Before the test, all subjects had a brief practice block (20 trials only) to get familiar with the task specifics, make sure that they understood the test requirements, and that they could recognize the target stimulus correctly. There were a total of 240 trials in the study including a practice block that took around 20–25 min to complete. Participants had at least one lab visit to ensure habituation to the experimental setting and conditioning to the EEG sensor net and the lab environment.
Behavioral Responses
Motor response measures used in the study included reaction time (RT in ms) and accuracy (percentage of correct responses) from where we calculated rates of commission and omission errors and total percentage of errors. For the calculation of post-error slowing measure, RT of the first correct response after committed error (either omission of commission error) was compared to mean RT. The difference between post-error RT and mean correct RT to target was then used to calculate normative post-error RT slowing measure (Franken et al., 2007; Sokhadze et al., 2010a, 2012a,b). Distribution of reaction time in both correct and error responses was analyzed and plotted using a sigmoid curve methodology and normalized histograms according to the technique described in Opris et al. (2016).
Event-Related Potential Recording
The dense-array (128 channel) electroencephalogram (EEG) was recorded with an Electrical Geodesics Inc. Netstation system (EGI-Philips, Eugene, OR, United States). Experimental control (e.g., stimulus presentation, reaction time) was executed using E-prime software [Psychological Software Tools (PST), Inc., Pittsburg, PA, United States]. Visual stimuli were presented on a monitor located in front of the subject, while motor responses were recorded with a 4-bitton keypad (PST’s Serial Box). EEG was recorded with 512 Hz sampling rate, analog Notch (60 Hz, IIR) filter and analog bandpass elliptical filters set at 0.1–100 Hz range. Electrodes impedance was kept under 40 KΩ. Raw EEG recordings were segmented off-line spanning 200 ms pre-stimulus baseline and 800 ms epoch post-stimulus. EEG data was screened for artifacting purposes and all trials that had eye blinks, gross movements and other artifacts were removed using Netstation artifact rejection tools (Srinivasan et al., 1998; Luu et al., 2001). The artifact-free data for correct responses was filtered using digital Notch filter (IIR, 5th order) and also 0.3–20 Hz IIR elliptical bandpass filter. ERPs after averaging were baseline corrected (200 ms) and then re-referenced into an average reference. Commission error response-locked EEG recordings were segmented into 500 ms pre-response to 500 ms post-response. Details of our experimental procedure and EEG data acquisition, pre-processing and analysis can be found in our prior studies using the same methodology (Baruth et al., 2010a,c; Casanova et al., 2012, 2014b; Sokhadze et al., 2012b, 2014a,b).
Stimulus-locked dependent ERP variables for the frontal and fronto-central region-of-interest (ROI: F1, F2, F3, EGI channel 12, FC1, FZ, FCz, F2, F4, EGI channel 5, FC2) were N100 (80–180 ms), and P3a (300–600 ms), and for the parietal and parieto-occipital ROI (P1, P2, PO3, Pz, CPz, P3, P4, PO4) were P100 (100–180 ms) and P3b (320–600 ms) ERP components.
Response-locked ERPs dependent variables in this study were amplitude and latency of the Error-related Negativity (ERN, 40–150 ms post commission error) and Error-related Positivity (Pe, 100–300 ms post-error). The ROI for ERN and Pe components were sites between FCz and FC3- C1, between FCz and FC2-C2, and FCz).
Transcranial Magnetic Stimulation
For repetitive TMS administration we used a Magstim Rapid device (Magstim Co., Sheffield, United Kingdom) with a 70-mm wing span figure-eight coil. To identify resting motor threshold (MT) for each hemisphere the output of the magnetic stimulator was increased by 5% steps until a 50 μV deflection of electromyogram (EMG) or a visible twitch in the First Dorsal Interosseous (FDI) muscle was detected in at least 2 or 3 trials of TMS delivered over the motor cortex controlling the contralateral FDI. EMG was recorded with a portable C-2 J&J Engineering Inc. physiological monitor with USE-3 software and Physiodata applications (J&J Engineering, Inc., Seattle, WA, United States).
In all treatment groups, the rTMS was administered on a weekly basis with the following stimulation parameters: 1.0 Hz frequency, 90% MT, 180 pulses per session with 9 trains of 20 pulses each with 20–30 s intervals between the trains. In the 6 TMS group six weekly rTMS session were administered over the left DLPFC, in the 12 TMS group 12 weekly rTMS sessions with the first six treatments were over the left DLPFC, while the next 6 were over the right DLPFC, while in the 18 TMS group the additional 6 treatments were done bilaterally over the DLPFC (evenly over the left and right DLPFC). The procedure for stimulation placed the TMS coil 5 cm anterior, and in a parasagittal plane, to the site of maximal FDI response as judged by the FDI EMG response (Figure 2). A swimming cap was used to ensure better positioning of the TMS coil. Positioning of the TMS coil followed recommendations that take into consideration anatomical landmarks (Mir-Moghtadaei et al., 2015; Pommier et al., 2017) and could be approximately described as the scalp region used for F3 and F4 EEG electrode placements in the 10–20 International System.
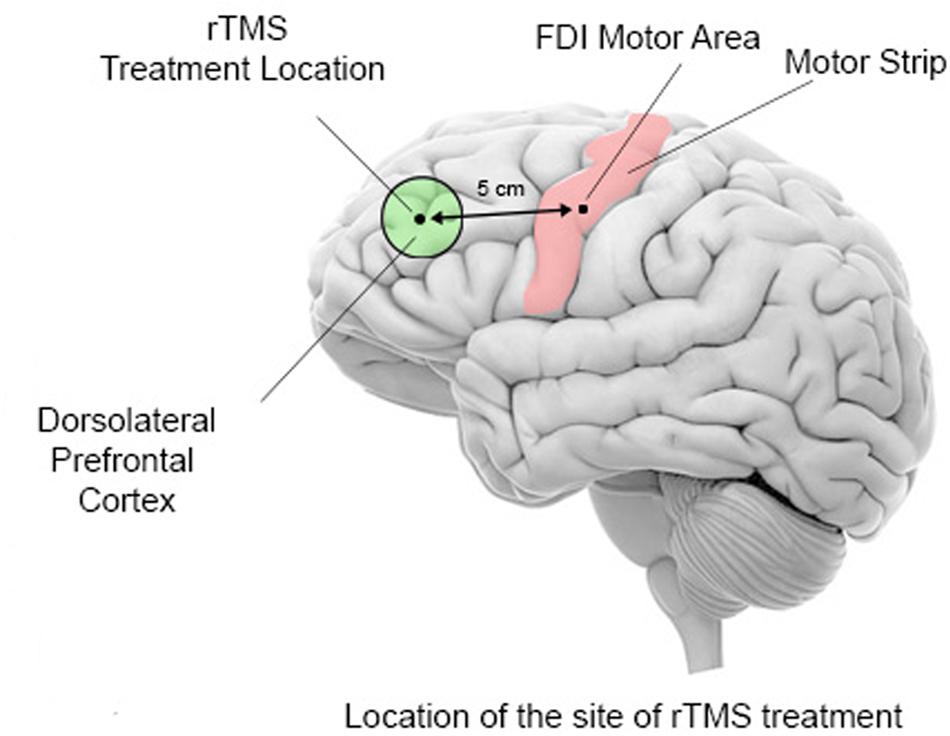
FIGURE 2. Schematic representation of TMS coil location for the stimulation of the dorsolateral prefrontal cortex. Stimulations is administered first over the left motor cortex (motor strip) to determine the optimal area for stimulation of the first dorsal interossei (FDI) muscle of the right hand. The output of the TMS machine is increased until the least amount of machine power that induces an EMG response or a visible twitch is identified in 4 out of 5 trials over the motor cortical area controlling the contralateral FDI. The site for rTMS treatment location is then placed 5 cm anterior to, and in a parasagittal plane to the site of maximal FDI stimulation.
Selection of 90% of the MT was based on data from prior studies where low frequency rTMS was used for the stimulation of DLPFC in various neurological and psychiatric disorders (Pascual-Leone et al., 2000; Wassermann and Lisanby, 2001; Daskalakis et al., 2002; Gershon et al., 2003; Loo and Mitchell, 2005; Wassermann and Zimmermann, 2012). Furthermore, we decided to have stimulation power below MT as a safety precaution measure to lower the probability of seizure risk in this study population. The decision to use low frequency (below or equal 1 Hz) was based on the finding that at this frequency range rTMS exerts an inhibitory influence on the stimulated cortex (Maeda et al., 2000).
Behavioral and Social Functioning Evaluation
Social and behavioral functioning was evaluated using caregiver (parent or guardian) reports. Participants in each group were evaluated before the TMS course (within a period of 6 weeks prestudy) and within a week following treatment. Parental reports using Aberrant Behavior Checklist [(ABC), Aman and Singh, 1994; Aman, 2004] were collected to assess irritability, lethargy/social withdrawal, stereotypy, hyperactivity, and inappropriate speech rating scores. Another parental report, specifically Repetitive Behavior Scale—Revised [(RBS-R), Bodfish et al., 1999, 2000], was used to assess stereotyped, self-injurious, compulsive, ritualistic, sameness, and restricted behaviors rating scores.
Statistical Analysis
Repeated measure ANOVA was the primary model for statistical analyses of subject-averaged ERP, motor response and behavioral questionnaires data. Dependent behavioral variables were RT, omission and commission response rate, total accuracy, post-error RT change vs. mean correct RT, and mean RT in commission errors. Dependent stimulus-locked ERP variables were amplitude and latency of ERPs (N100, P100, P3a, and P3b) at pre-determined frontal and parietal ROIs. The within-participant factors were the following: Stimulus (Target Kanizsa, Non-target Kanizsa, Standard non-Kanizsa), Hemisphere (Left, Right), and Time Point (Baseline, Post-treatment). Response-locked ERPs (ERN/Pe) analysis was conducted in the same manner except using Stimulus and Hemisphere factors. The between-subject factor was Group (Waitlist, 6 TMS, 12 TMS, and 18 TMS). Post hoc analyses using Tukey and Duncan tests were conducted where appropriate. For behavioral rating scores a Treatment (pre- vs. post-TMS/or waiting period) factor was used. ANOVA was completed to determine changes associated with 6, 12, and 18 TMS and waitlist conditions as compared to baseline. Histograms with distribution curves were obtained for each dependent variable to determine normality of distribution and appropriateness of data for ANOVA and post hoc t-tests. For normality analysis we used the Shapiro–Wilk test. All dependent variables in the study had normal distribution. Greenhouse-Geisser (GG) corrected p-values were employed where appropriate in all ANOVAs. For the estimation of the effect size and power (Murphy and Myors, 2004) we used a Partial Eta Squared () and observed power computed using α= 0.05. IBM SPSS 23.0 and Sigma Stat 9.1 statistical software was used for data analysis.
Results
Behavioral Motor Responses (RT, Error Rate, Post-error RT)
Reaction Time (RT)
Effects of TMS session number [Session (0 in waitlist, 6 TMS, 12 TMS, 18 TMS)] on RT to targets were not significant. Comparison of RT to targets yielded no group differences in terms of session number.
Accuracy
There were group differences in accuracy, namely in total percentage of errors [F(4,207) = 3.77, p = 0.001]. Group differences in omission and commission error percentage were also statistically significant [omission errors, F(4,207) = 2.79, p = 0.012; commission errors, F(4,207) = 3.67, p = 0.002]. Statistical significant difference in total error rate was between 18 TMS group, waitlist and baseline (5.2 ± 8.5% in 18 TMS vs. 12.9 ± 14.3% in WTL vs. 13.5 ± 18.3% at baseline, ps < 0.05, see Figure 3A and Table 1). Accuracy difference between 3 TMS groups was not significant. Mean and standard deviations of reaction time, accuracy and post-error RT changes are presented on Table 1.
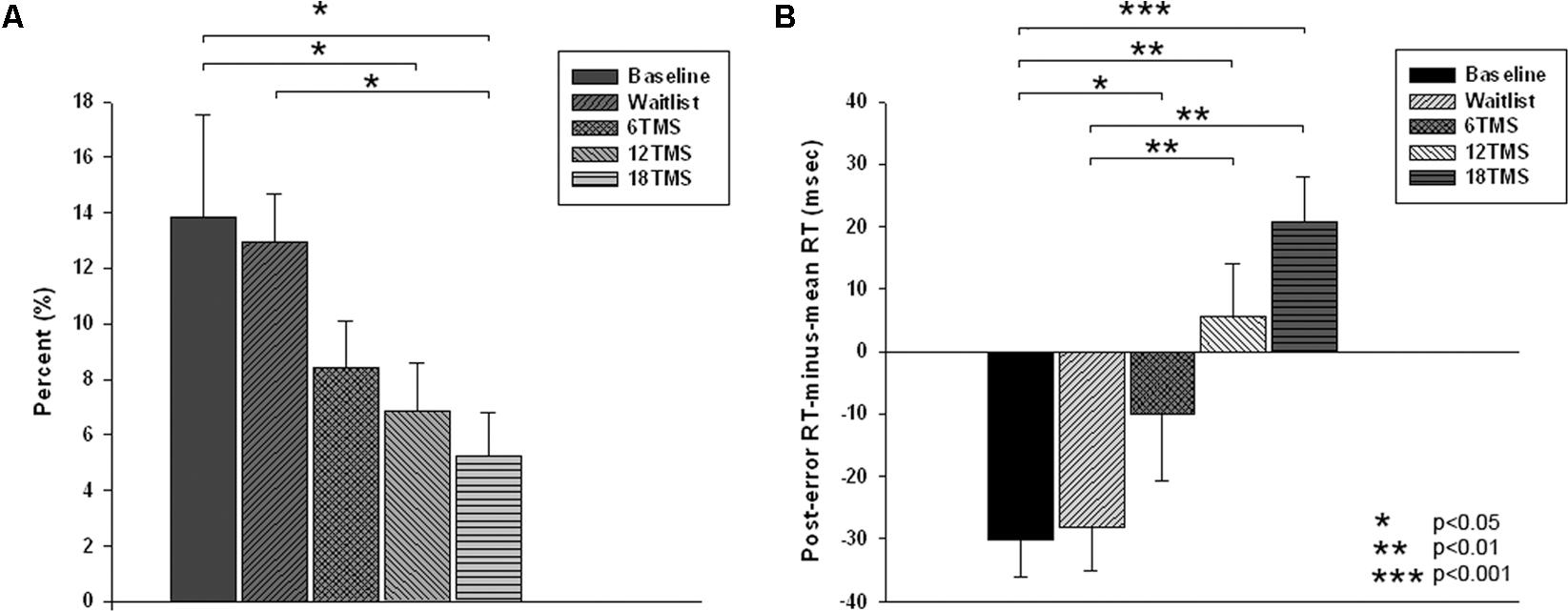
FIGURE 3. (A) Total error rate (in %) in oddball test at baseline, post-wait period, and post-treatment in 6 TMS, 12 TMS, and 18 TMS groups of children with ASD. Accuracy gradually improved in all TMS groups. Most significant difference was between the 18 TMS group as compared to baseline and waitlist (p < 0.05). Accuracy difference between three TMS groups was not significant. (B) Post-error reaction time (RT), calculated as first RT post-error minus mean RT, in visual oddball test at baseline and post treatment in waitlist, 6 TMS, 12 TMS, and 18 TMS groups. Most significant differences were noted between 18 TMS and baseline (p < 0.001), 18 TMS and waitlist (p < 0.001), as well as between 12 TMS and baseline (p = 0.004). Both 12 TMS and 18 TMS groups showed normative post-error slowing.
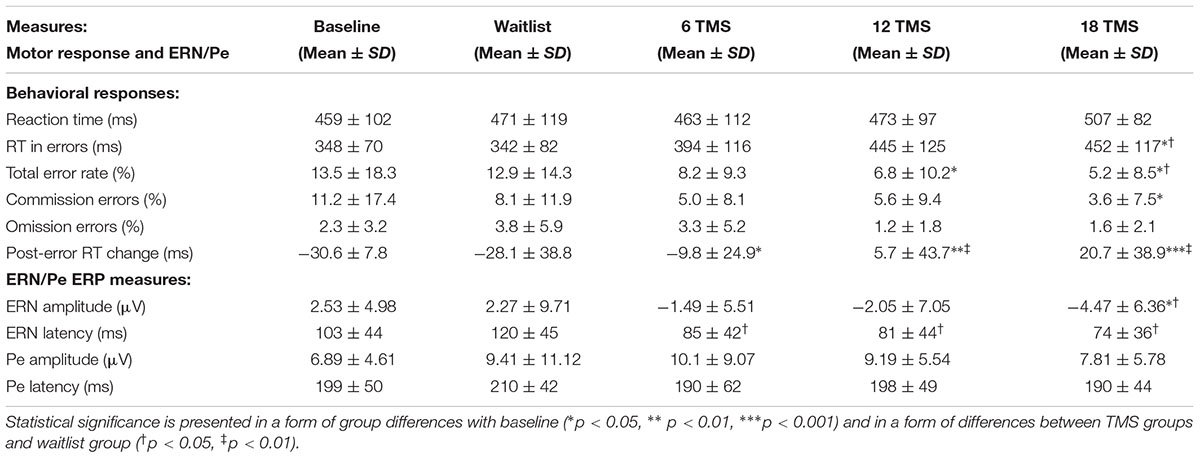
TABLE 1. Mean and standard deviation values of reaction time measures (RT, total accuracy, commission and omission rate, post-error RT change) and stimulus-licked ERN and Pe ERP components during performance on visual oddball task with illusory figures for baseline and for waitlist (N = 26), 6 TMS (N = 22), 12 TMS (N = 24), and 18 TMS (N = 23) groups.
Post-error RT
There were significant differences in post-error RT changes vs. mean RT, i.e., in normative post-error slowing phenomenon. Post error-RT change, calculated as the first post-error RT difference with the mean RT in correct trials, showed significant group differences [F(4,207) = 10.03, p = <0.001]. Statistically significant differences were observed between 18 TMS and baseline (-61.5 ± 12.8 ms, p < 0.001), 18 TMS vs. WTL (-68.1 ± 13.3 ms, p < 0.001), as well as between 12 TMS and baseline (-48.5 ± 12.2 ms, p = 0.004). Both 12 TMS and 18 TMS groups showed normative post-error RT slowing (e.g., 20.6 ± 38.9 ms in 18 TMS), different from the baseline and WTL group test where post-error RT was shorter than mean RT, for instance WTL group showed -28.1 ± 38.8 ms speeding. Figure 3B illustrates group differences in post-error RT changes.
Reaction Time in Correct vs. Commission Error Responses
Main effect of response correctness on RT was significant [F(1,210) = 58.16, p < 0.001]. Group differences (Correctness ×Group) were also significant [F(4,207) = 4.06, p = 0.004, = 0.105, observed power = 0.907]. Post hoc analysis showed significant difference in error RT between 18 TMS group vs. baseline (p = 0.032) with error RT post-18 TMS being slower (452 ± 116 vs. 348 ± 70 ms).
Posterior ERP Components (Parietal P100, P3b)
P100
Transcranial magnetic stimulation session numbers had an effect on both amplitude and latency of parietal P100 component at all conditions (non-Kanizsa standard, non-target Kanizsa distracter, Kanizsa target). F-values (df = 4, 207) were in 14.8–22.36 range with all ps < 0.001. Amplitude of P100 post rTMS (either 6, 12, or 18 rTMS sessions) was lower and latency of P100 longer than at the baseline or in the waitlist group (ps < 0.01), bilaterally for standard, distracter and target stimuli. Analysis of P100 latency showed Hemisphere ×Group interaction [F(4,207) = 5.43, p < 0.001, = 0.113, observed power = 0.972]. This effect was expressed in a prolonged latency of the P100 over the right hemisphere in all TMS groups as compared to baseline and waitlist group (Figure 4A). Comparison of the P100 amplitude of all three post-TMS groups as a subset showed significant differences from mean amplitude at baseline and in the waitlist group (ps < 0.05). Duncan test showed that 6 and 12 sessions of rTMS exerted stronger effect rather than 18 sessions and TMS group subset was not homogenous as compared to baseline and waitlist.
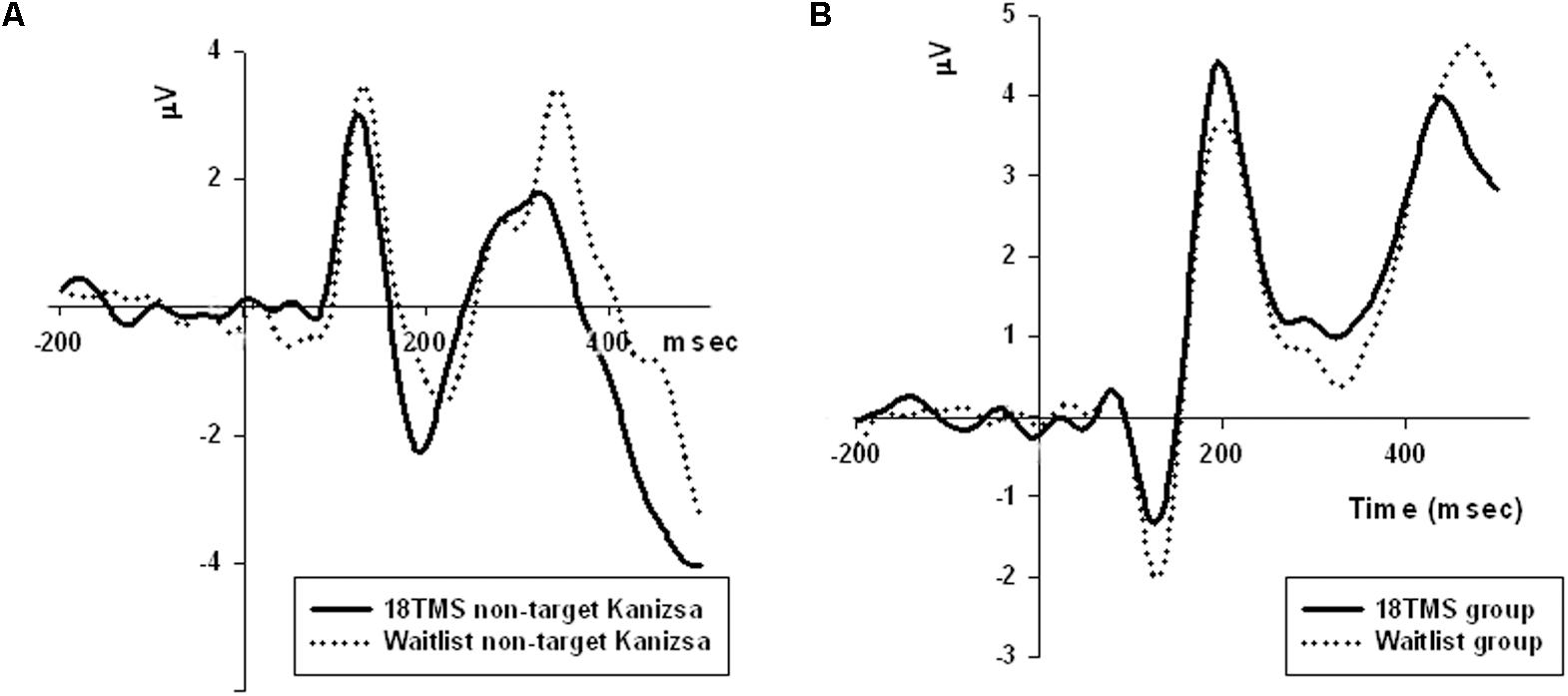
FIGURE 4. (A) Amplitude of P100 component at the parietal sites (ROI of five parietal channels) in response to non-target Kanizsa stimuli was significantly attenuated post-TMS, and is illustrated by comparing ERP waveforms in 18 TMS and waitlist groups. Post-TMS treatment differences (as compared to baseline and waitlist) were statistically different only for standard (p = 0.023) and non-target Kanizsa (p < 0.001) stimuli. (B) Latency of the frontal P3a component to non-target Kanizsa stimuli was shorter in the 18 TMS group as compared to the waitlist (p < 0.01), though amplitude differences were not reaching statistical significance level.
P3b
Stimulus type (standard, distracter, and target) had main effect on P3b amplitude [F(2,209) = 35.72, p < 0.001] and P3b latency [F(2,209) = 15.61, p < 0.001], with amplitude being higher and latencies longer in response to targets as compared to standards and distracters. This difference was statistically significant when post-18 TMS and waiting group P3b amplitude and latencies were compared (respectively, p = 0.016 and p = 0.004). Main effect of group factor was significant both for amplitude and latency (ps < 0.001). The latency of P3b yielded Stimulus × Group interaction [GG-corrected F(8,194) = 3.19, p = 0.003, = 0.066, observed power = 0.851], expressed as bilaterally longer latency to targets rather than to standard and distracter stimuli in the 18 TMS group as compared to the waitlist group (p = 0.004). Similar effect was observed as well in the 6 TMS group (p = 0.006). Post-treatment P3b amplitude group differences were significant for standard (p = 0.023) and distracter (p < 0.001) but not target (p = 0.11) stimuli, though group differences were significant for P3b latency for all type of stimuli (see Figures 5A, 6A and Table 2). Post-12 TMS and 18 TMS amplitude of P3b was lower and latency longer to both standards and distracters as compared to baseline and waitlist (all ps < 0.05). Latency of P3b post-18 TMS was prolonged as compared to waitlist (difference was 33.3 ± 9.4 ms, F = 3.01, p = 0.004) bilaterally. Hemispheric group differences were not significant for both latency and amplitude of P3b component.
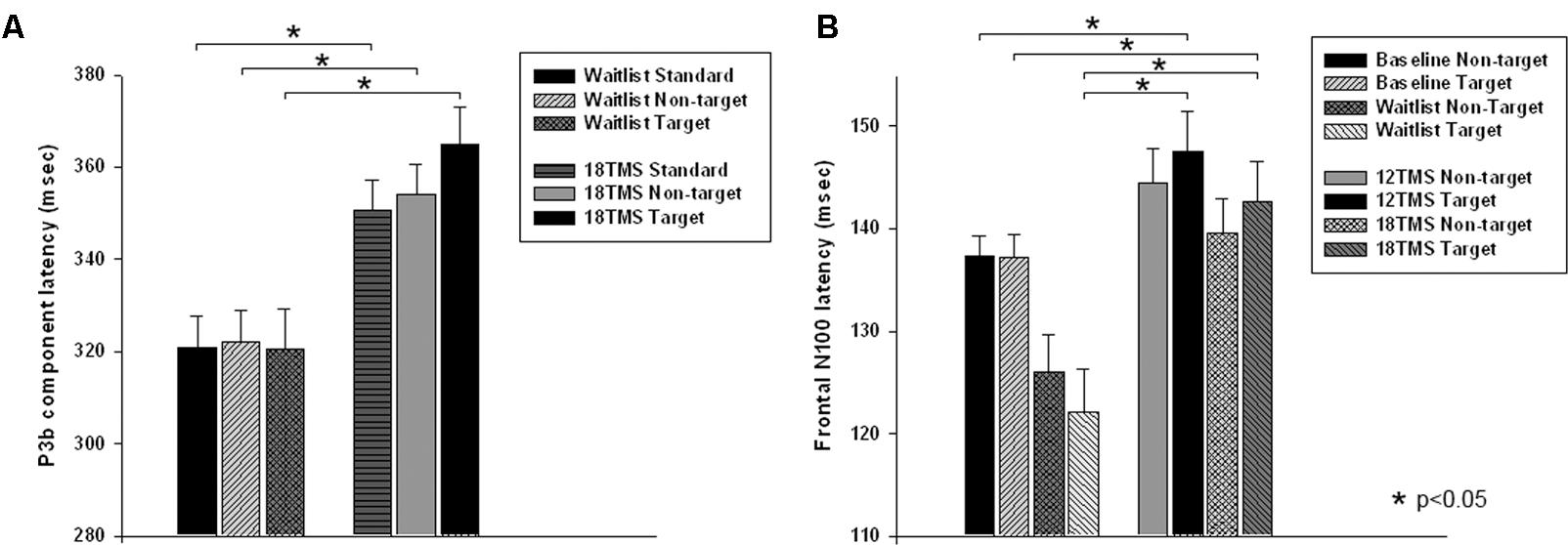
FIGURE 5. (A) Latency of P3b component in response to three types of stimuli (target, rare non-target, and standard) in visual oddball test in the waitlist and 18 TMS groups. Note longer latency of P3b to all stimuli, more significant in response to targets, in the 18 TMS group as compared to the waitlist group. (B) Latency of the frontal N100 component to non-target and target Kanizsa figures show Stimulus ×Group interaction with prolonged latencies of N100 in response to target Kanizsa as compared to non-target Kanizsa distracters in TMS groups. In particular, 12 TMS and 18 TMS groups had longer latencies to targets as compared to the waitlist group (p = 0.001 and p = 0.012, respectively).
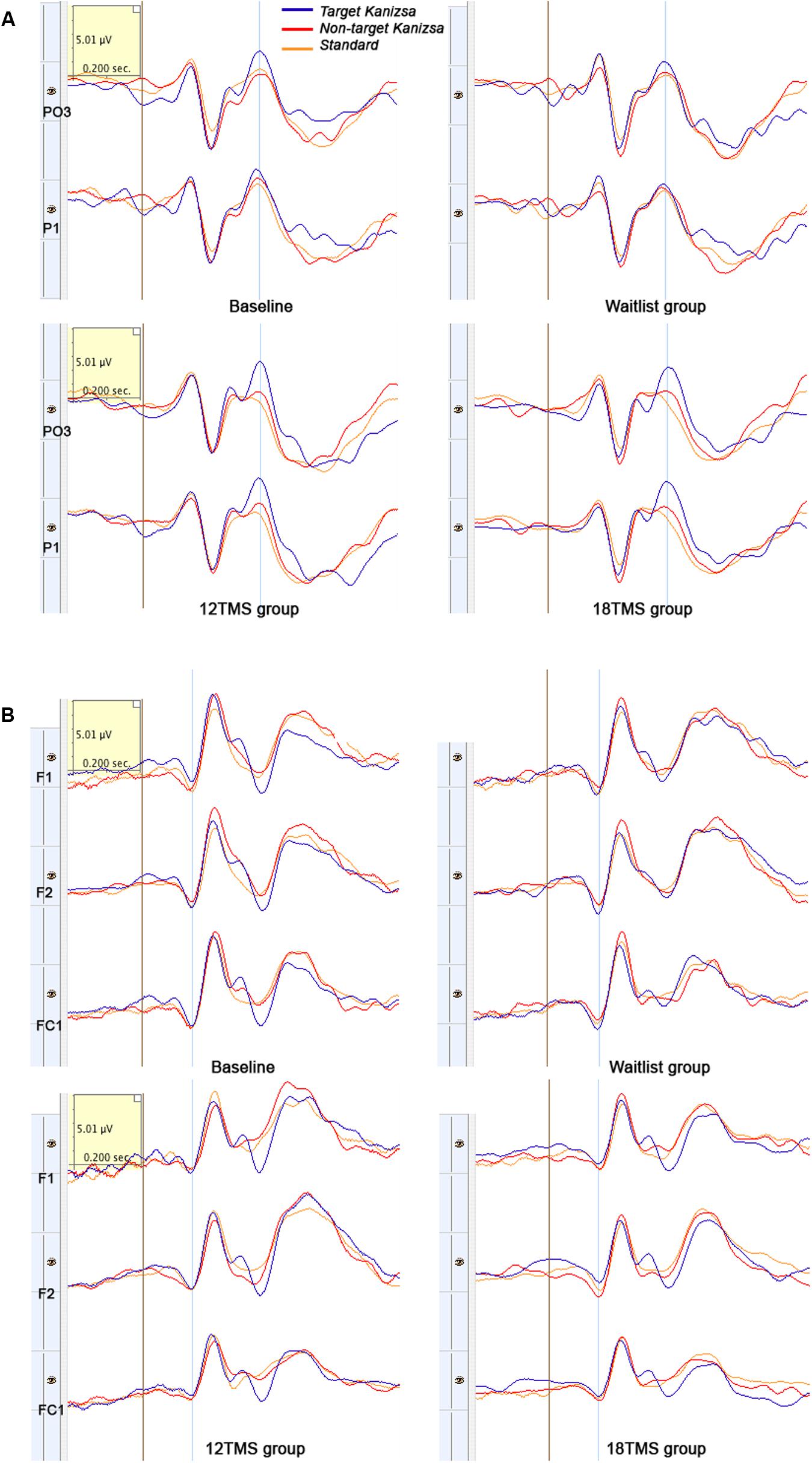
FIGURE 6. (A) Left parieto-occipital (PO3) and parietal (P1) ERPs to target Kanizsa, non-target Kanizsa and non-Kanizsa standard stimuli at baseline, and in waitlist, 12 TMS and 18 TMS groups. Both 12 TMS and 18 TMS groups show lower amplitude of the P3b components (marked by blue line) to non-target stimuli. In the waitlist group ERPs to all three types of stimuli are comparable by their P3b amplitude, especially at the P1 site. (B) Frontal (F1, F2) and fronto-central (FC1) ERPs to three types of stimuli in oddball task at baseline and in waitlist, 12 TMS, and 18 TMS groups. Amplitude to all types of stimuli post-TMS treatment was decreased, more in the 18 TMS group. Note delayed latency and higher amplitude of the P3a component in response to non-target Kanizsa distracter in the waitlist group.
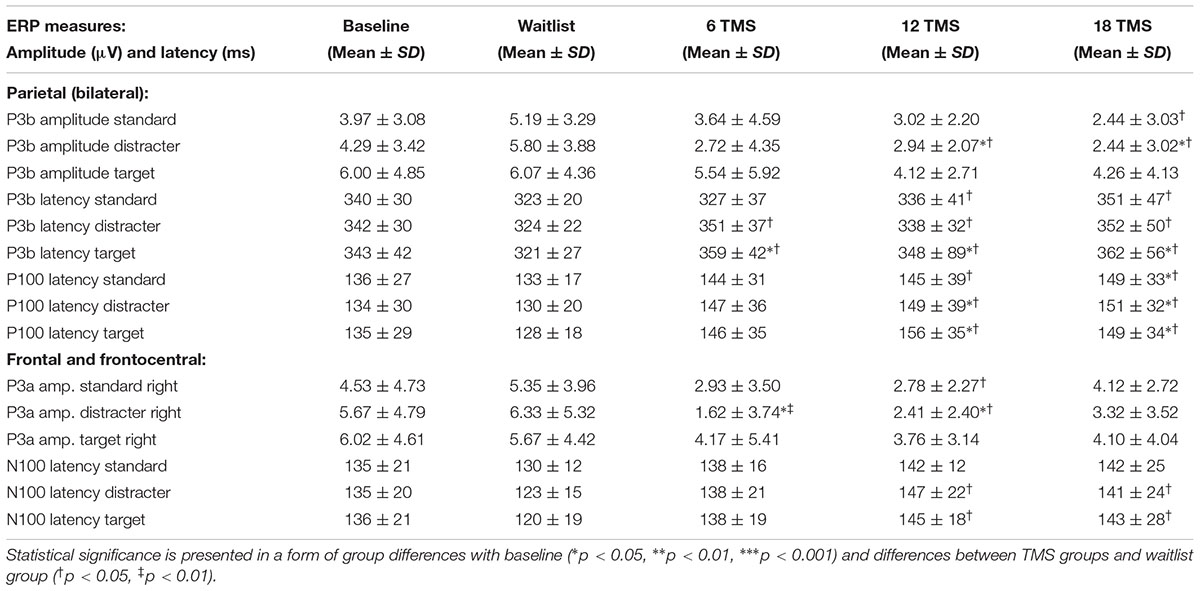
TABLE 2. Mean and standard deviation values of amplitude and latency of the parietal P3 and P100 ERP components and latencies of the frontal P3a and N100 ERP components for baseline and for waitlist (N = 26), 6 TMS (N = 25), 12 TMS (N = 27) and 18 TMS (N = 28) groups.
Anterior ERP Components (Frontal N100, P3a)
N100
Stimulus type had no main effect on frontal N100 amplitude. Analysis of Stimulus and Hemisphere factors effects on N100 latency yielded both Stimulus ×Group [F(8,414) = 2.03, p = 0.042, = 0.040, observed power = 0.825] and Hemisphere × Group [F(4,207) = 40.28, p < 0.001, = 0.287, observed power = 0.991] interactions. These interactions can be described as prolonged latencies of N100 in response to target Kanizsa as compared to non-target Kanizsa distracters in TMS groups with effect being more pronounced at the right hemisphere. In particular, 12 TMS and 18 TMS groups had longer latencies as compared to the waitlist group (p = 0.001 and p = 0.012, respectively). For instance, N100 latency to targets over the right frontal and fronto-central ROI was significantly shorter in waitlist as compared to 12 TMS and 18 TMS groups (123.4 ± 14.6 ms in WTL, 147.2 ± 41.5 ms in 12 TMS, and 142.4 ± 23.5 ms in 18 TMS, all ps < 0.05). Comparison of latency of N100 ERP component in TMS and waitlist groups waitlist showed prolongation to target and shortening in response to non-target Kanizsa figures in the TMS groups, whereas latency of N100 in all conditions remained the same in the WTL group (Figure 5B and Table 2). We could not find any statistically significant group differences in N100 amplitude.
P300 (P3a)
Stimulus type (standard, distracter, and target) bilaterally had main effect on P3a amplitude [F(2,209) = 7.19, p = 0.001] and on P3a latency [F(2,209) = 6.19, p = 0.002].
Group differences in amplitude were significant only for non-target stimuli type at the right hemisphere, specifically for standards (F = 4.18, p = 0.003) and non-target Kanizsa distracters (F = 7.62, p < 0.001, Figure 4B). However, latency of P3a showed group differences in response to all three conditions at both hemispheres (all p < 0.01). Latency of P3a showed significant Hemisphere ×Group interaction [F(4,207) = 23.77, p < 0.001, = 0.334, observed power = 0.992]. The interaction was characterized by lower latency of P3a at the right hemisphere in the TMS groups as compared to waitlist group. Both 12 TMS and 18 TMS groups had shorter latencies to non-target Kanizsa distracters as compared to waitlist group (WTL, 396 ± 41 ms; 12 TMS, 348 ± 35 ms; 18 TMS, 379 ± 42 ms, ps < 0.01). Similar effect was observed for standards in these two TMS groups (ps < 0.01) but not for target Kanizsa stimuli (Figure 6B).
Response-Locked ERPs (Frontal and Fronto-Central ERN/Pe)
Since eight subjects showed insufficient number of commission errors, they were excluded in the statistical analysis. Effects of group factor on ERN amplitude [F(4,199) = 3.75, p = 0.007] and ERN latency (F = 5.54, p = 0.001) were found to be statistically significant. Post hoc analysis showed that the amplitude of ERN in post-18 TMS group was more negative and significantly different both from both baseline (by -7.00 ± 2.30 μV, p = 0.023) and waitlist group (-8.74 ± 2.97 μV, p = 0.032). Latency of ERN post-18 TMS was shorter than in the waitlist group (45.9 ± 14.9 ms, p = 0.022). There were no group differences in amplitude and latency of the Pe component.
Clinical Behavior Evaluations Post- TMS
Repetitive Behavior Scale Outcomes
Repetitive behavior subscales (RBS-R, Bodfish et al., 1999) showed group difference for Stereotype Behavior [F(4,205) = 2.68, p = 0.035, see Figure 7A] and Total Repetitive Behaviors T-score [F(4,205) = 3.26, p = 0.014]. Post hoc analysis showed significant lower T-score of RBS-R in 18 TMS group as compared to baseline (14.6 ± 12.8 vs. 26.5 ± 15.2, p = 0.014). Ritualistic Behavior rating decreased from baseline in the 18 TMS group (from 9.61 ± 6.00 down to 5.55 ± 6.18 post-treatment, p = 0.017, Figure 7A, left). Stereotype Behavior rating in this TMS group also decreased as compared to baseline (from 5.71 ± 4.20 down to 2.73 ± 3.29, p = 0.037, Figure 7A, right).
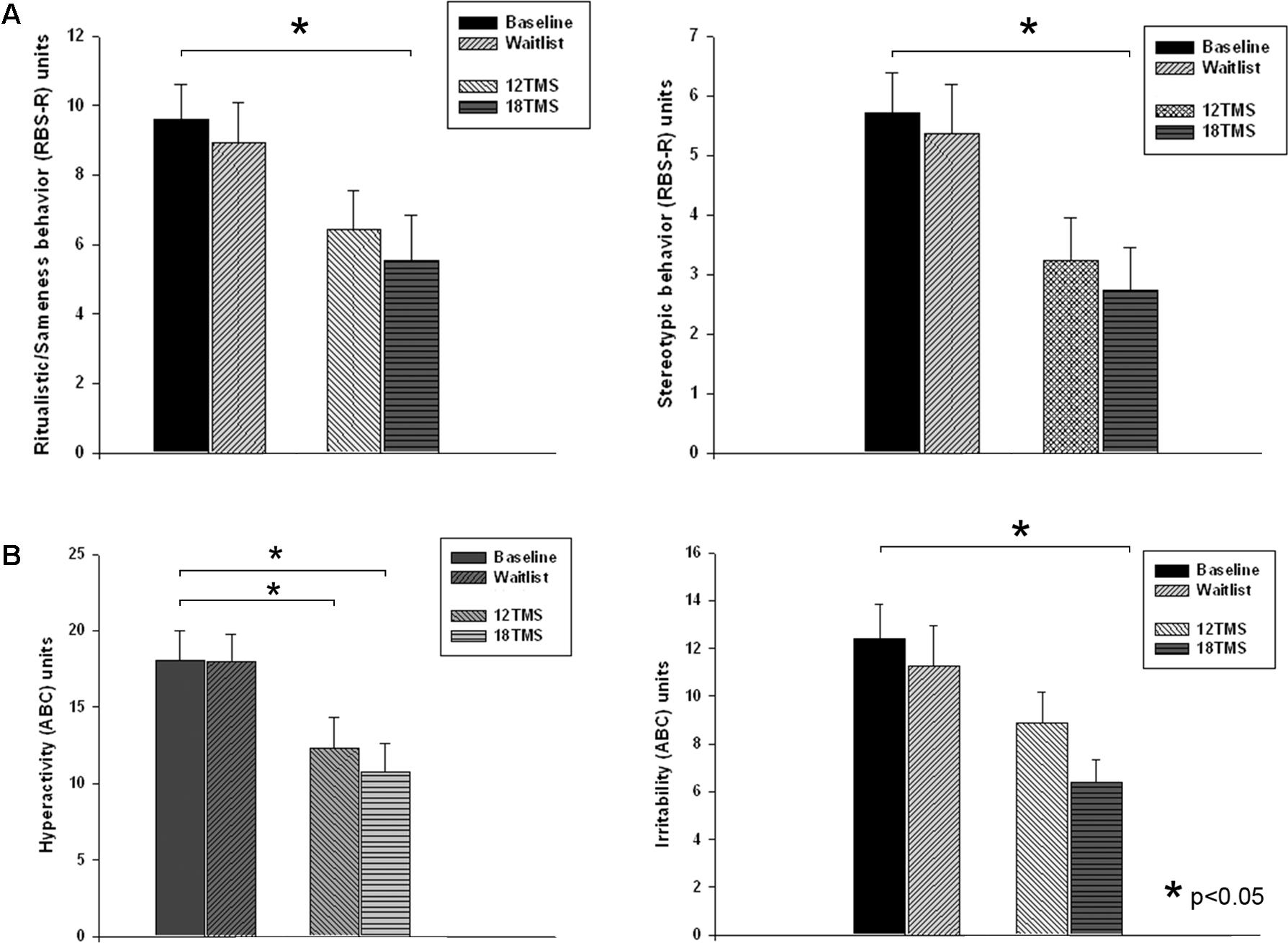
FIGURE 7. (A) Ritualistic/Sameness behavior (left) and Stereotype behavior (right) rating scores of RBS-R questionnaire at baseline, post-waiting period, and post 12 and 18 sessions of rTMS. Most dramatic decrease of scores was observed in the 18TSM group. (B) Hyperactivity (left) and Irritability (right) rating scores of ABC questionnaire at baseline, post-waiting period, and post 12 and 18 sessions of rTMS. The Irritability scores in the 18 TMS group decreased practically in half (–6.01, p = 0.029) as compared to the baseline.
Aberrant Behavior Checklist Outcomes
Three of the ABC (Aman and Singh, 1994) subscales showed significant between-group differences [Irritability, F(4,205) = 3.14, p = 0.028; Lethargy/Social Withdrawal, F(4,205) = 3.52, p = 0.017; and Hyperactivity, F(4,205) = 3.99, p = 0.009]. Post hoc analysis showed significant Irritability scores decrease post-18 TMS vs. baseline (-6.01 ± 2.02, from 12.39 ± 9.63 to 6.38 ± 4.59, p = 0.029, Figure 7B left), as well as Lethargy/Social Withdrawal scores decrease (-5.08 ± 1.78, from 11.50 ± 8.09 to 6.42 ± 5.91, p = 0.040) along with decreased Hyperactivity scores (-7.34 ± 2.81, from 18.09 ± 12.74 to 10.75 ± 9.22, p = 0.049, Figure 7B right).
Discussion
Results of our exploratory clinical research study showed significant changes from baseline in functional measures of performance and behavioral symptoms rating during an oddball task in children with autism following 18 sessions of rTMS treatment. Several functional measures showed a difference from baseline and waitlist in RT and ERP variables after 12 sessions of rTMS, but only a few of them reached statistical significance post-6 session rTMS course. It should be noted, as a limitation of this study, that in this particular group rTMS was administered only over the left DLPFC and this could have biased the outcome. For the purposes of our study, demonstration of preference and advantages of 18 sessions of rTMS applied bilaterally over DLPFC was the most striking finding.
Among the most notable ERP changes in our study are the attenuated amplitude and longer latency of posterior P100 and anterior N100 ERPs to all types of stimuli, with post-TMS changes being more pronounced in response to non-target Kanizsa stimuli. Parietal P3b in response to targets was found to be prolonged without any amplitude change, while latency of P3b to non-targets was shorter in all TMS groups, more so in the 18 TMS group. At the frontal topography, latency of N100 was prolonged to targets and shortened to non-target items in the TMS groups, while remaining unchanged to all stimuli in the waitlist group. Frontal P3a was characterized by a shorter latency to non-target Kanizsa distracters and standards after TMS treatment but not after wait period.
Several behavioral symptoms of autism showed improvement post-TMS according to caregivers’ reports (RBS-R, Bodfish et al., 1999; ABC, Aman and Singh, 1994). Most notable were a decrease of T-score of the RBS-R after 18 sessions of rTMS, along with decreased irritability, lethargy/social withdrawal and hyperactivity rating scores of the ABC questionnaire. Changes in the aforementioned measures of aberrant behavior and repetitive and stereotyped behaviors were comparable to those found in our prior studies where similar parameters and length of TMS intervention were used in children and adolescents with autism (Sokhadze et al., 2014a,b).
In order to better understand the effects of rTMS-based neuromodulation, it is important to briefly review differences in RT, ERN/Pe and stimulus-locked ERPs between children with ASD and neurotypical controls. In a series of studies (Sokhadze et al., 2009b, 2010b, 2013) using different variations of three-stimulus oddball tasks we reported delayed latencies of early and late ERP components to non-target items without significant differences in amplitude characteristics in response to targets in children with autism as compared to neurotypical peers. We interpreted our finding as indicative of a deficient ability to recognize the distinction between task-relevant and task-irrelevant stimuli.
In other studies, using a similar oddball paradigm (Sokhadze et al., 2009b, 2010b; Baruth et al., 2010c), we found, in accordance with the majority of results of RT tasks in children with autism, that differences in motor responses during oddball tests were observed in accuracy measures and in post-error RT adjustments rather than in reaction time (Baruth et al., 2010c; Sokhadze et al., 2012b, 2017). We interpreted these deficits in post-error response speed correction, as well as impulsive perseverative fast post-error response, as a manifestation of an abnormality of error monitoring and correction function (Sokhadze et al., 2010a, 2012b, 2014a,b, 2016, 2017). Deficient magnitude of ERN was reported by other ERP studies (Henderson et al., 2006; Bogte et al., 2007; Thakkar et al., 2008; Vlamings et al., 2008) with an indication that individuals with autism tend to exhibit reduced performance monitoring ability and often fail to adjust behavior after a committed error. This performance monitoring and error correction deficit reflects a lower sensitivity of error detection and a reduced erratic response correction capacity in ASD individuals. Most studies on ERN/Pe components could not find differences in the Pe measure between children with autism and neurotypical children (reviewed in Sokhadze et al., 2010a, 2012b, 2017). In our studies using an oddball paradigm, children with autism did not improve accuracy after committed errors and did not show normative post-error RT slowing (Sokhadze et al., 2012a,b). As a rule, in typical controls the performance on trials immediately following commission errors tend to improve as a result of a change in the speed–accuracy strategy. Implementation of this strategy results in a normative post-error RT slowing and less errors committed; a process dependent on executive control functioning. Impairment in error detection and adjustment of post-error performance in children with autism points at an executive control deficiency that may have negative consequences since effective error detection and correction function is necessary for adequate behavior adjustment (Sokhadze et al., 2010a, 2012b).
Since we are not aware of other studies where ERPs were used as outcomes of rTMS in children with ASD, we can only compare the findings of this study with our own prior preliminary studies (Sokhadze et al., 2009a,b, 2012a, 2014a,b; Baruth et al., 2010a,c; Casanova et al., 2012, 2014b). Most notable, our studies found that neuromodulation based on rTMS facilitated target recognition in reaction time tests. Quite important in this regard was the positive trends for changes in both P3a and P3b potentials and in the enhanced reactivity of these cognitive ERPs to target stimuli vs. non-target stimuli. The latter findings reflect enhanced discrimination of features determining specifics of the target illusory stimuli (i.e., Kanizsa square vs. Kanizsa triangle). A reduction in amplitude and latency of cognitive ERPs (P3a and P3b) to frequent standard non-Kanizsa and rare non-target Kanizsa figures post-TMS in children with autism, especially in the 18 TMS group as compared to the baseline and also waitlist group outcomes, can be considered as a sign of improved target discrimination. Prolonged latency of the frontal P3a to infrequent distracters at baseline test can be considered a potential biomarker of impaired orientation to novelty. In our study, TMS improvement of this measure represents an enhancement of this important integrative function.
Over-activation in the parietal and parieto-occipital cortex at the initial stages of unattended stimuli, along with delayed and prolonged activation of the frontal cortex at the later stages of attended stimuli processing has been reported in children with ASD in a similar visual oddball test with novel distracters (Sokhadze et al., 2010a,b, 2017). We interpreted these results as a manifestation of low selectivity in perceptual processing that leads to an over-processing of unattended stimuli at the later stages. Treatment using low frequency rTMS exerted positive effects on both early and late stages of signal processing and improved ability of less effortful differentiation of targets from non-target items.
The results of the current study indicate that rTMS, the effect being better expressed after 18 rTMS treatment, may facilitate cognitive top-down control and enhance target discrimination specifically during the processing of infrequent task-relevant and task-irrelevant illusory Kanizsa figures. It is noteworthy that the latency of P3b post-TMS was longer to targets and at the same time shorter to both rare non-targets and frequent standards. The P3b is the best studied cognitive component (Polich, 2003, 2007) and has been considered as a marker of task-relevance evaluation, memory-updating and individual trial processing closure (Picton, 1992). Most of the ERP studies in autism focused on outcomes of the endogenous cognitive potentials such as P3b (Courchesne et al., 1989; Ciesielski et al., 1990; Kemner et al., 1994, 1999; Jeste and Nelson, 2009) and to a lesser extent P3a (Townsend et al., 2001). This frontal cognitive potential in our prior studies was delayed but not significantly reduced in children with ASD as compared to neurotypical children (Sokhadze et al., 2009a,b, 2010b, 2012b). In the current study, the P3a component was also found to be prolonged.
Atypicality of exogenous ERP components in individuals with autism has been the object of few studies. Some of these studies have reported abnormalities of sensory perceptual processes, low selectivity both in visual and auditory modalities of stimulation, and most importantly deficits of cognitive control which negatively affects information integration processes (Belmonte and Yurgelun-Todd, 2003). The outcomes of the current study emphasize facilitated target discrimination and enhanced habituation to non-target stimuli post-TMS treatment, more pronounced in the 18 TMS group. Behavioral responses changes (e.g., RT, accuracy) are considered as a very robust measure in oddball tasks, and hence it was very important to find significant improvements in the accuracy of performance on tasks, restoration of normative post-error RT slowing, along with slower RT during commission errors (markers of impulsivity) following 18 session-long rTMS course. Outcomes of 12 session-long rTMS course had similar trends though some of the above indices did not reach the level of statistical significance. In general, our current study indicates improvements in attention, executive functions (e.g., performance monitoring), and enhanced irrelevant response inhibition following rTMS-based intervention in children with ASD. An important addition to our previous studies was the ability to compare the dosage effects of rTMS over different number of sessions.
Executive deficits have always been of interest in autism research (Ozonoff et al., 1991). Abnormalities in error detection, performance monitoring and response conflict resolution help explain many clinically relevant behavioral symptoms present in autism. These executive functions can be readily measured using response-locked ERPs, specifically ERN and Pe (Gehring et al., 1993; Carter et al., 1998; Van Veen and Carter, 2002; Mars et al., 2005; Arbel an Donchin, 2009; Arbel and Donchin, 2011). The ERN is a well-studied ERP with ties to response error processing (Carter et al., 1998; Falkenstein et al., 2000; Gehring and Knight, 2000; Van Veen and Carter, 2002; Davies et al., 2004; Huizinga et al., 2006). The ERN abnormalities, and more specifically attenuated amplitude or delayed latency of this measure, are interpreted as indices of error processing impairments (Ridderinkhof et al., 2004). In this context, it should be outlined that among the most important findings of the current study was a replication of the increase in ERN magnitude following TMS-based neuromodulation that was reported in our prior studies (Sokhadze et al., 2012b, 2014a,b; Casanova et al., 2014b). In these studies, we found enhanced ERN amplitude post-TMS without any Pe changes. This component is thought to reflect conscious assessment that an error was committed (Falkenstein et al., 2000; Nieuwenhuis et al., 2001; Overbeek et al., 2005).
In our preliminary trials using rTMS intervention in children with autism (Sokhadze et al., 2009a,b, 2010b; Baruth et al., 2010a,c) we found that most ERP changes were observed at the early phases of visual signal processing resulting in easier discrimination of targets in oddball task. Enhanced target recognition post-TMS intervention and facilitated pre-attentive inhibition of task-irrelevant stimuli resulted in a lesser load during processing of non-target during later stages. The present study reproduces the positive effects of rTMS on the early ERPs probably due to enhanced suppression of distracters leading to less effortful discrimination of targets from rare non-targets and frequent standards during performance in oddball task (Casanova et al., 2014b; Sokhadze et al., 2017).
Several limitations to the study should be mentioned. One of them is related to the frequency of rTMS sessions, as some of rTMS-based intervention in various psychiatric treatment studies used a more intensive schedule of stimulation (e.g., daily, twice or thrice per week). We selected a weekly regimen because it was better accepted by the families of our study participants. We believe that the effects of rTMS do not wash out in a week’s period, and based on our empirical observations led to good retention, while providing improvements in behavioral symptoms as well as functional outcomes. It is possible to suggest that the length of treatment course (e.g., 12 or 18 weeks) rather than the treatment frequency is one of the main factors of observed behavioral and ERP improvements in our trials in children with autism (Baruth et al., 2010c; Sokhadze et al., 2010b, 2012a, 2017; Casanova et al., 2012). It must be noted that the selected power of rTMS pulses was lower than motor threshold and total number of administered TMS pulses was relatively lower as compared to other established rTMS treatment protocols (e.g., rTMS for major depression). One of the safety related reasons of our intensity preference was the fact that we were among the first groups that started using rTMS in this particular population of children known to be predisposed to seizures.
An additional limitation of the study was the selection of a waiting list group as a form of a control condition instead of using a randomized clinical trial (RCT) design with participants being randomly assigned to either active or sham rTMS group. Custom-made sham coil and interface for double-blinding of TMS delivery are available in our lab, however, they do not mask the muscle contractions of the scalp. There are still several factors that can be investigated using waitlist group design rather than immediately progressing to a double-blind RCT with a sham TMS arm. One of the very important feasibility issues is the number of rTMS sessions and this particular question was addressed in our current study.
Among the limitations that should be considered is the fact that we only stimulated the left hemisphere in the 6 TMS group. This could serve as a confound when making between group comparisons, though all three groups had at least six rTMS treatments over the left DLPFC. Nevertheless, positive changes from the baseline in the 18 TMS group as compared to waitlist group serves to confirm the advantage of this particular dosage of low frequency rTMS over DLPFC for inducing improvements in functional measures of performance on cognitive task and in some of behavioral symptoms of the autism spectrum.
Conclusion
Our comparative effectiveness study confirmed that intervention using low frequency rTMS over DLPFC in children with ASD has positive effects on performance in a visual oddball test and improves parental behavioral questionnaires scores reports. Among the most notable findings were improvements in ERP correlates of effective target discrimination, along with reduced excessive responsiveness to non-target task-irrelevant stimuli, accuracy of behavioral responses, and enhanced behavioral and ERN correlates of effective error detection, monitoring and correction function. Neuromodulation using rTMS significantly reduced rating scores of repetitive and stereotypic behaviors, as well as hyperactivity, social withdrawal and irritability scores according to social and behavioral evaluations. Based on observed changes of magnitude of dependent variables used in our comparative effectiveness study it is possible to conclude that longer courses of neuromodulation using low frequency rTMS offer significant improvements in measures of executive functions and behavior in children with ASD. The study also provides additional support to the statement that low frequency rTMS administered weekly over the DLPFC, with sufficient number of stimulation sessions, is a potentially effective treatment option targeting autistic symptoms such as executive function deficits, and aberrant/repetitive behaviors.
Author Contributions
ES designed ERP test, analyzed ERP data, conducted rTMS stimulation, and participated in interpretation of data and manuscript preparation. EL and EC coordinated study, subjects recruitment, conducted part of statistical analysis, illustrations, and helped in manuscript preparation. DK consulted on all clinical questions related to autism spectrum disorder and participated in manuscript preparation. IO developed method for reaction time and other behavioral measures analysis and interpretation. AT consulted on clinical issues related to autism and other aspects of TMS application in psychiatry, participated in review of manuscript. MC designed approach for TMS application in autism treatment and research, developed theoretical framework of the study, actively participated in interpretation of data, and manuscript preparation for publication.
Funding
This study was partially supported by National Institutes of Health Eureka R01 grant MH86784 to MC.
Conflict of Interest Statement
The authors declare that the research was conducted in the absence of any commercial or financial relationships that could be construed as a potential conflict of interest.
References
Aman, M. G. (2004). Management of hyperactivity and other acting out problems in patients with autism spectrum disorder. Semin. Pediatr. Neurol. 11, 225–228. doi: 10.1016/j.spen.2004.07.006
Aman, M. G., and Singh, N. N. (1994). Aberrant Behavior Checklist—Community. Supplementary Manual. East Aurora, NY: Slosson Educational Publications.
American Psychiatric Association [APA] (2000). Diagnostic and Statistical Manual of Mental Disorders (DSM-IV-TR), 4th Edn. Washington, DC: American Psychiatric Association.
American Psychiatric Association [APA] (2013). Diagnostic and Statistical Manual of Mental Disorders (DSM-V), 5th Edn. Washington, DC: American Psychiatric Association. doi: 10.1176/appi.books.9780890425596
Arbel, Y., and Donchin, E. (2009). Parsing the componential structure of post-error ERPs: a principal component analysis of ERPs following errors. Psychophysiology 46, 1288–1298. doi: 10.1111/j.1469-8986.2009.00857.x
Arbel, Y., and Donchin, E. (2011). When a child errs: the ERN and the Pe complex. Psychophysiology 48, 55–63. doi: 10.1111/j.1469-8986.2010.01042.x
Baron-Cohen, S. (1989). The theory of mind hypothesis of autism: a reply to Boucher. Br. J. Disord. Commun. 24, 199–200. doi: 10.3109/13682828909011956
Baron-Cohen, S. (2004). The cognitive neuroscience of autism. J Neurol. Neurosurg. Psychiatry 75, 945–948. doi: 10.1136/jnnp.2003.018713
Baruth, J. M., Casanova, M. F., El-Baz, A., Horrell, T., Mathai, G., Sears, L., et al. (2010a). Low-frequency repetitive transcranial magnetic stimulation modulates evoked-gamma frequency oscillations in autism spectrum disorders. J. Neurother. 14, 179–194.
Baruth, J. M., Casanova, M. F., Sears, L., and Sokhadze, E. (2010b). Early-stage visual processing abnormalities in high-functioning autism spectrum disorder (ASD). Transl. Neurosci. 1, 177–187.
Baruth, J. M., Sokhadze, E., El-Baz, A., Mathai, G., Sears, L., and Casanova, M. F. (2010c). “Transcranial magnetic stimulation as a treatment for autism,” in Cutting Edge Therapies for Autism, eds K. Siri and T. Lyons (New York, NY: Skyhorse Publishing), 388–397.
Belmonte, M. K., Allen, G., Beckel-Mitchener, A., Boulanger, L. M., Carper, R. A., and Webb, S. J. (2004). Autism and abnormal development of brain connectivity. J. Neurosci. 24, 9228–9231. doi: 10.1523/JNEUROSCI.3340-04.2004
Belmonte, M. K., and Yurgelun-Todd, D. A. (2003). Functional anatomy of impaired selective attention and compensatory processing in autism. Brain Res. Cogn. Brain Res. 17, 651–664. doi: 10.1016/S0926-6410(03)00189-7
Bodfish, J. W., Symons, F. J., and Lewis, M. H. (1999). Repetitive Behavior Scale. Morganton, NC: Western Carolina Center Research Reports.
Bodfish, J. W., Symons, F. S., Parker, D. E., and Lewis, M. H. (2000). Varieties of repetitive behavior in autism: comparisons to mental retardation. J. Autism Dev. Disord. 30, 237–243. doi: 10.1023/A:1005596502855
Bogte, H., Flamma, B., van der Meere, J., and van Engeland, H. (2007). Post-error adaptation inadults with high functioning autism. Neuropsychologia 45, 1707–1714. doi: 10.1016/j.neuropsychologia.2006.12.020
Carter, C. S., Braver, T. S., Barch, D. M., Botvinick, M. M., Noll, D., and Cohen, J. D. (1998). Anterior cingulate cortex, error detection, and the online monitoring of performance. Science 280, 747–749. doi: 10.1126/science.280.5364.747
Casanova, M. F. (2005). “Minicolumnar pathology in autism,” in Recent Developments in Autism Research, ed. M. F. Casanova (New York, NY: Nova Biomedical Books), 133–144.
Casanova, M. F. (2007). The neuropathology of autism. Brain Pathol. 17, 422–433. doi: 10.1111/j.1750-3639.2007.00100.x
Casanova, M. F., Baruth, J., El-Baz, A., Tasman, A., Sears, L., and Sokhadze, E. (2012). Repetitive transcranial magnetic stimulation (rTMS) modulates event-related potential (ERP) indices of attention in autism. Transl. Neurosci. 3, 170–180. doi: 10.2478/s13380-012-0022-0
Casanova, M. F., Buxhoeveden, D., and Gomez, J. (2003). Disruption in the inhibitory architecture of the cell minicolumns: implications for autism. Neuroscientist 9, 496–507. doi: 10.1177/1073858403253552
Casanova, M. F., Buxhoeveden, D. P., and Brown, C. (2002a). Clinical and macroscopic correlates of minicolumnar pathology in autism. J. Child Neurol. 17, 692–695.
Casanova, M. F., Buxhoeveden, D. P., Switala, A. E., and Roy, E. (2002b). Minicolumnar pathology in autism. Neurology 58, 428–432. doi: 10.1212/WNL.58.3.428
Casanova, M. F., Buxhoeveden, D. P., Switala, A. E., and Roy, E. (2002c). Asperger’s syndrome and cortical neuropathology. J. Child Neurol. 17, 142–145. doi: 10.1177/088307380201700211
Casanova, M. F., El-Baz, A. S., Kamat, S. S., Dombroski, B. A., Khalifa, F., Elnakib, A., et al. (2014a). Focal cortical displasias in autism spectrum disorders. Acta Neuropathol. Commun. 1:67. doi: 10.1186/2051-5960-1-67
Casanova, M. F., Hensley, M. K., Sokhadze, E. M., El-Baz, A. S., Wang, Y., Li, X., et al. (2014b). Effects of weekly low-frequency rTMS on autonomic measures in children with autism spectrum disorders. Front. Hum. Neurosci. 8:851. doi: 10.3389/fnhum.2014.00851
Casanova, M. F., Sokhadze, E., Opris, I., Wang, Y., and Li, X. (2015). Autism spectrum disorders: linking neuropathological findings to treatment with transcranial magnetic stimulation. Acta Pediatr. 104, 346–355. doi: 10.1111/apa.12943
Casanova, M. F., van Kooten, I., Switala, A. E., van England, H., Heinsen, H., Steinbuch, H. W., et al. (2006a). Abnormalities of cortical minicolumnar organization in the prefrontal lobes of autistic patients. Clin. Neurosci. Res. 6, 127–133. doi: 10.1016/j.cnr.2006.06.003
Casanova, M. F., van Kooten, I., van Engeland, H., Heinsen, H., Steinbursch, H. W. M., Hof, P. R., et al. (2006b). Minicolumnar abnormalities in autism II. Neuronal size and number. Acta Neuropathol. 112, 287–303. doi: 10.1007/s00401-006-0085-5
Ciesielski, K. T., Courchesne, E., and Elmasian, R. (1990). Effects of focused attention tasks on event-related potentials in autistic and normal individuals. Electroencephalogr. Clin. Neurophysiol. 75, 207–220. doi: 10.1016/0013-4694(90)90174-I
Courchesne, E., Lincoln, A. J., Yeung-Courchesne, R., Elmasian, R., and Grillon, C. (1989). Pathophysiologic findings in nonretarded autism and receptive developmental disorder. J. Autism Dev. Disord. 19, 1–17. doi: 10.1007/BF02212714
Croarkin, P. E., Wall, C. A., and Lee, J. (2011). Applications of transcranial magnetic stimulation (TMS) in child and adolescent psychiatry. Int. Rev. Psychiatry 23, 445–453. doi: 10.3109/09540261.2011.623688
Dapretto, M., Davies, M. S., Pfeifer, J. H., Scott, A. A., Sigman, M., Bookheimer, S. Y., et al. (2006). Understanding emotions in others: mirror neuron dysfunction in children withautism spectrum disorders. Nat. Neurosci. 9, 28–30. doi: 10.1038/nn1611
Daskalakis, Z. J., Christensen, B. K., Fitzgerald, P. B., and Chen, R. (2002). Transcranial magnetic stimulation: a new investigational and treatment tool in psychiatry. J. Neuropsychiatry Clin. Neurosci. 14, 406–415. doi: 10.1176/jnp.14.4.406
Davies, P. L., Segalowitz, S. J., and Gavin, W. J. (2004). Development of response-monitoring ERPs in 7- to 25-year-olds. Dev. Neuropsychol. 25, 355–376. doi: 10.1207/s15326942dn2503_6
Enticott, P. G., Fitzgibbon, B. M., Kennedy, H. A., Arnold, S. L., Elliot, D., Peachey, A., et al. (2014). A double-blind, randomized trial of deep repetitive transcranial magnetic stimulation (rTMS) for autism spectrum disorder. Brain Stimul. 7, 206–211. doi: 10.1016/j.brs.2013.10.004
Falkenstein, M., Hoormann, J., Christ, S., and Hohnsbein, J. (2000). ERP components on reaction errors and their functional significance: a tutorial. Biol. Psychol. 51, 87–107. doi: 10.1016/S0301-0511(99)00031-9
Franken, I. H., van Strien, J. W., Franzek, E. J., and van de Wetering, B. J. (2007). Error-processing deficits in patients with cocaine dependence. Biol. Psychol. 75, 45–51. doi: 10.1016/j.biopsycho.2006.11.003
Frith, U., and Happé, F. (1994). Autism: beyond theory of mind. Cognition 50, 115–132. doi: 10.1016/0010-0277(94)90024-8
Garvey, M. A., and Gilbert, D. L. (2004). Transcranial magnetic stimulation in children. Eur. J. Paediatr. Neurol. 8, 7–19. doi: 10.1016/j.ejpn.2003.11.002
Garvey, M. A., and Mall, V. (2008). Transcranial magnetic stimulation in children. Clin. Neurophysiol. 119, 973–984. doi: 10.1016/j.clinph.2007.11.048
Gehring, W. J., Goss, B., Coles, M. G., Meyer, D. E., and Donchin, E. (1993). A neural system for error detection and compensation. Psychol. Sci. 4, 385–390. doi: 10.1118/1.4868459
Gehring, W. J., and Knight, R. T. (2000). Prefrontal-cingulate interactions in action monitoring. Nat. Neurosci. 3, 516–520. doi: 10.1038/74899
George, M. S., Lisanby, S. H., and Sackeim, H. A. (1999). Transcranial magnetic stimulation: applications in neuropsychiatry. Arch. Gen. Psychiatry 56, 300–331. doi: 10.1001/archpsyc.56.4.300
George, M. S., Nahas, J., Kozol, F. A., Li, X., Yamanaka, K., Mishory, A., et al. (2003). Mechanisms and the current state of transcranial magnetic stimulation. CNS Spectr. 8, 496–514. doi: 10.1017/S1092852900018976
Gershon, A. A., Dannon, P. N., and Grunhaus, L. (2003). Transcranial magnetic stimulation in the treatment of depression. Am. J. Psychiatr. 160, 835–845. doi: 10.1176/appi.ajp.160.5.835
Gilbert, D. L., Garvey, M. A., Bansal, A. S., Lipps, T., Zhang, J., and Wassermann, E. M. (2004). Should transcranial magnetic stimulation research in children be considered minimal risk? Clin. Neurophysiol. 115, 1730–1739. doi: 10.1016/j.clinph.2003.10.037
Henderson, H., Schwartz, C., Mundy, P., Burnette, C., Sutton, S., Zahka, N., et al. (2006). Response monitoring, the error-related negativity, and differences in social behavior in autism. Brain Cogn. 61, 96–109. doi: 10.1016/j.bandc.2005.12.009
Hoffman, R. E., and Cavus, I. (2002). Slow transcranial magnetic stimulation, long-term depotentiation, and brain hyperexcitability disorders. Am. J. Psychiatr. 159, 1093–1102. doi: 10.1176/appi.ajp.159.7.1093
Hong, Y. H., Wu, S. W., Pedapati, E. V., Hom, P. S., Huddleston, D. A., Laue, C. S., et al. (2015). Safety and tolerability of theta burst stimulation vs. single and paired pulse transcranial magnetic stimulation: a comparative study of 165 pediatric subjects. Front. Hum. Neurosci. 9:29. doi: 10.3389/fnhum.2015.00029
Huizinga, M., Dolan, C. V., and Van DerMolen, M. W. (2006). Age-related change in executive function: developmental trends and a latent variable analysis. Neuropsychologia 44, 2017–2036. doi: 10.1016/j.neuropsychologia.2006.01.010
Jeste, S. S., and Nelson, C. A. III (2009). Event related potentials in the understanding of autism spectrum disorders: an analytical review. J. Autism Dev. Disord. 39, 495–510. doi: 10.1007/s10803-008-0652-9
Just, M. A., Cherkassky, V. L., Keller, T. A., and Minshew, N. J. (2004). Cortical activation and synchronization during sentence comprehension in high-functioning autism: evidence of underconnectivity. Brain 127, 1811–1821. doi: 10.1093/brain/awh199
Kanizsa, G. (1976). Subjective contours. Sci. Am. 235, 48–52. doi: 10.1038/scientificamerican0476-48
Kemner, C., van der Gaag, R. J., Verbaten, M., and van Engeland, H. (1999). ERP differences among subtypes of pervasive developmental disorders. Biol. Psychiatry 46, 781–789. doi: 10.1016/S0006-3223(99)00003-7
Kemner, C., Verbaten, M. N., Cuperus, J. M., Camfferman, G., and Van Engeland, H. (1994). Visual and somatosensory event-related brain potentials in autistic children and three different control groups. Electroencephalogr. Clin. Neurophysiol. 92, 225–237.
Klomjai, W., Katz, R., and Lackmy-Vallee, A. (2015). Basic principles of transcranial magnetic stimulation (TMS) and repetitive TMS (rTMS). Ann. Phys. Rehabil. Med. 58, 208–213. doi: 10.1016/j.rehab.2015.05.005
Krishnan, C., Santos, L., Peterson, M. D., and Ehinger, M. (2015). Safety of noninvasive brain stimulation in children and adolescents. Brain Stimul. 8, 76–87. doi: 10.1016/j.brs.2014.10.012
Le, K., Liu, L., Sun, M., Hu, L., and Xiao, N. (2013). Transcranial magnetic stimulation at 1 Hz improves clinical symptoms in children with Tourette syndrome for at least 6 months. J. Clin. Neurosci. 20, 257–262. doi: 10.1016/j.jocn.2012.01.049
Le Couteur, A., Lord, C., and Rutter, M. (2003). The Autism Diagnostic Interview—Revised (ADI-R). Los Angeles, CA: Western Psychological Services.
Loo, C., and Mitchell, P. (2005). A review of the efficacy of transcranial magnetic stimulation (TMS) treatment for depression, and current and future strategies to optimize efficacy. J. Affect. Disord. 88, 255–267. doi: 10.1016/j.jad.2005.08.001
Luu, P., Tucker, D. M., Englander, R., Lockfeld, A., Lutsep, H., and Oken, B. (2001). Localizing acute stroke-related EEC changes: assessing the effects of spatial undersampling. J. Clin. Neurophysiol. 18, 302–317. doi: 10.1097/00004691-200107000-00002
Maeda, F., Keenan, J. P., Tormos, J. M., Topka, H., and Pascual-Leone, A. (2000). Modulation of corticospinal excitability by repetitive transcranial magnetic stimulation. Clin. Neurophysiol. 111, 800–805. doi: 10.1016/S1388-2457(99)00323-5
Mars, R. B., Coles, M. G., Grol, M. J., Holroyd, C. B., Nieuwenhuis, S., Hulstijn, W., et al. (2005). Neural dynamics of error processing in medial frontal cortex. Neuroimage 28, 1007–1013. doi: 10.1016/j.neuroimage.2005.06.041
Minshew, N. J., Goldstein, G., and Siegel, D. J. (1997). Neuropsychologic functioning in autism: profile of a complex information processing disorder. Int. Neuropsychol. Soc. 3, 303–316.
Mir-Moghtadaei, A., Caballero, R., Fried, P., Fox, M. D., Lee, K., Giacobbe, P., et al. (2015). Concordance between BeamF3 and MRI-neuronavigated target sites for repetitive transcranial magnetic stimulation of the left dorsolateral prefrontal cortex. Brain Stimul. 8, 965–973. doi: 10.1016/j.brs.2015.05.008
Murphy, K. R., and Myors, B. (2004). Statistical Power Analysis. A Simple and General Model for Traditional and Modern Hypothesis Tests, 2nd Edn. Mahwah, NJ: Lawrence Erlbaum Associates.
Ni, H. C., Hung, J., Wu, C. T., Wu, Y. Y., Chang, C. J., Chen, R. S., et al. (2017). The impact of single session intermittent theta-burst stimulation over the dorsolateral prefrontal cortex and posterior superior temporal sulcus on adults with autism spectrum disorder. Front. Neurosci. 11:255. doi: 10.3389/fnins.2017.00255
Nieuwenhuis, S., Ridderinkhof, K. R., Blom, J., Band, G. P., and Kok, A. (2001). Error-related brain potentials are differentially related to awareness of response errors: evidence from an antisaccade task. Psychophysiology 38, 752–760. doi: 10.1111/1469-8986.3850752
Oberman, L., Ifert-Miller, F., Najib, U., Bashir, S., Woollacott, I., Gonzalez-Heydrich, J., et al. (2010). Transcranial magnetic stimulation provides means to assess cortical plasticity and excitability in humans with fragile X syndrome and autism spectrum disorder. Front. Synaptic Neurosci. 2:26. doi: 10.3389/fnsyn.2010.00026
Oberman, L. M., and Enticott, P. G. (2015). The safety and efficacy of noninvasive brain stimulation in development and neurodevelopmental disorders. Front. Hum. Neurosci. 9:544. doi: 10.3389/fnhum.2015.00544
Oberman, L. M., Enticott, P. G., Casanova, M. F., Rotenberg, A., Pascual-Leone, A., McCracken, J. T., et al. (2016). Transcranial magnetic stimulation in autism spectrum disorder: challenges, promise, and roadmap for future research. Autism Res. 9, 184–203. doi: 10.1002/aur.1567
Oberman, L. M., Rotenberg, A., and Pascual-Leone, A. (2015). Use of transcranial magnetic stimulation in autism spectrum disorders. J. Autism Dev. Disord. 45, 524–536. doi: 10.1007/s10803-013-1960-2
Opris, I., Lebedev, M. A., and Nelson, R. J. (2016). Neostriatal neuronal activity correlates better with movement kinematics under certain reward. Front. Neurosci. 10:336. doi: 10.3389/fnins.2016.00336
Overbeek, T. J. M., Nieuwenhuis, S., and Ridderinkhof, K. R. (2005). Dissociable components of error processing. J. Psychophysiol. 19, 319–329. doi: 10.1027/0269-8803.19.4.319
Ozonoff, S., Pennington, B., and Rogers, S. J. (1991). Executive function deficits in high-functioning autistic individuals: relationship to theory of mind. J. Child Psychol. Psychiatry 32, 1081–1105. doi: 10.1111/j.1469-7610.1991.tb00351.x
Pascual-Leone, A., Davey, N. J., Rothwell, J., Wasserman, E. M., and Puri, B. K. (2002). Handbook of Transcranial Magnetic Stimulation. New York, NY: Oxford University Press.
Pascual-Leone, A., Walsh, V., and Rothwell, J. (2000). Transcranial magnetic stimulation in cognitive neuroscience–virtual lesion, chronometry, and functional connectivity. Curr. Opin. Neurobiol. 10, 232–237. doi: 10.1016/S0959-4388(00)00081-7
Picton, T. W. (1992). The P300 wave of the human event-related potential. J. Clin. Neurophysiol. 9, 456–479. doi: 10.1097/00004691-199210000-00002
Polich, J. (2003). “Theoretical overview of P3a and P3b,” in Detection of Change. Event-related Potential and fMRI Findings, ed. J. Polich (Boston, MA: Kluwer Academic Press).
Polich, J. (2007). Updating P300: an integrative theory of P3a and P3b. J. Clin. Neurophysiol. 118, 2128–2148. doi: 10.1016/j.clinph.2007.04.019
Pommier, B., Vassal, F., Boutet, C., Jeannin, S., Peyron, R., and Faillenot, I. (2017). Easy methods to make the neuronavigated targeting of DLPFC accurate and routinely accessible for rTMS. Neurophysiol. Clin. 7, 35–46. doi: 10.1016/j.neucli.2017.01.007
Quintana, H. (2005). Transcranial magnetic stimulation in persons younger than the age of 18. J. ECT 21, 89–95. doi: 10.1097/01.yct.0000162556.02720.58
Ridderinkhof, K. R., Ullsperger, M., Crone, E. A., and Nieuwenhuis, S. (2004). The role of the medial frontal cortex in cognitive control. Science 306, 443–447. doi: 10.1126/science.1100301
Rossi, S., Hallet, M., Rossini, P., Pascual-Leone, A., and Safety of Tms Consensus Group (2009). Safety, ethical considerations, and application guidelines for the use of transcranial magnetic stimulation in clinical practice and research. Clin. Neurophysiol. 120, 2008–2039. doi: 10.1016/j.clinph.2009.08.016
Rotenberg, A., Horvath, J. C., and Pascual-Leone, A. (2014). Transcranial Magnetic Stimulation. New York, NY: Human Press.
Rubenstein, J. L., and Merzenich, M. M. (2003). Model of autism: increased ratio of excitation/inhibition in key neural systems. Gen. Brain Behav. 2, 255–267. doi: 10.1034/j.1601-183X.2003.00037.x
Rudiak, D., and Marg, E. (1994). Finding the depth of magnetic brain stimulation: a re-evaluation. Electroencephalogr. Clin. Neurophysiol. 93, 358–371. doi: 10.1016/0168-5597(94)90124-4
Sokhadze, E., Baruth, J., El-Baz, A., Horrell, T., Sokhadze, G., Carroll, T., et al. (2010a). Impaired error monitoring and correction function in autism. J. Neurother. 14, 79–95.
Sokhadze, E., Baruth, J., Tasman, A., Mansoor, M., Ramaswamy, R., Sears, L., et al. (2010b). Low-frequency repetitive transcranial magnetic stimulation (rTMS) affects event-related potential measures of novelty processing in autism. Appl. Psychophysiol. Biofeedback 35, 147–161. doi: 10.1007/s10484-009-9121-2
Sokhadze, E., Baruth, J., Tasman, A., Sears, L., Mathai, G., El-Baz, A., et al. (2009a). Event-related potential study of novelty processing abnormalities in autism. Appl. Psychophysiol. Biofeedback 34, 37–51. doi: 10.1007/s10484-009-9074-5
Sokhadze, E., El-Baz, A., Baruth, J., Mathai, G., Sears, L., and Casanova, M. F. (2009b). Effects of a low-frequency repetitive transcranial magnetic stimulation (rTMS) on induced gamma frequency oscillations and event-related potentials during processing of illusory figures in autism spectrum disorders. J. Autism Dev. Disord. 39, 619–634. doi: 10.1007/s10803-008-0662-7
Sokhadze, E., Baruth, J. M., Sears, L., Sokhadze, G. E., El-Baz, A. S., and Casanova, M. F. (2012a). Prefrontal neuromodulation using rTMS improves error monitoring and correction functions in autism. Appl. Psychophysiol. Biofeedback 37, 91–102. doi: 10.3389/fnsys.2014.00134
Sokhadze, E., Baruth, J. M., Sears, L., Sokhadze, G. E., El-Baz, A. S., Williams, E. L., et al. (2012b). Event-related potential study of attention regulation during illusory figure categorization task in ADHD, Autism Spectrum Disorder, and typical children. J. Neurother. 16, 12–31.
Sokhadze, E. M., Casanova, M. F., and Baruth, J. (2013). “Transcranial magnetic stimulation inautism spectrum disorders,” in Transcranial Magnetic Stimulation: Methods, Clinical Uses and Effect on the Brain, ed. L. Alba-Ferrara (New York, NY: NOVA Publishers), 219–231.
Sokhadze, E. M., Casanova, M. F., Casanova, E., Kelly, D. P., Khachidze, I., Wang, Y., et al. (2017). “Applications of ERPs in autism research and as functional outcomes of neuromodulation treatment,” in Event-Related Potential (ERP): Methods, Outcomes and Research Insights, ed. S. R. Harris (New York, NY: NOVA Science Publishers), 27–88.
Sokhadze, E. M., Casanova, M. F., El-Baez, A. S., Farag, H. E., Li, X., and Wang, Y. (2016). TMS-based neuromodulation of evoked and induced gamma oscillations and event-related potentials in children with autism. Neuroregulation 3, 101–126. doi: 10.15540/nr.3.3.101
Sokhadze, E. M., El-Baz, A. S., Sears, L. L., Opris, I., and Casanova, M. F. (2014a). rTMS neuromodulation improves electrocortical functional measures of information processing and behavioral responses in autism. Front. Syst. Neurosci. 8:134. doi: 10.3389/fnsys.2014.00134
Sokhadze, E. M., El-Baz, A. S., Tasman, A., Sears, L. L., Wang, Y., Lamina, E. V., et al. (2014b). Neuromodulation integrating rTMS and neurofeedback for the treatment of autism spectrum disorder: an exploratory study. Appl. Psychophysiol. Biofeedback 39, 237–257. doi: 10.1007/s10484-014-9264-7
Srinivasan, R., Tucker, D. M., and Murias, M. (1998). Estimating the spatial Nyquist of the human EEG. Behav. Res. Meth. Instrum. Comput. 30, 8–19. doi: 10.3758/BF03209412
Thakkar, K. N., Polli, F. E., Joseph, R. M., Tuch, D. S., Hadjikhani, N., Barton, J., et al. (2008). Response monitoring, repetitive behaviour and anterior cingulate abnormalities in autism spectrum disorders (ASD). Brain 131, 2464–2478. doi: 10.1093/brain/awn099
Townsend, J., Westerfield, M., Leaver, E., Makeig, S., Jung, T., Pierce, K., et al. (2001). Event-related brain response abnormalities in autism: evidence for impaired cerebello-frontal spatial attention networks. Brain Res. Cogn. Brain Res. 11, 127–145. doi: 10.1016/S0926-6410(00)00072-0
Uzunova, G., Pallanti, S., and Hollander, E. (2016). Excitatory/inhibitory imbalance in autismspectrum disorders: implications for interventions and therapeutics. World J. Biol. Psychiatry 17, 174–186. doi: 10.3109/15622975.2015.1085597
Van Veen, V., and Carter, C. S. (2002). The timing of action-monitoring process in the anterior cingulate cortex. J. Cogn. Neurosci. 14, 593–602. doi: 10.1162/08989290260045837
Vlamings, P. H., Jonkman, L. M., Hoeksma, M. R., van Engeland, H., and Kemner, C. (2008). Reduced error monitoring in children with autism spectrum disorder: an ERP study. Eur. J. Neurosci. 28, 399–406. doi: 10.1111/j.1460-9568.2008.06336.x
Wagner, T., Rushmore, J., Eden, U., and Valero-Cabre, A. (2009). Biophysical foundations underlying TMS: setting the stage for an effective use of neurostimulation in the cognitive neurosciences. Cortex 45, 1025–1034. doi: 10.1016/j.cortex.2008.10.002
Walter, G., Tormos, J. M., Isrel, J. A., and Pascual-Leone, A. (2001). Transcranial magnetic stimulation in young persons: a review of known cases. J. Child Adolesc. Psychopharmacol. 11, 69–75. doi: 10.1089/104454601750143483
Wang, Y., Hensley, M. K., Tasman, A., Sears, L., Casanova, M. F., and Sokhadze, E. M. (2016). Heart rate variability and skin conductance during repetitive TMS course in children with autism. Appl. Psychophysiol. Biofeedback 40, 47–60. doi: 10.1007/s10484-015-9311-z
Wassermann, E. M., and Lisanby, S. H. (2001). Therapeutic application of repetitive transcranial magnetic stimulation: a review. Clin. Neurophysiol. 112, 1367–1377. doi: 10.1016/S1388-2457(01)00585-5
Wassermann, E. M., and Zimmermann, T. (2012). Transcranial magnetic stimulation: therapeutic promises and scientific gaps. Pharmacol. Ther. 133, 98–107. doi: 10.1016/j.pharmthera.2011.09.003
Wechsler, D. (1999). Wechsler Abbreviated Scale of Intelligence. San Antonio, TX: Harcourt Assessment, Inc.
Wechsler, D. (2003). Wechsler Intelligence Scale for Children, 4th Edn. San Antonio, TX: Harcourt Assessment, Inc.
Keywords: autism spectrum disorder, TMS, ERP, reaction time, executive functions, oddball task, aberrant and repetitive behaviors
Citation: Sokhadze EM, Lamina EV, Casanova EL, Kelly DP, Opris I, Tasman A and Casanova MF (2018) Exploratory Study of rTMS Neuromodulation Effects on Electrocortical Functional Measures of Performance in an Oddball Test and Behavioral Symptoms in Autism. Front. Syst. Neurosci. 12:20. doi: 10.3389/fnsys.2018.00020
Received: 30 December 2017; Accepted: 02 May 2018;
Published: 28 May 2018.
Edited by:
Preston E. Garraghty, Indiana University Bloomington, United StatesReviewed by:
Richard Eugene Frye, Phoenix Children’s Hospital, United StatesJose L. Pardo-Vazquez, Universidade de Santiago de Compostela, Spain
Copyright © 2018 Sokhadze, Lamina, Casanova, Kelly, Opris, Tasman and Casanova. This is an open-access article distributed under the terms of the Creative Commons Attribution License (CC BY). The use, distribution or reproduction in other forums is permitted, provided the original author(s) and the copyright owner are credited and that the original publication in this journal is cited, in accordance with accepted academic practice. No use, distribution or reproduction is permitted which does not comply with these terms.
*Correspondence: Estate M. Sokhadze, c29raGFkemVAZ3JlZW52aWxsZW1lZC5zYy5lZHU=; dGF0by5zb2toYWR6ZUBsb3Vpc3ZpbGxlLmVkdQ==