- 1Laboratory of Chemical Neuroanatomy, Department of Anatomy, Institute of Biomedical Sciences, University of São Paulo, São Paulo, Brazil
- 2Center for Neuroscience and Behavior, Institute of Psychology, University of São Paulo, São Paulo, Brazil
The melanin-concentrating hormone (MCH) is an important peptide implicated in the control of motivated behaviors. History, however, made this peptide first known for its participation in the control of skin pigmentation, from which its name derives. In addition to this peripheral role, MCH is strongly implicated in motivated behaviors, such as feeding, drinking, mating and, more recently, maternal behavior. It is suggested that MCH acts as an integrative peptide, converging sensory information and contributing to a general arousal of the organism. In this review, we will discuss the various aspects of energy homeostasis to which MCH has been associated to, focusing on the different inputs that feed the MCH peptidergic system with information regarding the homeostatic status of the organism and the exogenous sensory information that drives this system, as well as the outputs that allow MCH to act over a wide range of homeostatic and behavioral controls, highlighting the available morphological and hodological aspects that underlie these integrative actions. Besides the well-described role of MCH in feeding behavior, a prime example of hypothalamic-mediated integration, we will also examine those functions in which the participation of MCH has not yet been extensively characterized, including sexual, maternal, and defensive behaviors. We also evaluated the available data on the distribution of MCH and its function in the context of animals in their natural environment. Finally, we briefly comment on the evidence for MCH acting as a coordinator between different modalities of motivated behaviors, highlighting the most pressing open questions that are open for investigations and that could provide us with important insights about hypothalamic-dependent homeostatic integration.
The Melanin-Concentrating Hormone[S]
The existence of the MCH was postulated in the 1930s as a factor that induces pallor in the skin of amphibians and participates in these animals’ mechanism of background color adaptation (Hogben and Slome, 1935). It took almost 50 years, however, for MCH to be isolated for the first time from the chum salmon (Oncorhynchus keta) hypophysis, where it plays exactly its hypothesized role in skin pigmentation by triggering a concentration of melanin in melanophores that results in pallor (Kawauchi et al., 1983). Although the chronology of MCH discovery emphasized its role in skin pigmentation, it is now proposed that this function only emerged for the first time in the last universal common ancestor of teleosts and holoceans, millions of years after the appearance of MCH synthesis in chordates (Kawauchi and Baker, 2004).
It was later discovered that teleosts have two paralogs of MCH, termed MCH1 and MCH2 (Ono et al., 1988), that have different patterns of distribution in the CNS. MCH1 corresponds to the peptide isolated by Kawauchi et al. (1983) and is mostly associated with the control of skin pigmentation. Berman et al. (2009), working with the zebrafish (Danio rerio) hypothalamus, demonstrated that MCH1 immunoreactivity is largely impacted by background color, reflecting its role in skin pigmentation, while only MCH2 displays pronounced alterations in fasted animals. This correlation between hypothalamic MCH2 and the energy balance of the organism indicates that MCH may be linked to motivated behaviors and integrative functions at least since the divergence of tetrapods and ray-finned fishes at ∼440 million years ago (Amores et al., 2011).
Six years after the discovery of MCH1 in the salmon hypophysis, Nahon et al. (1989) and Vaughan et al. (1989) described for the first time the mammalian MCH coding gene and structure. Using rat samples, these researchers showed that there is a single mammalian MCH, with 19 amino acids and a cysteine bridge that confers it a cyclic structure, synthesized from a 165 amino acids precursor coded by the Pmch gene. This precursor also contains the sequence of two other peptides, NEI and NGE, and although some functions are now attributed to the former (for a review, see Bittencourt and Celis, 2008), the biological activity of the latter is largely unknown. Synteny, genomic structure, and sequence identity indicate that the mammalian MCH is an ortholog of teleost MCH2 (Berman et al., 2009) and shows remarkable conservation when compared to the single MCH of a cartilaginous fish, the scalloped hammerhead shark (Sphyrna lewini), with a single substitution at the C-Terminal (Mizusawa et al., 2012).
Anatomical Aspects
The synthesis of MCH shows two remarkably conserved features: most, if not all, MCH-immunoreactive (MCH-ir) neurons are found in the hypothalamus, and those neurons have widespread projections throughout the CNS. The first anatomical characterization of MCH was made in the male and female rat by Bittencourt et al. (1992). In this species, most MCH-ir neurons are found in the LHA and, to a lesser extent, in the AHA and PHA of the medial zone and in the dorsalmost area of the periventricular zone. A second group of rostral neurons is found in the IHy, previously known as rostromedial ZI (Bittencourt et al., 1992; Murray et al., 2000c), where MCH-ir neurons are intermingled with the DA neurons of the A13 group (Sita et al., 2003, 2007). Exclusively during the postpartum period, immunoreactivity to MCH can be detected in neurons of the MPOA and at the rostralmost aspect of the PVH, with an intensity that follows the progression of lactation and, with weaning, disappears (Knollema et al., 1992; Rondini et al., 2010). Outside the hypothalamus, MCH-ir neurons were described in the OT, ZI, and paramedian PnRt (Bittencourt et al., 1992) and in the LDTg of the female rat (Rondini et al., 2007). Little is known about the role played by MCH in these extra-hypothalamic areas. Figure 1 summarizes the loci of MCH synthesis in the rat CNS and the main pathways formed by MCH-ir fibers.
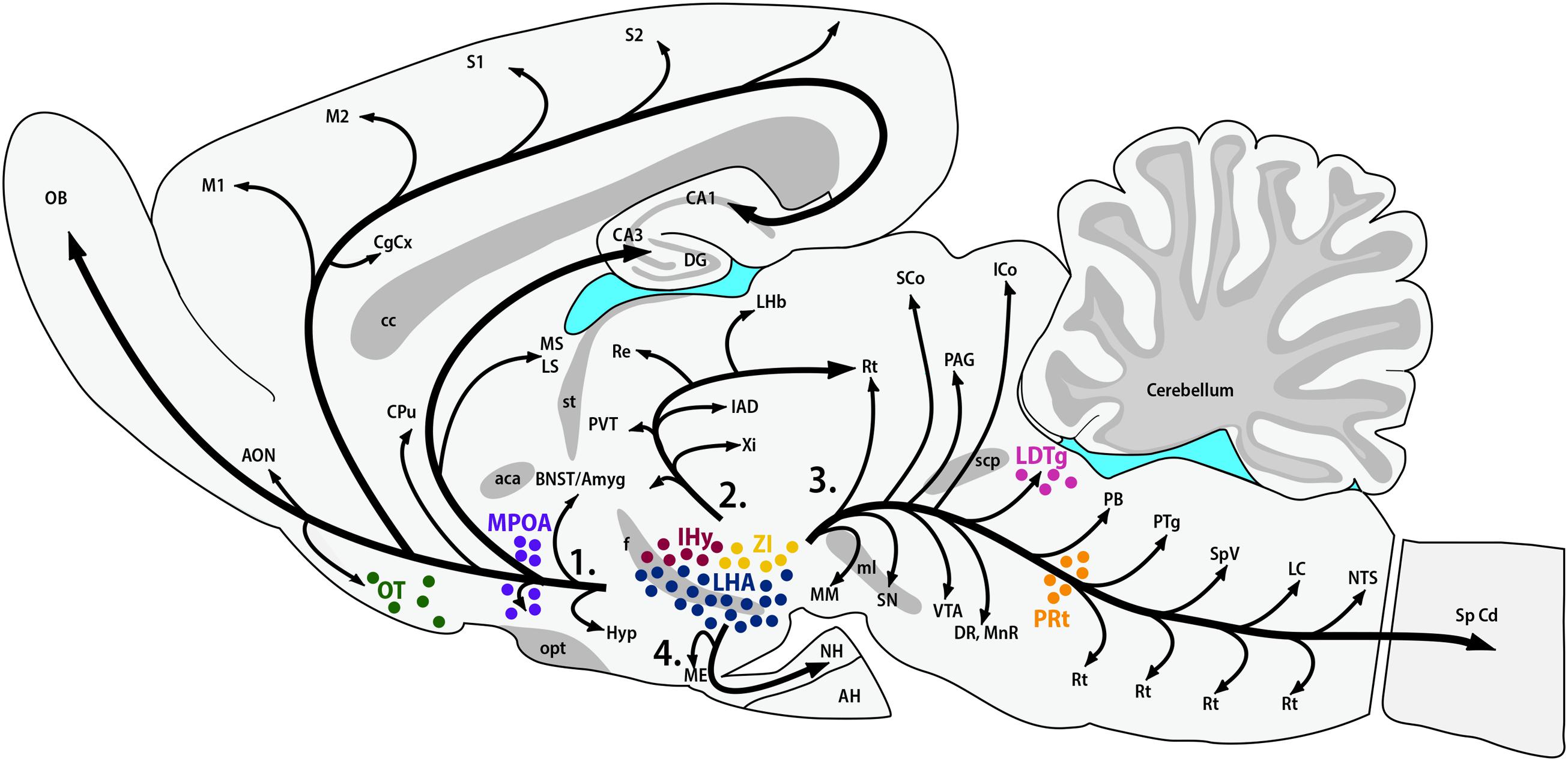
FIGURE 1. MCH synthesis is found in discrete areas of the rat nervous system. Sagittal representation of the rat central nervous system illustrating the main areas where MCH-ir neurons are found. The hypothalamic areas of synthesis in all animals are: the LHA (blue) and the IHy (red). Extra-hypothalamic areas are: the ZI (yellow), the OT (green) and the paramedian PnRt (orange). Exclusively in female rats, one extra-hypothalamic site can be found, in the laterodorsal tegmental nucleus (pink). During the lactation period, MCH-immunoreactive neurons can be detected in the medial preoptic area (purple). The four main pathways of MCH-ir fibers are also represented: 1 – ascending pathway; 2 – periventricular pathway; 3 – descending pathway; and 4 – hypophysaire pathway.
Contrasting to the highly restricted pattern of MCH synthesis, MCH-ir fibers are found widespread throughout the whole CNS. Among the regions that contain fibers are olfactory areas, the septal nuclei, the basal nuclei, the HF, the neocortex, several diencephalic nuclei, the mesencephalic and PnRt, the PAG and all levels of the SpCd. The regions with the sparsest presence MCH-ir fibers are the cerebellum and some motor nuclei of the brainstem. In most of these areas, MCH-ir fibers are varicose and present many boutons en passage and boutons terminaux, suggesting an extensive synaptic activity (Bittencourt et al., 1992). Figure 2A is a visual representation of the MCH-ir fiber density in different areas of the rat CNS.
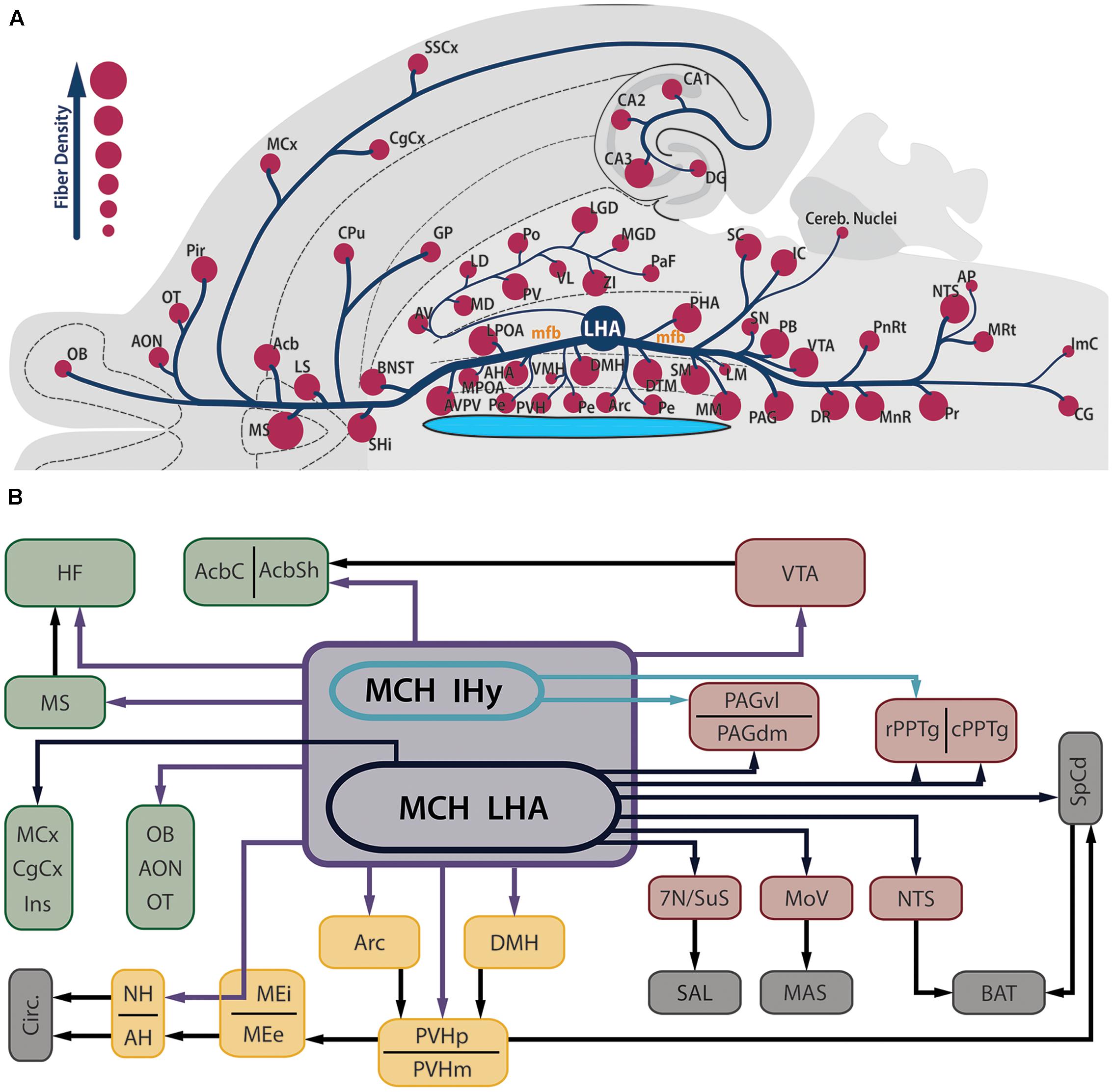
FIGURE 2. MCH-immunoreactive fibers are profusely distributed in the neuroaxis and are found in several areas related to feeding behavior and energy homeostasis. (A) Flatmap representation of the rat central nervous system illustrating the main areas where MCH-ir fibers can be found. The relative density of MCH-ir fibers in each area is indicated by the size of the circles. Observe that septal, hypothalamic and brainstem areas are densely innervated by MCH neurons, while cortical and thalamic areas display a smaller number of fibers. Due to the position of the LHA, most of the MCH-ir fibers leave this region intermingled to the mfb, with the periventricular pathway as a notable exception to this rule. (B) Schematic representation of the main targets of MCH-ir fibers in the rat CNS. Dark blue arrows represent projections originated from MCH-ir neurons located in the LHA, while green arrows indicate projections preferentially originated from the IHy. Purple arrows indicate projections originated from both areas or a lack of hodological studies to pinpoint the origin of such projections. Dark arrows indicate indirect connections relevant to this circuit. The main targets of MCH innervation can be arranged in three groups: telencephalic projections, in green; hypothalamic-hypophysaire projections, in yellow; and brainstem projections, in red. Downstream targets of MCH action are represented in gray.
The distribution of MCH-ir cells has been studied in a wide range of animals, including agnathans (Mizuno, 1991; Bird et al., 2001), cartilaginous fish (elasmobranchii) (Vallarino et al., 1989; Mizusawa et al., 2012), teleosts (Naito et al., 1985; Batten and Baker, 1988; Powell and Baker, 1988; Bird and Baker, 1989; Minth et al., 1989; Gröneveld et al., 1995; Mancera and Fernandez-Llebrez, 1995; Amano et al., 2003; Huesa et al., 2005; Matsuda et al., 2009; Suzuki and Yamamoto, 2013), anurans (Andersen et al., 1986; Francis and Baker, 1995; Lázár et al., 2002), reptiles (Cardot et al., 1994), and birds (Cardot et al., 1998, 1999). In mammals, in addition to the rat (Bittencourt et al., 1992), MCH-ir cell bodies have been mapped in the mouse (Elias et al., 2001), Djungarian hamster (Khorooshi and Klingenspor, 2005), Syrian hamster (Vidal et al., 2005), tufted capuchin monkey (Bittencourt et al., 1998), humans (Elias et al., 2001), pigs (Chometton et al., 2014), sheep (Tillet et al., 1996), and cats (Torterolo et al., 2006). For a review of the anatomical aspects of MCH in mammals, see Bittencourt (2011).
Albeit there are some differences between these animals, the main locus of MCH synthesis in all of them is the hypothalamus or homologous structures, indicating that MCH-ir neurons reside this region at least since the emergence of the last vertebrate common ancestor. There is also a remarkable conservation in the widespread distribution of MCH-ir fibers. The hypothalamus, through its extensive projections, is a key structure for the execution of motivated behaviors, as understood as behavioral programs directed to exogenous factors that are executed by animals and result in a benefit to the survival of the organism or of its species (Swanson, 2005). This long phylogenetic history accounts, at least partially, for the numerous functions on which MCH has been suggested to play a role in, and may explain how MCH acquired an important integrative role in motivated behaviors centered on its hypothalamic localization supported by a widespread fiber distribution.
Feeding Behavior
The most thoroughly described function of MCH is on the modulation of feeding behavior. The injection of MCH in the ventricle of rats (Qu et al., 1996; Rossi et al., 1997; Della-Zuana et al., 2002; Clegg et al., 2003; Duncan et al., 2005; Sakamaki et al., 2005) and mice (Gomori et al., 2003; Glick et al., 2009), as well as in the PVH (Rossi et al., 1997) or in the Acb of rats (Georgescu et al., 2005; Mul et al., 2011), provokes an increase in chow consumption followed by a gain of body weight and fat mass. Rossi et al. (1997), however, demonstrated that only acute injections of MCH can induce these effects, while chronic administration is unable to maintain it. Injections of MCH in the 3V also induce an increase in water and saccharin consumption in rats, independently of chow availability, indicating that MCH participates in hydric homeostasis processes (Clegg et al., 2003; Sakamaki et al., 2005; Furudono et al., 2006). This same treatment, however, increases alcohol consumption, suggesting that MCH participates in hedonistic aspects of this behavior as well (Duncan et al., 2005). Mice deficient in leptin synthesis [Lepob/ob] with an obese phenotype display a higher expression of Pmch mRNA in the LHA when compared to WT mice, and this expression increases following fasting (Qu et al., 1996). MCH-overexpressing mice display higher chow consumption and increased body weight, as well as circulating levels of leptin and insulin resistance (Ludwig et al., 2001).
Data obtained from genetic models started broadening the roles associated to MCH. Shimada et al. (1998) report that Pmch-/- mice are leaner than Pmch+ cohorts, but several evidence point to this leaner phenotype as the result of multiple physiological alterations. Not only Pmch-/- animals eat less, but they displayed increased oxygen consumption rates; their ability to overeat in response to starvation is not affected, although Pmch-/- animals have greater weight loss and higher mortality rate; and restricted food availability resulted in similar decreases in food intake between Pmch-/- and Pmch+ mice, but deficient animals displayed increased weight loss. Another important evidence came with the work of Marsh et al. (2002), that reports that MCHR1-deficient mice have increased locomotor activity and energy expenditure that underlie an apparently contradictory hyperphagia observed in these animals. Finally, Lepob/ob Pmch-/- mice are leaner than Lepob/obPmch+, but their food consumption is not decreased (Segal-Lieberman et al., 2003). This leaner phenotype is attributed to factors linked to energy expenditure, with higher motor activity and basal temperature in response to cold. Taken together, these results indicate that MCH acts on energy homeostasis through multiple pathways, including energy expenditure, locomotor activity and reward, while increases in ingestive behavior promoted by MCH are secondary in nature. In this section, we will review the multiple aspects of MCH action over nutritional homeostasis.
Although variations in dietary parameters are capable of modulating MCH neurons, with fasting increasing Pmch expression, variations in this expression may also underlie the preference of some animals for a specific diet. For example, Morganstern et al. (2010b) identified a subset of Sprague-Dawley rats that are prone to overconsume a high-fat diet (HFD) when compared to animals that show a baseline consumption of HFD. These authors demonstrated that animals that ingest a higher amount of HFD during a 5-day period (as measured by caloric intake) have increased levels of Pmch expression in the LHA when compared to animals that were kept in the same diet but ingested a lower number of calories. This difference persists even after the animals have been maintained in regular chow for weeks, suggesting that this intrinsic variation in Pmch levels may generate the preference of these animals for the higher fat content or increased palatability of the diet. This is extremely relevant because a similar mechanism may underlie human pathologies related to the excessive consumption of fat-rich foods. To explore alterations in the MCH peptidergic system in other models of natural or induced preference may generate important knowledge on the human physiology of ingestive behaviors (Barson et al., 2012).
Inputs
Melanin-concentrating hormone neurons are influenced by many inputs, including other neurons in the LHA, adjoining hypothalamic nuclei, telencephalic and brainstem areas, in addition to circulating factors that act as metabolic cues. Figure 3 summarizes the main homeostatic-relevant inputs to MCH neurons.
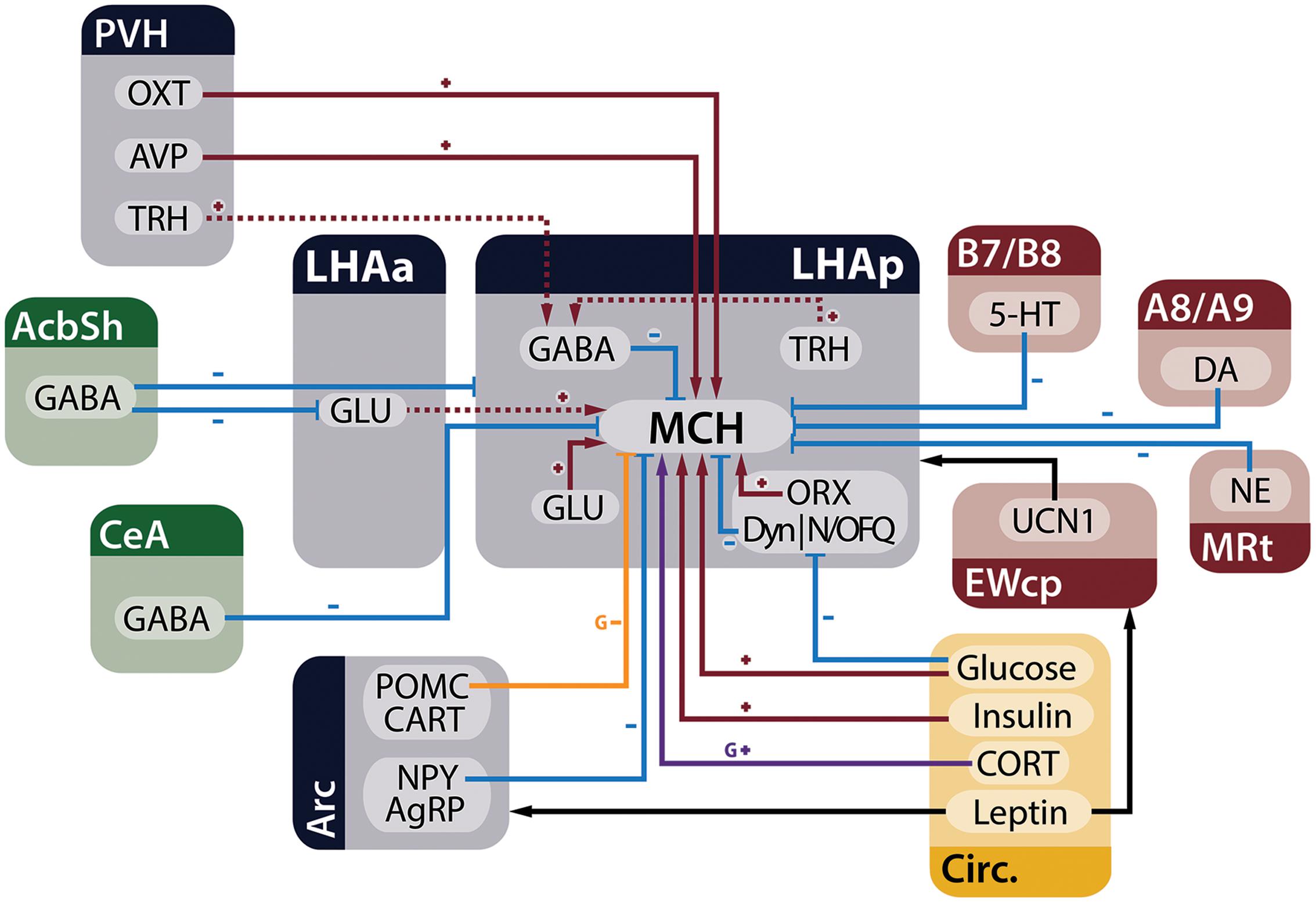
FIGURE 3. MCH neurons receive several inputs relevant to the control of energy homeostasis. Neurons from the telencephalon (green), hypothalamus (blue), and brainstem (red), in addition to circulating factors (yellow), are capable of modulating electrophysiological and genetic expression properties of MCH neurons in the LHA. Red arrows with plus signs represent depolarizing inputs, blue arrows with minus signs represent hyperpolarizing inputs, black arrows indicate relevant indirect connections to the circuit, the purple arrow represents increased expression of the Pmch gene and the orange arrow an input that decreases gene expression. Dashed lines indicate connections that have ample physiological support but lack morphological demonstration or may be composed of multiple polysynaptic elements. Some presynaptic modulatory inputs to GABA- and GLUergic neurons were omitted for clarity.
Lateral Hypothalamic Area
The LHA is classically characterized by two populations of neurons, one that is MCH-ir (Bittencourt et al., 1992) and one that is ORX-ir (Peyron et al., 1998), both intermingled with GABAergic and GLUergic interneurons (Gao and van den Pol, 2001) who provide extensive input to MCH neurons through ionic and metabotropic channels (Gao and van den Pol, 2001; Huang and van den Pol, 2007). These two populations coexist in the LHA but are almost completely segregated, with a minimal number of neurons that co-synthesize these two peptides (Broberger et al., 1998; Elias et al., 1998; Peyron et al., 1998). There are two orexin peptides, ORXA and ORXB, coded by the same gene (Hcrt) and involved in multiple functions related to homeostatic control and arousal (for recent reviews, see Mahler et al., 2014 and Sakurai, 2014). Both MCH and ORX neurons have profuse immunoreactive fibers in the LHA, contacting numerous neighboring cells (Bittencourt et al., 1992; Peyron et al., 1998). Although the high density of MCH-ir fibers close to MCH-ir cells could point to a recurrent effect of MCH on MCH cells, probably inhibitory due the nature of MCH neurons (Gao and van den Pol, 2001), there are no alterations in the electrophysiological properties of MCH neurons after MCH application in LHA slices (van den Pol et al., 2004).
MCH-ir neurons are densely contacted by ORX neurons, with some single MCH-ir cells receiving contacts by up to 30 individual ORX-ir boutons. Both ORXA and ORXB have a postsynaptic excitatory effect over MCH cells, depolarizing their membrane potential and increasing spike frequency (Figure 4A). ORXB has also a presynaptic effect, increasing GLUergic input to MCH cells (van den Pol et al., 2004) (Figure 5). The similar response amplitude of MCH neurons to ORXA and ORXB suggests that this signaling occurs through ORXR2, as ORXR1 responds poorly to ORXB (Sakurai et al., 1998). Apergis-Schoute et al. (2015), however, provided evidence that the relationship between ORX and MCH may be more complex, since optogenetic stimulation of ORX neurons in LHA slices promoted inhibition of some MCH neurons through a presynaptic mechanism, increasing GABAergic input on MCH cells, while other MCH neurons were excited by ORX stimulus. Regardless, all these results suggest that these two LHA populations are connected, with ORX influencing close MCH neurons in multiple ways.
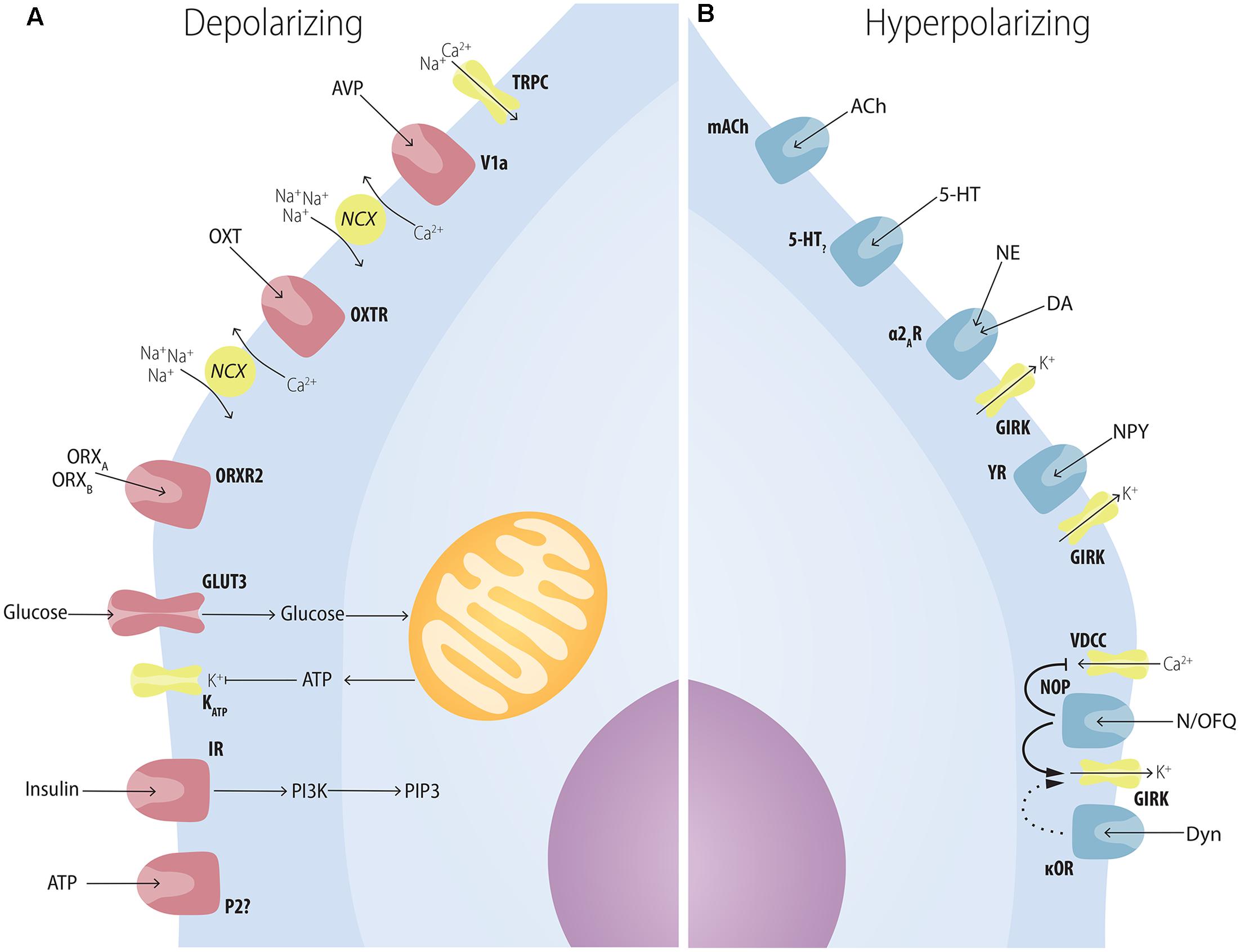
FIGURE 4. Multiple factors have postsynaptic effects on MCH neurons. (A) Several neuropeptides increase the excitability of MCH neurons through postsynaptic mechanisms. AVP depolarizes MCH neurons through NCXs and TRPC; OXT increases Na+ currents through NCXs; ATP in the extracellular medium increases MCH excitability through unknown mechanisms that may depend on P2?; orexins depolarize MCH neurons with special affinity for ORXB, suggesting an ORXR2-dependent pathway; glucose increases neuronal excitability in a mechanism similar to pancreatic β-cells: GLUT3 transporters increase the amount of glucose in the intracellular medium, boosting the amount of produced ATP, what blocks ATP-sensitive potassium channels (KATP); insulin excites MCH neurons and activates PIP3-dependent pathways. (B) Several neuropeptides decrease the excitability of MCH neurons through postsynaptic mechanisms. ACh and 5-HT, two important elements of the central arousal system, inhibit MCH neurons; both NE and DA are capable of decreasing MCH excitability through the activation of the adrenoceptor type 2A and resulting activation of K+ currents through GIRK channels; NPY hyperpolarizes MCH neurons also through GIRK currents; opioids such as N/OFQ and Dyn hyperpolarize MCH neurons with convergent mechanisms through the blockage of VDCC and an increase in GIRK currents.
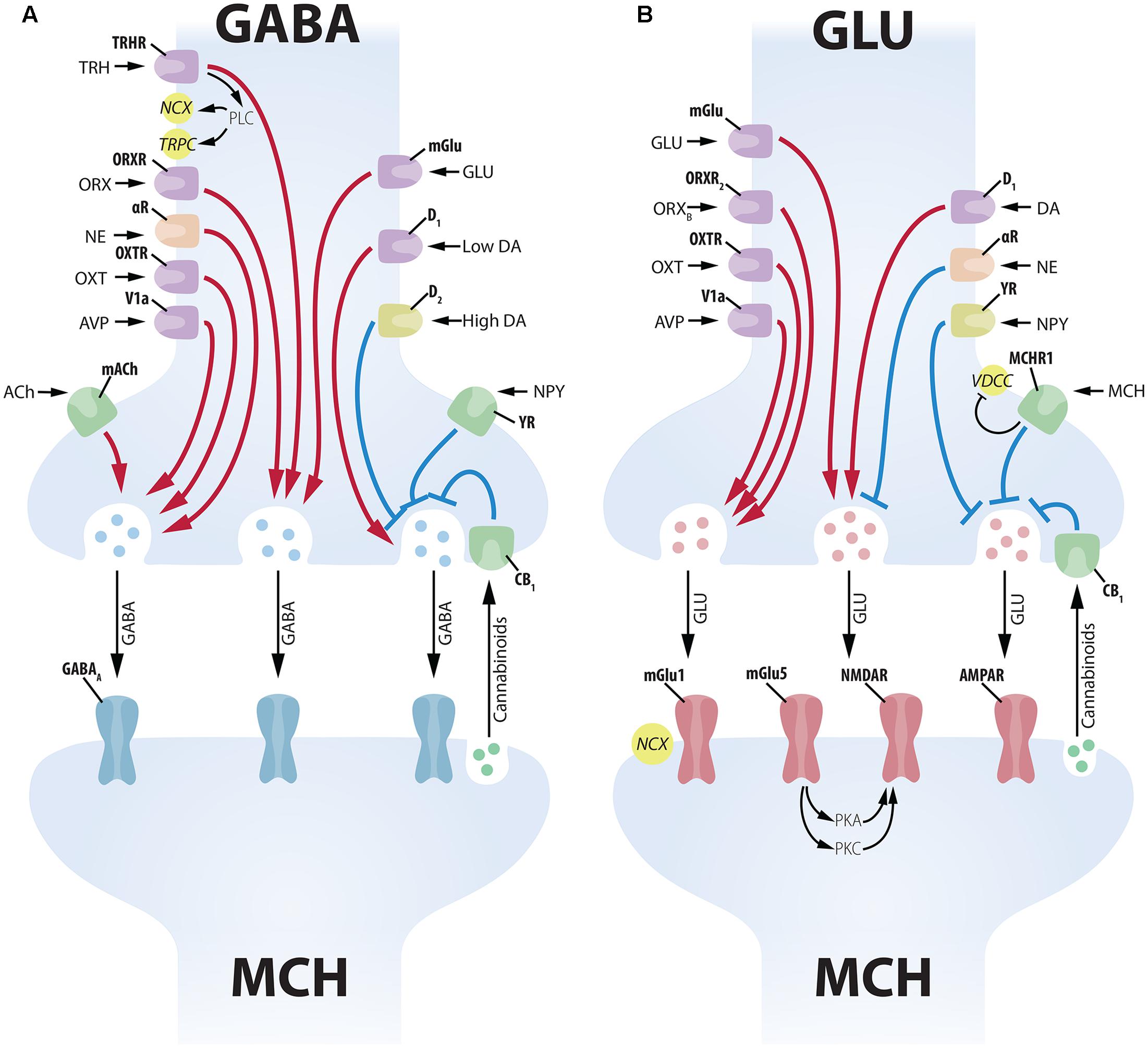
FIGURE 5. Complex presynaptic modulation of MCH neurons. (A) Several neuropeptides increase GABAergic input to MCH neurons acting on the soma of GABA neurons, such as: TRH, ORX, NE, OXT, AVP, GLU and low levels of DA. ACh increases GABAergic input to MCH neurons acting on axon terminals. High levels of DA, on the other hand, promote an inhibition of presynaptic GABAergic input through D2 receptors. NPY decreases inhibitory input acting on presynaptic terminals, while cannabinoids released by the MCH postsynaptic terminal decrease vesicle release (Huang et al., 2007). GABAergic input to MCH neurons is vehiculated mainly by GABAA receptors. (B) The GLUergic input to MCH neurons is similarly under the control of numerous inputs. GLU, ORX, OXT, AVP, and DA act on the soma of GLU cells to increase excitatory input to MCH neurons. NE and NPY have the opposite effect, decreasing GLU input to MCH neurons. MCH has an inhibitory presynaptic effect through an MCHR1-dependent decrease in Ca2+ currents. Cannabinoid activation of CB1 has a similar inhibitory effect on vesicle release (Huang et al., 2007). GLU input to MCH neurons is conveyed by multiple receptors, including ionic (NMDAR and AMPAR) and mGlu1 and mGlu5. The activation of mGlu5 in MCH neurons leads to a potentiation of NMDAR responses through protein kinases A and C (PKA and PKC).
While some MCH neurons co-synthesize the CART (Elias et al., 2001) and nesfatin (Brailoiu et al., 2007; Fort et al., 2008), almost all ORX neurons co-synthesize Dyn or N/OFQ, both endogenous opioids (Maolood and Meister, 2010). The application of Dyn or N/OFQ hyperpolarizes MCH cells, increasing their input resistance (Li and van den Pol, 2006; Parsons and Hirasawa, 2011), probably through κ-opioid (κOR) and NOP receptors present in these cells (Parks et al., 2014) (Figure 4B). These results suggest that MCH neurons could potentially be stimulated by ORX and inhibited by Dyn and N/OFQ originated from the same fibers. Li and van den Pol (2006) and Parsons and Hirasawa (2011) report that MCH cells quickly desensitize to Dyn and N/OFQ, while the response to ORX remains largely unaltered after sequential applications. Through this mechanism, Dyn and N/OFQ could damp the MCH response to brief spontaneous activation of ORX cells, since only constant ORX input would surpass the desensitized inhibitory effect of Dyn and N/OFQ and activate MCH cells. In other words, these endogenous opioids provide the ORX-MCH circuit with a mechanism for noise filtering.
In addition to the chiefly LHA-centered MCH and ORX populations, neurons with distinct chemical signatures and broader distributions are also found in the LHA. One example is the TRH-ir neuronal population located in the LHA, that does not co-localize with MCH or ORX (Horjales-Araujo et al., 2014) and can also be identified in the PVH, VMH, DMH, suprachiasmatic, Pe and Arc hypothalamic nuclei (Brownstein et al., 1974). Besides its role in thyroid function regulation through the AH, TRH is also associated with the control of energy homeostasis through central pathways: icv injections decrease feeding and increase thermogenesis and locomotor activity (for a review on TRH functions, see Lechan and Fekete, 2006). The central effects of TRH are all antagonistic to MCH actions, suggesting that TRH-mediated inhibition of MCH neurons could account, at least partially, for the observed results. Indeed, Zhang and van den Pol (2012) report that TRH inhibit MCH neurons by increasing presynaptic GABAergic input to MCH neurons (Figure 5). In conclusion, TRH may modulate feeding behavior and energy expenditure by inhibiting MCH neurons in the LHA, and the source of this TRH input may be from the LHA itself or from other hypothalamic nuclei linked to energy balance, as the PVH, VMH, DMH, and Arc.
Recently, another population of LHA neurons has emerged an important mediator of energy homeostasis: Nts neurons that also contain LepR, known as Nts-LepR. These neurons are found in the LHA (Leinninger et al., 2011) and make synaptic contacts with local neurons (Louis et al., 2010; Leinninger et al., 2011). Although Leinninger et al. (2011) have shown that Nts-LepR neurons make inhibitory synaptic contacts with ORX neurons, but not MCH neurons, it is possible that a polysynaptic circuit may link Nts-LepR neurons to MCH. The selective ablation of LHA Nts-LepR neurons leads to a phenotype of increased body weight, but these mice have only a slightly increased food consumption when young. On the other hand, these animals have increased adiposity and decreased VO2 and ambulatory activity (Leinninger et al., 2011). This phenotype inversely mirrors that observed in Pmch-/- and Mchr1-/- mice, that show a reduction in chow consumption that is too small to explain their weight deficit, increased O2 consumption and increased ambulatory activity (Shimada et al., 1998; Marsh et al., 2002). These energy expenditure alterations seen in Nts-LepR mice are more compatible with overactivity of MCH neurons than exclusively of ORX. As Nts-LepR neurons are predominantly GABAergic (Leinninger et al., 2009; Goforth et al., 2014), synaptic spilling or GABA-mediated decrease of GLUergic input to MCH neurons may explain how these neurons modulate MCH neurons. As leptin serves as a metabolic signal for high energy reserves, the inhibition of MCH neurons by Nts-LepR neurons may be a mechanism to decrease MCH-mediated energy conservation in states of positive energy balance.
Arcuate Nucleus
The Arc is a classical component of the feeding behavior circuitry, as lesions in this nucleus and the adjoining VMH by gold thioglucose promote hyperphagia and obesity in mice (Debons et al., 1962). This nucleus contains neuronal populations that produce anorectic peptides, such as the POMC-derived α-MSH (Jacobowitz and O’Donohue, 1978; Elias et al., 1998; Ludwig et al., 1998) and CART (Koylu et al., 1997, 1998; Kristensen et al., 1998), as well as orexigenic peptides as the NPY (Allen et al., 1983; Clark et al., 1984) and the AgRP (Ollmann et al., 1997; Shutter et al., 1997). This nucleus is also influenced by insulin (van Houten et al., 1980; Niswender et al., 2003), glucose (Leloup et al., 1994; Muroya et al., 1999; Parton et al., 2007), and leptin (Mercer et al., 1996; Schwartz et al., 1996; Cheung et al., 1997), all of them capable of conveying the short- and long-term energetic state of the organism from the CSF to the Arc through the ME (Woods et al., 1979; Banks et al., 1996), positioning the Arc as the ideal receptor for these circulating metabolic markers.
Elias et al. (1998) showed that both major populations of the Arc, NPY/AgRP and POMC/CART, project densely to the LHA, with immunoreactive fibers in close apposition to MCH neurons. van den Pol et al. (2004) report that NPY inhibits MCH neurons, decreasing spike frequency and hyperpolarizing membrane potential, both postsynaptically through GIRK currents and presynaptically inhibiting GLU and GABA release to MCH neurons (Figures 4B, 5). To better understand these observations, it is important to consider that the LHA and the Arc present reciprocal connections. Direct injections of MCH in the Arc promote an increase in food consumption, placing this area as a site of MCH action (Abbott et al., 2003). Using hypothalamic cultures, these authors demonstrated that the addition of MCH to the culture induces a release of NPY, at the same time decreasing the amount of the anorectic α-MSH released by a hypothalamic explant. These data suggest that MCH projections from the LHA to the Arc may increase the release of the orexigenic peptide NPY and decrease the anorectic α-MSH, resulting in an overall increase in food consumption.
The results of Abbott et al. (2003) allow us to speculate that NPY inhibition of MCH neurons may be part of a negative feedback loop, with increased MCH activity on the Arc resulting in a depression of MCH neurons by NPY, avoiding excessive stimulation of this orexigenic circuit. Another possibility is that NPY–MCH interactions may be secondary to some other Arc-LHA circuits, as suggested by Segal-Lieberman et al. (2003) and van den Pol et al. (2004). Parallel to this negative feedback loop, a positive feedback loop may exist between MCH and the anorectic peptides of the Arc. Although the addition of α-MSH agonists or antagonists has no major electrophysiological effect on MCH neurons (van den Pol et al., 2004), the selective deletion of the Pomc gene results in a marked increase in Pmch mRNA, suggesting that POMC-derived peptides are capable of inhibiting Pmch expression (Challis et al., 2004). In this case, inhibition of α-MSH neurons by MCH would result in decreased inhibition of Pmch expression, potentially increasing the stimulation of NPY by MCH and reinforcing the negative feedback loop. It is important to consider, however, that these circuits may be polysynaptic, since there is little evidence for NPY and melanocortin receptors in MCH neurons (Liu et al., 2003; Parks et al., 2014). Therefore, these circuits may employ interneurons or even ORX neurons, since these are susceptible to multiple regulatory mechanisms by NPY (Fu et al., 2004). Finally, another possibility is that Arc projections interact with MCH-ir fibers at the PVH, a target nucleus that display important effector actions regarding feeding behavior and energy homeostasis.
Paraventricular Hypothalamic Nucleus
The PVH is one of the better-described nuclei in the whole hypothalamus. It has eight major subdivisions, with three magnocellular subdivisions containing neurons that primarily synthesize OXT and AVP, and five parvocellular subdivisions containing neurons that synthesize CRF and TRH, although we now know that many other neurochemical populations are present in the PVH with distinct distributions (Swanson and Sawchenko, 1983; Simmons and Swanson, 2008, 2009). In many ways, the PVH can be regarded as the motor nucleus of the endocrine system, directly releasing OXT and AVP in the NH and CRF and TRH in the portal circulation to modulate AH activity and influence two of the major neuroendocrine axis in the brain, the HPT and the HPA axis. Projections from these neurons, however, are not restricted to the hypophysis, as they project densely to other areas of the brain and all of them show, to some extent, modulatory effects.
To investigate if OXT and AVP neurons could exert effects through MCH neurons, Yao et al. (2012) evaluated the electrophysiological response of MCH cells to these neuromodulators. AVP directly excites MCH neurons through the V1a receptor, depolarizing their membrane potential and increasing spike frequency through NCX and the opening of TRPC (Figure 4A). AVP also acts presynaptically increasing GLU- and GABAergic input to MCH neurons (Figure 5). OXT provides excitatory input to MCH neurons as well, through the OXTR, depolarizing their membrane potential and increasing spike frequency through NCX (Yao et al., 2012) (Figure 4A). In addition to OXT and AVP, neuropeptides from the PVHp may also contribute to MCH-dependent modulation of ingestive behavior. As discussed in the previous section, TRH modulates MCH function and it is not possible, for now, to specify the exact source of this input to presynaptic GABA neurons in the LHA. Moreover, little has been described about the effects of CRF-synthesizing neurons over MCH cells albeit that, if such mechanism exists, it must involve polysynaptic circuits, since Parks et al. (2014) report that CRF receptors are not significantly found in MCH neurons.
Limbic and Mesolimbic
The Acb is an important component of the ventral striatum, participating in several processes, including reward and aversion (for a review, see Carlezon and Thomas, 2009). The Acb can be divided into two subdivisions, a core (AcbC) and a shell (AcbSh), with distinct connectivity, cellular composition and function. AcbSh activity has been associated with feeding inhibition, as electrical and optogenetic stimulation of AcbSh MSNs are capable of quickly interrupting a feeding bout; a subset of AcbSh neurons decrease their activity immediately before the onset of a sucrose solution consumption; and photoinhibition of dopamine-sensitive (D1) MSNs increases liquid fat intake (Krause et al., 2010; O’Connor et al., 2015).
AcbSh MSNs are GABA cells that project to the rostral parts of the LHA, suggesting the inhibition of orexinergic neurons of this area (MCH and ORX) as a putative mechanism for AcbSh-mediated inhibition of feeding. It is now understood, however, that AcbSh input to the LHA does not reach MCH or ORX neurons directly. Sano and Yokoi (2007) identified a group of GLU neurons in the anterior part of the LHA (LHAa) that receive dense projections from the AcbSh and project to the posterior part of the LHA (LHAp), suggesting that a GLU node exists between accumbal projections and neurons in the LHAp, where MCH neurons are located. O’Connor et al. (2015) identified a second pathway, reporting that D1-MSNs from the AcbSh project to the LHA and inhibit GABA interneurons in this area to promote accumbal inhibition of feeding. As an AcbSh|GABA-LHA|GABA-LHA|MCH circuit would be ultimately excitatory for MCH neurons, it is likely that additional nodes are present in this pathway. Further experiments will be necessary to elucidate the intra-LHA circuit that conveys accumbal information to MCH and ORX neurons.
The inhibition of MCH neurons by accumbal projections agrees well with the theory proposed by Kelley et al. (2005) that the AcbSh functions as a “sensory sentinel,” quickly inhibiting a feeding bout in response to an environment cue that may need the animal to respond to, such as a predator approaching, even if the animal’s metabolic state would drive him to continue feeding. Since MCH neurons project to the MCx (Elias et al., 2008) and decrease locomotor activity, an inhibition of these neurons could, at the same time, decrease the drive to feed and disinhibit the MCx, prompting the animal to show a motor response to the environmental cue.
Amygdaloid input to MCH neurons may also pay a role in the inputs to this system. Nakamura et al. (2009) report that anterograde tracer injections centered in the CeA reveal numerous varicose fibers in the LHA, chiefly on its dorsolateral most area. These fibers often make contact to MCH-ir and ORX-ir neurons and these terminals are immunoreactive for a GABA marker, suggesting an inhibitory property for these CeA projections.
Midbrain and Hindbrain
The midbrain contains some of the most important nuclei containing DA-synthesizing neurons in the whole CNS, including the A8, SN – A9, and VTA – A10 groups (Dahlström and Fuxe, 1963). These groups project densely to the forebrain, including the neocortex, the striatum and the diencephalon (Andén et al., 1966). Leibowitz and Brown (1980) showed that ventral A8 and A9 cells provide an important CA input to the perifornical area, and lesions of the A8 and A9 deplete the LHA of CA-ir, reduce the anorectic effect of the DA presynaptic drug amphetamine and potentiate the anorectic effect of DA itself. Alberto et al. (2011) and Conductier et al. (2011) report that DA produces hyperpolarization of MCH neurons through GIRK mediated outward currents, but DA receptors (D1 and D2) antagonists failed to induce similar currents, suggesting that the observed DA actions are not mediated by DA receptors (Figure 4B). Conductier et al. (2011) also found that DA can alter the presynaptic input to MCH neurons, with low concentrations of DA (1 μM) potentiating both GABA and GLU release through presynaptic D1 receptors, while high concentrations of DA (100 μM) inhibits GABA release through D2 receptors, with GABA effects more pronounced than GLU effects suggesting a predominantly inhibitory action for DA over MCH neurons (Figure 5). Both Alberto et al. (2011) and Conductier et al. (2011) found as well that the postsynaptic effect of DA depends on the adrenergic receptor α2A, since specific antagonists blocks DA-induced currents and α2A is present in MCH neurons (Modirrousta et al., 2005).
These results are particularly noteworthy because the α2A is also the receptor through which NE inhibits MCH action. The LHA is moderately innervated by NE terminals originated from the brainstem (Palkovits et al., 1974), with an important contribution from the lateral tegmental NE system (Moore and Bloom, 1979). The work of van den Pol et al. (2004) has provided evidence that NE promotes a hyperpolarization of the membrane potential and a decrease in spike frequency of active MCH neurons by mobilizing GIRK currents, and this effect is blocked by a selective α2A antagonist (Figure 4B). NE also acts on the synaptic input to MCH neurons, decreasing GLUergic and increasing GABAergic input, resulting in an overall inhibitory action (Figure 5). Taken together, the results of van den Pol et al. (2004), Alberto et al. (2011), and Conductier et al. (2011) point to a synergistic convergence of both DA and NE systems through the α2A. This convergence may limit how much synergistic inhibition acts over MCH neurons, since DA inhibition would desensitize the receptors for NE inhibition and vice-versa. The DA and NE systems are, therefore, competitive elements instead of synergistic, even though both have inhibitory properties over MCH neurons.
Serotonin is a monoamine neurotransmitter synthesized in numerous groups of neurons of the brainstem and with widespread projections throughout the CNS (Dahlström and Fuxe, 1963; Steinbusch, 1981). Hypothalamic injections of 5-HT have been extensively correlated to a decrease in feeding (for a review, see Leibowitz and Alexander, 1998) through multiple receptors and different mechanisms (for a review, see De Vry and Schreiber, 2000). van den Pol et al. (2004) report that 5-HT hyperpolarizes MCH neurons and decreases spike frequency through postsynaptic mechanisms (Figure 4B). Taken into consideration, these data indicate that 5-HT may decrease feeding through the inhibition of MCH neurons in addition to 5-HT interactions with DA, although further experimental work is necessary to confirm this hypothesis and detect the specific 5-HT receptor subtypes responsible for this action.
The urocortins (UCN1, UCN2, and UCN3) are members of the mammalian CRF family, with a special affinity for the CRF receptor subtype 2 (Vaughan et al., 1995; Lewis et al., 2001; Reyes et al., 2001). UCN1 has been mapped in the albino rat by Kozicz et al. (1998) and Bittencourt et al. (1999), who have shown that the main sites of Ucn1 mRNA expression and UCN1 synthesis are the EW and the superior olivary nucleus, both brainstem structures, with a broad distribution of UCN1-ir fibers throughout the CNS. Although the EW was initially recognized as a motor nucleus for vision related muscles, it is now recognized that the EW has two components: a preganglionic EW, responsible for this cholinergic motor control, and the centrally-projecting EW, involved in a series of functions such as feeding, stress and addiction (for a review in EW structure and function, we point the reader to Kozicz et al., 2011). Of special importance to us is the role of UCN1 in feeding behavior, as UCN1 acts as a powerful anorectic peptide in nanomolar concentrations (Spina et al., 1996) and diet/fasting can regulate Ucn1 mRNA expression (Legendre et al., 2007; Xu et al., 2009). Júnior et al. (2015) have shown that UCN1-ir neurons or the EWcp send projections to the LHA and these fibers are in close proximity to MCH-ir neurons, so this UCN1|EWcp-MCH|LHA circuit could represent the pathway through which UCN1 influences feeding behavior. Although the low levels of CRF receptors in MCH could be taken as an evidence against this particular pathway, it is noteworthy that these receptors are found on the LHA (Chalmers et al., 1995), so the action of UCN1 could involve the modulation of GABAergic or GLUergic interneuronal input to MCH neurons. Further studies will be necessary to elucidate the physiological aspects of this circuit.
Circulating Factors
The MCH peptidergic system appears to be heavily influenced by leptin, a major circulating factor of satiety produced by adipocytes (Zhang et al., 1994; Campfield et al., 1995; Halaas et al., 1995; Pelleymounter et al., 1995). Qu et al. (1996) were the first to identify a relationship between MCH and leptin, reporting that Lepob/ob mice have twice the level of Pmch mRNA than WT animals, suggesting a leptin inhibition of Pmch expression. Agreeing to that, obese rats with a point mutation in the long form of the LepR gene (Zucker rats LepRfa/fa) display higher levels of Pmch mRNA and decreased levels of Mchr1 mRNA in comparison to lean rats, suggesting that deficient leptin signaling can upregulate the MCH system (Stricker-Krongrad et al., 2001). Likewise, Gavrila et al. (2005), studying humans, report that fasting (with a concomitant fall of circulating leptin levels) significantly increases serum levels of MCH, and supplementation with r-metHuLep prevents the raise of seric MCH. The mechanism through which MCH responds to leptin, however, must be polysynaptic, as studies using a reporter associated to the Lepr gene indicate that Lepr-expressing neurons are not MCH or ORX (Leinninger et al., 2009; Louis et al., 2010).
Other important factors for metabolism regulation are glucose and insulin. Although the periphery holds the machinery for glucose-mediated insulin production, and this machinery works efficiently to keep the blood levels of glucose in a strict range, we now include the CNS as an important sensor for these metabolic cues and as an effector to keep their physiological levels (Myers and Olson, 2012). The IR is widely distributed in the CNS (Mayer et al., 1978), with deleterious effects on energy homeostasis occurring after its specific deletion (Brüning et al., 2000). Hausen et al. (2016) provide evidence that MCH neurons can respond to insulin, reporting that 200 nM of it is capable of activating PIP3-dependent pathways and invoking action potentials in 6 of 13 neurons tested, while 3 neurons displayed a decrease in firing and the remaining neurons showed no alteration, suggesting that subpopulations of MCH neurons are responsive to insulin with different dynamics (Figure 4A).
In addition to insulin, Burdakov et al. (2005) provided evidence that glucose is also able to modulate MCH neurons. Although not as high as plasma levels, the brain is subjected to variations in glucose concentration that mirror those of the plasma, with a physiological range that goes from ∼0.7–2.5 mM and may reach as low as 0.2 mM in conditions of hypoglycemia and 5 mM in hyperglycemia (Routh, 2002). A subset of approximately 70% of MCH-ir neurons is excited by high levels of glucose (5 mM) and dose-dependently hyperpolarizes as glucose levels reach 0.2 mM (Burdakov et al., 2005; Kong et al., 2010). Kong et al. (2010) demonstrated that glucose sensing by MCH neurons works in a similar fashion to pancreatic β-cells, in which an increase in intracellular glucose (driven by an increase in extracellular glucose carried by the GLUT3 transporter) results in increased ATP production which, in turn, induces the closure of KATP with consequent depolarization, and this effect is dependent of SUR1-containing KATP, establishing the SUR1 protein as a marker for glucose-sensitive MCH neurons (Figure 4A).
Although the activation of MCH in conditions of high glucose may seem counterintuitive, it is possible to fit it in a larger role for MCH in energy homeostasis. MCH is suggested to be involved in energy conservation, decreasing locomotor activity and metabolic parameters and increasing sleep in conditions of positive energy balance, thus its activation in response to high glucose (Burdakov et al., 2013). This led us to hypothesize that the orexigenic activity of MCH is not incompatible with that suggestion, when we consider the probable environment of an animal regarding food availability. It would be dangerous for animals to wait for their energy levels get low, as this could impair their capacity to obtain food, so it is beneficial for animals to eat periodically, even when their energy balance is positive. In most situations, animals have some awareness of their surroundings, and sources of food probably have been scouted, with animals preferentially occupying areas with abundant food availability. In that case, animals do not have to explore for food, so they may display decreased locomotor activity and longer periods of rest. Still, they must have some drive to feed periodically, and MCH could account for this effect, integrating not only glucose levels but also the other input discussed in this section. Food depletion of their environment or hazards (such as predators) may impair their ability to keep this constant state, in a situation that would lead to decreased levels of glucose. In this case, MCH would need to be shut off (low-glucose induced hyperpolarization) and a system that promotes food-seeking could be activated.
A possible component of this food circuit is ORX, as ORX-ir cells are excited by low levels of glucose (Burdakov et al., 2005) and their activation is also implicated in arousal (Alexandre et al., 2013), a necessary condition for active food-seeking. In this model, these two peptides could act in different aspects of feeding behavior: MCH is responsible for keeping a positive energy-balance state, while the ORX system “kicks in” when the animal reaches a negative energy balance and must return to a safer range. Since MCH is a “baseline stabilizer,” avoiding big fluctuations in energy homeostasis, MCH inactivation would not promote big alterations in feeding, since other ingestive-controlling systems would act nevertheless when the animal entered deficient energy states, possibly consuming an amount of food similar to that of an MCH-preserved animal. This appears to be the case, since the selective ablation of MCH-neurons in adult mice by diphtheria toxin promotes leanness and hyperactivity, but the animals display only a non-significant tendency to eat less than control mice (Whiddon and Palmiter, 2013).
Another possible peripheral signal that may influence MCH neurons is CORT, a glucocorticoid produced by the adrenal gland that freely crosses the BBB to influence the regulation of energy balance, with central and systemic administration of glucocorticoids promoting an increase in food intake and body weight (Freedman et al., 1986; Green et al., 1992; Tataranni et al., 1996; Zakrzewska et al., 1999). Drazen et al. (2004) have shown that, in ADX animals, the icv injection of MCH has a less potent effect than in sham animals regarding both food and fluid intake, and this effect is reversed in ADX animals that receive CORT supplementation in their water. These authors also report that hypothalamic expression of Pmch mRNA is decreased in ADX animals. This is in good agreement with the results of Viale et al. (1997) that showed the partial sequence of a glucocorticoid response element in the 5′-flanking region of the Pmch gene, which could account for the mechanism through which the absence of CORT after ADX promotes a decrease in Pmch mRNA expression.
Amylin is a pancreatic peptide hormone, co-synthesized with insulin by β-cells in response to feeding (Leffert et al., 1989), although central AMY has been described as a lactation-related peptide synthesized in the MPOA (Dobolyi, 2009). AMY has an anorectic action, suppressing feeding in both rats and mice, suggesting a post-prandial satiety effect and long-term lipostatic signaling (Lutz et al., 2001; Rushing, 2003). The intraperitoneal injection in rats of salmon calcitonin, an AMY receptor agonist, decreases the levels of LHA Pmch mRNA (Barth et al., 2003). This effect is probably not direct, since there is little evidence for AMY binding in the LHA (Sexton et al., 1994), suggesting that AMY signaling reaches MCH neurons through circuits that may include the AP (Lutz et al., 2001), VTA (Mietlicki-Baase et al., 2013, 2015) and LDTg (Reiner et al., 2017).
Outputs
The regulation of feeding by MCH neurons is a complex mechanism that involves many aspects of this behavior, including the energy balance of the organism, metabolic rate, rewarding circuits, locomotor activity, arousal, spatial memory, olfactory cues and complex interactions with other peptidergic systems. In this section, we will highlight the main actions of MCH and its underlying connectivity, illustrated in Figure 2B.
Locomotor Activity
MCH-ir neurons project diffusely to numerous areas of the isocortex, including the SSCx, MCx, infralimbic, and Ins, as shown by Saper et al. (1986) using an antibody for α-MSH that was, as we now know, cross-reacting to MCH. This pattern of MCH projections was confirmed by Cvetkovic et al. (2003) using an antibody for salmonid MCH and further explored by Elias et al. (2008), that showed extensive innervation of the MCx by MCH neurons of the LHA. These authors also reported that a subset of these MCH-ir MCx-innervating neurons also innervate the PPTg, what may also contribute to a motor regulation through the mesencephalic locomotor region of this area. This circuit supports an action of MCH decreasing locomotor activity as reported in Pmch-/- and Mchr1-/- mice (Segal-Lieberman et al., 2003; Willie et al., 2008), as well as progressive (Alon and Friedman, 2006) and sudden (Whiddon and Palmiter, 2013) adult ablation of MCH neurons. Through this pathway, MCH neurons decrease locomotor activity and energy expenditure to revert states of negative energy balance or sustain states of positive energy balance.
Energy Expenditure
Locomotor activity is not the only way the CNS can modulate energy expenditure, since thermogenesis also play an important role in this aspect of homeostatic balance. The first link between MCH and energy expenditure control was drawn by Shimada et al. (1998), that noticed a reduction in body weight that surpassed the reduction in food intake in Pmch-/- animals. Although these animals did not have a lower rectal temperature when compared to WT mice, their rate of O2 consumption normalized for body weight was 20% higher. Mice lacking MCHR1 also display higher metabolic rates during the dark phase as measured by indirect calorimetry (Marsh et al., 2002).
Using a model of cold exposure, Pereira-da-Silva et al. (2003) showed that MCH is increased by almost 60% in cold-exposed animals. These animals display a series of physiological adaptations to the challenging temperature that include an increase in food consumption, fall of body weight and thermogenesis. These authors report that cold-exposed animals with impaired MCH synthesis have an increase in the molecular machinery necessary for BAT-dependent thermogenesis when compared to control animals, suggesting that MCH inhibits BAT thermogenic activity, potentially conserving energy for homeostatic purposes. To investigate the chemical signature of the hypothalamic circuits modulating BAT activity, Oldfield et al. (2002) injected a polysynaptic viral tracer in the BAT of adult rats combined to immunohistochemistry for different neuropeptides synthesized in the hypothalamus. Third-order MCH-ir neurons were found in the LHA, suggesting that MCH neurons provide input to the BAT through brainstem nuclei.
These results are reinforced by the works of Guesdon et al. (2009) and Chung et al. (2010). Guesdon et al. (2009) report that intraventricular injections of MCH decrease lipid oxidation and Chung et al. (2010) demonstrated that Mchr1-/- animals are resistant to weight gain when exposed to HFD due increased lipid metabolism. Furthermore, Verty et al. (2010), using temperature-sensitive telemetry probes, registered a significant increase in BAT temperature after intraventricular injection of an MCHR1 antagonist. All these data suggest that LHA MCH neurons polysynaptically decrease thermogenesis in BAT to reduce lipid metabolism and energy expenditure, an important mechanism to sustain a positive energy balance or revert a scenario of negative energy balance.
Autonomic Modulation
Saper et al. (1976) have shown that LHA neurons project both to autonomic centers of the brainstem, such as the dorsal motor nucleus of the vagus and the NTS, and directly to the SpCd, including the ImC in thoracic levels, suggesting a descending hypothalamo-autonomic pathway that access directly the preganglionic cells of the parasympathetic and sympathetic systems. Since MCH-ir fibers from the LHA also sparsely innervate the autonomic nuclei of the brainstem and all levels of the SpCd (Kohler et al., 1984; Bittencourt et al., 1992; Bittencourt and Elias, 1998), this represents a possible pathway through which MCH may integrate ingestive behavior to autonomic function, although further evidence is necessary to establish if there is a direct contact between ganglionic cells and MCH neurons, possibly through specific viral tracers. More recently, Pérez et al. (2011) injected a polysynaptic viral tracer in the SAL and MAS of mice and discovered that MCH-ir neurons of the LHA provide inputs to brainstem nuclei involved in the control of these structures (MoV – MAS innervation; 7N and SuS – SAL innervation). Through this innervation, LHA MCH-containing neurons can gate two important preparatory responses to feeding: salivation, an autonomic response necessary for bolus formation and swallowing, and mastication, a motor response which involves complex coordination between muscles of the jaw, face, and tongue. Another interesting aspect of this MCH input is the fact that both salivation and mastication are under the control of higher-order cortical centers (Thexton, 1992; Hubschle et al., 1998), and MCH could potentially integrate this higher-center input and provide the output to lower brainstem nuclei.
An indirect mechanism for this feeding-autonomic coupling is through the PVH. An important number of labeled cells are found in the PVH after retrograde tracer injection in the SpCd, suggesting that this nucleus also acts over autonomic systems (Hancock, 1976; Saper et al., 1976; Ono et al., 1978; Blessing and Chalmers, 1979; Hosoya and Matsushita, 1979). The PVH neurons that project to the SpCd and to the dorsal vagal complex are concentrated in the dorsal, lateral, and medial parts of the PVHp (Swanson and Kuypers, 1980), regions that receive more MCH-ir innervation than the PVHm (Bittencourt et al., 1992). Since injections of MCH restricted to the PVH without spilling to the ventricular system promote an increase in feeding behavior (Abbott et al., 2003), this could represent another node through which MCH integrates feeding behavior and autonomic function, triggering the necessary autonomic preparatory processes to feeding.
Thyroid Modulation
Kennedy et al. (2001) suggest that MCH could be part of the peptidergic modulation of the HPT axis. After icv injections of MCH, there is an in vivo decrease in the plasmatic levels of TSH (10 and 60 min after injection). In vitro, the addition of MCH and NEI in hypothalamic cultures promotes a decrease in the levels of TRH, and MCH co-applied with TRH decreases the secretion of TSH in cultures of hypophysaire cells when compared to TRH alone. MCH may act both through a direct and an indirect pathway to control the HPT axis activity. Directly, MCH-ir fibers from the LHA could innervate TRH-ir neurons in the PVH, decreasing their secretory activity. In the indirect pathway, MCH-ir projections to the Arc could potentially act over the α-MSH/CART and NPY/AgRP systems to promote downstream alterations in TRH synthesis. There is anatomical support for both pathways. Both MCH-ir fibers and MCHR1 immunoreactivity are found in the PVH of rats (Bittencourt et al., 1992; Hervieu et al., 2000; Saito et al., 2001) and a reporter to associated to Mchr1 can be detected in TRH-ir cells of the PVH (Chee et al., 2013). An Arc mediated pathway is supported by the observation that the neonatal ablation of the Arc abolishes the decrease in PVH| TRH resulting from fasting (Legradi et al., 1998). NPY-ir and AgRP-ir nerve terminals contact TRH-ir neurons in the PVH, and the main source for this innervation is the Arc (Legradi and Lechan, 1998, 1999). A similar innervation of pro-TRH mRNA expressing-neurons is also observed for α-MSH and CART fibers (Fekete et al., 2000). Regardless of the pathway, considering the energy conservation effects of MCH, it is likely that this peptide acts on the HPT axis to decrease energy expenditure.
Circulation
There are few studies that examined the levels of MCH in the bloodstream. Evaluating the possibility that MCH was produced and released by adipocytes, Bradley et al. (2000) did not detect Pmch mRNA in these cells, but they detected MCH in the plasma through a RIA. Stricker-Krongrad et al. (2001) evaluated the amount of MCH in the serum of Zucker rats and found that MCH levels were increased in the obese animals compared to the lean ones. On the other hand, in Wistar rats with obesity resulting from electrolytic VMH or PVH lesions, there was a decrease in the intensity of MCH staining in the LHA (9.3% for VMH lesions and 21.9% for PVH lesions), but the decrease in serum MCH levels did not reach statistical significance (Sun et al., 2004). It is important to consider, however, that Sun et al. (2004) used densitometry in LHA slices after immunohistochemistry to quantify the alterations in MCH immunofluorescence, a method that allows the detection of general differences but renders impossible to define if subsets of neurons had alterations. Therefore, it is still possible that some neurons displayed a decrease in MCH synthesis in response to the lesions, but the neurosecretory neurons kept stable levels of synthesis.
Gavrila et al. (2005) examined serum levels of MCH in humans, evaluating correlation factors for diverse parameters such body fat, leptin, estrogen and testosterone, dietary patterns and fasting. These authors found a direct correlation between serum MCH and fat mass and fat percentile, but no correlation could be drawn between MCH levels and estrogen, testosterone or dietary parameters. Working also with humans, Carnier et al. (2010) evaluated obese adolescents with eating disorders after 6 months and 1 year of interdisciplinary therapy, and observed a short-term increase of MCH in the blood of these patients followed by a decrease after 1 year of therapy. These authors suggest that the short-term increase in energy demand upregulate the release of MCH and the long-term correction of dietary habits promote a lower MCH baseline. During the preparation of this review, a new study about circulating MCH in humans was published by Naufahu et al. (2017). In this study, a reliable RIA was developed and tested in over 200 humans of both sexes and different body fat profiles, revealing a plasma MCH baseline of 19.5–55.4 pg/ml and some differential regulation of MCH that depends on both gender and adiposity, although further studies are necessary to fully elucidate how that regulation occurs.
The data regarding circulating MCH must be examined with caution. One major caveat is that Waters and Krause (2005), in a letter to the editor addressing the work of Gavrila et al. (2005), report that the commercial RIA used in most of these studies for MCH has non-specific reactivity with serum elements, since samples from Pmch-/- mice resulted in a positive signal. However, it is now hard to dispute that MCH is found in physiological levels in the bloodstream after the experiments ran by Naufahu et al. (2017). Another important aspect about circulating MCH is that its source is still unknown. Several peripheral tissues synthesize MCH (Hervieu and Nahon, 1995), and the role of this synthesis is, in many cases, still not associated with specific functions. Viale et al. (1997) showed that peripheral MCH has a different structure when compared to central MCH, as it is processed differently and its sequence combines central MCH and NEI. Since many peripheral tissues display MCHR1 mRNA (Saito et al., 1999; Hill et al., 2001), it is highly likely that MCH released in the bloodstream from one or more sources can reach those tissues and play modulatory roles. The study of MCH as a secreted factor in the bloodstream represents, therefore, a prolific field of MCH study.
Reward
In an oversimplification, feeding behavior is stimulated by two main drives: a metabolic drive, guided by homeostatic cues aiming to keep the energy balance of the animal, and a reward drive that involves complex emotional aspects and is directly linked to the subjective experience of pleasure. The reward aspect of feeding is a major component of that behavior, playing a preeminent role in our modern society. Rats are willing to expose themselves to aversive stimuli, such as foot-shock, painful heat and intense cold to obtain palatable food, such as candies and soda (Cabanac and Johnson, 1983; Foo and Mason, 2005; Oswald et al., 2011). As the complex relationship between metabolic and reward aspects has been thoroughly examined by others (Saper et al., 2002; Berthoud, 2011; Ferrario et al., 2016), in this review, we will focus on the role that MCH may play coordinating these different drivers.
The motivation and reward system involves a complex circuit with many relevant nodes, including the prefrontal cortex, striatum/Acb and the VTA. One of the regions with highest Mchr1 mRNA expression is the Acb (Hervieu et al., 2000; Saito et al., 2001) and MCH fibers are found in this area (Bittencourt et al., 1992), especially numerous in the septal pole of the AcbSh (corresponding to the dorsocaudomedial AcbSh) (Haemmerle et al., 2015). Haemmerle et al. (2015) also report that accumbal MCH-innervation originates from neurons in all major hypothalamic areas containing MCH-ir neurons, with the highest rate of neurons simultaneously labeled for MCH and retrograde tracer found in the IHy. These results are in good agreement to those of Kampe et al. (2009) that report AcbSh-projecting MCH neurons in the lateral hypothalamus that also project to the CgCx/Ins.
MCH influences feeding behavior through the AcbSh, as MCH injected in this area promotes an increase in chow consumption, while an MCHR1 antagonist injected in this area decreases feeding (Georgescu et al., 2005). These authors also report that MCHR1 co-localizes to enkephalin and Dyn in the AcbSh, suggesting that MCH may influence the opioid system through these cells, a possible crossroad between MCH and the reward system (Holtzman, 1974; Frenk and Rogers, 1979). These results are supported by the work of Mul et al. (2011) that, using the only published model of Pmch-/- rat, found that the MCH signal on the AcbSh is important to keep the motivational aspects of the feeding behavior. Therefore, this hodological aspect between the LHA, the AcbSh and limbic areas is fundamental for the characteristic control of energy balance and to increase motivational or incentive-related aspects of food consumption.
A new facet of MCH actions on reward was provided by Karlsson et al. (2012). Using an MCHR1 antagonist combined with self-administration of sucrose (caloric and sweet) or saccharin (just sweet), these authors found that antagonist administration reduces sucrose self-administration, but not saccharin-reinforced lever-pressing, indicating that MCH conveys rewarding signals from the caloric content of consumed food. The antagonist administration also decreased cue-induced reinstatement of sucrose seeking, confirming that MCH participates in the rewarding properties of sucrose.
Domingos et al. (2013) extended these result employing optogenetic manipulation of MCH neurons in mice. Photostimulation of MCH neurons is able to revert the innate preference of mice for sucrose over saccharin and stimulate DA release in the striatum. A similar effect is observed in sweet-blind mice, suggesting that taste is not necessary for MCH-dependent preference for caloric ingestion. Contributing to these effects may be an activation of DA neurons in the VTA, as MCH ablation reduced FOS synthesis in this area. The VTA is densely innervated by MCH (Bittencourt et al., 1992) and MCHR1 is found in low (Saito et al., 2001; Pissios et al., 2008) to moderate (Hervieu et al., 2000) numbers in this area. CART/MCH terminals are found in the VTA, and CART-ir fibers contact DA neurons, suggesting that MCH and CART may work in tandem to modulate DA release by VTA neurons (Dallvechia-Adams et al., 2002), although electrophysiological data suggests otherwise (Korotkova et al., 2003). One possible explanation is that CART and MCH may not act directly on DA neurons, but modulate their auto inhibition. CART impairs DA-D2 binding when co-applied with stimulatory substances (Jaworski et al., 2003; Kim et al., 2003; Moffett et al., 2011), and DA neurons are auto inhibited through D2 (Johnson and North, 1992; Momiyama et al., 1993), suggesting that CART may decrease DA-dependent inhibition of DA neurons, increasing their activity. Since MCH can depress the presynaptic machinery of release (Gao and van den Pol, 2001), MCH released with CART in the synapse could depress further CART release, preventing too much disinhibition of DA neurons. A similar mechanism to this has an experimental basis in the work of Yang and Shieh (2005), on which MCH impaired CART stimulation of DA activity in the Acb. Further experimental evidence, however, is necessary to test if this is the mechanism of action for MCH, CART, and DA in the VTA.
The interactions between MCH and DA are not limited to the VTA, however, as these two may also interact directly on the Acb. When Chung et al. (2009) applied MCH or D1 and D2 agonists separately in the AcbSh, there was no change in animal behavior, but when the three were co-applied there was a potentiation of the cocaine rewarding response. Furthermore, Mchr1-/- animals displayed resistance to the rewarding effects of cocaine, in a similar way to the pharmacological blockade of this receptor. More work will be necessary to fully elucidate the interaction between MCH and DA, however, as Smith et al. (2005) observed upregulation of D1 and D2 in the AcbSh of Mchr1-/- mice and hyper sensibility to amphetamine, while methamphetamine responses were attenuated after injections in the AcbSh of MCH and amplified after MCHR1 agonists injections (Sun et al., 2013). Albeit Chung et al. (2009) suggest that these apparent contradictions can be explained by the different mechanisms of cocaine and amphetamine action, more evidence is necessary to confirm this and to allow us to understand the role that MCH may play in reward and addiction.
As a final consideration about MCH and reward, although alcohol consumption is not the theme of this review, both drug and food consumption have a common ground that is the reward motivation. Alcohol is a particularly interesting model, as it is both a stimulatory factor of the reward system and a source of caloric nutrient. Duncan et al. (2005) found an increase in 10% alcohol and isocaloric sucrose solutions intake after 3V MCH injections, suggesting that MCH may drive the pursuit of these substances for their rewarding or caloric value. Morganstern et al. (2010a) developed on these results, demonstrating that injections of MCH restricted to the PVH and Acb were able to increase alcohol consumption, suggesting those nuclei as downstream targets of MCH action on alcohol consumption. The exact mechanism by which this MCH modulation happens was, however, contentious, as some reports suggested that MCHR1 was not involved in the alcohol consumption-promoting effect of MCH (Duncan et al., 2006, 2007), while others found a potent decrease in alcohol consumption and reinstatement after an MCHR1 antagonist treatment (Cippitelli et al., 2010). Recently, Karlsson et al. (2016) reported that MCH and MCHR1 have a dual role in the regulation of alcohol intake through mechanisms related both to caloric intake and reward motivation. Further experiments on MCH alterations linked to alcohol consumption, however, are still necessary to provide an overarching explanation for all results reported in the literature. Understanding the mechanisms through which MCH modulates alcohol consumption may provide insightful views on addictive and excessive behaviors.
Foraging/Predation
The participation of MCH in the pre-ingestive steps of feeding is perhaps its most elusive aspect so far. Albeit the ad libitum diet used in most experimental designs is convenient to standardize parameters, it also creates a highly artificial setup for the animal. In its natural environment, the animal must constantly make decisions regarding food acquisition while factoring elements such as its current energetic availability compared to other needs, foreseen energetic necessity, proximity to food sources and the risk of being eaten by a predator or injured by another environmental hazard. Some animals must also decide when to hunt for prey, since predation may reward it with metabolic needs, if successful, but may also incur in wasted energy if a failure.
Among the many complex elements of foraging and predation, there are three aspects to which MCH may be associated to: sensory integration, decision-making, and memory. The first role proposed for MCH in the mammalian brain was over the modulation of auditory stimuli, by Miller et al. (1993). Using an auditory gating paradigm, these authors have shown that the suppression of a redundant auditory signal is abolished after MCH addition to the CSF, suggesting that MCH can induce a state of higher vigilance at the cost of decreased focus on a single signal. Besides auditory stimuli, Adams et al. (2011) provided evidence that MCH participates in the integration of olfactory stimuli through numerous MCH-ir fibers that are found in olfactory-linked structures such as the Pir and the OB. Using Pmch-/- mice, these researchers observed a decreased capacity for them to find food using olfactory cues, suggesting that MCH may display an important role in the search for food. MCH is, therefore, an important actor in the gating of sensorial information, altering perceptual properties of the animal (such as vigilance and focus) depending on the available energy stores.
Regarding decision-making, there is a paucity of functional information relating it to MCH. Projections arising from MCH neurons in the IHy and tuberal LHA densely and diffusely reach the PAG (Elias and Bittencourt, 1997). Comoli et al. (2003) have shown that the lateral aspects of the PAG are involved in predatory behavior, as FOS synthesis in this area is increased in rats after cockroach predation compared to control animals. It is possible, therefore, that MCH-ir projections to the PAG may influence predatory behavior, but experimental evidence is necessary to establish the PAG-mediated actions of MCH.
The third important aspect of foraging is memory, as animals that can adequately remember the location of food sources will have a survival advantage. The HF has been thoroughly implicated in memory (Scoville and Milner, 1957; Zola-Morgan et al., 1986; Squire, 1992) and is one of the major targets of MCH-ir fibers and MCHR1 synthesis in the rat brain (Bittencourt et al., 1992; Hervieu et al., 2000; Saito et al., 2001). Lima et al. (2013) provided a more detailed description of the MCH input to the HF, describing a higher density of MCH-ir fibers in the dorsal HF, especially in the CA3 field, and the presence of MCH-ir fibers plexuses around GABAergic basket cells in CA1 and CA3, in addition to apparent contacts between MCH-ir fibers and HF-projecting cholinergic cells of the MS.
Several authors have provided evidence that MCH plays a role in HF modulation. After CA1 MCH injections, rats show: improved acquisition and consolidation in an inhibitory avoidance test (Monzon et al., 1999); a decrease in the levels of nitric oxide and its second messenger cGMP (Varas et al., 2002a); and a facilitation in long-term potentiation through alterations in the NMDA receptor-gated channel (Varas et al., 2002b, 2003). Adamantidis et al. (2005) provided further evidence for these memory-facilitation actions of MCH by reporting that Mchr1-/- mice have worse memory retention of aversive stimuli and decreased response of pyramidal cells to NMDA in the CA1. Pachoud et al. (2010) expanded the roles of MCH in HF plasticity, reporting that Mchr1-/- mice have decreased output from excitatory GLUergic Schaffer Collaterals from the CA3 to the CA1 through both AMPA- and NMDA-mediated responses. In summary, these results point to MCH influencing GLUergic transmission in the CA1 field through both presynaptic mechanisms and the increase of NMDAR and AMPAR.
While most studies focused on the effects of MCH in the CA1 field, Sita et al. (2016) investigated the effects of MCH immunoneutralization centered on the CA3. Animals with impaired MCH signaling in the CA3 were more effective recalling the place where food was buried during the training phase, while control animals took longer and dug in other sites before attempting the right quadrant. The most reasonable explanation for the observed results is that animals could perceive olfactory cues indicating that there was no food in the test trial, so control animals searched for food in the arena while MCH-impaired animals were unable to incorporate these cues to the previously stored information.
What circuit could underlie the observations of Sita et al. (2016)? According to Easton et al. (2012), there is a dichotomy between the encoding of new afferent information into preexisting memories, a phenomenon dependent of the entorhinal cortex and the CA1 field, and the retrieval of memories, based on CA3–CA1 interactions. Therefore, the inhibition of MCH in the CA3 may have favored this latter circuit, allowing the animal to better recruit the location of the buried empty petri dish, but impairing its ability to perceive the absence of food, indicating that normal MCH activity in the CA3 is necessary for regular integration of sensory information to acquired memories, in a similar fashion to cholinergic input (Easton et al., 2012).
Other Behaviors
The actions of MCH on behaviors not related to energy homeostasis are less understood, although recent developments began to shine some light in these other actions (Figure 6). There is ample morphological support for MCH actions over sexual behavior, as MCH-ir fibers are found in multiple relevant areas such as the AVPV, the MPOA, the AHA, and the ME (Bittencourt et al., 1992), where they may contact GnRH-ir neurons (Williamson-Hughes et al., 2005; Ward et al., 2009; Wu et al., 2009; Skrapits et al., 2015) and fibers (Williamson-Hughes et al., 2005; Ward et al., 2009). This MCH–GnRH interaction may underlie the modulation of MCH over LH release, although the exact mechanism through which this modulation occurs is still contentious (Gonzalez et al., 1997; Murray et al., 2000a, 2006; Tsukamura et al., 2000; Wu et al., 2009). In this regard, the MPOA and the IHy seem to play an important role (Murray et al., 2000b,c, 2006).
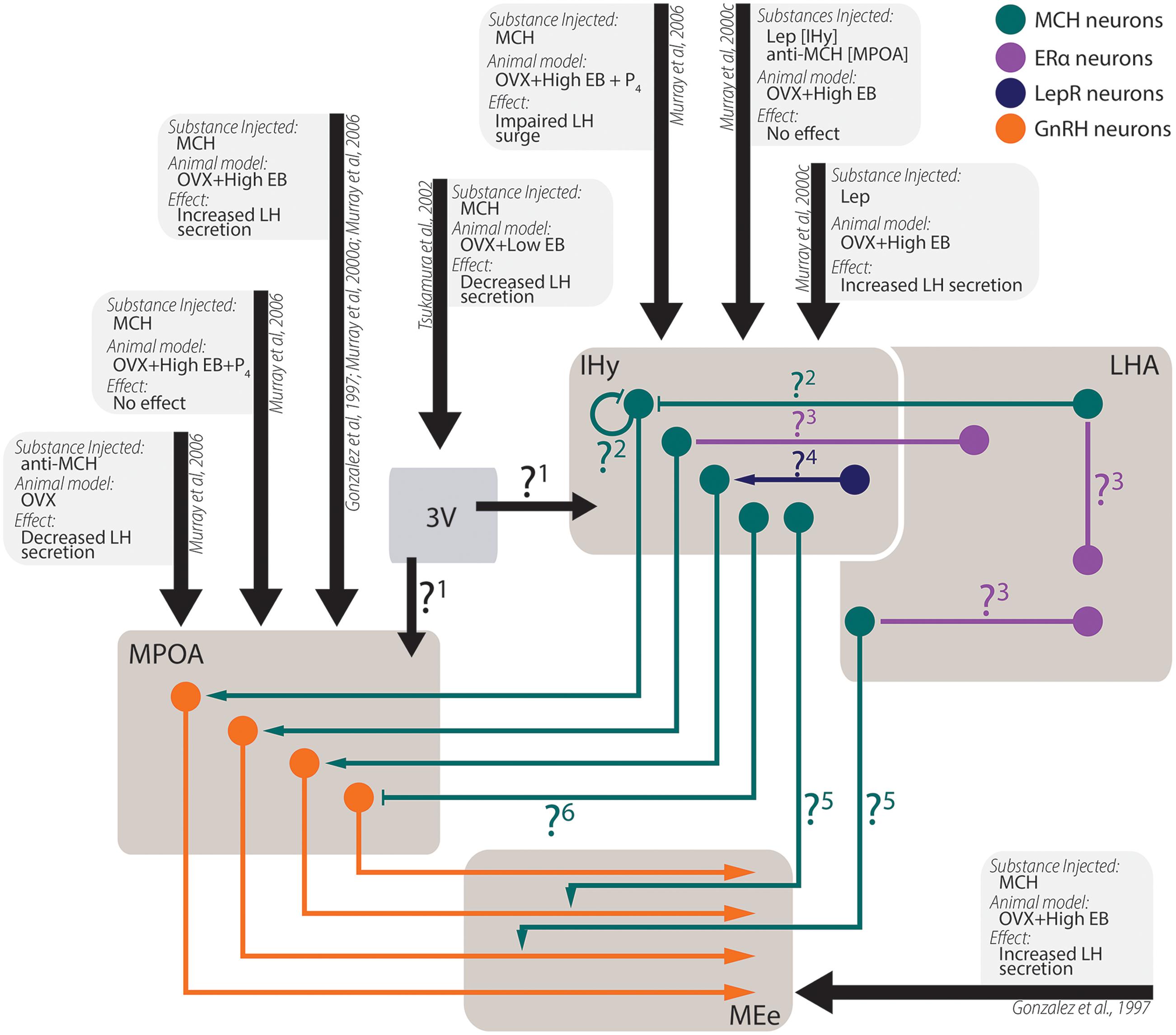
FIGURE 6. Experimental data from in vivo experiments regarding MCH actions on sexual physiology. Simplified schematic representation of the main findings regarding MCH actions after intracerebroventricular injections in areas related to sexual physiology and the circuit underlying these observations. Several questions remain open regarding the pathways necessary for the observed results: 1. After intraventricular injections, a decrease in LH secretion was observed, although several other authors report increases in LH secretion after intranuclear injections. It is unknown, at this time, if this contradictory observation stems from MCH action on areas that may negatively modulate LH secretion or if the low EB milieu employed by the authors may have contributed to the observed differences; 2. MCH injections centered on the IHy impair the LH surge expected after OVX+EB+P4 treatment, suggesting that auto-receptors or extra-IHy MCH inputs may negatively modulate LH release; 3. Although physiological E2 levels are necessary for MCH-dependent modulation of LH secretion, MCH neurons do not co-localize with ERα, suggesting that E2 action over MCH neurons depend on polysynaptic circuits. These circuits are yet to be demonstrated; 4. Leptin injected in the IHy increases MCH dependent-LH secretion, but MCH neurons do not co-localize to LepR, suggesting that polysynaptic circuits also convey leptinergic information to MCH neurons; 5. MCH-ir axons are found near GnRH fibers, and the density of MCH fibers in the MEe vary according to the estrous cycle of female, but the source of these MCH-ir fibers is still unknown; 6. Although several groups reported that MCH increases LH secretion by GnRH cells, electrophysiological data indicated that MCH hyperpolarizes GnRH neurons through postsynaptic mechanisms, therefore the exact mechanism for MCH-dependent LH secretion is uncertain.
Not only MCH appears to impact the sexual physiology, but steroidal hormones seem to influence the MCH peptidergic system as well. In OVX rats supplemented with EB, the orexigenic effects of MCH are impaired in comparison to male and OVX-only rats, an observation that can be extended to physiological conditions (Messina et al., 2006; Santollo and Eckel, 2008, 2013). These effects of E2, however, appear to be dependent on polysynaptic mechanisms, since MCH neurons are close to ERα-containing neurons, but they do not co-localize (Muschamp and Hull, 2007; Santollo and Eckel, 2013). Steroidal cues also play a role in MCH fiber density, as the number of MCH-ir fibers in the external layer of the ME (MEe) is increased in the diestrus and proestrus, in sharp contrast to what is observed during estrus or in males (Chiocchio et al., 2001; Gallardo et al., 2004).
In conclusion, although there is an important amount of information regarding the relationship between MCH and sexual behavior, especially through the modulation of gonadotropin release, further experiments will be necessary to understand the exact mechanism of MCH action in animals in their natural environment, in light of the complex influence that steroidal hormones and sites of action appear to exert. It is noteworthy, as others have pointed (Naufahu et al., 2013), that MCH actions on sexual behavior probably incur in important survival gains by better coordinating the time for animals to engage in reproductive behaviors, since doing so in states of negative energy balance could incur in danger to the animal’s life and their potential offspring.
There is also evidence for MCH actions on maternal behavior and the lactation period. The very first functional clue was presented by Parkes and Vale (1993), that applied MCH and NEI in a NH cell culture and found that the amount of OXT secreted increases around 188 and 245%, respectively. Adams et al. (2011) described that female Pmch-/- mice show a higher litter postpartum mortality (31.1 ± 13.6% Pmch-/- vs. 7.3 ± 4.2% WT), an observation that they attribute to the reduced capability of the female to integrate olfactory stimuli. Alvisi et al. (2016) report that pup-suckling stimulus does not elicit MCH neurons activation or increase the number of MCH-ir neurons in the MPOA, suggesting that hormonal and other sensorial cues have prevalent interactions modulating the MCH system during this period. Recently, Benedetto et al. (2014) and Alachkar et al. (2016) demonstrated that MCH has an important role in the expression of maternal behavior through the MPOA, acting as a promoter of maternal behavior in the early postpartum period and a selective inhibitor of appetitive components of this behavior at late stages. It is noteworthy that Alachkar et al. (2016) describe impaired maternal aggression in Mchr1-/-, while Adams et al. (2011) report that Pmch-/- animals have increased levels of aggression when on a group and faster initial aggressive response. Besides those two works, very little is known about a possible role for MCH in aggression and defensive behavior, so further experimental investigation is necessary to better elucidate this aspect of the MCH peptidergic system.
Although not motivated behaviors per se, sleep and arousal are essential processes with an important interplay with motivated behaviors, especially through hypothalamic circuits (Saper et al., 2001, 2005). Intraventricular injections of MCH increase the amount of time spent by rats in REM and slow-wave sleep (Verret et al., 2003). Likewise, the subcutaneous treatment with an MCHR1 antagonist decreases the amount of REM sleep (Ahnaou et al., 2008). Recently, Vetrivelan et al. (2016) demonstrated that MCH neuronal activity increases REM sleep, but MCH neurons are necessary for the normal wake-REM sleep rhythm. MCH actions on sleep depend on projections to the DR (Lagos et al., 2009, 2011), oral pontine reticular nucleus (Torterolo et al., 2009), horizontal limb of the diagonal band of Broca (Lagos et al., 2012), ventrolateral preoptic area (Benedetto et al., 2013) and to the locus coeruleus (Monti et al., 2015). Sleep-promoting actions of MCH may be important to reduce activity and conserve energy in states of negative energy balance, when the animal has a propensity to increase arousal to maximize foraging. The results of Willie et al. (2008) suggest that this is the case, as fasted Pmch-/- animals have increased activity and exaggerated REM sleep time reduction.
Concluding Remarks
In this review, we highlighted the circuitry that underlies the integrative functions of MCH. The MCH circuitry is an extremely relevant tool because it allows us to dissect some of the multiple inputs that reach the hypothalamus and the many targets that can be affected by this system. However, this exactly plethora of connections makes this peptidergic system overwhelmingly hard to study and to comprehend, as each experimental manipulation affects multiple physiological variables. It is not uncommon, therefore, to observe slightly distinct experimental approaches resulting in widely different results, sometimes to the point of an apparent contradiction between obtained data. Although diverse reasons can be pointed to explain this, it is remarkable to us that studying the MCH neuron as a single entity may be hampering the field. Studies like those of Burdakov et al. (2005) and Hausen et al. (2016) tell us that subsets of MCH neurons differ in respect to their electrophysiological responses to varied stimuli, while studies like those of Elias et al. (2008) and Kampe et al. (2009) provide evidence for differential projection fields among MCH neurons. This may be in the heart of our inability to turn MCH into a valid pharmacological target to treat obesity or psychiatric disorders (Méndez-Andino and Wos, 2007; Högberg et al., 2012), as the desirable effects of MCHR1 antagonism are overshadowed by unwanted side effects. Not everything is grim in the future of MCH study, however, as works that better characterize the morphology, the hodology and the chemical signatures of the LHA, such as those of Swanson et al. (2005), Hahn (2010), and Hahn and Swanson (2015), are important steps in the better characterization of the intrinsic properties of subsets of MCH neurons. Once we understand their variability, we may be able to design drugs to act only on the desired aspects of the MCH peptidergic system and preserve those that must not be manipulated. Accurate morphological data, nevertheless, continues to be an essential tool for the better understanding of peptidergic systems and of the integrative properties of the hypothalamus as a whole.
Author Contributions
GD and JB contributed with the writing of this article and approved it for publication.
Funding
This article was supported by Fundação de Amparo à Pesquisa do Estado de São Paulo (São Paulo Research Foundation – FAPESP) grants n° 2016/02748-0 (GD) and n° 2010/52068-0 (JB). We would also like to thank Coordenação de Aperfeiçoamento de Pessoal de Nível Superior (Agency for the Advancement of Higher Education – CAPES, Grant #848/15). JB is an Investigator with the Conselho Nacional de Desenvolvimento Científico e Tecnológico (National Council for Scientific and Technological Development – CNPq).
Conflict of Interest Statement
The authors declare that the research was conducted in the absence of any commercial or financial relationships that could be construed as a potential conflict of interest.
The reviewer SEK and handling Editor declared their shared affiliation, and the handling Editor states that the process nevertheless met the standards of a fair and objective review.
Acknowledgment
We would like to thank Dr. Luciane Sita for her contributions revising some sections of this article, especially those regarding reproductive behavior.
Abbreviations
3V, third ventricle; 5-HT, serotonin; 5-HT?, unspecified serotonin receptor; 7N, nucleus of the cranial nerve VII; A10, ventral tegmental area dopaminergic group; A13, incerto-hypothalamic dopaminergic group; A8, retrorubral dopaminergic group; A9, substantia nigra dopaminergic group; ac, anterior commissure; Acb, nucleus accumbens; AcbC, nucleus accumbens, core subdivision; AcbSh, nucleus accumbens, shell subdivision; ACh, acetylcholine; ADX, adrenalectomized; AgRP, agouti-related protein; AH, adenohypophysis/anterior lobe of the pituitary; AHA, anterior hypothalamic area; AMPAR, AMPA ionotropic glutamate receptor; AMY, amylin; Amyg, amygdaloid nuclei; AON, anterior olfactory nucleus; AP, area postrema; Arc, arcuate nucleus; AV, anteroventral thalamic nucleus; AVP, vasopressin; AVPV, anteroventral periventricular nucleus; B7, dorsal raphe serotonergic group; B8, median raphe serotonergic group; BAT, brown adipose tissue; BNST, bed nucleus of the stria terminalis; CA, catecholamine; CA1, CA1 field of the hippocampus; CA2, CA2 field of the hippocampus; CA3, CA3 field of the hippocampus; CART, cocaine- and amphetamine-regulated transcript; CB1, cannabinoid receptor type 1; cc, corpus callosum; CeA, central nucleus of the amygdala; CG, central gray of the spinal cord; CgCx, cingulate cortex; CORT, cortisol/corticosterone; cPPTg, caudal pedunculopontine tegmental nucleus; CPu, caudate putamen; CRF, corticotrophin-releasing factor; D1, dopamine receptor subtype 1; D2, dopamine receptor subtype 2; DA, dopamine; DG, dentate gyrus; DMH, dorsomedial hypothalamic nucleus; DR, dorsal raphe nucleus; DTM, dorsal tuberomammillary nucleus; Dyn, dynorphins; EB, estradiol benzoate; ERα, estrogen receptor type α; EW, Edinger–Westphal nucleus; EWcp, centrally projecting Edinger–Westphal nucleus; f, fornix; FOS, FOS proto-oncogene, AP-1 transcription factor subunit; GABAA, GABA ionotropic receptor; GIRK, G protein-coupled inwardly rectifying K+; GLUT3, glucose transporter 3; GnRH, gonadotropin-releasing hormone; GP, globus pallidus; Hab, habenular nuclei; Hcrt, Orexin/hypocretin gene; HF, hippocampal formation; HPA, hypothalamus-pituitary-adrenal; HPT, hypothalamus-pituitary-thyroid; Hyp, hypothalamic nuclei; IC, inferior colliculus; IHy, incerto-hypothalamic area; ImC, intermediolateral column of the spinal cord; Ins, insular cortex; IR, insulin receptor; KATP, ATP-sensitive potassium channel; LD, laterodorsal thalamic nucleus; LDTg, laterodorsal tegmental nucleus; Lep, leptin gene; LepR, leptin receptor; LepR, leptin receptor gene; LG, lateral geniculate nucleus; LH, luteinizing hormone; LHA, lateral hypothalamic area; LHAa, anterior part of the LHA; LHAp, posterior part of the LHA; LM, lateral mammillary nucleus; LPOA, lateral preoptic area; LS, lateral septal nucleus; mACh, metabotropic ACh receptor; MAS, masseter muscle; MCH, melanin-concentrating hormone; MCH1, teleost melanin-concentrating hormone subtype 1; MCH2, teleost melanin-concentrating hormone subtype 2; MCHR1, MCH receptor type 1; Mchr1, MCH receptor 1 gene; MCx, motor cortex; MD, mediodorsal thalamic nucleus; ME, median eminence; MEe, external layer of the median eminence; MEi, internal layer of the median eminence; mfb, medial forebrain bundle; MG, medial geniculate nucleus; mGlu, unspecified metabotropic GLU receptor; mGlu1, metabotropic GLU receptor subtype 1; mGlu5, metabotropic GLU receptor subtype 5; MidThal, midline thalamic nuclei; ml, medial lemniscus; MM, medial mammillary nucleus; MnR, median raphe nucleus; MoV, motor nucleus of the V nerve; MPOA, medial preoptic area; MRt, medullary reticular formation; MS, medial septal nucleus; MSN, medium spiny neuron; N/OFQ, nociceptin/orphanin FQ; NCX, Na+/Ca2+ exchanger; NE, norepinephrine; NEI, neuropeptide E-I; NGE, neuropeptide G-E; NH, neurohypophysis/posterior lobe of the pituitary; NMDAR, NMDA ionotropic GLU receptor; NOP, nociceptin/orphanin Q receptor; NPY, neuropeptide Y; NREM, non-rapid eye movement sleep; NTS, nucleus of the solitary tract; Nts, neurotensin; OB, main olfactory bulb; opt, optic tract; ORX, orexins/hypocretins; ORXA, orexin A/hypocretin-1; ORXB, orexin B/hypocretin-2; ORXR, unspecified orexin receptor; ORXR2, orexin receptor type 2; OT, olfactory tubercle; OXT, oxytocin; OXTR, oxytocin receptor; OVX, ovariectomized; P4, progesterone; P2?, unspecified purinergic receptor; PAG, periaqueductal gray matter; PAGvl, ventrolateral periaqueductal gray matter; PAGdm, dorsomedial periaqueductal gray matter; PB, parabrachial nucleus; Pe, periventricular hypothalamic nucleus; PaF, parafascicular thalamic nucleus; PHA, posterior hypothalamic area; PI3K, phosphatidylinositol 3-kinase; PIP3, phosphatidylinositol-3,4,5-trisphosphate; PKA, protein kinase A; PKC, protein kinase C; PLC, phospholipase C; Pmch, Pro-melanin-concentrating hormone gene; PnRt, pontine reticular formation; Po, posterior thalamic complex; POMC, proopiomelanocortin; PPTg, pedunculopontine tegmental nucleus; Pr, prepositus nucleus; PreCBL, precerebellar nuclei; Pretect, pretectal nuclei; PV, paraventricular thalamic nucleus; PVH, paraventricular hypothalamic nucleus; PVHp, parvocellular subdivision of the paraventricular hypothalamic nucleus; PVHm, magnocellular subdivision of the paraventricular hypothalamic nucleus; Pir, piriform cortex; REM, rapid eye movement sleep; RIA, radioimmunoassay; rPPTg, rostral pedunculopontine tegmental nucleus; SAL, salivary glands; SuS, superior salivatory nucleus; SC, superior colliculus; scp, superior cerebellar peduncle; SHi, septohippocampal nucleus; SM, supramammillary nucleus; SN, substantia nigra; SpCd, spinal cord; SpV, spinal trigeminal nucleus; SSCx, somatosensory cortex; st, stria terminalis; SubThal, subthalamus; TRH, thyrotropin-releasing hormone; TRHR, unspecified thyrotropin-releasing hormone receptor; TRPC, transient receptor potential channel; TSH, thyroid-stimulating hormone; UCN1, urocortin 1; UCN2, urocortin 2; UCN3, urocortin 3; V1a, vasopressin receptor subtype 1a; VDCC, voltage-dependent calcium channel; Vest, vestibular nuclei; VL, ventrolateral thalamic nucleus; VMH, ventromedial hypothalamic nucleus; VTA, ventral tegmental area; YR, unspecified NPY receptor; ZI, zona incerta; α-MSH, α-melanocyte-stimulating hormone; αR, unspecified adrenergic receptor; α2A, adrenergic receptor type 2A; κOR, opioid receptor type κ.
References
Abbott, C. R., Kennedy, A. R., Wren, A. M., Rossi, M., Murphy, K. G., Seal, L. J., et al. (2003). Identification of hypothalamic nuclei involved in the orexigenic effect of melanin-concentrating hormone. Endocrinology 144, 3943–3949. doi: 10.1210/en.2003-0149
Adamantidis, A., Thomas, E., Foidart, A., Tyhon, A., Coumans, B., Minet, A., et al. (2005). Disrupting the melanin-concentrating hormone receptor 1 in mice leads to cognitive deficits and alterations of NMDA receptor function. Eur. J. Neurosci. 21, 2837–2844. doi: 10.1111/j.1460-9568.2005.04100.x
Adams, A. C., Domouzoglou, E. M., Chee, M. J., Segal-Lieberman, G., Pissios, P., and Maratos-Flier, E. (2011). Ablation of the hypothalamic neuropeptide melanin concentrating hormone is associated with behavioral abnormalities that reflect impaired olfactory integration. Behav. Brain Res. 224, 195–200. doi: 10.1016/j.bbr.2011.05.039
Ahnaou, A., Drinkenburg, W. H., Bouwknecht, J. A., Alcazar, J., Steckler, T., and Dautzenberg, F. M. (2008). Blocking melanin-concentrating hormone MCH 1 receptor affects rat sleep–wake architecture. Eur. J. Pharmacol. 579, 177–188. doi: 10.1016/j.ejphar.2007.10.017
Alachkar, A., Alhassen, L., Wang, Z., Wang, L., Onouye, K., Sanathara, N., et al. (2016). Inactivation of the melanin concentrating hormone system impairs maternal behavior. Eur. Neuropsychopharmacol. 26, 1826–1835. doi: 10.1016/j.euroneuro.2016.08.014
Alberto, C. O., Trask, R. B., and Hirasawa, M. (2011). Dopamine acts as a partial agonist for α2A adrenoceptor in melanin-concentrating hormone neurons. J. Neurosci. 31, 10671–10676. doi: 10.1523/JNEUROSCI.6245-10.2011
Alexandre, C., Andermann, M. L., and Scammell, T. E. (2013). Control of arousal by the orexin neurons. Curr. Opin. Neurobiol. 23, 752–759. doi: 10.1016/j.conb.2013.04.008
Allen, Y. S., Adrian, T. E., Allen, J. M., Tatemoto, K., Crow, T. J., Bloom, S. R., et al. (1983). Neuropeptide Y distribution in the rat brain. Science 221, 877–879. doi: 10.1126/science.6136091
Alon, T., and Friedman, J. M. (2006). Late-onset leanness in mice with targeted ablation of melanin concentrating hormone neurons. J. Neurosci. 26, 389–397. doi: 10.1523/JNEUROSCI.1203-05.2006
Alvisi, R., Diniz, G., Da-Silva, J., Bittencourt, J., and Felicio, L. (2016). Suckling-induced Fos activation and melanin-concentrating hormone immunoreactivity during late lactation. Life Sci. 148, 241–246. doi: 10.1016/j.lfs.2016.02.038
Amano, M., Takahashi, A., Oka, Y., Yamanome, T., Kawauchi, H., and Yamamori, K. (2003). Immunocytochemical localization and ontogenic development of melanin-concentrating hormone in the brain of a pleuronectiform fish, the barfin flounder. Cell Tissue Res. 311, 71–77. doi: 10.1007/s00441-002-0660-6
Amores, A., Catchen, J., Ferrara, A., Fontenot, Q., and Postlethwait, J. H. (2011). Genome evolution and meiotic maps by massively parallel DNA sequencing: spotted gar, an outgroup for the teleost genome duplication. Genetics 188, 799–808. doi: 10.1534/genetics.111.127324
Andén, N. E., Dahlström, A., Fuxe, K., Larsson, K., Olson, L., and Ungerstedt, U. (1966). Ascending monoamine neurons to the telencephalon and diencephalon. Acta Physiol. 67, 313–326. doi: 10.1111/j.1748-1716.1966.tb03318.x
Andersen, A. C., Pelletier, G., Eberle, A. N., Leroux, P., Jegou, S., and Vaudry, H. (1986). Localization of melanin-concentrating hormone-like immunoreactivity in the brain and pituitary of the frog Rana ridibunda. Peptides 7, 941–951. doi: 10.1016/0196-9781(86)90119-1
Apergis-Schoute, J., Iordanidou, P., Faure, C., Jego, S., Schöne, C., Aitta-Aho, T., et al. (2015). Optogenetic evidence for inhibitory signaling from orexin to MCH neurons via local microcircuits. J. Neurosci. 35, 5435–5441. doi: 10.1523/JNEUROSCI.5269-14.2015
Banks, W. A., Kastin, A. J., Huang, W., Jaspan, J. B., and Maness, L. M. (1996). Leptin enters the brain by a saturable system independent of insulin. Peptides 17, 305–311. doi: 10.1016/0196-9781(96)00025-3
Barson, J. R., Morganstern, I., and Leibowitz, S. F. (2012). Neurobiology of consummatory behavior: mechanisms underlying overeating and drug use. ILAR J. 53, 35–58. doi: 10.1093/ilar.53.1.35
Barth, S. W., Riediger, T., Lutz, T. A., and Rechkemmer, G. (2003). Differential effects of amylin and salmon calcitonin on neuropeptide gene expression in the lateral hypothalamic area and the arcuate nucleus of the rat. Neurosci. Lett. 341, 131–134. doi: 10.1016/S0304-3940(03)00190-3
Batten, T., and Baker, B. (1988). Melanin-concentrating hormone (MCH) immunoreactive hypophysial neurosecretory system in the teleost Poecilia latipinna: light and electron microscopic study. Gen. Comp. Endocrinol. 70, 193–205. doi: 10.1016/0016-6480(88)90140-2
Benedetto, L., Pereira, M., Ferreira, A., and Torterolo, P. (2014). Melanin-concentrating hormone in the medial preoptic area reduces active components of maternal behavior in rats. Peptides 58, 20–25. doi: 10.1016/j.peptides.2014.05.012
Benedetto, L., Rodriguez-Servetti, Z., Lagos, P., D’almeida, V., Monti, J. M., and Torterolo, P. (2013). Microinjection of melanin concentrating hormone into the lateral preoptic area promotes non-REM sleep in the rat. Peptides 39, 11–15. doi: 10.1016/j.peptides.2012.10.005
Berman, J. R., Skariah, G., Maro, G. S., Mignot, E., and Mourrain, P. (2009). Characterization of two melanin-concentrating hormone genes in zebrafish reveals evolutionary and physiological links with the mammalian MCH system. J. Comp. Neurol. 517, 695–710. doi: 10.1002/cne.22171
Berthoud, H. R. (2011). Metabolic and hedonic drives in the neural control of appetite: who is the boss? Curr. Opin. Neurobiol. 21, 888–896. doi: 10.1016/j.conb.2011.09.004
Bird, D. J., and Baker, B. I. (1989). An immunological study of the secretory activity of neurons producing melanin-concentrating hormone in a teleost. Neuroscience 28, 245–251. doi: 10.1016/0306-4522(89)90248-0
Bird, D. J., Potter, I. C., Sower, S. A., and Baker, B. I. (2001). The distribution of melanin-concentrating hormone in the lamprey brain. Gen. Comp. Endocrinol. 121, 232–241. doi: 10.1006/gcen.2001.7609
Bittencourt, J., and Celis, M. E. (2008). Anatomy, function and regulation of neuropeptide EI (NEI). Peptides 29, 1441–1450. doi: 10.1016/j.peptides.2008.03.012
Bittencourt, J. C. (2011). Anatomical organization of the melanin-concentrating hormone peptide family in the mammalian brain. Gen. Comp. Endocrinol. 172, 185–197. doi: 10.1016/j.ygcen.2011.03.028
Bittencourt, J. C., and Elias, C. F. (1998). Melanin-concentrating hormone and neuropeptide EI projections from the lateral hypothalamic area and zona incerta to the medial septal nucleus and spinal cord: a study using multiple neuronal tracers. Brain Res. 805, 1–19. doi: 10.1016/S0006-8993(98)00598-8
Bittencourt, J. C., Frigo, L., Rissman, R. A., Casatti, C. A., Nahon, J. L., and Bauer, J. A. (1998). The distribution of melanin-concentrating hormone in the monkey brain (Cebus apella). Brain Res. 804, 140–143. doi: 10.1016/S0006-8993(98)00662-3
Bittencourt, J. C., Presse, F., Arias, C., Peto, C., Vaughan, J., Nahon, J. L., et al. (1992). The melanin-concentrating hormone system of the rat brain: an immuno- and hybridization histochemical characterization. J. Comp. Neurol. 319, 218–245. doi: 10.1002/cne.903190204
Bittencourt, J. C., Vaughan, J., Arias, C., Rissman, R. A., Vale, W. W., and Sawchenko, P. E. (1999). Urocortin expression in rat brain: evidence against a pervasive relationship of Urocortin-Containing projections with targets bearing type 2 CRF receptors. J. Comp. Neurol. 415, 285–312. doi: 10.1002/(SICI)1096-9861(19991220)415:3<285::AID-CNE1>3.0.CO;2-0
Blessing, W. W., and Chalmers, J. P. (1979). Direct projection of catecholamine (presumably dopamine)-containing neurons from hypothalamus to spinal cord. Neurosci. Lett. 11, 35–40. doi: 10.1016/0304-3940(79)90052-1
Bradley, R. L., Kokkotou, E. G., Maratos-Flier, E., and Cheatham, B. (2000). Melanin-concentrating hormone regulates leptin synthesis and secretion in rat adipocytes. Diabetes Metab. Res. Rev. 49, 1073–1077. doi: 10.2337/diabetes.49.7.1073
Brailoiu, G. C., Dun, S. L., Brailoiu, E., Inan, S., Yang, J., Chang, J. K., et al. (2007). Nesfatin-1: distribution and interaction with a G protein-coupled receptor in the rat brain. Endocrinology 148, 5088–5094. doi: 10.1210/en.2007-0701
Broberger, C., De Lecea, L., Sutcliffe, J. G., and Hokfelt, T. (1998). Hypocretin/orexin- and melanin-concentrating hormone-expressing cells form distinct populations in the rodent lateral hypothalamus: relationship to the neuropeptide Y and agouti gene-related protein systems. J. Comp. Neurol. 402, 460–474. doi: 10.1002/(SICI)1096-9861(19981228)402:4<460::AID-CNE3>3.0.CO;2-S
Brownstein, M. J., Palkovits, M., Saavedra, J. M., Bassiri, R. M., and Utiger, R. D. (1974). Thyrotropin-releasing hormone in specific nuclei of rat brain. Science 185, 267–269. doi: 10.1126/science.185.4147.267
Brüning, J. C., Gautam, D., Burks, D. J., Gillette, J., Schubert, M., Orban, P. C., et al. (2000). Role of brain insulin receptor in control of body weight and reproduction. Science 289, 2122–2125. doi: 10.1126/science.289.5487.2122
Burdakov, D., Gerasimenko, O., and Verkhratsky, A. (2005). Physiological changes in glucose differentially modulate the excitability of hypothalamic melanin-concentrating hormone and orexin neurons in situ. J. Neurosci. 25, 2429–2433. doi: 10.1523/JNEUROSCI.4925-04.2005
Burdakov, D., Karnani, M. M., and Gonzalez, A. (2013). Lateral hypothalamus as a sensor-regulator in respiratory and metabolic control. Physiol. Behav. 121, 117–124. doi: 10.1016/j.physbeh.2013.03.023
Cabanac, M., and Johnson, K. (1983). Analysis of a conflict between palatability and cold exposure in rats. Physiol. Behav. 31, 249–253. doi: 10.1016/0031-9384(83)90128-2
Campfield, L. A., Smith, F. J., Guisez, Y., Devos, R., and Burn, P. (1995). Recombinant mouse OB protein: evidence for a peripheral signal linking adiposity and central neural networks. Science 269, 546. doi: 10.1126/science.7624778
Cardot, J., Fellmann, D., and Bugnon, C. (1994). Melanin-concentrating hormone-producing neurons in reptiles. Gen. Comp. Endocrinol. 94, 23–32. doi: 10.1006/gcen.1994.1056
Cardot, J., Griffond, B., Blahser, S., and Fellmann, D. (1998). Melanin-concentrating hormone in the cock. Ann. N. Y. Acad. Sci. 839, 631–633. doi: 10.1111/j.1749-6632.1998.tb10900.x
Cardot, J., Griffond, B., Risold, P. Y., Blahser, S., and Fellmann, D. (1999). Melanin-concentrating hormone-producing neurons in birds. J. Comp. Neurol. 411, 239–256. doi: 10.1002/(SICI)1096-9861(19990823)411:2<239::AID-CNE5>3.0.CO;2-7
Carlezon, W. A., and Thomas, M. J. (2009). Biological substrates of reward and aversion: a nucleus accumbens activity hypothesis. Neuropharmacology 56, 122–132. doi: 10.1016/j.neuropharm.2008.06.075
Carnier, J., De Piano, A., De Lima Sanches, P., Tock, L., Do Nascimento, C. M., Oyama, L. M., et al. (2010). The role of orexigenic and anorexigenic factors in an interdisciplinary weight loss therapy for obese adolescents with symptoms of eating disorders. Int. J. Clin. Pract. 64, 784–790. doi: 10.1111/j.1742-1241.2009.02306.x
Challis, B., Coll, A., Yeo, G., Pinnock, S., Dickson, S. L., Thresher, R., et al. (2004). Mice lacking pro-opiomelanocortin are sensitive to high-fat feeding but respond normally to the acute anorectic effects of peptide-YY3-36. Proc. Natl. Acad. Sci. U.S.A. 101, 4695–4700. doi: 10.1073/pnas.0306931101
Chalmers, D. T., Lovenberg, T. W., and De Souza, E. B. (1995). Localization of novel corticotropin-releasing factor receptor (CRF2) mRNA expression to specific subcortical nuclei in rat brain: comparison with CRF1 receptor mRNA expression. J. Neurosci. 15, 6340–6350.
Chee, M. J., Pissios, P., and Maratos-Flier, E. (2013). Neurochemical characterization of neurons expressing melanin-concentrating hormone receptor 1 in the mouse hypothalamus. J. Comp. Neurol. 521, 2208–2234. doi: 10.1002/cne.23273
Cheung, C. C., Clifton, D. K., and Steiner, R. A. (1997). Proopiomelanocortin neurons are direct targets for leptin in the hypothalamus. Endocrinology 138, 4489–4492. doi: 10.1210/endo.138.10.5570
Chiocchio, S. R., Gallardo, M. G., Louzan, P., Gutnisky, V., and Tramezzani, J. H. (2001). Melanin-concentrating hormone stimulates the release of luteinizing hormone-releasing hormone and gonadotropins in the female rat acting at both median eminence and pituitary levels. Biol. Reprod. 64, 1466–1472. doi: 10.1095/biolreprod64.5.1466
Chometton, S., Franchi, G., Houdayer, C., Mariot, A., Poncet, F., Fellmann, D., et al. (2014). Different distributions of preproMCH and hypocretin/orexin in the forebrain of the pig (Sus scrofa domesticus). J. Chem. Neuroanat. 6, 72–82. doi: 10.1016/j.jchemneu.2014.08.001
Chung, S., Hopf, F. W., Nagasaki, H., Li, C. Y., Belluzzi, J. D., Bonci, A., et al. (2009). The melanin-concentrating hormone system modulates cocaine reward. Proc. Natl. Acad. Sci. U.S.A. 106, 6772–6777. doi: 10.1073/pnas.0811331106
Chung, S., Wong, T., Nagasaki, H., and Civelli, O. (2010). Acute homeostatic responses to increased fat consumption in MCH1R knockout mice. J. Mol. Neurosci. 42, 459–463. doi: 10.1007/s12031-010-9358-5
Cippitelli, A., Karlsson, C., Shaw, J. L., Thorsell, A., Gehlert, D. R., and Heilig, M. (2010). Suppression of alcohol self-administration and reinstatement of alcohol seeking by melanin-concentrating hormone receptor 1 (MCH1-R) antagonism in Wistar rats. Psychopharmacology 211, 367–375. doi: 10.1007/s00213-010-1891-y
Clark, J. T., Kalra, P. S., Crowley, W. R., and Kalra, S. P. (1984). Neuropeptide Y and human pancreatic polypeptide stimulate feeding behavior in rats. Endocrinology 115, 427–429. doi: 10.1210/endo-115-1-427
Clegg, D. J., Air, E. L., Benoit, S. C., Sakai, R. S., Seeley, R. J., and Woods, S. C. (2003). Intraventricular melanin-concentrating hormone stimulates water intake independent of food intake. Am. J. Physiol. Regul. Integr. Comp. Physiol. 284, R494–R499. doi: 10.1152/ajpregu.00399.2002
Comoli, E., Ribeiro-Barbosa, E. R., and Canteras, N. S. (2003). Predatory hunting and exposure to a live predator induce opposite patterns of Fos immunoreactivity in the PAG. Behav. Brain Res. 138, 17–28. doi: 10.1016/S0166-4328(02)00197-3
Conductier, G., Nahon, J.-L., and Guyon, A. (2011). Dopamine depresses melanin concentrating hormone neuronal activity through multiple effects on α2-noradrenergic, D1 and D2-like dopaminergic receptors. Neuroscience 178, 89–100. doi: 10.1016/j.neuroscience.2011.01.030
Cvetkovic, V., Brischoux, F., Griffond, B., Bernard, G., Jacquemard, C., Fellmann, D., et al. (2003). Evidence of melanin-concentrating hormone-containing neurons supplying both cortical and neuroendocrine projections. Neuroscience 116, 31–35. doi: 10.1016/S0306-4522(02)00557-2
Dahlström, A., and Fuxe, K. (1963). Evidence for the existence of monoamine-containing neurons in the central nervous system. I. Demonstration of monoamines in the cell bodies of brain stem neurons. Acta Physiol. Scand. Suppl. 232, 231–255.
Dallvechia-Adams, S., Kuhar, M. J., and Smith, Y. (2002). Cocaine- and amphetamine-regulated transcript peptide projections in the ventral midbrain: colocalization with gamma-aminobutyric acid, melanin-concentrating hormone, dynorphin, and synaptic interactions with dopamine neurons. J. Comp. Neurol. 448, 360–372. doi: 10.1002/cne.10268
De Vry, J., and Schreiber, R. (2000). Effects of selected serotonin 5-HT 1 and 5-HT 2 receptor agonists on feeding behavior: possible mechanisms of action. Neurosci. Biobehav. Rev. 24, 341–353. doi: 10.1016/S0149-7634(99)00083-4
Debons, A. F., Silver, L., Cronkite, E. P., Johnson, H. A., Brecher, G., Tenzer, D., et al. (1962). Localization of gold in mouse brain in relation to gold thioglucose obesity. Am. J. Physiol. 202, 743–750.
Della-Zuana, O., Presse, F., Ortola, C., Duhault, J., Nahon, J. L., and Levens, N. (2002). Acute and chronic administration of melanin-concentrating hormone enhances food intake and body weight in Wistar and Sprague-Dawley rats. Int. J. Obes. Relat. Metab. Disord. 26, 1289–1295. doi: 10.1038/sj.ijo.0802079
Dobolyi, A. (2009). Central amylin expression and its induction in rat dams. J. Neurochem. 111, 1490–1500. doi: 10.1111/j.1471-4159.2009.06422.x
Domingos, A. I., Sordillo, A., Dietrich, M. O., Liu, Z. W., Tellez, L. A., Vaynshteyn, J., et al. (2013). Hypothalamic melanin concentrating hormone neurons communicate the nutrient value of sugar. Elife 2:e01462. doi: 10.7554/eLife.01462
Drazen, D. L., Coolen, L. M., Strader, A. D., Wortman, M. D., Woods, S. C., and Seeley, R. J. (2004). Differential effects of adrenalectomy on melanin-concentrating hormone and orexin A. Endocrinology 145, 3404–3412. doi: 10.1210/en.2003-1760
Duncan, E. A., Proulx, K., and Woods, S. C. (2005). Central administration of melanin-concentrating hormone increases alcohol and sucrose/quinine intake in rats. Alcohol. Clin. Exp. Res. 29, 958–964. doi: 10.1097/01.ALC.0000167741.42353.10
Duncan, E. A., Rider, T. R., Jandacek, R. J., Clegg, D. J., Benoit, S. C., Tso, P., et al. (2006). The regulation of alcohol intake by melanin-concentrating hormone in rats. Pharmacol. Biochem. Behav. 85, 728–735. doi: 10.1016/j.pbb.2006.11.004
Duncan, E. A., Sorrell, J. E., Adamantidis, A., Rider, T., Jandacek, R. J., Seeley, R. J., et al. (2007). Alcohol drinking in MCH receptor-1-deficient mice. Alcoholism 31, 1325–1337. doi: 10.1111/j.1530-0277.2007.00427.x
Easton, A., Douchamps, V., Eacott, M., and Lever, C. (2012). A specific role for septohippocampal acetylcholine in memory? Neuropsychologia 50, 3156–3168. doi: 10.1016/j.neuropsychologia.2012.07.022
Elias, C. F., and Bittencourt, J. C. (1997). Study of the origins of melanin-concentrating hormone and neuropeptide EI immunoreactive projections to the periaqueductal gray matter. Brain Res. 755, 255–271. doi: 10.1016/S0006-8993(97)00104-2
Elias, C. F., Lee, C. E., Kelly, J. F., Ahima, R. S., Kuhar, M., Saper, C. B., et al. (2001). Characterization of CART neurons in the rat and human hypothalamus. J. Comp. Neurol. 432, 1–19. doi: 10.1002/cne.1085
Elias, C. F., Saper, C. B., Maratos-Flier, E., Tritos, N. A., Lee, C., Kelly, J., et al. (1998). Chemically defined projections linking the mediobasal hypothalamus and the lateral hypothalamic area. J. Comp. Neurol. 402, 442–459. doi: 10.1002/(SICI)1096-9861(19981228)402:4<442::AID-CNE2>3.0.CO;2-R
Elias, C. F., Sita, L. V., Zambon, B. K., Oliveira, E. R., Vasconcelos, L. A., and Bittencourt, J. C. (2008). Melanin-concentrating hormone projections to areas involved in somatomotor responses. J. Chem. Neuroanat. 35, 188–201. doi: 10.1016/j.jchemneu.2007.10.002
Fekete, C., Mihaly, E., Luo, L. G., Kelly, J., Clausen, J. T., Mao, Q., et al. (2000). Association of cocaine- and amphetamine-regulated transcript-immunoreactive elements with thyrotropin-releasing hormone-synthesizing neurons in the hypothalamic paraventricular nucleus and its role in the regulation of the hypothalamic-pituitary-thyroid axis during fasting. J. Neurosci. 20, 9224–9234.
Ferrario, C. R., Labouèbe, G., Liu, S., Nieh, E. H., Routh, V. H., Xu, S., et al. (2016). Homeostasis meets motivation in the battle to control food intake. J. Neurosci. 36, 11469–11481. doi: 10.1523/JNEUROSCI.2338-16.2016
Foo, H., and Mason, P. (2005). Sensory suppression during feeding. Proc. Natl. Acad. Sci. U.S.A. 102, 16865–16869. doi: 10.1073/pnas.0506226102
Fort, P., Salvert, D., Hanriot, L., Jego, S., Shimizu, H., Hashimoto, K., et al. (2008). The satiety molecule nesfatin-1 is co-expressed with melanin concentrating hormone in tuberal hypothalamic neurons of the rat. Neuroscience 155, 174–181. doi: 10.1016/j.neuroscience.2008.05.035
Francis, K., and Baker, B. I. (1995). Developmental changes in melanin-concentrating hormone in Rana temporaria. Gen. Comp. Endocrinol. 98, 157–165. doi: 10.1006/gcen.1995.1056
Freedman, M. R., Horwitz, B. A., and Stern, J. S. (1986). Effect of adrenalectomy and glucocorticoid replacement on development of obesity. Am. J. Physiol. 250, R595–R607.
Frenk, H., and Rogers, G. H. (1979). The suppressant effects of naloxone on food and water intake in the rat. Behav. Neural Biol. 26, 23–40. doi: 10.1016/S0163-1047(79)92855-3
Fu, L.-Y., Acuna-Goycolea, C., and Van Den Pol, A. N. (2004). Neuropeptide Y inhibits hypocretin/orexin neurons by multiple presynaptic and postsynaptic mechanisms: tonic depression of the hypothalamic arousal system. J. Neurosci. 24, 8741–8751. doi: 10.1523/JNEUROSCI.2268-04.2004
Furudono, Y., Ando, C., Yamamoto, C., Kobashi, M., and Yamamoto, T. (2006). Involvement of specific orexigenic neuropeptides in sweetener-induced overconsumption in rats. Behav. Brain Res. 175, 241–248. doi: 10.1016/j.bbr.2006.08.031
Gallardo, M. G., Chiocchio, S. R., and Tramezzani, J. H. (2004). Changes of melanin-concentrating hormone related to LHRH release in the median eminence of rats. Brain Res. 1030, 152–158. doi: 10.1016/j.brainres.2004.10.005
Gao, X. B., and van den Pol, A. N. (2001). Melanin concentrating hormone depresses synaptic activity of glutamate and GABA neurons from rat lateral hypothalamus. J. Physiol. 533, 237–252. doi: 10.1111/j.1469-7793.2001.0237b.x
Gavrila, A., Chan, J. L., Miller, L. C., Heist, K., Yiannakouris, N., and Mantzoros, C. S. (2005). Circulating melanin-concentrating hormone, agouti-related protein, and alpha-melanocyte-stimulating hormone levels in relation to body composition: alterations in response to food deprivation and recombinant human leptin administration. J. Clin. Endocrinol. Metab. 90, 1047–1054. doi: 10.1210/jc.2004-1124
Georgescu, D., Sears, R. M., Hommel, J. D., Barrot, M., Bolanos, C. A., Marsh, D. J., et al. (2005). The hypothalamic neuropeptide melanin-concentrating hormone acts in the nucleus accumbens to modulate feeding behavior and forced-swim performance. J. Neurosci. 25, 2933–2940. doi: 10.1523/JNEUROSCI.1714-04.2005
Glick, M., Segal-Lieberman, G., Cohen, R., and Kronfeld-Schor, N. (2009). Chronic MCH infusion causes a decrease in energy expenditure and body temperature, and an increase in serum IGF-1 levels in mice. Endocrine 36, 479–485. doi: 10.1007/s12020-009-9252-5
Goforth, P. B., Leinninger, G. M., Patterson, C. M., Satin, L. S., and Myers, M. G. (2014). Leptin acts via lateral hypothalamic area neurotensin neurons to inhibit orexin neurons by multiple GABA-independent mechanisms. J. Neurosci. 34, 11405–11415. doi: 10.1523/JNEUROSCI.5167-13.2014
Gomori, A., Ishihara, A., Ito, M., Mashiko, S., Matsushita, H., Yumoto, M., et al. (2003). Chronic intracerebroventricular infusion of MCH causes obesity in mice. Melanin-concentrating hormone. Am. J. Physiol. Endocrinol. Metab. 284, E583–E588. doi: 10.1152/ajpendo.00350.2002
Gonzalez, M. I., Baker, B. I., and Wilson, C. A. (1997). Stimulatory effect of melanin-concentrating hormone on luteinising hormone release. Neuroendocrinology 66, 254–262. doi: 10.1159/000127246
Green, P. K., Wilkinson, C. W., and Woods, S. C. (1992). Intraventricular corticosterone increases the rate of body weight gain in underweight adrenalectomized rats. Endocrinology 130, 269–275. doi: 10.1210/endo.130.1.1727703
Gröneveld, D., Balm, P., and Wendelaar Bonga, S. (1995). Identification, cellular localization and in vitro release of a novel teleost melanin-concentrating hormone gene-related peptide. Neuroendocrinology 62, 498–505. doi: 10.1159/000127040
Guesdon, B., Paradis, E., Samson, P., and Richard, D. (2009). Effects of intracerebroventricular and intra-accumbens melanin-concentrating hormone agonism on food intake and energy expenditure. Am. J. Physiol. Regul. Integr. Comp. Physiol. 296, R469–R475. doi: 10.1152/ajpregu.90556.2008
Haemmerle, C. A., Campos, A. M., and Bittencourt, J. C. (2015). Melanin-concentrating hormone inputs to the nucleus accumbens originate from distinct hypothalamic sources and are apposed to GABAergic and cholinergic cells in the Long-Evans rat brain. Neuroscience 289, 392–405. doi: 10.1016/j.neuroscience.2015.01.014
Hahn, J. D. (2010). Comparison of melanin-concentrating hormone and hypocretin/orexin peptide expression patterns in a current parceling scheme of the lateral hypothalamic zone. Neurosci. Lett. 468, 12–17. doi: 10.1016/j.neulet.2009.10.047
Hahn, J. D., and Swanson, L. W. (2015). Connections of the juxtaventromedial region of the lateral hypothalamic area in the male rat. Front. Syst. Neurosci. 9:66. doi: 10.3389/fnsys.2015.00066
Halaas, J. L., Gajiwala, K. S., Maffei, M., and Cohen, S. L. (1995). Weight-reducing effects of the plasma protein encoded by the obese gene. Science 269, 543. doi: 10.1126/science.7624777
Hancock, M. B. (1976). Cells of origin of hypothalamo-spinal projections in the rat. Neurosci. Lett. 3, 179–184. doi: 10.1016/0304-3940(76)90070-7
Hausen, A. C., Ruud, J., Jiang, H., Hess, S., Varbanov, H., Kloppenburg, P., et al. (2016). Insulin-dependent activation of MCH neurons impairs locomotor activity and insulin sensitivity in obesity. Cell Rep. 17, 2512–2521. doi: 10.1016/j.celrep.2016.11.030
Hervieu, G., and Nahon, J. L. (1995). Pro-melanin concentrating hormone messenger ribonucleic acid and peptides expression in peripheral tissues of the rat. Neuroendocrinology 61, 348–364. doi: 10.1159/000126857
Hervieu, G. J., Cluderay, J. E., Harrison, D., Meakin, J., Maycox, P., Nasir, S., et al. (2000). The distribution of the mRNA and protein products of the melanin-concentrating hormone (MCH) receptor gene, slc-1, in the central nervous system of the rat. Eur. J. Neurosci. 12, 1194–1216. doi: 10.1046/j.1460-9568.2000.00008.x
Hill, J., Duckworth, M., Murdock, P., Rennie, G., Sabido-David, C., Ames, R. S., et al. (2001). Molecular cloning and functional characterization of MCH2, a novel human MCH receptor. J. Biol. Chem. 276, 20125–20129. doi: 10.1074/jbc.M102068200
Hogben, L., and Slome, D. (1935). The Pigmentary Effector System. VIII–The Dual Receptive Mechanism of the Amphibian Background Response. London: The Royal Society.
Högberg, T., Frimurer, T. M., and Sasmal, P. K. (2012). Melanin concentrating hormone receptor 1 (MCHR1) antagonists–Still a viable approach for obesity treatment? Bioorg. Med. Chem. Lett. 22, 6039–6047. doi: 10.1016/j.bmcl.2012.08.025
Holtzman, S. G. (1974). Behavioral effects of separate and combined administration of naloxone and d-amphetamine. J. Pharmacol. Exp. Ther. 189, 51–60.
Horjales-Araujo, E., Hellysaz, A., and Broberger, C. (2014). Lateral hypothalamic thyrotropin-releasing hormone neurons: distribution and relationship to histochemically defined cell populations in the rat. Neuroscience 277, 87–102. doi: 10.1016/j.neuroscience.2014.06.043
Hosoya, Y., and Matsushita, M. (1979). Identification and distribution of the spinal and hypophyseal projection neurons in the paraventricular nucleus of the rat. A light and electron microscopic study with the horseradish peroxidase method. Exp. Brain Res. 35, 315–331. doi: 10.1007/BF00236618
Huang, H., Acuna-Goycolea, C., Li, Y., Cheng, H., Obrietan, K., and Van Den Pol, A. N. (2007). Cannabinoids excite hypothalamic melanin-concentrating hormone but inhibit hypocretin/orexin neurons: implications for cannabinoid actions on food intake and cognitive arousal. J. Neurosci. 27, 4870–4881. doi: 10.1523/JNEUROSCI.0732-07.2007
Huang, H., and van den Pol, A. N. (2007). Rapid direct excitation and long-lasting enhancement of NMDA response by group I metabotropic glutamate receptor activation of hypothalamic melanin-concentrating hormone neurons. J. Neurosci. 27, 11560–11572. doi: 10.1523/JNEUROSCI.2147-07.2007
Hubschle, T., Mckinley, M. J., and Oldfield, B. J. (1998). Efferent connections of the lamina terminalis, the preoptic area and the insular cortex to submandibular and sublingual gland of the rat traced with pseudorabies virus. Brain Res. 806, 219–231. doi: 10.1016/S0006-8993(98)00765-3
Huesa, G., Van Den Pol, A. N., and Finger, T. E. (2005). Differential distribution of hypocretin (orexin) and melanin-concentrating hormone in the goldfish brain. J. Comp. Neurol. 488, 476–491. doi: 10.1002/cne.20610
Jacobowitz, D. M., and O’Donohue, T. L. (1978). alpha-Melanocyte stimulating hormone: immunohistochemical identification and mapping in neurons of rat brain. Proc. Natl. Acad. Sci. U.S.A. 75, 6300–6304. doi: 10.1073/pnas.75.12.6300
Jaworski, J. N., Kozel, M. A., Philpot, K. B., and Kuhar, M. J. (2003). Intra-accumbal injection of CART (cocaine-amphetamine regulated transcript) peptide reduces cocaine-induced locomotor activity. J. Pharmacol. Exp. Ther. 307, 1038–1044. doi: 10.1124/jpet.103.052332
Johnson, S. W., and North, R. A. (1992). Two types of neurone in the rat ventral tegmental area and their synaptic inputs. J. Physiol. 450, 455. doi: 10.1113/jphysiol.1992.sp019136
Júnior, E. D. D. S., Da Silva, A. V., Da Silva, K. R., Haemmerle, C. A., Batagello, D. S., Da Silva, J. M., et al. (2015). The centrally projecting edinger–westphal nucleus–I: efferents in the rat brain. J. Chem. Neuroanat. 68, 22–38. doi: 10.1016/j.jchemneu.2015.07.002
Kampe, J., Tschop, M. H., Hollis, J. H., and Oldfield, B. J. (2009). An anatomic basis for the communication of hypothalamic, cortical and mesolimbic circuitry in the regulation of energy balance. Eur. J. Neurosci. 30, 415–430. doi: 10.1111/j.1460-9568.2009.06818.x
Karlsson, C., Aziz, A. M. A., Rehman, F., Pitcairn, C., Barchiesi, R., Barbier, E., et al. (2016). Melanin-concentrating hormone and Its MCH-1 receptor: relationship between effects on alcohol and caloric intake. Alcoholism 40, 2199–2207. doi: 10.1111/acer.13181
Karlsson, C., Zook, M., Ciccocioppo, R., Gehlert, D. R., Thorsell, A., Heilig, M., et al. (2012). Melanin-concentrating hormone receptor 1 (MCH1-R) antagonism: reduced appetite for calories and suppression of addictive-like behaviors. Pharmacol. Biochem. Behav. 102, 400–406. doi: 10.1016/j.pbb.2012.06.010
Kawauchi, H., and Baker, B. I. (2004). Melanin-concentrating hormone signaling systems in fish. Peptides 25, 1577–1584. doi: 10.1016/j.peptides.2004.03.025
Kawauchi, H., Kawazoe, I., Tsubokawa, M., Kishida, M., and Baker, B. I. (1983). Characterization of melanin-concentrating hormone in chum salmon pituitaries. Nature 305, 321–323. doi: 10.1038/305321a0
Kelley, A. E., Baldo, B. A., Pratt, W. E., and Will, M. J. (2005). Corticostriatal-hypothalamic circuitry and food motivation: integration of energy, action and reward. Physiol. Behav. 86, 773–795. doi: 10.1016/j.physbeh.2005.08.066
Kennedy, A. R., Todd, J. F., Stanley, S. A., Abbott, C. R., Small, C. J., Ghatei, M. A., et al. (2001). Melanin-concentrating hormone (MCH) suppresses thyroid stimulating hormone (TSH) release, in vivo and in vitro, via the hypothalamus and the pituitary. Endocrinology 142, 3265–3268. doi: 10.1210/endo.142.7.8374
Khorooshi, R. M., and Klingenspor, M. (2005). Neuronal distribution of melanin-concentrating hormone, cocaine- and amphetamine-regulated transcript and orexin B in the brain of the Djungarian hamster (Phodopus sungorus). J. Chem. Neuroanat. 29, 137–148. doi: 10.1016/j.jchemneu.2004.10.003
Kim, J.-H., Creekmore, E., and Vezina, P. (2003). Microinjection of CART peptide 55–102 into the nucleus accumbens blocks amphetamine-induced locomotion. Neuropeptides 37, 369–373. doi: 10.1016/j.npep.2003.10.001
Knollema, S., Brown, E. R., Vale, W., and Sawchenko, P. E. (1992). Novel hypothalamic and preoptic sites of prepro-melanin-concentrating hormone messenger ribonucleic Acid and Peptide expression in lactating rats. J. Neuroendocrinol. 4, 709–717. doi: 10.1111/j.1365-2826.1992.tb00222.x
Kohler, C., Haglund, L., and Swanson, L. W. (1984). A diffuse alpha MSH-immunoreactive projection to the hippocampus and spinal cord from individual neurons in the lateral hypothalamic area and zona incerta. J. Comp. Neurol. 223, 501–514. doi: 10.1002/cne.902230404
Kong, D., Vong, L., Parton, L. E., Ye, C., Tong, Q., Hu, X., et al. (2010). Glucose stimulation of hypothalamic MCH neurons involves K ATP channels, is modulated by UCP2, and regulates peripheral glucose homeostasis. Cell Metab. 12, 545–552. doi: 10.1016/j.cmet.2010.09.013
Korotkova, T. M., Sergeeva, O. A., Eriksson, K. S., Haas, H. L., and Brown, R. E. (2003). Excitation of ventral tegmental area dopaminergic and nondopaminergic neurons by orexins/hypocretins. J. Neurosci. 23, 7–11.
Koylu, E. O., Couceyro, P. R., Lambert, P. D., and Kuhar, M. J. (1998). Cocaine- and amphetamine-regulated transcript peptide immunohistochemical localization in the rat brain. J. Comp. Neurol. 391, 115–132. doi: 10.1002/(SICI)1096-9861(19980202)391:1<115::AID-CNE10>3.0.CO;2-X
Koylu, E. O., Couceyro, P. R., Lambert, P. D., Ling, N. C., Desouza, E. B., and Kuhar, M. J. (1997). Immunohistochemical localization of novel CART peptides in rat hypothalamus, pituitary and adrenal gland. J. Neuroendocrinol. 9, 823–833. doi: 10.1046/j.1365-2826.1997.00651.x
Kozicz, T., Bittencourt, J. C., May, P. J., Reiner, A., Gamlin, P. D., Palkovits, M., et al. (2011). The Edinger-Westphal nucleus: a historical, structural, and functional perspective on a dichotomous terminology. J. Comp. Neurol. 519, 1413–1434. doi: 10.1002/cne.22580
Kozicz, T., Yanaihara, H., and Arimura, A. (1998). Distribution of urocortin-like immunoreactivity in the central nervous system of the rat. J. Comp. Neurol. 391, 1–10. doi: 10.1002/(SICI)1096-9861(19980202)391:1<1::AID-CNE1>3.0.CO;2-6
Krause, M., German, P. W., Taha, S. A., and Fields, H. L. (2010). A pause in nucleus accumbens neuron firing is required to initiate and maintain feeding. J. Neurosci. 30, 4746–4756. doi: 10.1523/JNEUROSCI.0197-10.2010
Kristensen, P., Judge, M. E., Thim, L., Ribel, U., Christjansen, K. N., Wulff, B. S., et al. (1998). Hypothalamic CART is a new anorectic peptide regulated by leptin. Nature 393, 72–76. doi: 10.1038/29993
Lagos, P., Monti, J. M., Jantos, H., and Torterolo, P. (2012). Microinjection of the melanin-concentrating hormone into the lateral basal forebrain increases REM sleep and reduces wakefulness in the rat. Life Sci. 90, 895–899. doi: 10.1016/j.lfs.2012.04.019
Lagos, P., Torterolo, P., Jantos, H., Chase, M. H., and Monti, J. M. (2009). Effects on sleep of melanin-concentrating hormone (MCH) microinjections into the dorsal raphe nucleus. Brain Res. 1265, 103–110. doi: 10.1016/j.brainres.2009.02.010
Lagos, P., Torterolo, P., Jantos, H., and Monti, J. M. (2011). Immunoneutralization of melanin-concentrating hormone (MCH) in the dorsal raphe nucleus: effects on sleep and wakefulness. Brain Res. 1369, 112–118. doi: 10.1016/j.brainres.2010.11.027
Lázár, G., Maderdrut, J. L., and Merchenthaler, I. (2002). Distribution of melanin-concentrating hormone-like immunoreactivity in the central nervous system of Rana esculenta. Brain Res. Bull. 57, 401–407. doi: 10.1016/S0361-9230(01)00675-X
Lechan, R. M., and Fekete, C. (2006). The TRH neuron: a hypothalamic integrator of energy metabolism. Prog. Brain Res. 153, 209–235. doi: 10.1016/S0079-6123(06)53012-2
Leffert, J. D., Newgard, C. B., Okamoto, H., Milburn, J. L., and Luskey, K. L. (1989). Rat amylin: cloning and tissue-specific expression in pancreatic islets. Proc. Natl. Acad. Sci. U.S.A. 86, 3127–3130. doi: 10.1073/pnas.86.9.3127
Legendre, A., Papakonstantinou, E., Roy, M.-C., Richard, D., and Harris, R. B. (2007). Differences in response to corticotropin-releasing factor after short-and long-term consumption of a high-fat diet. Am. J. Physiol. Regul. Integr. Comp. Physiol. 293, R1076–R1085. doi: 10.1152/ajpregu.00592.2006
Legradi, G., Emerson, C. H., Ahima, R. S., Rand, W. M., Flier, J. S., and Lechan, R. M. (1998). Arcuate nucleus ablation prevents fasting-induced suppression of ProTRH mRNA in the hypothalamic paraventricular nucleus. Neuroendocrinology 68, 89–97. doi: 10.1159/000054354
Legradi, G., and Lechan, R. M. (1998). The arcuate nucleus is the major source for neuropeptide Y-innervation of thyrotropin-releasing hormone neurons in the hypothalamic paraventricular nucleus. Endocrinology 139, 3262–3270. doi: 10.1210/endo.139.7.6113
Legradi, G., and Lechan, R. M. (1999). Agouti-related protein containing nerve terminals innervate thyrotropin-releasing hormone neurons in the hypothalamic paraventricular nucleus. Endocrinology 140, 3643–3652. doi: 10.1210/endo.140.8.6935
Leibowitz, S. F., and Alexander, J. T. (1998). Hypothalamic serotonin in control of eating behavior, meal size, and body weight. Biol. Psychiatry 44, 851–864. doi: 10.1016/S0006-3223(98)00186-3
Leibowitz, S. F., and Brown, L. L. (1980). Histochemical and pharmacological analysis of catecholaminergic projections to the perifornical hypothalamus in relation to feeding inhibition. Brain Res. 201, 315–345. doi: 10.1016/0006-8993(80)91038-0
Leinninger, G. M., Jo, Y.-H., Leshan, R. L., Louis, G. W., Yang, H., Barrera, J. G., et al. (2009). Leptin acts via leptin receptor-expressing lateral hypothalamic neurons to modulate the mesolimbic dopamine system and suppress feeding. Cell Metab. 10, 89–98. doi: 10.1016/j.cmet.2009.06.011
Leinninger, G. M., Opland, D. M., Jo, Y.-H., Faouzi, M., Christensen, L., Cappellucci, L. A., et al. (2011). Leptin action via neurotensin neurons controls orexin, the mesolimbic dopamine system and energy balance. Cell Metab. 14, 313–323. doi: 10.1016/j.cmet.2011.06.016
Leloup, C., Arluison, M., Lepetit, N., Cartier, N., Marfaing-Jallat, P., Ferré, P., et al. (1994). Glucose transporter 2 (GLUT 2): expression in specific brain nuclei. Brain Res. 638, 221–226. doi: 10.1016/0006-8993(94)90653-X
Lewis, K., Li, C., Perrin, M., Blount, A., Kunitake, K., Donaldson, C., et al. (2001). Identification of urocortin III, an additional member of the corticotropin-releasing factor (CRF) family with high affinity for the CRF2 receptor. Proc. Natl. Acad. Sci. U.S.A. 98, 7570–7575. doi: 10.1073/pnas.121165198
Li, Y., and van den Pol, A. N. (2006). Differential target-dependent actions of coexpressed inhibitory dynorphin and excitatory hypocretin/orexin neuropeptides. J. Neurosci. 26, 13037–13047. doi: 10.1523/JNEUROSCI.3380-06.2006
Lima, F. F., Sita, L. V., Oliveira, A. R., Costa, H. C., Da Silva, J. M., Mortara, R. A., et al. (2013). Hypothalamic melanin-concentrating hormone projections to the septo-hippocampal complex in the rat. J. Chem. Neuroanat. 47, 1–14. doi: 10.1016/j.jchemneu.2012.10.003
Liu, H., Kishi, T., Roseberry, A. G., Cai, X., Lee, C. E., Montez, J. M., et al. (2003). Transgenic mice expressing green fluorescent protein under the control of the melanocortin-4 receptor promoter. J. Neurosci. 23, 7143–7154.
Louis, G. W., Leinninger, G. M., Rhodes, C. J., and Myers, M. G. Jr. (2010). Direct innervation and modulation of orexin neurons by lateral hypothalamic LepRb neurons. J. Neurosci. 30, 11278–11287. doi: 10.1523/JNEUROSCI.1340-10.2010
Ludwig, D. S., Mountjoy, K. G., Tatro, J. B., Gillette, J. A., Frederich, R. C., Flier, J. S., et al. (1998). Melanin-concentrating hormone: a functional melanocortin antagonist in the hypothalamus. Am. J. Physiol. 274, E627–E633.
Ludwig, D. S., Tritos, N. A., Mastaitis, J. W., Kulkarni, R., Kokkotou, E., Elmquist, J., et al. (2001). Melanin-concentrating hormone overexpression in transgenic mice leads to obesity and insulin resistance. J. Clin. Invest. 107, 379–386. doi: 10.1172/JCI10660
Lutz, T., Mollet, A., Rushing, P., Riediger, T., and Scharrer, E. (2001). The anorectic effect of a chronic peripheral infusion of amylin is abolished in area postrema/nucleus of the solitary tract (AP/NTS) lesioned rats. Int. J. Obes. 25, 1005. doi: 10.1038/sj.ijo.0801664
Mahler, S. V., Moorman, D. E., Smith, R. J., James, M. H., and Aston-Jones, G. (2014). Motivational activation: a unifying hypothesis of orexin/hypocretin function. Nat. Neurosci. 17, 1298–1303. doi: 10.1038/nn.3810
Mancera, J. M., and Fernandez-Llebrez, P. (1995). Development of melanin-concentrating hormone-immunoreactive elements in the brain of gilthead seabream (Sparus aurata). Cell Tissue Res. 282, 523–526. doi: 10.1007/BF00318885
Maolood, N., and Meister, B. (2010). Nociceptin/orphanin FQ peptide in hypothalamic neurones associated with the control of feeding behaviour. J. Neuroendocrinol. 22, 75–82. doi: 10.1111/j.1365-2826.2009.01946.x
Marsh, D. J., Weingarth, D. T., Novi, D. E., Chen, H. Y., Trumbauer, M. E., Chen, A. S., et al. (2002). Melanin-concentrating hormone 1 receptor-deficient mice are lean, hyperactive, and hyperphagic and have altered metabolism. Proc. Natl. Acad. Sci. U.S.A. 99, 3240–3245. doi: 10.1073/pnas.052706899
Matsuda, K., Kojima, K., Shimakura, S.-I., Miura, T., Uchiyama, M., Shioda, S., et al. (2009). Relationship between melanin-concentrating hormone-and neuropeptide Y-containing neurons in the goldfish hypothalamus. Comp. Biochem. Physiol. A Mol. Integr. Physiol. 153, 3–7. doi: 10.1016/j.cbpa.2008.10.002
Mayer, D., Price, D., Lewis, V., and Gebhart, G. (1978). Insulin receptors are widely distributed in the central nervous system of the rat. Nature 272, 27.
Méndez-Andino, J. L., and Wos, J. A. (2007). MCH-R1 antagonists: what is keeping most research programs away from the clinic? Drug Discov. Today 12, 972–979. doi: 10.1016/j.drudis.2007.08.010
Mercer, J. G., Hoggard, N., Williams, L. M., Lawrence, C. B., Hannah, L. T., Morgan, P. J., et al. (1996). Coexpression of leptin receptor and preproneuropeptide Y mRNA in arcuate nucleus of mouse hypothalamus. J. Neuroendocrinol. 8, 733–735. doi: 10.1046/j.1365-2826.1996.05161.x
Messina, M. M., Boersma, G., Overton, J. M., and Eckel, L. A. (2006). Estradiol decreases the orexigenic effect of melanin-concentrating hormone in ovariectomized rats. Physiol. Behav. 88, 523–528. doi: 10.1016/j.physbeh.2006.05.002
Mietlicki-Baase, E. G., Olivos, D. R., Jeffrey, B. A., and Hayes, M. R. (2015). Cooperative interaction between leptin and amylin signaling in the ventral tegmental area for the control of food intake. Am. J. Physiol. Endocrinol. Metab. 308, E1116–E1122. doi: 10.1152/ajpendo.00087.2015
Mietlicki-Baase, E. G., Rupprecht, L. E., Olivos, D. R., Zimmer, D. J., Alter, M. D., Pierce, R. C., et al. (2013). Amylin receptor signaling in the ventral tegmental area is physiologically relevant for the control of food intake. Neuropsychopharmacology 38, 1685–1697. doi: 10.1038/npp.2013.66
Miller, C. L., Hruby, V. J., Matsunaga, T. O., and Bickford, P. C. (1993). Alpha-MSH and MCH are functional antagonists in a CNS auditory gating paradigm. Peptides 14, 431–440. doi: 10.1016/0196-9781(93)90128-4
Minth, C. D., Qiu, H., Akil, H., Watson, S. J., and Dixon, J. E. (1989). Two precursors of melanin-concentrating hormone: DNA sequence analysis and in situ immunochemical localization. Proc. Natl. Acad. Sci. U.S.A. 86, 4292–4296. doi: 10.1073/pnas.86.11.4292
Mizuno, N. (1991). Electron microscopic identification of axons containing melanin-concentrating hormone in the lamprey, Lampetra fluviatilis L. Neurosci. Lett. 128, 249–252. doi: 10.1016/0304-3940(91)90272-U
Mizusawa, K., Amiya, N., Yamaguchi, Y., Takabe, S., Amano, M., Breves, J. P., et al. (2012). Identification of mRNAs coding for mammalian-type melanin-concentrating hormone and its receptors in the scalloped hammerhead shark Sphyrna lewini. Gen. Comp. Endocrinol. 179, 78–87. doi: 10.1016/j.ygcen.2012.07.023
Modirrousta, M., Mainville, L., and Jones, B. E. (2005). Orexin and MCH neurons express c-Fos differently after sleep deprivation vs. recovery and bear different adrenergic receptors. Eur. J. Neurosci. 21, 2807–2816. doi: 10.1111/j.1460-9568.2005.04104.x
Moffett, M. C., Song, J., and Kuhar, M. J. (2011). CART peptide inhibits locomotor activity induced by simultaneous stimulation of D1 and D2 receptors, but not by stimulation of individual dopamine receptors. Synapse 65, 1–7. doi: 10.1002/syn.20815
Momiyama, T., Todo, N., and Sasa, M. (1993). A mechanism underlying dopamine D1 and D2 receptor-mediated inhibition of dopaminergic neurones in the ventral tegmental area in vitro. Br. J. Pharmacol. 109, 933–940. doi: 10.1111/j.1476-5381.1993.tb13710.x
Monti, J. M., Lagos, P., Jantos, H., and Torterolo, P. (2015). Increased REM sleep after intra-locus coeruleus nucleus microinjection of melanin-concentrating hormone (MCH) in the rat. Prog. Neuropsychopharmacol. Biol. Psychiatry 56, 185–188. doi: 10.1016/j.pnpbp.2014.09.003
Monzon, M. E., De Souza, M. M., Izquierdo, L. A., Izquierdo, I., Barros, D. M., and De Barioglio, S. R. (1999). Melanin-concentrating hormone (MCH) modifies memory retention in rats. Peptides 20, 1517–1519. doi: 10.1016/S0196-9781(99)00164-3
Moore, R., and Bloom, F. (1979). Central catecholamine neuron systems: anatomy and physiology of the norepinephrine and epinephrine systems. Annu. Rev. Neurosci. 2, 113–168. doi: 10.1146/annurev.ne.02.030179.000553
Morganstern, I., Chang, G.-Q., Chen, Y.-W., Barson, J., Zhiyu, Y., Hoebel, B., et al. (2010a). Role of melanin-concentrating hormone in the control of ethanol consumption: region-specific effects revealed by expression and injection studies. Physiol. Behav. 101, 428–437. doi: 10.1016/j.physbeh.2010.07.009
Morganstern, I., Chang, G.-Q., Karatayev, O., and Leibowitz, S. F. (2010b). Increased orexin and melanin-concentrating hormone expression in the perifornical lateral hypothalamus of rats prone to overconsuming a fat-rich diet. Pharmacol. Biochem. Behav. 96, 413–422. doi: 10.1016/j.pbb.2010.06.013
Mul, J. D., La Fleur, S. E., Toonen, P. W., Afrasiab-Middelman, A., Binnekade, R., Schetters, D., et al. (2011). Chronic loss of melanin-concentrating hormone affects motivational aspects of feeding in the rat. PLoS ONE 6:e19600. doi: 10.1371/journal.pone.0019600
Muroya, S., Yada, T., Shioda, S., and Takigawa, M. (1999). Glucose-sensitive neurons in the rat arcuate nucleus contain neuropeptide Y. Neurosci. Lett. 264, 113–116. doi: 10.1016/S0304-3940(99)00185-8
Murray, J. F., Adan, R. A., Walker, R., Baker, B. I., Thody, A. J., Nijenhuis, W. A., et al. (2000a). Melanin-concentrating hormone, melanocortin receptors and regulation of luteinizing hormone release. J. Neuroendocrinol. 12, 217–223. doi: 10.1046/j.1365-2826.2000.00440.x
Murray, J. F., Baker, B. I., Levy, A., and Wilson, C. A. (2000b). The influence of gonadal steroids on pre-pro melanin-concentrating hormone mRNA in female rats. J. Neuroendocrinol. 12, 53–59.
Murray, J. F., Mercer, J. G., Adan, R. A., Datta, J. J., Aldairy, C., Moar, K. M., et al. (2000c). The effect of leptin on luteinizing hormone release is exerted in the zona incerta and mediated by melanin-concentrating hormone. J. Neuroendocrinol. 12, 1133–1139.
Murray, J. F., Hahn, J., Kennedy, A., Small, C., Bloom, S., Haskell-Luevano, C., et al. (2006). Evidence for a stimulatory action of melanin-concentrating hormone on luteinising hormone release involving MCH1 and melanocortin-5 receptors. J. Neuroendocrinol. 18, 157–167. doi: 10.1111/j.1365-2826.2005.01397.x
Muschamp, J. W., and Hull, E. M. (2007). Melanin concentrating hormone and estrogen receptor-α are coexstensive but not coexpressed in cells of male rat hypothalamus. Neurosci. Lett. 427, 123–126. doi: 10.1016/j.neulet.2007.09.031
Myers, M. G. Jr., and Olson, D. P. (2012). Central nervous system control of metabolism. Nature 491, 357–363. doi: 10.1038/nature11705
Nahon, J. L., Presse, F., Bittencourt, J. C., Sawchenko, P. E., and Vale, W. (1989). The rat melanin-concentrating hormone messenger ribonucleic acid encodes multiple putative neuropeptides coexpressed in the dorsolateral hypothalamus. Endocrinology 125, 2056–2065. doi: 10.1210/endo-125-4-2056
Naito, N., Nakai, Y., Kawauchi, H., and Hayashi, Y. (1985). Immunocytochemical identification of melanin-concentrating hormone in the brain and pituitary gland of the teleost fishes Oncorhynchus keta and Salmo gairdneri. Cell Tissue Res. 242, 41–48. doi: 10.1007/BF00225561
Nakamura, S., Tsumori, T., Yokota, S., Oka, T., and Yasui, Y. (2009). Amygdaloid axons innervate melanin-concentrating hormone-and orexin-containing neurons in the mouse lateral hypothalamus. Brain Res. 1278, 66–74. doi: 10.1016/j.brainres.2009.04.049
Naufahu, J., Alzaid, F., Brito, M. F., Doslikova, B., Valencia, T. M., Cunliffe, A., et al. (2017). Melanin concentrating hormone in peripheral circulation in the human. J. Endocrinol. 232, 513–523. doi: 10.1530/JOE-16-0240
Naufahu, J., Cunliffe, A. D., and Murray, J. F. (2013). The roles of melanin-concentrating hormone in energy balance and reproductive function: are they connected? Reproduction 146, R141–R150. doi: 10.1530/REP-12-0385
Niswender, K. D., Morrison, C. D., Clegg, D. J., Olson, R., Baskin, D. G., Myers, M. G., et al. (2003). Insulin activation of phosphatidylinositol 3-kinase in the hypothalamic arcuate nucleus: a key mediator of insulin-induced anorexia. Diabetes Metab. Res. Rev. 52, 227–231. doi: 10.2337/diabetes.52.2.227
O’Connor, E. C., Kremer, Y., Lefort, S., Harada, M., Pascoli, V., Rohner, C., et al. (2015). Accumbal D1R neurons projecting to lateral hypothalamus authorize feeding. Neuron 88, 553–564. doi: 10.1016/j.neuron.2015.09.038
Oldfield, B. J., Giles, M. E., Watson, A., Anderson, C., Colvill, L. M., and Mckinley, M. J. (2002). The neurochemical characterisation of hypothalamic pathways projecting polysynaptically to brown adipose tissue in the rat. Neuroscience 110, 515–526. doi: 10.1016/S0306-4522(01)00555-3
Ollmann, M. M., Wilson, B. D., Yang, Y. K., Kerns, J. A., Chen, Y., Gantz, I., et al. (1997). Antagonism of central melanocortin receptors in vitro and in vivo by agouti-related protein. Science 278, 135–138. doi: 10.1126/science.278.5335.135
Ono, M., Wada, C., Oikawa, I., Kawazoe, I., and Kawauchi, H. (1988). Structures of two kinds of mRNA encoding the chum salmon melanin-concentrating hormone. Gene 71, 433–438. doi: 10.1016/0378-1119(88)90060-1
Ono, T., Nishino, H., Sasaka, K., Muramoto, K., Yano, I., and Simpson, A. (1978). Paraventricular nucleus connections to spinal cord and pituitary. Neurosci. Lett. 10, 141–146. doi: 10.1016/0304-3940(78)90025-3
Oswald, K. D., Murdaugh, D. L., King, V. L., and Boggiano, M. M. (2011). Motivation for palatable food despite consequences in an animal model of binge eating. Int. J. Eat. Disord. 44, 203–211. doi: 10.1002/eat.20808
Pachoud, B., Adamantidis, A., Ravassard, P., Luppi, P. H., Grisar, T., Lakaye, B., et al. (2010). Major impairments of glutamatergic transmission and long-term synaptic plasticity in the hippocampus of mice lacking the melanin-concentrating hormone receptor-1. J. Neurophysiol. 104, 1417–1425. doi: 10.1152/jn.01052.2009
Palkovits, M., Brownstein, M., Saavedra, J. M., and Axelrod, J. (1974). Norepinephrine and dopamine content of hypothalamic nuclei of the rat. Brain Res. 77, 137–149. doi: 10.1016/0006-8993(74)90810-5
Parkes, D. G., and Vale, W. W. (1993). Contrasting actions of melanin-concentrating hormone and neuropeptide-E-I on posterior pituitary function. Ann. N. Y. Acad. Sci. 680, 588–590. doi: 10.1111/j.1749-6632.1993.tb19746.x
Parks, G. S., Wang, L., Wang, Z., and Civelli, O. (2014). Identification of neuropeptide receptors expressed by melanin-concentrating hormone neurons. J. Comp. Neurol. 522, 3817–3833. doi: 10.1002/cne.23642
Parsons, M. P., and Hirasawa, M. (2011). GIRK channel-mediated inhibition of melanin-concentrating hormone neurons by nociceptin/orphanin FQ. J. Neurophysiol. 105, 1179–1184. doi: 10.1152/jn.00791.2010
Parton, L. E., Ye, C. P., Coppari, R., Enriori, P. J., Choi, B., Zhang, C.-Y., et al. (2007). Glucose sensing by POMC neurons regulates glucose homeostasis and is impaired in obesity. Nature 449, 228–232. doi: 10.1038/nature06098
Pelleymounter, M. A., Cullen, M. J., Baker, M. B., and Hecht, R. (1995). Effects of the obese gene product on body weight regulation in ob/ob mice. Science 269, 540. doi: 10.1126/science.7624776
Pereira-da-Silva, M., Torsoni, M. A., Nourani, H. V., Augusto, V. D., Souza, C. T., Gasparetti, A. L., et al. (2003). Hypothalamic melanin-concentrating hormone is induced by cold exposure and participates in the control of energy expenditure in rats. Endocrinology 144, 4831–4840. doi: 10.1210/en.2003-0243
Pérez, C. A., Stanley, S. A., Wysocki, R. W., Havranova, J., Ahrens-Nicklas, R., Onyimba, F., et al. (2011). Molecular annotation of integrative feeding neural circuits. Cell Metab. 13, 222–232. doi: 10.1016/j.cmet.2010.12.013
Peyron, C., Tighe, D. K., Van Den Pol, A. N., De Lecea, L., Heller, H. C., Sutcliffe, J. G., et al. (1998). Neurons containing hypocretin (orexin) project to multiple neuronal systems. J. Neurosci. 18, 9996–10015.
Pissios, P., Frank, L., Kennedy, A. R., Porter, D. R., Marino, F. E., Liu, F. F., et al. (2008). Dysregulation of the mesolimbic dopamine system and reward in MCH-/- mice. Biol. Psychiatry 64, 184–191. doi: 10.1016/j.biopsych.2007.12.011
Powell, K., and Baker, B. (1988). Structural studies of nerve terminals containing melanin-concentrating hormone in the eel, Anguilla anguilla. Cell Tissue Res. 251, 433–439. doi: 10.1007/BF00215852
Qu, D., Ludwig, D. S., Gammeltoft, S., Piper, M., Pelleymounter, M. A., Cullen, M. J., et al. (1996). A role for melanin-concentrating hormone in the central regulation of feeding behaviour. Nature 380, 243–247. doi: 10.1038/380243a0
Reiner, D. J., Mietlicki-Baase, E. G., Olivos, D. R., Mcgrath, L. E., Zimmer, D. J., Koch-Laskowski, K., et al. (2017). Amylin acts in the lateral dorsal tegmental nucleus to regulate energy balance through GABA signaling. Biol. Psychiatry (in press). doi: 10.1016/j.biopsych.2016.12.028
Reyes, T., Lewis, K., Perrin, M., Kunitake, K., Vaughan, J., Arias, C., et al. (2001). Urocortin II: a member of the corticotropin-releasing factor (CRF) neuropeptide family that is selectively bound by type 2 CRF receptors. Proc. Natl. Acad. Sci. U.S.A. 98, 2843–2848. doi: 10.1073/pnas.051626398
Rondini, T. A., Donato, J. Jr., Rodrigues Bde, C., Bittencourt, J. C., and Elias, C. F. (2010). Chemical identity and connections of medial preoptic area neurons expressing melanin-concentrating hormone during lactation. J. Chem. Neuroanat. 39, 51–62. doi: 10.1016/j.jchemneu.2009.10.005
Rondini, T. A., Rodrigues Bde, C., De Oliveira, A. P., Bittencourt, J. C., and Elias, C. F. (2007). Melanin-concentrating hormone is expressed in the laterodorsal tegmental nucleus only in female rats. Brain Res. Bull. 74, 21–28. doi: 10.1016/j.brainresbull.2007.04.006
Rossi, M., Choi, S. J., O’shea, D., Miyoshi, T., Ghatei, M. A., and Bloom, S. R. (1997). Melanin-concentrating hormone acutely stimulates feeding, but chronic administration has no effect on body weight. Endocrinology 138, 351–355. doi: 10.1210/endo.138.1.4887
Routh, V. H. (2002). Glucose-sensing neurons: are they physiologically relevant? Physiol. Behav. 76, 403–413. doi: 10.1016/s0031-9384(02)00761-8
Rushing, P. A. (2003). Central amylin signaling and the regulation of energy homeostasis. Curr. Pharm. Des. 9, 819–825. doi: 10.2174/1381612033455387
Saito, Y., Cheng, M., Leslie, F. M., and Civelli, O. (2001). Expression of the melanin-concentrating hormone (MCH) receptor mRNA in the rat brain. J. Comp. Neurol. 435, 26–40. doi: 10.1002/cne.1191
Saito, Y., Nothacker, H. P., Wang, Z., Lin, S. H., Leslie, F., and Civelli, O. (1999). Molecular characterization of the melanin-concentrating-hormone receptor. Nature 400, 265–269. doi: 10.1038/22321
Sakamaki, R., Uemoto, M., Inui, A., Asakawa, A., Ueno, N., Ishibashi, C., et al. (2005). Melanin-concentrating hormone enhances sucrose intake. Int. J. Mol. Med. 15, 1033–1039. doi: 10.3892/ijmm.15.6.1033
Sakurai, T. (2014). The role of orexin in motivated behaviours. Nat. Rev. Neurosci. 15, 719–731. doi: 10.1038/nrn3837
Sakurai, T., Amemiya, A., Ishii, M., Matsuzaki, I., Chemelli, R. M., Tanaka, H., et al. (1998). Orexins and orexin receptors: a family of hypothalamic neuropeptides and G protein-coupled receptors that regulate feeding behavior. Cell 92, 573–585. doi: 10.1016/S0092-8674(00)80949-6
Sano, H., and Yokoi, M. (2007). Striatal medium spiny neurons terminate in a distinct region in the lateral hypothalamic area and do not directly innervate orexin/hypocretin- or melanin-concentrating hormone-containing neurons. J. Neurosci. 27, 6948–6955. doi: 10.1523/JNEUROSCI.0514-07.2007
Santollo, J., and Eckel, L. A. (2008). The orexigenic effect of melanin-concentrating hormone (MCH) is influenced by sex and stage of the estrous cycle. Physiol. Behav. 93, 842–850. doi: 10.1016/j.physbeh.2007.11.050
Santollo, J., and Eckel, L. A. (2013). Oestradiol decreases melanin-concentrating hormone (MCH) and MCH receptor expression in the hypothalamus of female rats. J. Neuroendocrinol. 25, 570–579. doi: 10.1111/jne.12032
Saper, C. B., Akil, H., and Watson, S. J. (1986). Lateral hypothalamic innervation of the cerebral cortex: immunoreactive staining for a peptide resembling but immunochemically distinct from pituitary/arcuate alpha-melanocyte stimulating hormone. Brain Res. Bull. 16, 107–120. doi: 10.1016/0361-9230(86)90018-3
Saper, C. B., Chou, T. C., and Elmquist, J. K. (2002). The need to feed: homeostatic and hedonic control of eating. Neuron 36, 199–211. doi: 10.1016/S0896-6273(02)00969-8
Saper, C. B., Chou, T. C., and Scammell, T. E. (2001). The sleep switch: hypothalamic control of sleep and wakefulness. Trends Neurosci. 24, 726–731. doi: 10.1016/S0166-2236(00)02002-6
Saper, C. B., Loewy, A. D., Swanson, L. W., and Cowan, W. M. (1976). Direct hypothalamo-autonomic connections. Brain Res. 117, 305–312. doi: 10.1016/0006-8993(76)90738-1
Saper, C. B., Scammell, T. E., and Lu, J. (2005). Hypothalamic regulation of sleep and circadian rhythms. Nature 437, 1257–1263. doi: 10.1038/nature04284
Schwartz, M. W., Seeley, R. J., Campfield, L. A., Burn, P., and Baskin, D. G. (1996). Identification of targets of leptin action in rat hypothalamus. J. Clin. Invest. 98, 1101–1106. doi: 10.1172/JCI118891
Scoville, W. B., and Milner, B. (1957). Loss of recent memory after bilateral hippocampal lesions. J. Neurol. Neurosurg. Psychiatry 20, 11–21. doi: 10.1136/jnnp.20.1.11
Segal-Lieberman, G., Bradley, R. L., Kokkotou, E., Carlson, M., Trombly, D. J., Wang, X., et al. (2003). Melanin-concentrating hormone is a critical mediator of the leptin-deficient phenotype. Proc. Natl. Acad. Sci. U.S.A. 100, 10085–10090. doi: 10.1073/pnas.1633636100
Sexton, P., Paxinos, G., Kenney, M., Wookey, P., and Beaumont, K. (1994). In vitro autoradiographic localization of amylin binding sites in rat brain. Neuroscience 62, 553–567. doi: 10.1016/0306-4522(94)90388-3
Shimada, M., Tritos, N. A., Lowell, B. B., Flier, J. S., and Maratos-Flier, E. (1998). Mice lacking melanin-concentrating hormone are hypophagic and lean. Nature 396, 670–674. doi: 10.1038/25341
Shutter, J. R., Graham, M., Kinsey, A. C., Scully, S., Luthy, R., and Stark, K. L. (1997). Hypothalamic expression of ART, a novel gene related to agouti, is up-regulated in obese and diabetic mutant mice. Genes Dev. 11, 593–602. doi: 10.1101/gad.11.5.593
Simmons, D. M., and Swanson, L. W. (2008). High-resolution paraventricular nucleus serial section model constructed within a traditional rat brain atlas. Neurosci. Lett. 438, 85–89. doi: 10.1016/j.neulet.2008.04.057
Simmons, D. M., and Swanson, L. W. (2009). Comparison of the spatial distribution of seven types of neuroendocrine neurons in the rat paraventricular nucleus: toward a global 3D model. J. Comp. Neurol. 516, 423–441. doi: 10.1002/cne.22126
Sita, L. V., Diniz, G. B., Canteras, N. S., Xavier, G. F., and Bittencourt, J. C. (2016). Effect of intrahippocampal administration of anti-melanin-concentrating hormone on spatial food-seeking behavior in rats. Peptides 76, 130–138. doi: 10.1016/j.peptides.2015.12.007
Sita, L. V., Elias, C. F., and Bittencourt, J. C. (2003). Dopamine and melanin-concentrating hormone neurons are distinct populations in the rat rostromedial zona incerta. Brain Res. 970, 232–237. doi: 10.1016/S0006-8993(03)02345-X
Sita, L. V., Elias, C. F., and Bittencourt, J. C. (2007). Connectivity pattern suggests that incerto-hypothalamic area belongs to the medial hypothalamic system. Neuroscience 148, 949–969. doi: 10.1016/j.neuroscience.2007.07.010
Skrapits, K., Kanti, V., Savanyú, Z., Maurnyi, C., Szenci, O., Horváth, A., et al. (2015). Lateral hypothalamic orexin and melanin-concentrating hormone neurons provide direct input to gonadotropin-releasing hormone neurons in the human. Front. Cell. Neurosci. 9:348. doi: 10.3389/fncel.2015.00348
Smith, D. G., Tzavara, E. T., Shaw, J., Luecke, S., Wade, M., Davis, R., et al. (2005). Mesolimbic dopamine super-sensitivity in melanin-concentrating hormone-1 receptor-deficient mice. J. Neurosci. 25, 914–922. doi: 10.1523/JNEUROSCI.4079-04.2005
Spina, M., Merlo-Pich, E., Chan, R. K., Basso, A. M., Rivier, J., Vale, W., et al. (1996). Appetite-suppressing effects of urocortin, a CRF-related neuropeptide. Science 273, 1561–1564. doi: 10.1126/science.273.5281.1561
Squire, L. R. (1992). Memory and the hippocampus: a synthesis from findings with rats, monkeys, and humans. Psychol. Rev. 99, 195.
Steinbusch, H. W. M. (1981). Distribution of serotonin-immunoreactivity in the central nervous system of the rat—cell bodies and terminals. Neuroscience 6, 557–618. doi: 10.1016/0306-4522(81)90146-9
Stricker-Krongrad, A., Dimitrov, T., and Beck, B. (2001). Central and peripheral dysregulation of melanin-concentrating hormone in obese Zucker rats. Mol. Brain Res. 92, 43–48. doi: 10.1016/S0169-328X(01)00130-9
Sun, G., Tian, Z., Murata, T., Narita, K., Honda, K., and Higuchi, T. (2004). Central and peripheral immunoreactivity of melanin-concentrating hormone in hypothalamic obese and lactating rats. J. Neuroendocrinol. 16, 79–83. doi: 10.1111/j.1365-2826.2004.01124.x
Sun, L. L., Zhang, Y., Liu, J. F., Wang, J., Zhu, W. L., Zhao, L. Y., et al. (2013). Role of melanin-concentrating hormone in the nucleus accumbens shell in rats behaviourally sensitized to methamphetamine. Int. J. Neuropsychopharmacol. 16, 1767–1780. doi: 10.1017/S1461145713000072
Suzuki, H., and Yamamoto, T. (2013). Orexin-B-like immunoreactivity localizes in both luteinizing-hormone-containing cells and melanin-concentrating hormone-containing fibers in the red-bellied piranha (Pygocentrus nattereri) pituitary. Cell Tissue Res. 351, 175–182. doi: 10.1007/s00441-012-1516-3
Swanson, L. W. (2005). Anatomy of the soul as reflected in the cerebral hemispheres: neural circuits underlying voluntary control of basic motivated behaviors. J. Comp. Neurol. 493, 122–131. doi: 10.1002/cne.20733
Swanson, L. W., and Kuypers, H. G. (1980). The paraventricular nucleus of the hypothalamus: cytoarchitectonic subdivisions and organization of projections to the pituitary, dorsal vagal complex, and spinal cord as demonstrated by retrograde fluorescence double-labeling methods. J. Comp. Neurol. 194, 555–570. doi: 10.1002/cne.901940306
Swanson, L. W., Sanchez-Watts, G., and Watts, A. G. (2005). Comparison of melanin-concentrating hormone and hypocretin/orexin mRNA expression patterns in a new parceling scheme of the lateral hypothalamic zone. Neurosci. Lett. 387, 80–84. doi: 10.1016/j.neulet.2005.06.066
Swanson, L. W., and Sawchenko, P. E. (1983). Hypothalamic integration: organization of the paraventricular and supraoptic nuclei. Annu. Rev. Neurosci. 6, 269–324. doi: 10.1146/annurev.ne.06.030183.001413
Tataranni, P. A., Larson, D. E., Snitker, S., Young, J. B., Flatt, J., and Ravussin, E. (1996). Effects of glucocorticoids on energy metabolism and food intake in humans. Am. J. Physiol. Endocrinol. Metab. 271, E317–E325.
Thexton, A. J. (1992). Mastication and swallowing: an overview. Br. Dent. J. 173, 197–206. doi: 10.1038/sj.bdj.4808002
Tillet, Y., Batailler, M., and Fellmann, D. (1996). Distribution of melanin-concentrating hormone (MCH)-like immunoreactivity in neurons of the diencephalon of sheep. J. Chem. Neuroanat. 12, 135–145. doi: 10.1016/S0891-0618(96)00195-0
Torterolo, P., Sampogna, S., and Chase, M. H. (2009). MCHergic projections to the nucleus pontis oralis participate in the control of active (REM) sleep. Brain Res. 1268, 76–87. doi: 10.1016/j.brainres.2009.02.055
Torterolo, P., Sampogna, S., Morales, F. R., and Chase, M. H. (2006). MCH-containing neurons in the hypothalamus of the cat: searching for a role in the control of sleep and wakefulness. Brain Res. 1119, 101–114. doi: 10.1016/j.brainres.2006.08.100
Tsukamura, H., Thompson, R. C., Tsukahara, S., Ohkura, S., Maekawa, F., Moriyama, R., et al. (2000). Intracerebroventricular administration of melanin-concentrating hormone suppresses pulsatile luteinizing hormone release in the female rat. J. Neuroendocrinol. 12, 529–534. doi: 10.1046/j.1365-2826.2000.00482.x
Vallarino, M., Andersen, A. C., Delbende, C., Ottonello, I., Eberle, A. N., and Vaudry, H. (1989). Melanin-concentrating hormone (MCH) immunoreactivity in the brain and pituitary of the dogfish Scyliorhinus canicula. Colocalization with alpha-melanocyte-stimulating hormone (alpha-MSH) in hypothalamic neurons. Peptides 10, 375–382. doi: 10.1016/0196-9781(89)90046-6
van den Pol, A. N., Acuna-Goycolea, C., Clark, K. R., and Ghosh, P. K. (2004). Physiological properties of hypothalamic MCH neurons identified with selective expression of reporter gene after recombinant virus infection. Neuron 42, 635–652. doi: 10.1016/S0896-6273(04)00251-X
van Houten, M., Posner, B. I., Kopriwa, B. M., and Brawer, J. R. (1980). Insulin binding sites localized to nerve terminals in rat median eminence and arcuate nucleus. Science 207, 1081–1083. doi: 10.1126/science.6986652
Varas, M., Perez, M., Monzon, M. E., and De Barioglio, S. R. (2002a). Melanin-concentrating hormone, hippocampal nitric oxide levels and memory retention. Peptides 23, 2213–2221.
Varas, M., Perez, M., Ramirez, O., and De Barioglio, S. R. (2002b). Melanin concentrating hormone increase hippocampal synaptic transmission in the rat. Peptides 23, 151–155.
Varas, M. M., Perez, M. F., Ramirez, O. A., and De Barioglio, S. R. (2003). Increased susceptibility to LTP generation and changes in NMDA-NR1 and -NR2B subunits mRNA expression in rat hippocampus after MCH administration. Peptides 24, 1403–1411. doi: 10.1016/j.peptides.2003.09.006
Vaughan, J., Donaldson, C., Bittencourt, J., Perrin, M. H., Lewis, K., Sutton, S., et al. (1995). Urocortin, a mammalian neuropeptide related to fish urotensin I and to corticotropin-releasing factor. Nature 378, 287–292. doi: 10.1038/378287a0
Vaughan, J. M., Fischer, W. H., Hoeger, C., Rivier, J., and Vale, W. (1989). Characterization of melanin-concentrating hormone from rat hypothalamus. Endocrinology 125, 1660–1665. doi: 10.1210/endo-125-3-1660
Verret, L., Goutagny, R., Fort, P., Cagnon, L., Salvert, D., Leger, L., et al. (2003). A role of melanin-concentrating hormone producing neurons in the central regulation of paradoxical sleep. BMC Neurosci. 4:19. doi: 10.1186/1471-2202-4-19
Verty, A. N., Allen, A. M., and Oldfield, B. J. (2010). The endogenous actions of hypothalamic peptides on brown adipose tissue thermogenesis in the rat. Endocrinology 151, 4236–4246. doi: 10.1210/en.2009-1235
Vetrivelan, R., Kong, D., Ferrari, L. L., Arrigoni, E., Madara, J. C., Bandaru, S. S., et al. (2016). Melanin-concentrating hormone neurons specifically promote rapid eye movement sleep in mice. Neuroscience 336, 102–113. doi: 10.1016/j.neuroscience.2016.08.046
Viale, A., Zhixing, Y., Breton, C., Pedeutour, F., Coquerel, A., Jordan, D., et al. (1997). The melanin-concentrating hormone gene in human: flanking region analysis, fine chromosome mapping, and tissue-specific expression. Mol. Brain Res. 46, 243–255. doi: 10.1016/S0169-328X(97)00018-1
Vidal, L., Blanchard, J., and Morin, L. P. (2005). Hypothalamic and zona incerta neurons expressing hypocretin, but not melanin concentrating hormone, project to the hamster intergeniculate leaflet. Neuroscience 134, 1081–1090. doi: 10.1016/j.neuroscience.2005.03.062
Ward, D. R., Dear, F. M., Ward, I. A., Anderson, S. I., Spergel, D. J., Smith, P. A., et al. (2009). Innervation of gonadotropin-releasing hormone neurons by peptidergic neurons conveying circadian or energy balance information in the mouse. PLoS ONE 4:e5322. doi: 10.1371/journal.pone.0005322
Waters, S. M., and Krause, J. E. (2005). Letter re: melanin-concentrating hormone and energy balance. J. Clin. Endocrinol. Metab. 90:6337. doi: 10.1210/jc.2005-1367
Whiddon, B. B., and Palmiter, R. D. (2013). Ablation of neurons expressing melanin-concentrating hormone (MCH) in adult mice improves glucose tolerance independent of MCH signaling. J. Neurosci. 33, 2009–2016. doi: 10.1523/JNEUROSCI.3921-12.2013
Williamson-Hughes, P. S., Grove, K. L., and Smith, M. S. (2005). Melanin concentrating hormone (MCH): a novel neural pathway for regulation of GnRH neurons. Brain Res. 1041, 117–124. doi: 10.1016/j.brainres.2004.11.066
Willie, J. T., Sinton, C. M., Maratos-Flier, E., and Yanagisawa, M. (2008). Abnormal response of melanin-concentrating hormone deficient mice to fasting: hyperactivity and rapid eye movement sleep suppression. Neuroscience 156, 819–829. doi: 10.1016/j.neuroscience.2008.08.048
Woods, S. C., Lotter, E. C., Mckay, L. D., and Porte, D. Jr. (1979). Chronic intracerebroventricular infusion of insulin reduces food intake and body weight of baboons. Nature 282, 503–505. doi: 10.1038/282503a0
Wu, M., Dumalska, I., Morozova, E., Van Den Pol, A., and Alreja, M. (2009). Melanin-concentrating hormone directly inhibits GnRH neurons and blocks kisspeptin activation, linking energy balance to reproduction. Proc. Natl. Acad. Sci. U.S.A. 106, 17217–17222. doi: 10.1073/pnas.0908200106
Xu, L., Bloem, B., Gaszner, B., Roubos, E. W., and Kozicz, T. (2009). Sex-specific effects of fasting on urocortin 1, cocaine- and amphetamine-regulated transcript peptide and nesfatin-1 expression in the rat Edinger-Westphal nucleus. Neuroscience 162, 1141–1149. doi: 10.1016/j.neuroscience.2009.05.003
Yang, S. C., and Shieh, K. R. (2005). Differential effects of melanin concentrating hormone on the central dopaminergic neurons induced by the cocaine- and amphetamine-regulated transcript peptide. J. Neurochem. 92, 637–646. doi: 10.1111/j.1471-4159.2004.02896.x
Yao, Y., Fu, L. Y., Zhang, X., and Van Den Pol, A. N. (2012). Vasopressin and oxytocin excite MCH neurons, but not other lateral hypothalamic GABA neurons. Am. J. Physiol. Regul. Integr. Comp. Physiol. 302, R815–R824. doi: 10.1152/ajpregu.00452.2011
Zakrzewska, K. E., Cusin, I., Stricker-Krongrad, A., Boss, O., Ricquier, D., Jeanrenaud, B., et al. (1999). Induction of obesity and hyperleptinemia by central glucocorticoid infusion in the rat. Diabetes Metab. Res. Rev. 48, 365–370. doi: 10.2337/diabetes.48.2.365
Zhang, X., and van den Pol, A. N. (2012). Thyrotropin-releasing hormone (TRH) inhibits melanin-concentrating hormone neurons: implications for TRH-mediated anorexic and arousal actions. J. Neurosci. 32, 3032–3043. doi: 10.1523/JNEUROSCI.5966-11.2012
Zhang, Y., Proenca, R., Maffei, M., Barone, M., Leopold, L., and Friedman, J. M. (1994). Positional cloning of the mouse obese gene and its human homologue. Nature 372, 425. doi: 10.1038/372425a0
Keywords: MCH, hypothalamus, energy homeostasis, feeding behavior, reward, foraging, metabolism
Citation: Diniz GB and Bittencourt JC (2017) The Melanin-Concentrating Hormone as an Integrative Peptide Driving Motivated Behaviors. Front. Syst. Neurosci. 11:32. doi: 10.3389/fnsys.2017.00032
Received: 22 February 2017; Accepted: 04 May 2017;
Published: 29 May 2017.
Edited by:
Joel D. Hahn, University of Southern California, United StatesReviewed by:
Scott E. Kanoski, University of Southern California, United StatesJessica R. Barson, Drexel University College of Medicine, United States
Copyright © 2017 Diniz and Bittencourt. This is an open-access article distributed under the terms of the Creative Commons Attribution License (CC BY). The use, distribution or reproduction in other forums is permitted, provided the original author(s) or licensor are credited and that the original publication in this journal is cited, in accordance with accepted academic practice. No use, distribution or reproduction is permitted which does not comply with these terms.
*Correspondence: Jackson C. Bittencourt, amNiaXR0ZW5AaWNiLnVzcC5icg==