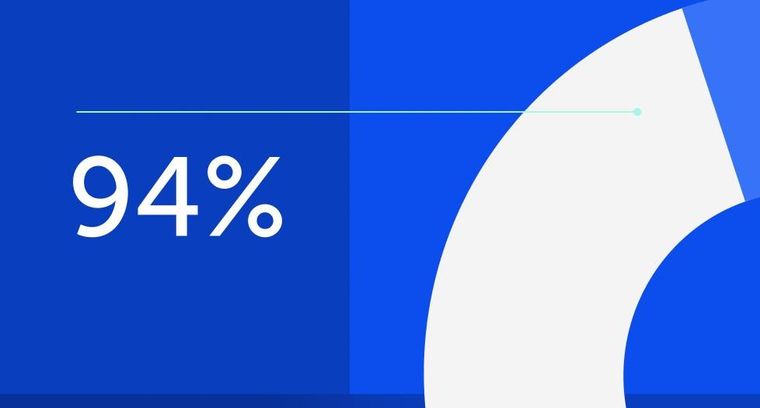
94% of researchers rate our articles as excellent or good
Learn more about the work of our research integrity team to safeguard the quality of each article we publish.
Find out more
REVIEW article
Front. Syst. Neurosci., 14 November 2013
Volume 7 - 2013 | https://doi.org/10.3389/fnsys.2013.00083
This article is part of the Research TopicDistributed networks: new outlooks on cerebellar function.View all 16 articles
The cerebellum has a well-established role in maintaining motor coordination and studies of cerebellar learning suggest that it does this by recognizing neural patterns, which it uses to predict optimal movements. Serious damage to the cerebellum impairs this learning and results in a set of motor disturbances called ataxia. However, recent work implicates the cerebellum in cognition and emotion, and it has been argued that cerebellar dysfunction contributes to non-motor conditions such as autism spectrum disorders (ASD). Based on human and animal model studies, two major questions arise. Does the cerebellum contribute to non-motor as well as motor diseases, and if so, how does altering its function contribute to such diverse symptoms? The architecture and connectivity of cerebellar circuits may hold the answers to these questions. An emerging view is that cerebellar defects can trigger motor and non-motor neurological conditions by globally influencing brain function. Furthermore, during development cerebellar circuits may play a role in wiring events necessary for higher cognitive functions such as social behavior and language. We discuss genetic, electrophysiological, and behavioral evidence that implicates Purkinje cell dysfunction as a major culprit in several diseases and offer a hypothesis as to how canonical cerebellar functions might be at fault in non-motor as well as motor diseases.
The cerebellum is essential for smooth, purposeful movement. Recently, human neuroimaging and animal behavior studies have implicated the cerebellum in the processing of signals for perception, cognition, and emotion (Schmahmann, 2010; Bastian, 2011; D'Angelo and Casali, 2012), particularly in circumstances involving predictions or timing. Participation of the cerebellum in higher order brain function is likely mediated by extensive connections with cortical and sub-cortical centers. These anatomical connections raise the intriguing possibility that cerebellar dysfunction may lead not only to motor impairments, but also to non-motor deficits in complex neurological conditions. Furthermore, the implication that cerebellar circuits malfunction in certain neurodevelopmental disorders suggests that cerebellar processing could be required during development for proper wiring in other brain areas (Kuemerle et al., 2007). We discuss the etiology of cerebellar disease in the context of how circuits are organized, and present evidence that cerebellar connectivity may be altered in ataxia, dystonia, and autism spectrum disorders ASD.
To appreciate how the cerebellum works, it is useful to first recall the major cell types, and revisit the relationships between them (Figure 1). Purkinje cells are the corner stone of all cerebellar circuits; during development they orchestrate morphogenesis, and in the adult each one computes hundreds of thousands of signals (Figure 1B). The elaborate dendrite of each Purkinje cell is directly innervated by a single excitatory climbing fiber that comes from the inferior olive in the brainstem (Figures 1, 2A). Purkinje cells also receive excitatory input indirectly from mossy fibers, which originate from over two-dozen brain and spinal cord nuclei. Approximately 25 million mossy fibers enter the cerebellum and synapse on ~50 billion granule cells (Palkovits et al., 1972; Andersen et al., 1992). Granule cells then converge massively (100,000 to 1) onto the dendrites of Purkinje cells. This striking expansion from mossy fibers to granule cells and equally striking contraction from granule cells onto the Purkinje cell dendrite is believed to provide a computational benefit, namely the ability of the cerebellum to discriminate a large number of different patterns (Marr, 1969; Albus, 1971; Brunel et al., 2004). Various inhibitory interneurons regulate the excitatory inputs onto Purkinje cells (Figure 1), and specialized astrocytes called Bergmann glia maintain efficient synaptic signaling. The Purkinje cells send exclusively inhibitory signals to the cerebellar nuclei, which control the final output of the cerebellum (White and Sillitoe, 2013). An excitatory feedback projection terminating in mossy fiber-like endings exists between the cerebellar nuclei and granule cell layer, and an inhibitory feedback connection is made from the cerebellar nuclei to the inferior olive. These two connections form parts of the nucleo-cortical (Tolbert et al., 1976; Hess, 1982) and olivo-cortico-nuclear loops (Angaut and Sotelo, 1987; Chaumont et al., 2013), respectively. This canonical cerebellar circuit, which was once thought to be simple and synonymous with motor signaling, is now thought to have underlying complexities that also mediate non-motor brain behaviors.
Figure 1. Cytoarchitecture and connectivity in the cerebellum. (A) Mouse brain shown from a lateral view with the cerebellum highlighted in color. (B) The basic cerebellar circuit is comprised of granule cells, Purkinje cells, stellate and basket cell interneurons, and deep nuclei. Afferent information is delivered to the cerebellum as climbing fibers or mossy fibers. The plus and minus signs indicate whether each synapse is excitatory or inhibitory. Note that inhibitory connections between the cerebellar nuclei and inferior olive complete the olivo-cortico-nuclear loop and excitatory projections from the cerebellar nuclei loop back to the cerebellar cortex. Panel (B) was modified from (Reeber et al., 2012). For simplicity we have not shown Golgi cells, unipolar brush cells, Lugaro cells, and candelabrum cells.
Figure 2. Purkinje cells have a distinct morphology and electrophysiological profile. (A) Purkinje cell labeled using the classic Golgi-Cox staining method, demonstrating the exquisite morphology and extensive dendritic branching of the Purkinje cell. (B) Purkinje cells can be identified by their unique activity: each one fires complex spikes that are triggered by climbing fibers (asterisks) and simples spikes that are driven either by intrinsic activity or by mossy fiber-granule cell inputs. (C) Higher power image of the Purkinje cell recording shown in panel (B). Defects in Purkinje cell morphology and/or firing are thought to instigate motor and non-motor neurological conditions.
The classic view that cerebellar function is restricted to controlling motor coordination has been challenged by recent imaging studies in humans that suggest cerebellar contributions to cognition (language), emotional behavior (fear), sleep, and even non-somatic, visceral responses (Demirtas-Tatlidede et al., 2011; Baumann and Mattingley, 2012; D'Angelo and Casali, 2012). Anatomical studies performed in non-human primates and rodents strongly support the imaging data. Extensive mono- and poly-synaptic pathways connect the cerebellum to the cerebral cortex, hippocampus, amygdala, hypothalamus, periaqueductal gray, basal ganglia, thalamus, brain stem, and spinal cord (Dietrichs and Haines, 1989; Middleton and Strick, 2001; Hoshi et al., 2005; Cerminara et al., 2009; Buckner et al., 2011; Dum and Strick, 2012). Considering such widespread connections between the cerebellum and the forebrain, dozens of brainstem nuclei, and with several major autonomic centers (Figure 3), it seems difficult to imagine that cerebellar circuit dysfunction would interfere only with the ability to perform motor tasks. Still, valid arguments against non-motor contributions of the cerebellum have been presented (Glickstein, 2007), and recent data demonstrates that caution should be taken when interpreting for cerebellar non-motor behavior in experimental preparations (Galliano et al., 2013). Keeping in mind that a lively debate continues as to whether the cerebellum is involved in non-motor function (Lemon and Edgley, 2010), in the following sections we present recent evidence that has unveiled unexpected roles for the cerebellum in conditions that are historically “non-cerebellar” (Table 1).
Figure 3. The cerebellum is extensively connected to the brain and spinal cord. (A) Schematic representation of brain regions that send input to the cerebellum. (B) Schematic representation of the regions that receive information from the cerebellum. Note that the TH is a major relay station for cerebellar input to the cortex while the PN is the primary gateway for cerebral cortical input to the cerebellum. Abbreviations: AMG, amygdala; BG, basal ganglia; ECN, external cuneate nucleus; HIP, hippocampus; HYP; hypothalamus; IO, inferior olive; LC, locus coeruleus; PAG, periaqueductal gray; PN, pontine nuclei; RET, reticular nucleus; RN, red nucleus; SC, spinal cord; SUP, superior colliculi; TH, thalamus; VN, vestibular nuclei.
Cerebellar damage causes a number of motor symptoms including dysmetria (in which patients overshoot (hypermetria) or undershoot (hypometria) a target during voluntary goal-directed tasks), hypotonia, tremor, and dysarthic speech. These symptoms, and the interpretations of what they mean to brain function date back to the pioneering neurological examinations of Sir Gordon Holmes (Holmes, 1939). However, descriptions of cerebellar diseases, and in particular those that affect Purkinje cell development and/or function, typically disrupt the accuracy and coordination of movement, conditions which are often cumulatively referred to as “ataxia.”
As a symptom, ataxia refers to uncoordinated movement and as a disorder it refers to a family of neurological diseases that typically involve neurodegeneration. Ataxia-related defects can also be acquired, and develop as a result of stroke, multiple sclerosis, tumors, alcoholism, peripheral neuropathy, metabolic disorders, and vitamin deficiencies (Klockgether, 2010). Ataxia can also arise sporadically (Klockgether, 2010). Patients with ataxia have poor muscle control, and when they have limb movement problems the lack of balance and coordination ultimately disturbs their gait, a symptom often associated with cerebellar defects.
Cerebellar ataxia is the most common form of ataxia. There are over 60 forms of inherited cerebellar-based ataxia, with more than half of them classified as either spinocerebellar ataxias, Friedreich's ataxia, episodic ataxia, or fragile X tremor/ataxia syndrome (Durr, 2010; Klockgether, 2010). Recapitulating disease mechanisms in engineered animal models has allowed major breakthroughs in our understanding of the pathogenesis of the cerebellar ataxias (Burright et al., 1995; Hamilton et al., 1996; Serra et al., 2006). A unifying cellular phenotype observed in the nervous system of ataxic mice and humans, regardless of the type of ataxia, is extensive Purkinje cell degeneration. However, while neuronal degeneration may be essential for the severe pathophysiological features of ataxia (Chen et al., 2008; Liu et al., 2009), electrophysiological and calcium imaging data show that Purkinje cells and their major inputs are dysfunctional prior to degeneration in ataxia mouse models coinciding with milder ataxic phenotypes (Barnes et al., 2011; Hourez et al., 2011; Shakkottai et al., 2011; Kasumu et al., 2012; Hansen et al., 2013). This prompts two considerations regarding disease etiology in any disorder that alters the brain at the level of circuits: (1) neuronal function can be affected in the absence of pathological defects, and (2) neuronal circuit dysfunction may be the primary cause of behavioral symptoms. A case in point is dystonia, where no clear or consistent pathology is evident, yet brain dysfunction can cause overt behaviors that are obstructive to daily life.
Dystonia is a complex movement disorder that causes involuntary, sustained muscle contractions that result in postural twisting and repetitive movements (Hallett, 2009; Shamim et al., 2011). Symptoms can be mild and transient, appearing only under conditions of exertion or fatigue, or severe and constant enough to make even simple day-to-day movements impossible. The involuntary painful muscle contractions can affect virtually any muscle in the body, causing blepharospams in the eyelids [a frequent result of anti-psychotic drugs; (Hallett, 2009)], to the common writer's cramp (Shamim et al., 2011), to inherited torsion dystonia that blocks the normal execution of trunk and limbs movements (Muller, 2009; Shamim et al., 2011). It can be acquired as a hereditary disorder, or spontaneously arise as an idiopathic condition. Although it is considered the third most common motor disease, the true prevalence of dystonia is difficult to estimate because it can be comorbid with other disorders such as Parkinson's disease, Huntington's disease, stroke, or ataxia, and many milder cases do not get reported (Muller, 2009; Asmus and Gasser, 2010). Despite the wide range of its manifestations and causes, dystonia consistently involves erroneous communication along circuits that link the cerebral cortex, basal ganglia, thalamus, and brainstem (Hendrix and Vitek, 2012). Recently, several groups have considerably deepened our understanding of dystonia by confirming that the cerebellum can play a role in the disorder (Argyelan et al., 2009; Calderon et al., 2011; LeDoux, 2011; Table 2). Now, the general consensus in field is that in dystonia, communication is disrupted along two primary pathways: the cerebello-thalamo-striatial (CTS) circuit and cerebello-thalamo-cortical (CTC) circuit (Niethammer et al., 2011). Moreover, a recent elegant study demonstrated using a model of rapid onset dystonia-parkinsonism that defects in either the basal ganglia or the cerebellum could instigate disease onset (Calderon et al., 2011; Table 2).
Functional imaging studies have revealed abnormal cerebellar activity in DYT1 dystonia (Eidelberg et al., 1998), hemi-dystonia (Ceballos-Baumann et al., 1995), exercise-induced paroxysmal dystonia (Kluge et al., 1998), writer's cramp (Odergren et al., 1998; Preibisch et al., 2001), cervical dystonia (Galardi et al., 1996) and blepharospasm (Hutchinson et al., 2000). Consistent with these human data, abnormal cerebellar activity is observed in several genetic models of dystonia, including dystonic (dt) rats, both transgenic and knock-in Dyt1 mice, and spontaneous mutant mice such as tottering (Brown and Lorden, 1989; Campbell and Hess, 1998; Calderon et al., 2011; Ulug et al., 2011; Zhao et al., 2011). In vivo electrophysiology recording in these rodent models reveals that Purkinje cells lose their regular firing patterns (Figures 2B,C) and instead fire in erratic “burst” patterns (LeDoux, 2011). Interestingly, in mice with ataxia it has been suggested that irregular firing of Purkinje cells is the primary alteration that causes motor defects (Walter et al., 2006). Surgical removal of the cerebellum terminates the dystonic attacks in rodents, supporting the notion that the cerebellum can drive dystonia (Neychev et al., 2008; LeDoux, 2011; Neychev et al., 2011). At the cellular level, Ellen Hess and colleagues have pioneered the view that cerebellar Purkinje cells may be the source of dystonia (Campbell et al., 1999). In their initial experiments they removed Purkinje cells by cross-breeding dystonic tottering mice with mutants that exhibit Purkinje cell degeneration (Campbell et al., 1999). Remarkably, doing so alleviated dystonia. In their recent studies, Hess' group showed using an elegant conditional genetic approach that selectively eliminating the Cacna1a calcium channel in Purkinje cells is sufficient to evoke widespread dystonic movements (Raike et al., 2012). Moreover, this conditional approach was further used to show that stress, caffeine, and alcohol can operate through shared mechanisms to trigger severe episodic dystonia attacks (Raike et al., 2013). While Purkinje cell defects induce dystonia, extra-cerebellar synapses may be targeted to block dystonia. Micro-lesions made in the central-lateral thalamus, which connects the cerebellum to the basal ganglia (Ichinohe et al., 2000), alleviated motor defects in mice with rapid-onset dystonia-parkinsonism (Calderon et al., 2011).
On the one hand, genetically altering Purkinje cells has revealed an unexpected requirement for the cerebellum in dystonia, yet on the other hand, it may not be entirely surprising that altering Purkinje cell function would produce complex motor deficits. In fact, one has to wonder whether defective Purkinje cell communication could also, and perhaps simultaneously, influence non-motor behavior. This logic was recently put to the test in experiments that sought to determine whether the cerebellum is linked to ASD.
The ASD's are developmental disorders characterized by impaired social communication, repetitive stereotypic behaviors, and delayed language development (Association, 1994). Individuals with ASD can also display dysfunction in both fine and gross motor skills (Fatemi et al., 2012). Although the signs and symptoms of ASD have become well appreciated, an ongoing debate has been centered on one important question: what regions of the brain are defective in ASD? Not so appreciated is that the cerebellum exhibits consistent neuropathological abnormalities in ASD (Ritvo et al., 1986; Bauman, 1991). In postmortem brain tissue from ASD patients, regardless of age, sex, and cognitive ability, a significant decrease in the number of Purkinje cells is reported (Bauman and Kemper, 2005; Whitney et al., 2008; Fatemi et al., 2012). In addition, functional neuroimaging demonstrates abnormal cerebellar activation in patients with ASD (Allen et al., 2004). Although controversial, in part due to co-morbidity with other developmental deficits, magnetic resonance imaging has also revealed hypoplasia of the cerebellum in some ASD patients (Courchesne et al., 1994; Stanfield et al., 2008; Scott et al., 2009).
Genetic studies also support a role for the cerebellum in ASD (Fatemi et al., 2012). For example, trinucleotide repeat expansions that cause fragile X syndrome by disrupting the function of the gene FMR1 lead to cerebellar vermis abnormalities. In both global and Purkinje cell-specific fragile X knockout mice, Purkinje cell spine morphology, synaptic plasticity, and cerebellar behaviors are impaired. Moreover, in humans, cerebellar learning is deficient as fragile X patients show abnormal eye blink conditioning (Koekkoek et al., 2005; Smit et al., 2008; Tobia and Woodruff-Pak, 2009). There is also a Fragile X associated ataxia/tremor syndrome exhibited by parents of Fragile X patients (Hagerman et al., 2001; Hall and O'Keefe, 2012). This syndrome is linked to “premutation” expansions in the fragile X gene and presents with classic cerebellar deficits of gait ataxia and tremor. Imaging studies show clear atrophy of the cerebellum.
Other genes highly expressed in cerebellum such as EN2, MET, and GABRB3 may also be associated with non-syndromic ASD. Each of these genes exhibits specific roles during cerebellar development. In mice, En2 is required for cell proliferation, tissue patterning, regional morphogenesis, and circuit formation in the cerebellum (White and Sillitoe, 2013). Importantly, two intronic polymorphisms in human EN2 have been reported to be associated with the risk of developing ASD (Gharani et al., 2004; Wang et al., 2008; Banerjee-Basu and Packer, 2010; Sen et al., 2010; Yang et al., 2010). Loss of En2 in mice results in altered aggressiveness and excessive grooming, which are hallmark ASD-like behaviors (Cheh et al., 2006; Brielmaier et al., 2012). Several studies have demonstrated an association between three MET single nucleotide polymorphisms and ASD (Campbell et al., 2008; Hedrick et al., 2012). MET is expressed in proliferating granule cell precursors and disrupting its function results in cerebellar hypoplasia (Ieraci et al., 2002; Fatemi et al., 2012). Positive associations with ASD have also been reported for both common and rare variants of the GABRB3 gene (Banerjee-Basu and Packer, 2010), and GABRB3 expression is reduced in the cerebellum of affected individuals. Importantly, GABRB3 is located within chromosome 15q11–13, a site linked to duplications that are associated with ASD (Fatemi et al., 2012). GABRB3 null mice display hypoplasia of the cerebellar vermis (DeLorey et al., 2008; Fatemi et al., 2009, 2012). Despite the potential links between cerebellar dysfunction and ASD pathogenesis, no clear view has emerged about why the cerebellum might be involved in ASD (genetic and cellular mechanisms?) or how it might be involved (specific brain connections or neural circuits?). However, recent landmark studies strongly support the idea that Purkinje cell dysfunction can result in ASD.
Tuberous sclerosis (TSC1, TSC2) is a rare disorder associated with ASD, and is characterized by benign tumors (harmartomas) that form in the brain, skin, eyes, kidneys, and heart (Curatolo et al., 2008). Interestingly, tuberous sclerosis patients with cerebellar lesions have more severe ASD symptoms than patients with lesions in only other brain regions (Eluvathingal et al., 2006). In a recent paper, Sahin and co-workers showed that loss of Tsc1 from cerebellar Purkinje cells is sufficient to cause social impairments, cognitive defects, abnormal vocalizations, and a number of motor problems (Tsai et al., 2012). The mutant mice also exhibited a reduction in the number of Purkinje cells, and an increase in the expression of endoplasmic reticulum and oxidative stress response markers. The study further showed that Purkinje cell excitability was altered in both heterozygous and homozygous Tsc1 mutants (Tsai et al., 2012) in a very similar manner as has been described in spinocerebellar ataxia models (Hourez et al., 2011; Shakkottai et al., 2011; Kasumu et al., 2012; Hansen et al., 2013). Both the pathology and abnormal ASD-like behaviors were successfully blocked in mutants treated with the mTOR inhibitor rapamycin. Together, these Tsc1 conditional mutants recapitulated several core features of human ASD and using their model the authors demonstrate that pharmacological treatments that target Purkinje cell function can alleviate multiple ASD-associated features (Tsai et al., 2012). In a different study, loss of Tsc2 in Purkinje cells resulted in neurodegeneration, increased repetitive behavior, and social interaction deficits (Reith et al., 2013). Cumulatively, the impressive body of data from these two studies suggests that Purkinje cell dysfunction can cause ASD-like behaviors, and that defects in specific cerebellar circuits might be sufficient to trigger downstream neuronal network alterations that contribute to severe abnormalities in motor and non-motor behaviors.
Although the identification of the Purkinje cell as a major player in motor and non-motor disease has opened new avenues for understanding complex brain disorders (Table 3), one question that immediately arises is how could a single cell type with seemingly unique and specialized functions encode such diverse disease-related information? One can speculate that some of the basic computational capacities of the cerebellum that have been studied with respect to motor behavior, such as the ability to discriminate patterns and the capacity to use these patterns to learn to make context-dependent predictions (Bastian, 2011), are useful to non-motor areas of the brain. For example, it is intriguing to consider whether cerebellar output may be required during a critical period of development so that cortical circuits responsible for complex social behavior and language can be properly wired. This may not be confined to cortical circuitry. Interestingly, Herrup and colleagues observed that loss of En2 resulted in defects in the position of the amygdala, a brain region regularly altered in individuals with ASD (Kuemerle et al., 2007). These findings suggest that a gene (En2) expressed exclusively in the mid/hindbrain region can affect distant cerebral cortex structures such as the amygdala. Herrup and colleagues hypothesized that disrupting the location of neurons relative to their efferent and afferent partners may have detrimental effects on cognition. In this scenario, cerebellar dysfunction during development could result in ASD-like symptoms (Kuemerle et al., 2007). In adults who suffer cerebellar damage, cognitive impairments may result from a loss of cerebellar processing power contributing to tasks involving prediction or complex sensory discrimination (Bastian, 2011). Such hypotheses derive support from the parallel anatomical substrates that connect the cerebellum to motor areas and non-motor areas. Progress on these fascinating questions is likely to emerge from studies of the remarkable patterning of the cerebellum into a complex array of topographic “zonal” circuits that are thought to shape cellular function during behavior (Figure 4).
Figure 4. The cerebellum is highly compartmentalized into functional regions. (A) Schematic illustrating the division of the cerebellum into behaviorally relevant domains. The cartoon is a simplified model of the functional cerebellum and is based on functional imaging, human and animal lesions, afferent connectivity, electrophysiology, and animal behavior studies. (B,C) Wholemount immunohistochemical staining of the mouse cerebellum—zebrin II expression reveals the intricate patterning of the cerebellum into zones. The scale bar in (C) = 2 mm (also applies to (B).
Natural selection has adorned the animal kingdom with a rich collection of exquisite patterns. We revel in admiration of butterfly wing spots, peacock feathers and zebra stripes. Beyond their beauty, these precise patterns are essential for sexual selection and evading predators. In humans, our own body parts such as ribs, vertebrae, and digits develop into patterns that permit the execution of essential day-to-day functions. The human brain, arguably the most complicated structure in nature, is no exception to the hypothesis that patterns are inherent to all forms, regardless of their simplicity or complexity. Much like the developing wings and body segments of an insect, the mammalian brain contains a striking array of patterns. Each of the billions of neurons in the human brain is decorated with a specific pattern of connections that drive brain function. Although many regions of the brain have patterned neural circuits (Reeber et al., 2012), the cerebellum arguably contains the most exquisitely patterned circuits of all central nervous system structures. This high level of patterning may be essential for packaging a large number of functionally distinct circuits into a logical network for seamless communication during behavior.
Cerebellar circuits are patterned into a topographic map of genetically determined “zones” (White and Sillitoe, 2013). Zones are best revealed by molecular expression patterns in Purkinje cells. The most comprehensively studied zonal marker is zebrin II (Brochu et al., 1990) (Figures 4B,C), also known as aldolase C. Zebrin II is expressed by a subset of Purkinje cells (zebrin II +) that alternate with Purkinje cells that do not express zebrin II (zebrin II −), thus forming complementary rows of biochemically distinct Purkinje cells. The zonal organization of zebrin II is symmetrical about the midline, highly reproducible between individuals, and conserved across species (Sillitoe et al., 2005). Molecular tools such as zebrin II expression have been used to show that zonal compartments divide the cerebellar cortex into thousands of reproducible units, with each one containing several hundred Purkinje cells (Apps and Hawkes, 2009). The Purkinje cell zonal plan has a predictable and well-defined relationship to its thousands of incoming afferent projections [Figure 3; (Reeber et al., 2012)]. Moreover, each cerebellar cortical region has a defined and strict relationship to specific cells within the cerebellar nuclei, which send topographic projections out of the cerebellum to unique regions within the brain and spinal cord [(Uusisaari and De Schutter, 2011); Figure 3]. Together, Purkinje cell zones and their associated synapses organize cerebellar function into a spatial map that encodes multiple behaviors (Wadiche and Jahr, 2005; Horn et al., 2010; Cerminara and Apps, 2011). It is intriguing to speculate that this zonal plan may extend beyond cerebellar circuits into connected regions such as the thalamus and cerebral cortex. Indeed, cerebellar efferent projections to the inferior olive and the basal ganglia are highly topographic, and because of bi-directional connectivity projections between these structures forms closed loop circuits (Middleton and Strick, 2000; Bazzigaluppi et al., 2012).
With such a high level of organization it seems plausible that certain circuits, and therefore certain zones, may be more affected by some diseases and not others. That is, could disrupting one set of zones cause ataxia while disrupting an adjacent set cause dystonia? Perhaps. However, given that each zone likely encodes multiple behaviors (Cerminara and Apps, 2011), manipulating the function of any given set would almost certainly result in a “mixed” disease outcome. The phenotypes of several animal models of episodic movement disorders support this idea. For instance, in Cacna1a mutant mice [tottering; (Alvina and Khodakhah, 2010)], loss of the Cav2.1 voltage dependent calcium channel not only causes ataxic episodes, but severe dystonia can also be induced in the same mice. Similarly, in Tsc1, Tsc2, and En2 mouse models of ASD, loss of cerebellar function triggers circuit defects that disrupt both motor and non-motor behaviors. And, En2 mutant mice exhibit severe alterations in Purkinje cell patterning and consequently, at least three functionally distinct classes of afferent fibers are mis-targeted into ectopic positions within the cerebellar cortex (White and Sillitoe, 2013). It is therefore intriguing that Purkinje cell loss may be patterned in dystonia and ASD since the cell loss has been described as “patchy” in both conditions (Carper et al., 2006; Prudente et al., 2013). The burning question that we must now tackle is does zonal function directly control disease related behaviors? The answer may lie within the operational units of the zones, which are referred to as cerebellar modules (Ruigrok, 2011). Each module is comprised of topographically organized afferent fibers, Purkinje cell stripe gene expression (e.g., zebrin II), and the zonally organized Purkinje cell efferent projections to the cerebellar nuclei. Systematic analyses will have to be conducted in existing mutant mouse models in order to determine how each module, or subsets of functionally related modules, operates during the expression of disease-related behaviors. In addition, however, powerful inducible approaches such as channelrhodopsin or CreER genetics should be used to target specific modules (or specific circuits within them) to ask whether defective connectivity in select pathways can recapitulate the disease phenotypes. Such models would be invaluable for therapeutic design and testing. In parallel, further studies in humans should be conducted. Recent likelihood meta-analysis of neuroimaging data demonstrated a precise functional topography in different lobules; subsets of lobules are apparently associated with specific functions (e.g., lobule V = sensorimotor function and lobule VII = cognitive function; (Stoodley and Schmahmann, 2009). Each set of lobules was also linked to specific cerebello-cerebral cortical loops (Stoodley and Schmahmann, 2010), which include connections with somatomotor, premotor and association cortices (Buckner et al., 2011). In general, these functional and anatomic topographies are remarkably reminiscent of the transverse divisions that were delineated by mouse developmental and genetic analyses of zones (Ozol et al., 1999; Sgaier et al., 2007). For more than 60 years zonal cerebellar circuits have been invaluable for understanding basic neuroanatomy, development, cellular function, and behavior. Now, the time is right to apply this wealth of knowledge toward understanding the cellular and molecular mechanisms of neurological disease using animal models and also human pathophysiology. Purkinje cell zones are highly attractive candidates for mediating cerebellar disease (Tolbert et al., 1995; Sarna and Hawkes, 2003). In this context, the expression of calcium associated proteins such as mGluR, EAAT4, and IP3R1 may render certain zones more vulnerable to particular insults (Welsh et al., 2002; Wadiche and Jahr, 2005; Schorge et al., 2010). Although, why particular zones are so susceptible to specific conditions whereas others are resistant, remains an intriguing mystery.
Despite the remarkable strides that have been made in understanding the role of the cerebellum in normal brain behavior and disease, we still do not have a consensus on either issue. We are far from fully understanding what the cerebellum does or what happens if it fails to work properly. Moreover, with only five major neuronal types, one wonders how diverse information from regions ranging from the spinal cord and brainstem to the hypothalamus and basal ganglia converge within functionally coherent circuits in the cerebellum. Even after more than 100 years since the seminal work of Cajal, the cerebellum remains one of the most intriguing structures in the body: despite its simple structure it has the computing power to process more information than any other brain region, and changes to its normal state translate into devastating conditions that apparently reverberate throughout the brains major circuits. While the precise mechanisms that mediate cerebellar (dys)function remain a mystery, powerful new genetic and circuit physiology approaches hold great promise for improving our understanding of this fascinating brain region.
The authors declare that the research was conducted in the absence of any commercial or financial relationships that could be construed as a potential conflict of interest.
Roy V. Sillitoe is supported by the Caroline Wiess Law Fund for Research in Molecular Medicine, a BCM IDDRC Project Development Award, and start-up funds from Baylor College of Medicine and Texas Children's Hospital (Houston, TX). This work was also supported by BCM IDDRC Grant Number 5P30HD024064 from the Eunice Kennedy Shriver National Institute Of Child Health & Human Development. Tom S. Otis is supported by NIH grants NS086429, NS033123 and by the McKnight Foundation.
Albus. (1971). A theory of cerebellar function. Math. Biosci. 10, 25–61. doi: 10.1016/0025-5564(71)90051-4
Allen, G., Muller, R. A., and Courchesne, E. (2004). Cerebellar function in autism: functional magnetic resonance image activation during a simple motor task. Biol. Psychiatry 56, 269–278. doi: 10.1016/j.biopsych.2004.06.005
Alvina, K., and Khodakhah, K. (2010). KCa channels as therapeutic targets in episodic ataxia type-2. J. Neurosci. 30, 7249–7257. doi: 10.1523/JNEUROSCI.6341-09.2010
Andersen, B. B., Korbo, L., and Pakkenberg, B. (1992). A quantitative study of the human cerebellum with unbiased stereological techniques. J. Comp. Neurol. 326, 549–560. doi: 10.1002/cne.903260405
Angaut, P., and Sotelo, C. (1987). The dentato-olivary projection in the rat as a presumptive GABAergic link in the olivo-cerebello-olivary loop. An ultrastructural study. Neurosci. Lett. 83, 227–231. doi: 10.1016/0304-3940(87)90090-5
Apps, R., and Hawkes, R. (2009). Cerebellar cortical organization: a one-map hypothesis. Nat. Rev. Neurosci. 10, 670–681. doi: 10.1038/nrn2698
Argyelan, M., Carbon, M., Niethammer, M., Ulug, A. M., Voss, H. U., Bressman, S. B., et al. (2009). Cerebellothalamocortical connectivity regulates penetrance in dystonia. J. Neurosci. 29, 9740–9747. doi: 10.1523/JNEUROSCI.2300-09.2009
Asmus, F., and Gasser, T. (2010). Dystonia-plus syndromes. Eur. J. Neurol. 17(Suppl. 1), 37–45. doi: 10.1111/j.1468-1331.2010.03049.x
Association, A. P. (1994). Diagnostic and Statistical Manual of Mental Disorders (DSM-4), 4th Edn. Washington, DC: APA.
Banerjee-Basu, S., and Packer, A. (2010). SFARI Gene: an evolving database for the autism research community. Dis. Model. Mech. 3, 133–135. doi: 10.1242/dmm.005439
Barnes, J. A., Ebner, B. A., Duvick, L. A., Gao, W., Chen, G., Orr, H. T., et al. (2011). Abnormalities in the climbing fiber-Purkinje cell circuitry contribute to neuronal dysfunction in ATXN1[82Q] mice. J. Neurosci. 31, 12778–12789. doi: 10.1523/JNEUROSCI.2579-11.2011
Bastian, A. J. (2011). Moving, sensing and learning with cerebellar damage. Curr. Opin. Neurobiol. 21, 596–601. doi: 10.1016/j.conb.2011.06.007
Bauman, M. L., and Kemper, T. L. (2005). Neuroanatomic observations of the brain in autism: a review and future directions. Int. J. Dev. Neurosci. 23, 183–187. doi: 10.1016/j.ijdevneu.2004.09.006
Baumann, O., and Mattingley, J. B. (2012). Functional topography of primary emotion processing in the human cerebellum. Neuroimage 61, 805–811. doi: 10.1016/j.neuroimage.2012.03.044
Bazzigaluppi, P., Ruigrok, T., Saisan, P., De Zeeuw, C. I., and de Jeu, M. (2012). Properties of the nucleo-olivary pathway: an in vivo whole-cell patch clamp study. PloS ONE 7:e46360. doi: 10.1371/journal.pone.0046360
Brielmaier, J., Matteson, P. G., Silverman, J. L., Senerth, J. M., Kelly, S., Genestine, M., et al. (2012). Autism-relevant social abnormalities and cognitive deficits in engrailed-2 knockout mice. PloS ONE 7:e40914. doi: 10.1371/journal.pone.0040914
Brochu, G., Maler, L., and Hawkes, R. (1990). Zebrin II: a polypeptide antigen expressed selectively by Purkinje cells reveals compartments in rat and fish cerebellum. J. Comp. Neurol. 291, 538–552. doi: 10.1002/cne.902910405
Brown, L. L., and Lorden, J. F. (1989). Regional cerebral glucose utilization reveals widespread abnormalities in the motor system of the rat mutant dystonic. J. Neurosci. 9, 4033–4041.
Brunel, N., Hakim, V., Isope, P., Nadal, J. P., and Barbour, B. (2004). Optimal information storage and the distribution of synaptic weights: perceptron versus Purkinje cell. Neuron 43, 745–757.
Buckner, R. L., Krienen, F. M., Castellanos, A., Diaz, J. C., and Yeo, B. T. (2011). The organization of the human cerebellum estimated by intrinsic functional connectivity. J. Neurophysiol. 106, 2322–2345. doi: 10.1152/jn.00339.2011
Burright, E. N., Clark, H. B., Servadio, A., Matilla, T., Feddersen, R. M., Yunis, W. S., et al. (1995). SCA1 transgenic mice: a model for neurodegeneration caused by an expanded CAG trinucleotide repeat. Cell 82, 937–948. doi: 10.1016/0092-8674(95)90273-2
Calderon, D. P., Fremont, R., Kraenzlin, F., and Khodakhah, K. (2011). The neural substrates of rapid-onset Dystonia-Parkinsonism. Nat. Neurosci. 14, 357–365. doi: 10.1038/nn.2753
Campbell, D. B., and Hess, E. J. (1998). Cerebellar circuitry is activated during convulsive episodes in the tottering (tg/tg) mutant mouse. Neuroscience 85, 773–783. doi: 10.1016/S0306-4522(97)00672-6
Campbell, D. B., Li, C., Sutcliffe, J. S., Persico, A. M., and Levitt, P. (2008). Genetic evidence implicating multiple genes in the MET receptor tyrosine kinase pathway in autism spectrum disorder. Autism Res. 1, 159–168. doi: 10.1002/aur.27
Campbell, D. B., North, J. B., and Hess, E. J. (1999). Tottering mouse motor dysfunction is abolished on the Purkinje cell degeneration (pcd) mutant background. Exp. Neurol. 160, 268–278. doi: 10.1006/exnr.1999.7171
Carbon, M., Raymond, D., Ozelius, L., Saunders-Pullman, R., Frucht, S., Dhawan, V., et al. (2013). Metabolic changes in DYT11 myoclonus-dystonia. Neurology 80, 385–391. doi: 10.1212/WNL.0b013e31827f0798
Carper, R. A., Wideman, G. M., and Courchesne, E. (2006). “Understanding autism from basic neuroscience to treatment,” in Structural Neuroimaging, eds Steve, O. Moldin and John, L. R. Rubenstein (Boca Raton, FL: CRC Press Taylor and Francis), 347–377.
Ceballos-Baumann, A. O., Passingham, R. E., Marsden, C. D., and Brooks, D. J. (1995). Motor reorganization in acquired hemidystonia. Ann. Neurol. 37, 746–757. doi: 10.1002/ana.410370608
Cerminara, N. L., and Apps, R. (2011). Behavioural significance of cerebellar modules. Cerebellum 10, 484–494. doi: 10.1007/s12311-010-0209-2
Cerminara, N. L., Koutsikou, S., Lumb, B. M., and Apps, R. (2009). The periaqueductal grey modulates sensory input to the cerebellum: a role in coping behaviour. Eur. J. Neurosci. 29, 2197–2206. doi: 10.1111/j.1460-9568.2009.06760.x
Chaumont, J., Guyon, N., Valera, A. M., Dugue, G. P., Popa, D., Marcaggi, P., et al. (2013). Clusters of cerebellar Purkinje cells control their afferent climbing fiber discharge. Proc. Natl. Acad. Sci. U.S.A. 110, 16223–16228. doi: 10.1073/pnas.1302310110
Cheh, M. A., Millonig, J. H., Roselli, L. M., Ming, X., Jacobsen, E., Kamdar, S., et al. (2006). En2 knockout mice display neurobehavioral and neurochemical alterations relevant to autism spectrum disorder. Brain Res. 1116, 166–176. doi: 10.1016/j.brainres.2006.07.086
Chen, X., Tang, T. S., Tu, H., Nelson, O., Pook, M., Hammer, R., et al. (2008). Deranged calcium signaling and neurodegeneration in spinocerebellar ataxia type 3. J. Neurosci. 28, 12713–12724. doi: 10.1523/JNEUROSCI.3909-08.2008
Courchesne, E., Saitoh, O., Yeung-Courchesne, R., Press, G. A., Lincoln, A. J., Haas, R. H., et al. (1994). Abnormality of cerebellar vermian lobules VI and VII in patients with infantile autism: identification of hypoplastic and hyperplastic subgroups with MR imaging. AJR. Am. J. Roentgenol. 162, 123–130. doi: 10.2214/ajr.162.1.8273650
Curatolo, P., Bombardieri, R., and Jozwiak, S. (2008). Tuberous sclerosis. Lancet 372, 657–668. doi: 10.1016/S0140-6736(08)61279-9
D'Angelo, E., and Casali, S. (2012). Seeking a unified framework for cerebellar function and dysfunction: from circuit operations to cognition. Front. Neural Circuits 6:116. doi: 10.3389/fncir.2012.00116
DeLorey, T. M., Sahbaie, P., Hashemi, E., Homanics, G. E., and Clark, J. D. (2008). Gabrb3 gene deficient mice exhibit impaired social and exploratory behaviors, deficits in non-selective attention and hypoplasia of cerebellar vermal lobules: a potential model of autism spectrum disorder. Behav. Brain Res. 187, 207–220. doi: 10.1016/j.bbr.2007.09.009
Demirtas-Tatlidede, A., Freitas, C., Pascual-Leone, A., and Schmahmann, J. D. (2011). Modulatory effects of theta burst stimulation on cerebellar nonsomatic functions. Cerebellum 10, 495–503. doi: 10.1007/s12311-010-0230-5
Dietrichs, E., and Haines, D. E. (1989). Interconnections between hypothalamus and cerebellum. Anat. Embryol. 179, 207–220. doi: 10.1007/BF00326585
Dum, R. P., and Strick, P. L. (2012). Transneuronal tracing with neurotropic viruses reveals network macroarchitecture. Curr. Opin. Neurobiol. 23, 245–249. doi: 10.1016/j.conb.2012.12.002
Durr, A. (2010). Autosomal dominant cerebellar ataxias: polyglutamine expansions and beyond. Lancet Neurol. 9, 885–894. doi: 10.1016/S1474-4422(10)70183-6
Eidelberg, D., Moeller, J. R., Antonini, A., Kazumata, K., Nakamura, T., Dhawan, V., et al. (1998). Functional brain networks in DYT1 dystonia. Ann. Neurol. 44, 303–312. doi: 10.1002/ana.410440304
Eluvathingal, T. J., Behen, M. E., Chugani, H. T., Janisse, J., Bernardi, B., Chakraborty, P., et al. (2006). Cerebellar lesions in tuberous sclerosis complex: neurobehavioral and neuroimaging correlates. J. Child Neurol. 21, 846–851. doi: 10.1177/08830738060210100301
Fatemi, S. H., Aldinger, K. A., Ashwood, P., Bauman, M. L., Blaha, C. D., Blatt, G. J., et al. (2012). Consensus paper: pathological role of the cerebellum in autism. Cerebellum 11, 777–807. doi: 10.1007/s12311-012-0355-9
Fatemi, S. H., Reutiman, T. J., Folsom, T. D., and Thuras, P. D. (2009). GABA(A) receptor downregulation in brains of subjects with autism. J. Autism Dev. Disord. 39, 223–230. doi: 10.1007/s10803-008-0646-7
Galardi, G., Perani, D., Grassi, F., Bressi, S., Amadio, S., Antoni, M., et al. (1996). Basal ganglia and thalamo-cortical hypermetabolism in patients with spasmodic torticollis. Acta Neurol. Scand. 94, 172–176. doi: 10.1111/j.1600-0404.1996.tb07049.x
Galliano, E., Potters, J. W., Elgersma, Y., Wisden, W., Kushner, S. A., De Zeeuw, C. I., et al. (2013). Synaptic transmission and plasticity at inputs to murine cerebellar Purkinje cells are largely dispensable for standard nonmotor tasks. J. Neurosci. 33, 12599–12618. doi: 10.1523/JNEUROSCI.1642-13.2013
Gharani, N., Benayed, R., Mancuso, V., Brzustowicz, L. M., and Millonig, J. H. (2004). Association of the homeobox transcription factor, ENGRAILED 2 3, with autism spectrum disorder. Mol. Psychiatry 9, 474–484. doi: 10.1038/sj.mp.4001498
Glickstein, M. (2007). What does the cerebellum really do. Curr. Biol. 17, R824–R827. doi: 10.1016/j.cub.2007.08.009
Hagerman, R. J., Leehey, M., Heinrichs, W., Tassone, F., Wilson, R., Hills, J., et al. (2001). Intention tremor, parkinsonism, and generalized brain atrophy in male carriers of fragile X. Neurology 57, 127–130. doi: 10.1212/WNL.57.1.127
Hall, D. A., and O'Keefe, J. A. (2012). Fragile x-associated tremor ataxia syndrome: the expanding clinical picture, pathophysiology, epidemiology, and update on treatment. Tremor Other Hyperkinet Mov. (N.Y.) 2, 1–11. pii: tre-02-56-352-1
Hallett, M. (2009). Dystonia: a sensory and motor disorder of short latency inhibition. Ann. Neurol. 66, 125–127. doi: 10.1002/ana.21762
Hamilton, B. A., Frankel, W. N., Kerrebrock, A. W., Hawkins, T. L., FitzHugh, W., Kusumi, K., et al. (1996). Disruption of the nuclear hormone receptor RORalpha in staggerer mice. Nature 379, 736–739. doi: 10.1038/379736a0
Hansen, S. T., Meera, P., Otis, T. S., and Pulst, S. M. (2013). Changes in Purkinje cell firing and gene expression precede behavioral pathology in a mouse model of SCA2. Hum. Mol. Genet. 22, 271–283. doi: 10.1093/hmg/dds427
Hedrick, A., Lee, Y., Wallace, G. L., Greenstein, D., Clasen, L., Giedd, J. N., et al. (2012). Autism risk gene MET variation and cortical thickness in typically developing children and adolescents. Autism Res. 5, 434–439. doi: 10.1002/aur.1256
Hendrix, C. M., and Vitek, J. L. (2012). Toward a network model of dystonia. Ann. N.Y. Acad. Sci. 1265, 46–55. doi: 10.1111/j.1749-6632.2012.06692.x
Hess, D. T. (1982). Cerebellar nucleo-cortical neurons projecting to the vermis of lobule VII in the rat. Brain Res. 248, 361–366. doi: 10.1016/0006-8993(82)90595-9
Horn, K. M., Pong, M., and Gibson, A. R. (2010). Functional relations of cerebellar modules of the cat. J. Neurosci. 30, 9411–9423. doi: 10.1523/JNEUROSCI.0440-10.2010
Hoshi, E., Tremblay, L., Feger, J., Carras, P. L., and Strick, P. L. (2005). The cerebellum communicates with the basal ganglia. Nat. Neurosci. 8, 1491–1493. doi: 10.1038/nn1544
Hourez, R., Servais, L., Orduz, D., Gall, D., Millard, I., de Kerchove D'Exaerde, A., et al. (2011). Aminopyridines correct early dysfunction and delay neurodegeneration in a mouse model of spinocerebellar ataxia type 1. J. Neurosci. 31, 11795–11807. doi: 10.1523/JNEUROSCI.0905-11.2011
Hutchinson, M., Nakamura, T., Moeller, J. R., Antonini, A., Belakhlef, A., Dhawan, V., et al. (2000). The metabolic topography of essential blepharospasm: a focal dystonia with general implications. Neurology 55, 673–677. doi: 10.1212/WNL.55.5.673
Ichinohe, N., Mori, F., and Shoumura, K. (2000). A di-synaptic projection from the lateral cerebellar nucleus to the laterodorsal part of the striatum via the central lateral nucleus of the thalamus in the rat. Brain Res. 880, 191–197. doi: 10.1016/S0006-8993(00)02744-X
Ieraci, A., Forni, P. E., and Ponzetto, C. (2002). Viable hypomorphic signaling mutant of the Met receptor reveals a role for hepatocyte growth factor in postnatal cerebellar development. Proc. Natl. Acad. Sci. U.S.A. 99, 15200–15205. doi: 10.1073/pnas.222362099
Kasumu, A. W., Liang, X., Egorova, P., Vorontsova, D., and Bezprozvanny, I. (2012). Chronic suppression of inositol 1 4, 5-triphosphate receptor-mediated calcium signaling in cerebellar purkinje cells alleviates pathological phenotype in spinocerebellar ataxia 2 mice. J. Neurosci. 32, 12786–12796. doi: 10.1523/JNEUROSCI.1643-12.2012
Klockgether, T. (2010). Sporadic ataxia with adult onset: classification and diagnostic criteria. Lancet Neurol. 9, 94–104. doi: 10.1016/S1474-4422(09)70305-9
Kluge, A., Kettner, B., Zschenderlein, R., Sandrock, D., Munz, D. L., Hesse, S., et al. (1998). Changes in perfusion pattern using ECD-SPECT indicate frontal lobe and cerebellar involvement in exercise-induced paroxysmal dystonia. Mov. Disord. 13, 125–134. doi: 10.1002/mds.870130124
Koekkoek, S. K., Yamaguchi, K., Milojkovic, B. A., Dortland, B. R., Ruigrok, T. J., Maex, R., et al. (2005). Deletion of FMR1 in Purkinje cells enhances parallel fiber LTD, enlarges spines, and attenuates cerebellar eyelid conditioning in Fragile X syndrome. Neuron 47, 339–352. doi: 10.1016/j.neuron.2005.07.005
Kuemerle, B., Gulden, F., Cherosky, N., Williams, E., and Herrup, K. (2007). The mouse Engrailed genes: a window into autism. Behav. Brain Res. 176, 121–132. doi: 10.1016/j.bbr.2006.09.009
LeDoux, M. S. (2011). Animal models of dystonia: lessons from a mutant rat. Neurobiol. Dis. 42, 152–161. doi: 10.1016/j.nbd.2010.11.006
LeDoux, M. S., Hurst, D. C., and Lorden, J. F. (1998). Single-unit activity of cerebellar nuclear cells in the awake genetically dystonic rat. Neuroscience 86, 533–545. doi: 10.1016/S0306-4522(98)00007-4
LeDoux, M. S., and Lorden, J. F. (2002). Abnormal spontaneous and harmaline-stimulated Purkinje cell activity in the awake genetically dystonic rat. Exp. Brain Res. 145, 457–467. doi: 10.1007/s00221-002-1127-4
LeDoux, M. S., Lorden, J. F., and Ervin, J. M. (1993). Cerebellectomy eliminates the motor syndrome of the genetically dystonic rat. Exp. Neurol. 120, 302–310. doi: 10.1006/exnr.1993.1064
Lemon, R. N., and Edgley, S. A. (2010). Life without a cerebellum. Brain 133, 652–654. doi: 10.1093/brain/awq030
Liu, J., Tang, T. S., Tu, H., Nelson, O., Herndon, E., Huynh, D. P., et al. (2009). Deranged calcium signaling and neurodegeneration in spinocerebellar ataxia type 2. J. Neurosci. 29, 9148–9162. doi: 10.1523/JNEUROSCI.0660-09.2009
Middleton, F. A., and Strick, P. L. (2000). Basal ganglia and cerebellar loops: motor and cognitive circuits. Brain Res. Brain Res. Rev. 31, 236–250. doi: 10.1016/S0165-0173(99)00040-5
Middleton, F. A., and Strick, P. L. (2001). Cerebellar projections to the prefrontal cortex of the primate. J. Neurosci. 21, 700–712.
Neychev, V. K., Fan, X., Mitev, V. I., Hess, E. J., and Jinnah, H. A. (2008). The basal ganglia and cerebellum interact in the expression of dystonic movement. Brain 131, 2499–2509. doi: 10.1093/brain/awn168
Neychev, V. K., Gross, R. E., Lehericy, S., Hess, E. J., and Jinnah, H. A. (2011). The functional neuroanatomy of dystonia. Neurobiol. Dis. 42, 185–201. doi: 10.1016/j.nbd.2011.01.026
Niethammer, M., Carbon, M., Argyelan, M., and Eidelberg, D. (2011). Hereditary dystonia as a neurodevelopmental circuit disorder: evidence from neuroimaging. Neurobiol. Dis. 42, 202–209. doi: 10.1016/j.nbd.2010.10.010
Odergren, T., Stone-Elander, S., and Ingvar, M. (1998). Cerebral and cerebellar activation in correlation to the action-induced dystonia in writer's cramp. Mov. Disord. 13, 497–508. doi: 10.1002/mds.870130321
Ozol, K., Hayden, J. M., Oberdick, J., and Hawkes, R. (1999). Transverse zones in the vermis of the mouse cerebellum. J. Comp. Neurol. 412, 95–111. doi: 10.1002/(SICI)1096-9861(19990913)412:1<95::AID-CNE7>3.3.CO;2-P
Palkovits, M., Magyar, P., and Szentagothai, J. (1972). Quantitative histological analysis of the cerebellar cortex in the cat. IV. Mossy fiber-Purkinje cell numerical transfer. Brain Res. 45, 15–29. doi: 10.1016/0006-8993(72)90213-2
Pizoli, C. E., Jinnah, H. A., Billingsley, M. L., and Hess, E. J. (2002). Abnormal cerebellar signaling induces dystonia in mice. J. Neurosci. 22, 7825–7833.
Preibisch, C., Berg, D., Hofmann, E., Solymosi, L., and Naumann, M. (2001). Cerebral activation patterns in patients with writer's cramp: a functional magnetic resonance imaging study. J. Neurol. 248, 10–17. doi: 10.1007/s004150170263
Prudente, C. N., Pardo, C. A., Xiao, J., Hanfelt, J., Hess, E. J., Ledoux, M. S., et al. (2013). Neuropathology of cervical dystonia. Exp. Neurol. 241, 95–104. doi: 10.1016/j.expneurol.2012.11.019
Raike, R. S., Pizoli, C. E., Weisz, C., van den Maagdenberg, A. M., Jinnah, H. A., and Hess, E. J. (2012). Limited regional cerebellar dysfunction induces focal dystonia in mice. Neurobiol. Dis. 49C, 200–210. doi: 10.1016/j.nbd.2012.07.019
Raike, R. S., Weisz, C., Hoebeek, F. E., Terzi, M. C., De Zeeuw, C. I., van den Maagdenberg, A. M., et al. (2013). Stress, caffeine and ethanol trigger transient neurological dysfunction through shared mechanisms in a mouse calcium channelopathy. Neurobiol. Dis. 50, 151–159. doi: 10.1016/j.nbd.2012.09.005
Reeber, S. L., White, J. J., George-Jones, N. A., and Sillitoe, R. V. (2012). Architecture and development of olivocerebellar circuit topography. Front. Neural Circuits 6:115. doi: 10.3389/fncir.2012.00115
Reith, R. M., McKenna, J., Wu, H., Hashmi, S. S., Cho, S. H., Dash, P. K., et al. (2013). Loss of Tsc2 in Purkinje cells is associated with autistic-like behavior in a mouse model of tuberous sclerosis complex. Neurobiol. Dis. 51, 93–103. doi: 10.1016/j.nbd.2012.10.014
Ritvo, E. R., Freeman, B. J., Scheibel, A. B., Duong, T., Robinson, H., Guthrie, D., et al. (1986). Lower Purkinje cell counts in the cerebella of four autistic subjects: initial findings of the UCLA-NSAC Autopsy Research Report. Am. J. Psychiatry 143, 862–866.
Ruigrok, T. J. (2011). Ins and outs of cerebellar modules. Cerebellum 10, 464–474. doi: 10.1007/s12311-010-0164-y
Sarna, J. R., and Hawkes, R. (2003). Patterned Purkinje cell death in the cerebellum. Prog. Neurobiol. 70, 473–507. doi: 10.1016/S0301-0082(03)00114-X
Schmahmann, J. D. (2010). The role of the cerebellum in cognition and emotion: personal reflections since 1982 on the dysmetria of thought hypothesis, and its historical evolution from theory to therapy. Neuropsychol. Rev. 20, 236–260. doi: 10.1007/s11065-010-9142-x
Schorge, S., van de Leemput, J., Singleton, A., Houlden, H., and Hardy, J. (2010). Human ataxias: a genetic dissection of inositol triphosphate receptor (ITPR1)-dependent signaling. Trends Neurosci. 33, 211–219. doi: 10.1016/j.tins.2010.02.005
Scott, J. A., Schumann, C. M., Goodlin-Jones, B. L., and Amaral, D. G. (2009). A comprehensive volumetric analysis of the cerebellum in children and adolescents with autism spectrum disorder. Autism Res. 2, 246–257. doi: 10.1002/aur.97
Sen, B., Singh, A. S., Sinha, S., Chatterjee, A., Ahmed, S., Ghosh, S., et al. (2010). Family-based studies indicate association of Engrailed 2 gene with autism in an Indian population. Genes Brain Behav. 9, 248–255. doi: 10.1111/j.1601-183X.2009.00556.x
Serra, H. G., Duvick, L., Zu, T., Carlson, K., Stevens, S., Jorgensen, N., et al. (2006). RORalpha-mediated Purkinje cell development determines disease severity in adult SCA1 mice. Cell 127, 697–708. doi: 10.1016/j.cell.2006.09.036
Sgaier, S. K., Lao, Z., Villanueva, M. P., Berenshteyn, F., Stephen, D., Turnbull, R. K., et al. (2007). Genetic subdivision of the tectum and cerebellum into functionally related regions based on differential sensitivity to engrailed proteins. Development 134, 2325–2335. doi: 10.1242/dev.000620
Shakkottai, V. G., do Carmo Costa, M., Dell'Orco, J. M., Sankaranarayanan, A., Wulff, H., and Paulson, H. L. (2011). Early changes in cerebellar physiology accompany motor dysfunction in the polyglutamine disease spinocerebellar ataxia type 3. J. Neurosci. 31, 13002–13014. doi: 10.1523/JNEUROSCI.2789-11.2011
Shamim, E. A., Chu, J., Scheider, L. H., Savitt, J., Jinnah, H. A., and Hallett, M. (2011). Extreme task specificity in writer's cramp. Mov. Disord. 26, 2107–2109. doi: 10.1002/mds.23827
Sillitoe, R. V., Marzban, H., Larouche, M., Zahedi, S., Affanni, J., and Hawkes, R. (2005). Conservation of the architecture of the anterior lobe vermis of the cerebellum across mammalian species. Prog. Brain Res. 148, 283–297. doi: 10.1016/S0079-6123(04)48022-4
Smit, A. E., van der Geest, J. N., Vellema, M., Koekkoek, S. K., Willemsen, R., Govaerts, L. C., et al. (2008). Savings and extinction of conditioned eyeblink responses in fragile X syndrome. Genes Brain Behav. 7, 770–777. doi: 10.1111/j.1601-183X.2008.00417.x
Stanfield, A. C., McIntosh, A. M., Spencer, M. D., Philip, R., Gaur, S., and Lawrie, S. M. (2008). Towards a neuroanatomy of autism: a systematic review and meta-analysis of structural magnetic resonance imaging studies. Eur. Psychiatry 23, 289–299. doi: 10.1016/j.eurpsy.2007.05.006
Stoodley, C. J., and Schmahmann, J. D. (2009). Functional topography in the human cerebellum: a meta-analysis of neuroimaging studies. Neuroimage 44, 489–501. doi: 10.1016/j.neuroimage.2008.08.039
Stoodley, C. J., and Schmahmann, J. D. (2010). Evidence for topographic organization in the cerebellum of motor control versus cognitive and affective processing. Cortex 46, 831–844. doi: 10.1016/j.cortex.2009.11.008
Teo, J. T., van de Warrenburg, B. P., Schneider, S. A., Rothwell, J. C., and Bhatia, K. P. (2009). Neurophysiological evidence for cerebellar dysfunction in primary focal dystonia. J. Neurol. Neurosurg. Psychiatry. 80, 80–83. doi: 10.1136/jnnp.2008.144626
Tobia, M. J., and Woodruff-Pak, D. S. (2009). Delay eyeblink classical conditioning is impaired in Fragile X syndrome. Behav. Neurosci. 123, 665–676. doi: 10.1037/a0015662
Tolbert, D. L., Bantli, H., and Bloedel, J. R. (1976). Anatomical and physiological evidence for a cerebellar nucleo-cortical projection in the cat. Neuroscience 1, 205–217. doi: 10.1016/0306-4522(76)90078-6
Tolbert, D. L., Ewald, M., Gutting, J., and La Regina, M. C. (1995). Spatial and temporal pattern of Purkinje cell degeneration in shaker mutant rats with hereditary cerebellar ataxia. J. Comp. Neurol. 355, 490–507. doi: 10.1002/cne.903550403
Tsai, P. T., Hull, C., Chu, Y., Greene-Colozzi, E., Sadowski, A. R., Leech, J. M., et al. (2012). Autistic-like behaviour and cerebellar dysfunction in Purkinje cell Tsc1 mutant mice. Nature 488, 647–651. doi: 10.1038/nature11310
Ulug, A. M., Vo, A., Argyelan, M., Tanabe, L., Schiffer, W. K., Dewey, S., et al. (2011). Cerebellothalamocortical pathway abnormalities in torsinA DYT1 knock-in mice. Proc. Natl. Acad. Sci. U.S.A. 108, 6638–6643. doi: 10.1073/pnas.1016445108
Uusisaari, M., and De Schutter, E. (2011). The mysterious microcircuitry of the cerebellar nuclei. J. Physiol. 589, 3441–3457. doi: 10.1113/jphysiol.2010.201582
Wadiche, J. I., and Jahr, C. E. (2005). Patterned expression of Purkinje cell glutamate transporters controls synaptic plasticity. Nat. Neurosci. 8, 1329–1334. doi: 10.1038/nn1539
Walter, J. T., Alvina, K., Womack, M. D., Chevez, C., and Khodakhah, K. (2006). Decreases in the precision of Purkinje cell pacemaking cause cerebellar dysfunction and ataxia. Nat. Neurosci. 9, 389–397. doi: 10.1038/nn1648
Wang, L., Jia, M., Yue, W., Tang, F., Qu, M., Ruan, Y., et al. (2008). Association of the ENGRAILED 2 (EN2) gene with autism in Chinese Han population. Am. J. Med.l Genet. B Neuropsychiatr. Genet. 147B, 434–438. doi: 10.1002/ajmg.b.30623
Welsh, J. P., Yuen, G., Placantonakis, D. G., Vu, T. Q., Haiss, F., O'Hearn, E., et al. (2002). Why do Purkinje cells die so easily after global brain ischemia? Aldolase, C, EAAT4, and the cerebellar contribution to posthypoxic myoclonus. Adv. Neurol. 89, 331–359.
White, J., and Sillitoe, R. V. (2013). Development of the cerebellum: from gene expression patterns to circuit maps. WIREs Dev. Biol. 2, 149–164. doi: 10.1002/wdev.65
Whitney, E. R., Kemper, T. L., Bauman, M. L., Rosene, D. L., and Blatt, G. J. (2008). Cerebellar Purkinje cells are reduced in a subpopulation of autistic brains: a stereological experiment using calbindin-D28k. Cerebellum 7, 406–416. doi: 10.1007/s12311-008-0043-y
Yang, P., Shu, B. C., Hallmayer, J. F., and Lung, F. W. (2010). Intronic single nucleotide polymorphisms of engrailed homeobox 2 modulate the disease vulnerability of autism in a han chinese population. Neuropsychobiology 62, 104–115. doi: 10.1159/000315441
Yokoi, F., Dang, M. T., and Li, Y. (2012). Improved motor performance in Dyt1 ΔGAG heterozygous knock-in mice by cerebellar Purkinje-cell specific Dyt1 conditional knocking-out. Behav. Brain Res. 230, 389–398. doi: 10.1016/j.bbr.2012.02.029
Keywords: neurological disorders, genetics, circuitry, neural activity, brain behavior
Citation: Reeber SL, Otis TS and Sillitoe RV (2013) New roles for the cerebellum in health and disease. Front. Syst. Neurosci. 7:83. doi: 10.3389/fnsys.2013.00083
Received: 29 August 2013; Accepted: 25 October 2013;
Published online: 14 November 2013.
Edited by:
Thomas C. Watson, University of Bristol, UKReviewed by:
Charles R. Watson, Curtin University, AustraliaCopyright © 2013 Reeber, Otis and Sillitoe. This is an open-access article distributed under the terms of the Creative Commons Attribution License (CC BY). The use, distribution or reproduction in other forums is permitted, provided the original author(s) or licensor are credited and that the original publication in this journal is cited, in accordance with accepted academic practice. No use, distribution or reproduction is permitted which does not comply with these terms.
*Correspondence: Tom S. Otis, Department of Neurobiology, Geffen School of Medicine at University of California, Los Angeles, 650 Charles Young Dr. South, Room 63-251, Los Angeles, CA 90095, USA e-mail:b3Rpc3RAdWNsYS5lZHU=;
Roy V. Sillitoe, Department of Pathology and Immunology, Department of Neuroscience, Baylor College of Medicine, Jan and Dan Duncan Neurological Research Institute of Texas Children's Hospital, 1250 Moursund Street, Suite 1325, Houston, TX 77030, USA e-mail:c2lsbGl0b2VAYmNtLmVkdQ==
Disclaimer: All claims expressed in this article are solely those of the authors and do not necessarily represent those of their affiliated organizations, or those of the publisher, the editors and the reviewers. Any product that may be evaluated in this article or claim that may be made by its manufacturer is not guaranteed or endorsed by the publisher.
Research integrity at Frontiers
Learn more about the work of our research integrity team to safeguard the quality of each article we publish.