- BioSystOmics, Bellaire, TX, United States
How can synaptic efficacies be maintained despite the fast turnover of proteins at synapses? Partially, we know that the synthesis of new proteins is essential for the induction of the late, long-lasting phase of long-term potentiation (L-LTP). Recent experiments suggest that the concentration of protein kinase Mζ (PKMζ) is increased during L-LTP and that inhibiting the PKMζ activity during the maintenance phase can effectively reverse L-LTP. Experiments have also shown that phosphorylation is necessary for the activation of PKMζ. However, it is not clear what mechanism maintains the level and activity of PKMζ despite protein turnover and phosphatase activity. Using a mathematical modeling framework, I examine the hypothesis that the activity of PKMζ is sustained through a local switching mechanism. The model for the switching mechanism is motivated by several experimental observations: 1) PKMζ has two phosphorylation sites; one is mediated by another constitutively active kinase, Phosphoinositide-dependent kinase 1 PDK1 (T410) and is essential for its activity, and another is an autophosphorylation site, T560. 2) The phosphorylation of PKMζ increases its stability and the doubly phosphorylated PKMζ has a significantly longer lifetime than the unphosphorylated and singly phosphorylated states of PKMζ. 3) The doubly phosphorylated PKMζ also regulates the new synthesis of PKMζ through a translation feedback loop. The present study implemented a mass action model consistent with these observations. The results show that such a model can be bistable and that L-LTP induction produces an increase in the total amount of PKMζ at active synapses. The increase in PKMζ concentration was maintained through the regulation of new protein synthesis by PKMζ. The results also show that blocking the activity of PKMζ in a dose-dependent manner can effectively abolish the increase in the total amount of PKMζ, which is consistent with the effect that the PKMζ inhibitor zeta inhibitory peptide (ZIP) has experimentally demonstrated. The model is consistent with available experimental results regarding the phosphorylation levels of PKMζ and the temporal aspects of blocking experiments and produces a new prediction.
Introduction
Memory is critical to life. How memories are stored and maintained in a mammalian brain is an intriguing question. Usually, a cellular model known as synaptic plasticity is used to study the storage of long-term memories (Hebb, 1949). Synaptic plasticity is a use-dependent change in the efficiency of synaptic transmission (Bliss and Lomo, 1973; Bear, 1996; Morris et al., 2000). Two key features characterize these changes in synaptic efficacy: 1) input specificity through which the changes in the synaptic transmission are restricted to active contacts and 2) long-term endurance, which explains how the changes in synaptic transmission are maintained for a long period of time. How are these characteristic features retained despite the constantly changing conditions in the mammalian brain? This raises an interesting question, especially when the effective residence time scale of substrate proteins is much shorter than the lifespan of long-term memories (Crick, 1984; Lisman and Goldring, 1988). This could be partially addressed through synapse-specific protein synthesis, supported by a self-sustained molecular mechanism within the scale of a single synapse. Plasticity-related proteins synthesized through a local translation mechanism could effectively preserve the synaptic efficacies in the face of protein turnover.
A persistent increase in the efficiency of synaptic strength is known as late long-term potentiation (L-LTP) and is widely used as a physiological substrate to study synaptic plasticity (Bliss and Lomo, 1973; Bear, 1996). L-LTP has two distinct temporal phases: 1) induction is characterized by the initiation of potentiation and 2) maintenance is related to sustaining the potentiation for an extended period. Several different types of molecular candidates, such as receptors and kinases, have been implicated in the induction of LTP (Soderling, 2000; Hudmon and Schulman, 2002; Smolen et al., 2006). However, the molecular signature of maintenance is still elusive. A natural choice could be one of the abundant brain proteins. Calmodulin-dependent protein kinase II (CaMKII) has been identified in some experiments as contributing to the maintenance of L-LTP. Other experiments show that inhibiting CaMKII during the enduring phase does not affect L-LTP (Malinow et al., 1989; Otmakhov et al., 2004; Sanhueza et al., 2007). Recently, a series of experiments have demonstrated the role of another kinase, protein kinase Mζ (PKMζ), specifically in the enduring phase of plasticity (Sacktor et al., 1993; Osten et al., 1996; Naik et al., 2000; Ling et al., 2002; Hernandez et al., 2003; Sajikumar et al., 2005; Serrano et al., 2005; Pastalkova et al., 2006; Kelly et al., 2007; Shema et al., 2007; Serrano et al., 2008; Yao et al., 2008).
PKMζ is a brain-specific kinase and is now considered one of the key candidates for long-term memory maintenance (Sacktor et al., 1993; Osten et al., 1996; Hernandez et al., 2003). The specific role of PKMζ during the maintenance of L-LTP is attributed to its unique structural properties. PKMζ does not require a second messenger to sustain its activity (Hernandez et al., 2003) because this kinase consists of an independent catalytic domain of the PKC isoform. The PKC isoforms are divided into three different classes: conventional, novel, and atypical. Each of these isoforms consists of a single polypeptide comprising an N-terminal regulatory domain and a C-terminal catalytic domain interconnected through a hinge. The regulatory domain contains the binding sites for second messengers and the autoinhibitory pseudosubstrate sequence that interacts with and blocks the active site of the catalytic domain. Second messengers stimulate PKC by binding to the regulatory domain, which changes the conformational state of the enzyme and activates it. However, it is possible for PKC to undergo cleavage, permanently removing the regulatory domain to form the independent catalytic PKMζ domain. Without a regulatory domain, PKMζ can be persistently active without the need for a signal from a second messenger. Therefore, the PKMζ can phosphorylate substrates in the absence of second messengers. Previous results show that once formed in LTP, the autonomous activity of PKMζ maintains the synaptic potentiation for several hours (Hernandez et al., 2003; Sajikumar et al., 2005; Serrano et al., 2005; Pastalkova et al., 2006; Kelly et al., 2007; Shema et al., 2007; Serrano et al., 2008; Yao et al., 2008).
How exactly this autonomously active PKMζ molecule maintains the expression of L-LTP for an extended period remains unexplained. However, a recent proposal suggests that PKMζ maintains the extended LTP through a mechanism that sustains the trafficking of α-amino-3-hydroxy-5-methyl-4-isoxazolepropionic acid receptors (AMPARs) to synaptic contacts during the long enduring phase of L-LTP. These results show that PKMζ acts through N-ethylmaleimide-sensitive Fusion Protein (NSF) on an extra-synaptic pool of GluR2-containing receptors and during the maintenance phase PKMζ directs the trafficking pattern of AMPARs. Blocking this trafficking can effectively reverse the long-term potentiation (Yao et al., 2008). Thus, PKMζ maintains the late-LTP by persistently regulating the GluR2-mediated trafficking pattern of AMPARs and subsequently modulating their insertion into active sites of synaptic communication.
One idea surrounding the synapse-specific maintenance of L-LTP is based on the possibility of a bistable molecular switch. A bistable molecular switch can partially address the question of how the consolidation of L-LTP can survive the rapid turnover of PSD proteins. In mechanistic terms, the possibility of a bistable switch can arise because, at least theoretically, long-term consolidation of L-LTP requires a functional positive feedback molecular mechanism. Once the signaling cascade of such a molecular feedback loop is activated, it can be persistent for an extended period with either no stimulus or a minimum basal level of stimulation. This type of molecular system could lead to on/off switching characteristics at the level of an individual synapse. Such a switching system should be operating at the level of a single synapse to provide the input specificity of L-LTP. In this connection, two interesting proposals are being pursued: 1. A kinase-based activation/deactivation switch (Zhabotinsky, 2000; Lisman and Zhabotinsky, 2001; Miller et al., 2005). 2. A local translation switch based on the self-sustained synthesis of plasticity-related protein in the single spine (Aslam et al., 2009). My previous work, based on the local translation switch, is focused on the local synthesis of CaMKII through a self-sustained kinase/translation factor loop (Aslam et al., 2009).
The main objective of this work is to explore the possibility of a local translation switch in modulating the sustained increase in the concentration and activity of PKMζ during the maintenance phase of L-LTP. The switching proposal of this work will specifically address the two key questions. 1. How PKMζ levels are maintained during L-LTP for long period of time despite the turnover of PKMζ protein; 2. what causes the rapid reversal of PKMζ levels when the activity inhibitor zeta inhibitory peptide (ZIP) is applied during the late phase of LTP? This study is motivated by the following experimental observations: 1) L-LTP requires new proteins (Stanton and Sarvey, 1984; Frey et al., 1988). 2) The components of translation machinery are constitutively localized at the level of a single spine (Gardiol et al., 1999; Pierce et al., 2000; Ostroff et al., 2002; Tang et al., 2002) (3) L-LTP leads to an increase in the concentration of total PKMζ in hippocampal slices (Kelly et al., 2007). 4) The ratio of phosphorylated PKMζ to total PKMζ remains the same before and after LTP (Kelly et al., 2007). 5) Persistent activity of PKMζ is both necessary and sufficient to maintain the late LTP in hippocampal slices (Sacktor et al., 1993; Osten et al., 1996; Naik et al., 2000; Ling et al., 2002; Hernandez et al., 2003; Sajikumar et al., 2005; Serrano et al., 2005; Pastalkova et al., 2006; Kelly et al., 2007; Shema et al., 2007; Serrano et al., 2008; Yao et al., 2008). 6) The application of PKMζ activity inhibitor ZIP rapidly reverses the established late LTP both in hippocampal slices and in vitro without affecting the early phase of LTP or the baseline (Sajikumar et al., 2005; Serrano et al., 2005) (7) Inhibiting PKMζ also abolishes spatial memories in rats (Pastalkova et al., 2006).
The possibility that local translation could be a likely mechanism mediating the increase in PKMζ levels during L-LTP is supported by experimental observations that alternative ζ RNA (considered to be the transcript for PKMζ protein) is found in dendrites in abundant quantities (Hernandez et al., 2003). The levels of ζ transcript do not change 1 h after tetanization (Yao et al., 2008). Moreover, the PKMζ levels increase within minutes of a high-frequency stimulus that is too fast for the transcription of a 100 kb RNA, when the maximum rate of transcription could only be 1–2 kb/min (Hernandez et al., 2003). Together, these results point toward a locally active mechanism that causes the increase in PKMζ concentration after LTP and is most likely due to the translation of pre-existing ζ RNA, which is probably regulated at the level of a single synaptic contact.
In this study, I examine the possibility that local translation of PKMζ can be regulated through an on/off switch. Through a computational framework, I show that the proposed translation and activation/deactivation loop of PKMζ can act as a bistable switch and thus stabilize the changes in synaptic transmission despite the constant turnover of its molecular components. I also show that with the application of a protein synthesis stimulus pulse that mimics the high-frequency stimulation (HFS) protocol of L-LTP, a long-lasting elevation of total PKMζ levels consistent with the previous experimental observations can be induced. The results also indicate that the fraction of phosphorylated PKMζ remains nearly the same before and after the L-LTP stimulation, which is again consistent with experimental recordings. As shown by previous experimental work, a sustained increase in the total concentration of PKMζ and an almost constant proportion of phosphorylated PKMζ to total is co-related with a sustained increase in synaptic efficacies (kelly et al., 2007). Therefore, I focus on these two variables in the simulation experiments. Experimental results show that the ZIP is completely ineffective during induction (Sajikumar et al., 2005; Serrano et al., 2005). However, when applied in the late phase, it rapidly reversed the established potentiation in a dose-dependent manner (Pastalkova et al., 2006).
This study assumes that stimulus-induced elevation in PKMζ concentration is linked to potentiation. Indirectly, activity-blocking experiments confirm the aforementioned observations (Sajikumar et al., 2005; Serrano et al., 2005; Pastalkova et al., 2006). In the modeling experiments, I made additional predictions by blocking the Phosphoinositide-dependent kinase 1 (PDK1) and protein synthesis in both the induction and maintenance phases. These predictions indicate that blocking the constitutively active kinase PDK1 during the early phase does not produce an induction in the concentration of total PKMζ. In contrast, blocking PDK1 during the late phase reverses the increase in the total amount of PKMζ, although on a relatively slower time scale than activity blocking.
Results
Molecular model of local PKMζ translation loop
The proposed local translation model of PKMζ is based on a molecular network that is composed of translation/degradation and activation/deactivation sub-networks (Figure 1). The PKMζ protein in this model can reside in three distinct states: an unphosphorylated or inactive state of the PKMζ protein that cannot phosphorylate other substrates, a single phosphorylated form of the PKMζ protein, that is, phosphorylation at site T410, and a double phosphorylated form of the PKMζ protein, that is, phosphorylation at sites T410 and T560. Both the single and double phosphorylated forms of PKMζ protein are active molecules and can phosphorylate other substrates. In this network (Figure 1), phosphorylation enhances the stability of the PKMζ protein. The unphosphorylated PKMζ protein degrades at a much faster rate, whereas the doubly phosphorylated form is the most stable and degrades at a much slower rate. PKMζ is synthesized in the unphosphorylated form from its messenger RNA. The new protein synthesis in this molecular model (Figure 1) is either regulated through second messenger-dependent upstream kinases or through a positive feedback loop between the active form of PKMζ proteins and its transcript. During the induction phase, a large amount of PKMζ protein is synthesized through Ca2+-dependent upstream kinases, and after an initial burst, the amount of PKMζ generated through this pathway is relatively small. In the long maintenance phase, PKMζ translation is regulated in an ongoing manner through the active form of its proteins and thus provides a self-sustained mechanism in an input-specific manner to stabilize the synaptic efficacies during the persistent phase of L-LTP. The degradation of the PKMζ protein is a combination of several processes, including protein turnover and permanent migration of non-bound proteins out of synapses. All the processes modeled herein are complex, and they have been approximated by simpler processes that maintain their key qualitative features. An implicit assumption of this model is that PKMζ levels are directly related to synaptic efficacies, which is consistent with several previous experiments (Osten et al., 1996; Naik et al., 2000; Ling et al., 2002; Hernandez et al., 2003; Sajikumar et al., 2005; Serrano et al., 2005; Pastalkova et al., 2006; Kelly et al., 2007; Shema et al., 2007; Serrano et al., 2008; Yao et al., 2008). The single and double phosphorylated forms of the PKMζ protein are constitutively active, independent of the protein synthesis signal U. The active phosphorylated states of the PKMζ protein can be dephosphorylated via a phosphatase. In these simulations, the basal levels of three PKMζ are assumed to be equal and set at .0001 nM for each form of protein. The biochemical reaction rates for the PKMζ molecular loop are taken from the literature (Le Good et al., 1998). The degradation rates of different forms of the PKMζ molecule are estimated from previous work on PKCζ and personal communications (Le Good et al., 1998; Lee Good and Brindley, 2004; Kelly et al., 2007; Yao et al., 2008). In the proposed model, PKMζ messenger RNA can be either in the inactive form or active form. The messenger RNA, which is bound with translation machinery T, is the active form of the transcript and can undergo translation. I assume that PKMζ is synthesized in its inactive state. This model differs qualitatively from most previous models of molecular bistability (Kholodenko, 2000; Tyson et al., 2003), in that it does not assume conserved quantities of molecules and includes both protein synthesis and degradation.
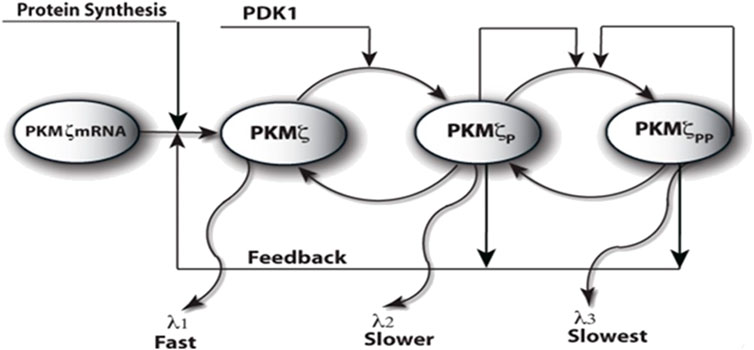
FIGURE 1. Proposed model of the local PKMζ synthesis through PKMζ translation/degradation and activation/deactivation molecular network. Here, the PKMζ molecule is in the inactive or the active and phosphorylated forms. The PKMζ is synthesized in naïve form from its messenger RNA through a protein synthesis stimulation mediated by multiple second messenger-dependent kinases. The unphosphorylated kinase form is rapidly phosphorylated at site T410 through another constitutively active kinase, PDK1. The singly phosphorylated form of PKMζ undergoes an autophosphorylation at site T560. The phosphorylation increases the stability of an otherwise unstable molecule. The PKMζ molecule has different turnover times in all three states. The turnover time of the naïve molecule is the shortest, and it is the longest for the doubly phosphorylated form. The active form of the molecule also regulates the new synthesis of PKMζ through a feedback loop.
Although this article primarily focuses on the maintenance phase of L-LTP, the induction of L-LTP is modeled using a brief pulse of protein synthesis, which represents all the upstream second messenger-dependent kinases involved in the synthesis of new proteins (Kelly et al., 2007). This pulse mimics the effect of an HFS induction protocol of L-LTP. This is a very simple approach that ignores many of the details of induction protocols and the complex signal transduction pathways that are involved. However, because maintenance and not induction is the focus of this work, a simple model was chosen so as not to obscure the essence of the results with details.
Memory switch
I next determined whether the local translation loop of PKMζ could operate as a bistable switch. I implemented the induction of L-LTP through a protein synthesis pulse U as mentioned in the previous section (Figure 2A). In the absence of a pulse (Figure 2—solid blue lines), with only a basal level of protein synthesis, the system moves from basal concentration to the lower steady state where the total concentration of PKMζ is .0003 μM (Figure 2A—solid blue line), as rest of the cell and the fraction of doubly phosphorylated PKMζ (T410 and T560) is 94% (Figure 2B—solid blue line). With the application of a protein synthesis pulse (15 min), PKMζ rapidly increases from basal level to 3.0 μM and is then maintained at this level for an extended period (Figure 2a—dotted red line). In addition, the fraction of doubly phosphorylated PKMζ becomes elevated (Figures 2b—dotted red line) and reaches 97%. These results are consistent with experimental observations (Kelly et al., 2007) in which the L-LTP increases the concentration of total PKMζ, and the ratio of doubly phosphorylated to total PKMζ remains unchanged during L-LTP.
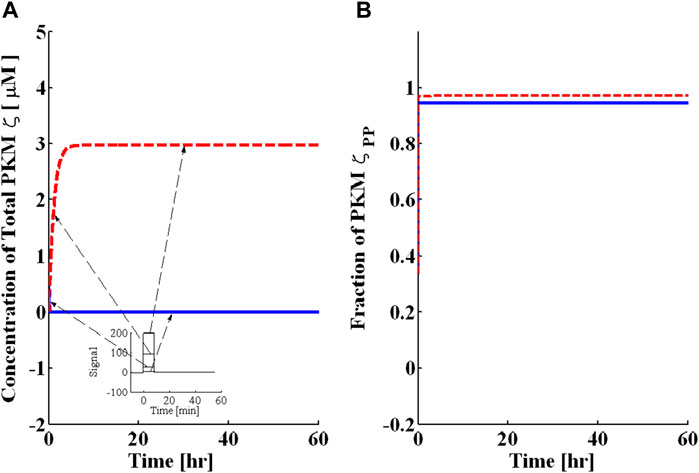
FIGURE 2. Bistability characteristics of the PKMζ local translation loop. A brief pulse of protein synthesis is used to mimic the induction of L-LTP by applying a high-frequency stimulus. The solid blue line represents PKMζ, the concentration at basal levels of protein synthesis (without the application of pulse), and the dashed red line represents the PKMζ concentration with the application of a protein synthesis pulse. (A) Total PKMζ concentration [μM] with the lower steady state at .0003 μM and the upper steady state at ≈ 3.0 μM. The inset mimics the HFS protocol representing the different strengths of protein synthesis pulse for L-LTP. (B) Fraction of doubly phosphorylated and active PKMζ with the lower steady state at ≈ 94% and the upper steady state at ≈ 98%. These results show that even with different amplitudes of protein synthesis pulse stimulus, the PKMζ loop converges to the same up or downregulated states. These results indicate that this system is bistable; that is, with a sufficiently strong stimulus, it becomes upregulated, and without enough stimulus, it is downregulated.
The temporal dynamics depicted in Figure 2 clearly show two phases of L-LTP, an early phase in which the concentration of PKMζ rapidly increases (60–90 min) followed by a persistent phase in which the concentration of PKMζ is maintained over a long period of time. The system depicted here is bistable because stimuli of different amplitudes can be provided, but the system will converge to one of only two possible stable states, depending upon the amplitude and duration of the stimulus (Figure 2). These two stable states are called the “up” and “down” states. The sustained increase in PKMζ amount and activity is both necessary and sufficient for late long-term potentiation.
Two key processes that might control the stability of the proposed molecular model are protein synthesis and protein degradation (Figure 1). In the simple model of degradation, protein turnover is characterized by turnover rates. Figure 3 presents the results of simulations with different levels of PDK1-mediated activation parameter k6. At small values of PDK1-mediated activation, there are not enough signals to stabilize the naïve PKMζ molecule; therefore, the system is monostable as all the new synthesis degrades from the naïve state at a much faster degradation rate (Supplementary Figure S1A). As the PDK1 parameter k6 increases, the system becomes bistable (Supplementary Figures S1B,C), and a balance between the degradation of PKMζ from the naïve state and its activation is established (Supplementary Figures S1B,C). When the PDK1 rate is high enough, the PKMζ is quickly activated and is trapped in stable states (T410 and T410, T560). The system loses its bistability and becomes monostable (Supplementary Figure S1D).
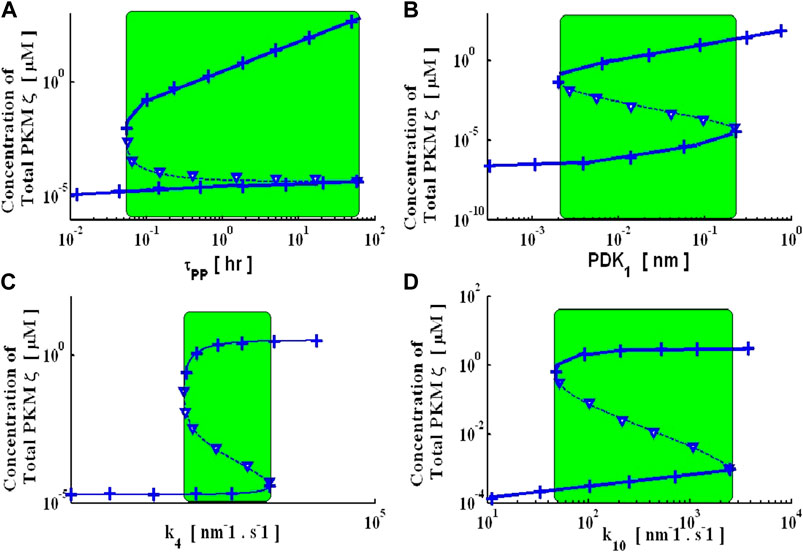
FIGURE 3. Bifurcation diagrams represent the steady-state solutions of this dynamical system with respect to model parameters. These steady-state solutions are generated with a non-linear continuation base global solver and through an analytical expression. Numeric (solid and dashed lines: solid lines represent stable up- and downstate solutions, whereas dashed lines represent an unstable solution) steady-state solutions are tracked with a non-linear global continuation-based solver. Analytical bifurcation diagrams (cross and downward triangle signs: where the cross represents stable up- and downstate analytical branches, and the downward triangle sign represents the unstable analytical branch) are generated using an analytical expression. Four key parameters are selected for generating bifurcation diagrams. (A) Turnover rate of doubly phosphorylated PKMζ (τPP), (B) intensity of the PDK1 signal, (C) feedback signal parameter to regulate protein synthesis rate-k4, (D) activity regulation parameter k10.
Bistability also critically depends on the feedback strength. Here, the feedback from the active and stable form of PKMζ regulates the new synthesis of proteins. The protein synthesis in the simple model is regulated by three different signals: an upstream second messenger-dependent signal, protein synthesis due to the feedback of singly phosphorylated PKMζ, and protein synthesis due to doubly phosphorylated PKMζ. Figure 4I shows how the feedback from the doubly phosphorylated form of PKMζ, that is, parameter k4, affects the dynamics of the model. This demonstrates that at low k4, the molecular switch has a monostable character (Supplementary Figure S2A), which implies that at lower feedback strength, the synthesis rate of new protein synthesis is not sufficient to provide a replacement of protein loss due to degradation, and, therefore, the upregulated state of PKMζ cannot be maintained for a long period of time, leading to a gradual loss of synaptic strength. However, at a high feedback rate k4, the synthesis of new PKMζ molecules sufficiently compensates for loss due to protein turnover. It also demonstrates that a higher synthesis rate of new proteins leads to higher steady-state levels of PKMζ (Supplementary Figures S2B,C). As the feedback strength is increased to a much higher strength, the rate of new protein synthesis is much higher than any loss due to degradation; thus, the system becomes monostable again (Supplementary Figure S2D).
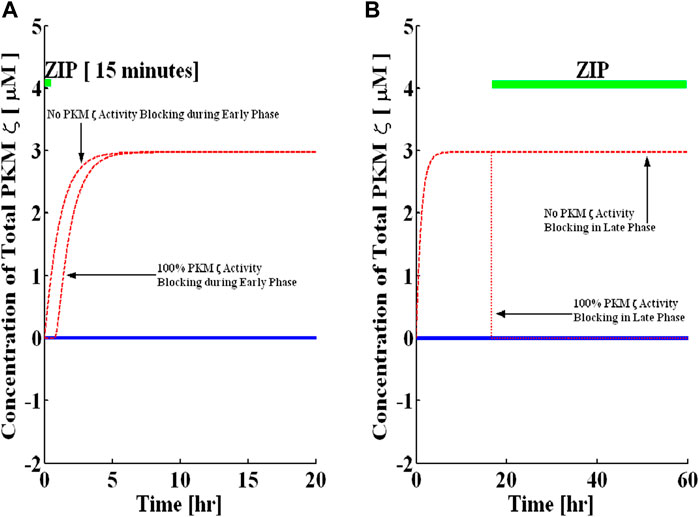
FIGURE 4. Blocking of PKMζ activity in the early and late phases. Simulations are implemented with and without protein synthesis pulse U. A brief pulse of protein synthesis is used to mimic the induction of L-LTP by applying a high-frequency stimulus. The solid blue line represents PKMζ, the concentration at basal levels of protein synthesis (without the application of pulse), and the dashed red line represents the PKMζ concentration with the application of a protein synthesis pulse. (A) PKMζ activity blocking during induction for 15-min (solid green line shows blocking time). Complete blocking of activity during the early phase does not affect the induction phase of L-LTP. (B) Activity blocking during maintenance for 90 h (solid green line shows blocking time). The complete activity blocking during maintenance rapidly reverses the established L-LTP from the up to the down state.
To systematically evaluate the influence of physiologically significant parameters on the stable states of this dynamical system, I developed bifurcation diagrams for several of the key parameters: the PDK1 signal, the turnover time of doubly phosphorylated PKMζ (τPP), the feedback parameter that regulates the synthesis of new proteins (k4), and the PKMζ activity parameter (k10) (Figure 3). All these bifurcation diagrams show regions of bistability in which there are three fixed points, two of them stable (solid line) and one unstable (dashed line). The bifurcation diagram for the turnover time τPP demonstrates there are three fixed points at intermediate degradation rates (Figure 3A). Two are stable (solid lines) and are termed the down and up states. For small τPP (very fast degradation), only the down state survives, suggesting that protein degradation rates are so fast that protein synthesis rates cannot catch up with the up state. For large τPP (slow degradation), only the up state survives. The bifurcation diagram with the PDK1 signal (Figure 3B) shows that at lower signal intensity, the molecular switch has only a down state and as signal intensity is increased, the molecular switch has both up and down states. The units of PDK1 used in this signal are nano-molar [nM]. Similarly, the bifurcation diagram with the protein synthesis parameters k4 and k10 (Figures 3C,D) shows both bistable and monostable regions depending on the parameters. These bifurcation diagrams have wide bistability regions, suggesting that this is a robust system.
The steady states of this complex dynamical system described by Equations 1–12) can be determined analytically. The system can be reduced to a fifth-order polynomial (Supplementary Material). The fixed points of this dynamical system are the zero crossings of this polynomial. Because this is a fifth-order polynomial, it has at most five real roots; two falls into a non-physiological regime (negative). Based on the analytical expression, I also developed the analytical bifurcation diagrams (Figure 3). A comparison of the analytical and numerical results demonstrates an exact match between the two approaches (Figure 3). A transition from a bi-stable to a monostable system means that as a parameter is changed, the number of positive real zeros of the polynomial changes from 3 to 1.
Blocking PKMζ activity during the induction and maintenance of L-LTP
Previous experimental results have indicated that PKMζ activity is not necessary for the induction of L-LTP (Sajikumar et al., 2005; Serrano et al., 2005). However, sustained activity is both necessary and sufficient for L-LTP maintenance for an extended period. Several experiments with hippocampal slices (Hernandez et al., 2003; Sajikumar et al., 2005; Serrano et al., 2005; Pastalkova et al., 2006; Yao et al., 2008) show that blocking PKMζ activity through a specific PKMζ activity inhibitor ZIP rapidly reverses the established L-LTP. The effectiveness of ZIP in reversing the L-LTP in slices is dependent on the dose and activity of the inhibitor peptide (Serrano et al., 2005). Another set of experimental recordings from an intact animal shows that ZIP is also effective in rapidly reversing the established L-LTP without affecting the baseline and early phase induction when applied after 22 h of initial stimulation (Pastalkova et al., 2006). The same work has also demonstrated that ZIP successfully abolished some spatial memories in rats (Pastalkova et al., 2006). Based on these observations, I set out to address the question of PKMζ activity blocking during induction and maintenance of L-LTP through the molecular network of local translation. I implemented PKMζ activity blocking in the simulations by simultaneously inhibiting the two-reaction rate constants k8 and k10. Both these forward reaction rate constants represent the autophosphorylation loop of PKMζ. The forward reaction rate constant k8 represents the autophosphorylation through neighbor–neighbor interaction between two singly phosphorylated (T410) PKMζ molecules, whereas the forward reaction rate constant k10 represents the autophosphorylation due to the interaction between a doubly phosphorylated PKMζ molecule (T410 and T560) and a singly phosphorylated PKMζ molecule (T410).
First, I simulate the application of PKMζ activity inhibitor ZIP during the induction phase of L-LTP (ZIP is applied during the first 15 min of L-LTP) by simultaneously reducing the forward rate constants of k8 and k10. Both these reaction rates are completely blocked. Figure 4A shows that the ZIP has no effect on the induction of L-LTP. This result is consistent with previous experimental recordings showing that ZIP has no effect on the induction of L-LTP in slices (Sajikumar et al., 2005; Serrano et al., 2005).
Next, the application of ZIP starting 16 h after the induction of L-LTP is simulated (Figure 4B). The results demonstrate that complete inhibition of PKMζ activity during the maintenance phase of L-LTP reversed the up state to the down state in a very rapid manner. Additionally, if ZIP is applied in a dose-dependent manner (Supplementary Figure S3), it requires 99% inhibition of activity to reverse L-LTP. If more than 99% of k8 and k10 are blocked, the system reverts to the down state and is no longer bistable. I also examined the minimum time required to inhibit activity for L-LTP reversal (Supplementary Figure S4) and found that at least 238 min of ZIP application is required for effective reversal of L-LTP.
This implies that a strong dose of ZIP is required to reverse L-LTP during the maintenance phase. The results also indicate that a dose-dependent application of ZIP can reverse the L-LTP in the enduring phase, and the dynamics of this reversal are dependent on the strength of the dose (Supplementary Figure S3 and Supplementary Figure S4). If the dose is very strong (100% inhibition of k8 and k10), the reversal is very rapid; otherwise, it is relatively slow (99% inhibition of k8 and k10; Supplementary Figure S3). Again, these dose-dependent dynamics are consistent with ZIP recordings in hippocampus slices (Serrano et al., 2005). Although it seems quantitative predictions could be made from this simulation framework, the proposed model is a simplified version of a complex network, and many of the parameters are unknown. Thus, the model cannot be expected to make precise quantitative predictions, and the numbers quoted previously should not be understood as precise predictions. However, because this system is robust, the qualitative predictions made here are likely to survive.
Blocking the PDK1 activity during the induction and maintenance of L-LTP
The role of another constitutively active kinase PDK1 is critical in stabilizing the PKMζ molecule and hence the L-LTP. In-brain PDK1 and PKMζ have stable and strong interactions (Kelly et al., 2007). Both PDK1 and PKMζ precipitate with each other and share a common binding domain (PIF). However, the effect of PDK1 on L-LTP is not clear. Thus, I set out to address the question of PDK1 activity blocking during the induction and maintenance of L-LTP through the local translation model. PDK1 activity blocking was implemented by inhibiting the forward reaction rate constant (k6).
Like PKMζ activity inhibitors, the application of PDK1 activity inhibitors during the induction phase of L-LTP (the first 15 min of L-LTP) is first simulated by reducing the value of k6. Note that, unlike ZIP, the complete PDK1 activity blocking abolishes the induction (Figure 5A). However, during the late phase when PDK1 activity is blocked at 16 h after induction (Figure 5B), the 100% blocking of PDK1 activity results in a complete reversal of the up state to the down state, although the dynamics of reversal are slower than those of ZIP blocking (Figure 6B). Here, the PDK1 blocking is also a dose-dependent phenomenon (Supplementary Figure S5), and the minimum time required for a complete reversal in the case of PDK1 is around 500 minutes (Supplementary Figure S6).
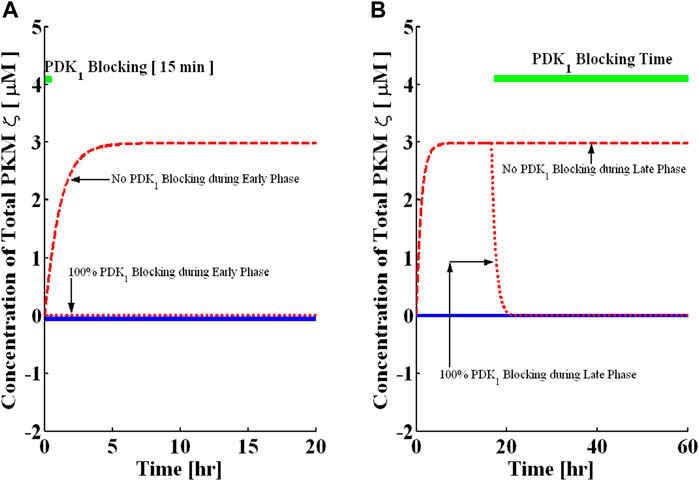
FIGURE 5. Blocking the PDK1 activity in the early and late phases. Simulations are implemented with and without protein synthesis pulse U. A brief pulse of protein synthesis is used to mimic the induction of L-LTP by applying a high-frequency stimulus. The solid blue line represents PKMζ, the concentration at basal levels of protein synthesis (without the application of pulse), and the dashed red line represents the PKMζ concentration with the application of a protein synthesis pulse. (A) PDK1 activity blocking during induction for 15 min (solid green line shows blocking time). Complete blocking of activity during the early phase completely abolishes the induction phase of L-LTP. (B) PDK1 activity blocking during maintenance for 90 h (solid green line shows blocking time). The complete activity blocking during maintenance reverses the established L-LTP from the up to the down state.
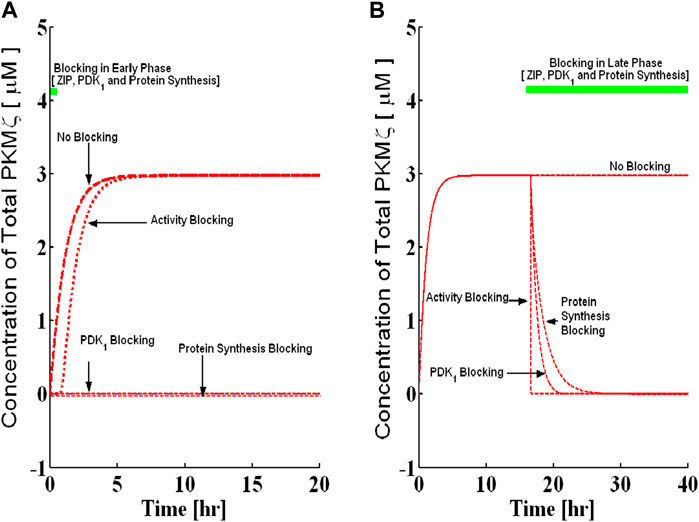
FIGURE 6. Blocking the protein synthesis in the early and late phases and its comparison with the PDK1 and PKMζ activity block. Simulations are implemented with and without protein synthesis pulse U. A brief pulse of protein synthesis is used to mimic the induction of L-LTP by applying a high-frequency stimulus. The solid blue line represents the PKMζ concentration at basal levels of protein synthesis (without the application of pulse), and the dashed red line represents the PKMζ concentration with the application of a protein synthesis pulse. (A) Complete block of protein synthesis during induction for 15 min (solid green line shows blocking time). Complete blocking of protein synthesis during the early phase completely abolishes the induction phase of L-LTP. (B) Blocking the protein synthesis during maintenance for 90 h (solid green line shows blocking time). The complete protein synthesis blocking during maintenance slowly reverses the established L-LTP from the up to the down state. Compared to the PKMζ and PDK1 activity block, the protein synthesis blocking reversed the established L-LTP on a slower timescale.
Blocking protein synthesis during the induction and maintenance of L-LTP
The translation inhibitors lead to quite different outcomes when applied during the induction and maintenance phases of L-LTP. When translation inhibitors are applied during the induction phase, L-LTP is compromised (Kang and Schuman, 1996; Frey and Morris, 1997). However, when applied later after the induction of L-LTP is complete, translation inhibitors do not reverse the potentiation (Frey and Morris, 1997; Fonseca et al., 2006). Thus, the effective blocking of L-LTP can only be accomplished during a transient window, that is, during induction. This might be taken to imply that protein synthesis is important for the induction but not the maintenance of L-LTP, posing a significant fundamental challenge to the theory of local translation. Previous work (Aslam et al., 2009) showed that the requirement of protein synthesis is very small during maintenance and requires a very high degree of inhibition before reversing the L-LTP. Here, protein synthesis blocking experiments were implemented on a mechanistic model of PKMζ translation. The three parameters are blocked simultaneously to mimic the effect of protein synthesis inhibitors. I simultaneously inhibit the kinetic parameters k3, k5, and k50 during different temporal windows to model the effects of protein synthesis inhibitors on the induction and maintenance of L-LTP.
First, the application of protein synthesis inhibitors during the induction phase of L-LTP (the first 33 min of L-LTP) is simulated by reducing the value of kinetic parameters k3, k5, and k50. The complete inhibition of protein synthesis in the early phase does not lead to any induction (Figure 7A). Furthermore, the outcome of blocking protein synthesis depends on the dose of the protein synthesis inhibitor. Here, when the protein synthesis inhibitor causes a reduction in the protein synthesis rate constant by less than 83%, the inhibitor does not produce any effect on L-LTP maintenance. However, as blocking levels are increased beyond 84%, the L-LTP is disrupted (Figure 7A).
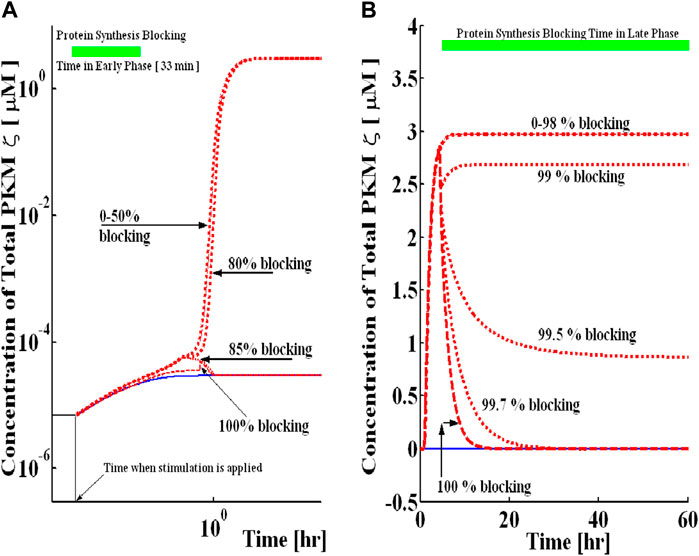
FIGURE 7. Blocking of protein synthesis during the early and late phases in a dose-dependent manner. Simulations are implemented with and without protein synthesis pulse U. A brief pulse of protein synthesis is used to mimic the induction of L-LTP by applying a high-frequency stimulus. The solid blue line represents the PKMζ concentration at basal levels of protein synthesis (without the application of pulse), and the dashed red line represents the PKMζ concentration with the application of a protein synthesis pulse. (A) Protein synthesis blocking during induction for 33 min (solid green line shows blocking time). For different levels of blocking, L-LTP has a different outcome during the maintenance phase. (B) Protein synthesis blocking during maintenance for 44 h (solid green line shows blocking time). Even for a very high percentage of blocking, the upregulated state can still be maintained, suggesting that if PKMζ is a trace for long-term memories, its expression can still be observed even if the synthesis of new proteins is blocked.
Simulations were also used to mimic the application of protein synthesis inhibitors during the maintenance phase of L-LTP. I selected a time point of 16 h during simulations to mimic the application of protein synthesis inhibitors (Figure 7B). The results demonstrate that inhibition of protein synthesis during the maintenance phase of L-LTP does not produce a significant change in the total concentration of PKMζ if less than 98% blocking of three kinetic parameters k3, k5, and k50 is carried out, and the system remains in the up state. If more than 99% of these parameters are blocked, the system reverts to the down state and is no longer bistable (Figure 7B). This implies that much stronger inhibition of protein synthesis is required to reverse L-LTP during the maintenance phase than to block it during the induction phase. Also, at least 13.33 h of protein synthesis blocking is needed for reversal (Supplementary Figure S7). Due to many simplifying assumptions and unknown kinetic parameters, the results presented here should not be taken for exact quantitative predictions. These results suggest a qualitative understanding of the complex processes mediating the maintenance mechanism of memory.
Discussion
This study suggests that the sustained increase in PKMζ activity during L-LTP can be modulated through a local switch. The local switching behavior is due to the synthesis/degradation and activation/deactivation of regulatory networks. This molecular switch is developed based on previous experimental observations (Sacktor et al., 1993; Osten et al., 1996; Le Good et al., 1998; Naik et al., 2000; Ling et al., 2002; Hernandez et al., 2003; Sajikumar et al., 2005; Serrano et al., 2005; Pastalkova et al., 2006; Kelly et al., 2007; Shema et al., 2007; Serrano et al., 2008; Yao et al., 2008). The bistability in this proposed molecular model is due to two key features: 1) The three different forms of PKMζ molecule in the network degrade at different rates; 2) The active form of PKMζ can regulate its synthesis through a positive feedback loop. The results indicate that the proposed molecular network can indeed behave as a two-state system, and the amount of total PKMζ increases in active synapses, whereas the ratio of phosphorylated to total PKMζ almost remains the same before and after the stimulation. These simulation results are consistent with previous experimental observations that show the amount of PKMζ increases in tetanized slices while the ratio of phosphorylated to total protein is almost constant before and after potentiation (Kelly et al., 2007).
The molecular network of local PKMζ translation is described by thirteen differential equations. Identifying the dynamical characteristics and locating the fixed points of this high-dimensional system is critical for understanding the local PKMζ translation and signaling. For reliability of computing, both numerical (Dhooge et al., 2003) and analytical approaches were used to solve this system. In the analytical approach, the non-linear system of thirteen differential equations is further simplified into a single fifth-order polynomial. This polynomial equation is then solved to generate analytical bifurcation diagrams, which match with bifurcation diagrams generated through a numerical package (Dhooge et al., 2003).
The bistable character of the local PKMζ translational switch ensures two stable states (up and down) in which the protein loss due to degradation is equal to the amount generated through de novo synthesis. The basal concentration of three different forms of PKMζ protein is set at a negligibly small value (.0001 nM). The baseline stimulation of protein synthesis transforms the basal PKMζ levels to a down state of the system that is slightly higher than the basal concentration. In contrast, a large magnitude protein synthesis pulse drives the system to the up state from basal levels. In the up state, the concentration of PKMζ is higher in stimulated synapses than the basal levels because at a specific stimulated synapse, the unphosphorylated PKMζ molecule is stabilized due to sequential phosphorylation of an otherwise unstable kinase. The stable and active PKMζ molecule also regulates its synthesis through a positive feedback loop and provides the new proteins during the maintenance phase. If a small perturbation is made in the up state by transiently increasing the concentration of active PKMζ, its degradation will also proportionally increase, thus reducing the rate of synthesis of naïve PKMζ and causing the system to return to the up state.
Bifurcation diagrams were generated for certain selected parameters. I selected τPP, PDK1, k4, and k10 as bifurcation parameters and through both an analytical expression and numerical package (Dhooge et al., 2003; Aslam and Sunol, 2006), and the bifurcation diagrams were tracked. These bifurcation diagrams show that the proposed molecular network is robustly bistable. They provide a simple and straightforward way to describe how the steady-state solutions of a non-linear system of equations describing the local network of PKMζ signaling may change with variations in biochemical parameters. These diagrams are also a convenient tool to locate all the steady-state solutions of a non-linear system and how the number of solutions may vary with variations in model parameters. For example, the bifurcation diagrams described here (Figure 3) show that within the certain range of parameters τPP (Figure 3A green shaded region), PDK1 (Figure 3B green shaded region), k4 (Figure 3C green shaded region), and k10 (Figure 3D green shaded region), the number of steady-state solutions is 3, whereas for parametric values beyond this region (both lower and higher), only one steady-state solution is identified. The green shaded region of Figure 3 defines the switchable parametric region characterized by two stable steady-state solutions and one unstable steady-state solution. Furthermore, within this parametric region, it may be possible to switch from down to upstate or vice versa with changes in parametric values. Interestingly, each of these bifurcation diagrams is generated by only varying one parameter at a time while keeping all other parameters fixed at baseline values (Supplementary Table S1). The width of the green region defines the robustness of switching behavior and, as is apparent from Figure 3, the switch described here is robust as the switchable region is wide. In this molecular network, the de novo synthesis of the PKMζ protein from its transcript is regulated at two distinct levels: 1. Synthesis through an external stimulation; 2. Synthesis is regulated through the positive feedback effects of active forms of the PKMζ protein.
Here, PKMζ translation is modeled through elementary biochemical reactions and does not provide a detailed account of various molecular steps involved in the complex process of translation (Watson et al., 2008). Previous experimental results suggest that multiple upstream kinases engage in the synthesis of PKMζ from its messenger RNA (Kelly et al., 2007). No attempt is made to model the multiple signaling networks involved in the local synthesis of PKMζ. An external signal U is used to implicitly represent the cumulative effect of multiple kinases involved in the synthesis of PKMζ. A simple translation model can only provide a conceptual representation of detailed and complex molecular processes involved. Future work might involve adding more details and coupling different upstream signaling cascades to this minimal model of local PKMζ translation.
The model described in this work assumes that enough PKMζ transcripts are available in local dendrites. This assumption is supported by previous experimental observations (Muslimov et al., 2004) that show that under basal conditions, PKMζ mRNA is present at postsynaptic contacts and is translationally repressed through another factor BC1. The synaptic stimulation would result in the de-repression of translation and promotes the local synthesis of PKMζ. Here, I do not have an estimate regarding the exact concentration of PKMζ mRNA at a single synapse. However, for simulation purposes, I assume a sufficiently large pool of PKMζ transcripts is localized at a single synapse. The minimal translation model also does not account for different pathways regulating the stability/degradation and transport of messenger RNA in dendrites.
Another assumption of this work is the requirement of PDK1 activity for the stability and activity of the PKMζ protein cascade. Here, I assume that PDK1 has a high basal activity even in non-stimulated cells, thus leading to the notion that it is a constitutively active kinase, and its activity is not critically regulated. This may not be an unrealistic assumption, as previous experiments (Kelly et al., 2007) indicate that the interactions between PDK1 and PKMζ in the brain are strong and stable, resulting in the maximum phosphorylation of PKMζ protein even with minimal activity levels of PDK1. This contrasts with only the transient interaction of c/nPKCs and PDK1; therefore, c/nPKCs further require the second messenger for their sustained activity. However, recent studies in other cells have also demonstrated that the function of PDK1 is under tight control, with phosphorylation depending on substrate conformation and subcellular localization (Toker and Newton, 2000).
This study also demonstrates that PKMζ activity inhibitor (ZIP) can rapidly reverse the L-LTP if applied during the maintenance phase but has no effect on the protein synthesis-independent early phase of LTP (E-LTP) when applied during induction (Sajikumar et al., 2005; Serrano et al., 2005).
This occurs because, during induction, the PKMζ activity is only blocked transiently (15 min). During this time window, the constant synthesis of naïve PKMζ is still going on (induction of L-LTP increases the protein synthesis). The unphosphorylated form of PKMζ protein undergoes rapid phosphorylation at site T-410 through a strong and stable interaction with PDK1. Although the unphosphorylated PKMζ molecule is unstable and degrades at a much faster rate, the rate of degradation of singly phosphorylated PKMζ at site T-410 is slower. Thus, as soon the activity is restored after the first 15 min, there is enough PKMζ in the T-410 state to trigger the autophosphorylation loop and transform the unstable state of kinase to a much more stable state.
However, during the maintenance phase, blocking the PKMζ activity turns off the only source of feedback. The feedback from the active form of kinase regulates the new synthesis of PKMζ protein. When this feedback is blocked through ZIP, new proteins are not synthesized, and the compensatory contribution of ongoing protein synthesis is no longer present to balance the protein degradation effects. The existing pool of proteins trapped in the up state quickly undergoes dephosphorylation to a non-phosphorylated form and is removed from the system. This whole cascade leads to a rapid reversal of L-LTP when ZIP is applied during the maintenance phase.
The results also indicate the role of PDK1 during the induction and maintenance of L-LTP. Blocking PDK1 during induction for 15 min does not yield any induction due to the fast degradation of the unphosphorylated PKMζ molecule. All the new synthesis of this form of PKMζ is removed because PDK1 is not available to transform it to a more stable form. However, blocking the PDK1 during the maintenance phase leads to the reversal of L-LTP. The reversal, in this case, is slower than the reversal when ZIP is applied during maintenance. This is because the ZIP and PDK1 inhibitors act at different sections of the molecular network. The blocking of ZIP terminates the processes through which PKMζ is synthesized and subsequently stabilized; therefore, the reversal is very rapid. In contrast, the PDK1 blocking only terminates the transformation process of the unstable naïve form of the molecule to a more stable form of PKMζ (phosphorylated at the T410 site). However, it does not have any effect on the autophosphorylation and feedback sub-sections of the network. Consequently, although the newly synthesized form of kinase cannot be moved to more stable forms due to PDK1 blocking, the existing proteins of the up state will take time to dephosphorylate and degrade. The PDK1-based reversal of L-LTP is slower than ZIP blocking during the maintenance phase.
This work is based on identifying and modeling the autonomous mechanisms supporting LTP maintenance that may regulate PKMζ levels at local synapses and impart optimal kinase activity required for LTP maintenance. Previous observations suggest stable and robust interactions between PDK1 and PKMζ in hippocampal slices (PKMζ precipitation leading to PDK1 precipitation and vice versa), leading to maximal activation-site phosphorylation (Kelly et al., 2007) and establishment of a self-sustaining loop to maintain PKMζ activity levels during the late phase of LTP despite ongoing protein turnover. Here, it is assumed that PDK1 activity in this complex is constitutive and is not regulated by any second messenger (Kelly et al., 2007). The role of constitutively active PDK1 in stabilizing the newly synthesized and labile PKMζ is one of the central assumptions of this study and may be questioned as controversial. However, previous observations in hippocampal slices show that phosphorylation states of PKMζ before and after LTP are equivalent and are not influenced by any inhibitors (Kelly et al., 2007). Additional observations show direct interactions between PKMζ and PDK1 (Kelly et al., 2007). These observations provide support for the assumption and suggest that activation loop phosphorylation of PDK1 is mediated by a constitutively active PDK1 during maintenance (Kelly et al., 2007). In contrast to autonomous PKMζ phosphorylation events, the phosphorylation of PKCi/λ is a regulated event (Kelly et al., 2007). The possibility of an autonomously active PDK1 in this model could be critiqued; certain systems show evidence that PDK1 activity can be regulated by receptor-linked phosphoinositide 3 (PI3)-kinase that produces the lipid second messenger phosphatidylinositol (3,4,5)-triphosphate (PtdIns (3,4,5)-P3). The PtdIns (3,4,5)-P3 can bind to PDK1 and increase its activity (Levina et al., 2022). This mechanism may also be functional here, especially during induction, but in maintenance, PI3-kinase and all other upstream kinases have already resolved to their baseline activity levels, and the observed PKMζ activity during the maintenance phase is due to direct binding of PDK1, which, in turn, autonomously activates the newly synthesized unphosphorylated PKMζ even long after the resolution of activation of upstream kinases (Kelly et al., 2007). This is based on observations that show levels of phosphorylated to total PKMζ remain unchanged after potentiation (Kelly et al., 2007). However, it is entirely possible that during the maintenance phase, the baseline activity of PI3-kinase may act to provide a minimum activity of PDK1, thus enabling the stabilization of the PKMζ molecule. A self-sustaining stabilization mechanism is necessary for optimal PKMζ activity during maintenance, as without such a mechanism, the observed actions of PKMζ inhibitor ZIP cannot be explained.
Recent experimental results show that even through a short-duration ZIP application, the L-LTP can be reversed. The in vitro (Serrano et al., 2005) results show that almost 2–3 h of ZIP application during the maintenance reversed the L-LTP, and the time window is even much shorter for the in vivo case (Pastalkova et al., 2006). Compared to these findings, the simulation results show a greatly extended blocking time for ZIP (Figure 4B). Short-term blocking of ZIP and PDK1 was also modeled (Supplementary Figure S4 and Supplementary Figure S6). The simulation results indicate that at least 200 min of activity blocking is needed to irreversibly move the up state to down, and almost 500 min of PDK1 blocking is required to transform the up state to down. The results in a qualitative sense are consistent with short duration blocking of ZIP in L-LTP (Serrano et al., 2005; Pastalkova et al., 2006).
I also implemented the blocking of protein synthesis in both the induction and maintenance phases. The results show that blocking the protein synthesis in the early phase does not lead to any induction while blocking during the enduring phase slowly reverses the up state to down. The slow reversal of L-LTP in this case is attributed to the slower degradation rate of doubly phosphorylated PKMζ. Previous work (Aslam et al., 2009) provides an explanation of different requirements of protein synthesis during induction and maintenance and how it could be related to different outcomes of protein synthesis inhibitors during induction and maintenance. Interestingly, even with this network model, I can again confirm this finding, suggesting that the protein synthesis requirement during induction is much larger than during maintenance.
Regarding the role of protein synthesis in the maintenance of L-LTP, this model makes two interesting predictions: 1. Protein synthesis inhibition for at least 13.33 h is needed to reverse the L-LTP; 2. The degree of protein synthesis inhibition needs to be more than 98% for reversal. Though measurable and physiologically relatable, these predictions may be challenging to reproduce physiologically by the current experimental paradigm and may be critiqued as a purely theoretical exercise. Indeed, protein synthesis inhibitors are not selective enough to restrict the translation at the level of PKMζ mRNA, and the predicted duration of inhibition of >13 h s is more than the effective time of the current class of protein synthesis inhibitors. The duration of inhibition for protein synthesis inhibitors by these computational experiments seems to be too long, as the current class of inhibitors may diffuse out or wash out more quickly than 13 h. For these predictions to be physiologically reproducible, one may need inhibitors with slower diffusion in brain slices or in ex vivo brain or control release mechanisms that may ensure the persistency of protein synthesis inhibitors for 13 h. Similarly, the current class of inhibitors like anisomycin and cycloheximide are only 85%–95% effective; to reach 98% effectiveness, better effectiveness and specific inhibitor actions that may only target the new synthesis of PKMζ are needed.
The recovery kinetics after the application of protein synthesis inhibitors (washout) for different duration seems to be similar and may be deemed unrealistic. However, this may be due to the highly stable doubly phosphorylated form of PKMζ because the lifetime of the doubly phosphorylated form of PKMζ in this model is more than the duration of inhibition. As soon as the protein synthesis block is removed in the L-LTP maintenance phase, this isoform triggers and induces the synthesis of more new proteins, thus restoring the up state. Thus, in the late maintenance phase, all upstream kinases (signal U) have already resolved to their baseline and have no role in restoring the PKMζ concentrations after the washout of protein synthesis inhibitors. The similarity in recovery kinetics is due to the common mechanism acting to restore the PKMζ concentration and fixed lifetime of PKMζPP.
This model suggests that phosphorylation at site T560 is solely an autophosphorylation event with neighbor–neighbor interactions of singly phosphorylated kinases, that is, PKMζP--PKMζP, and interactions between doubly phosphorylated kinase and singly phosphorylated kinase, that is, PKMζP---PKMζPP, leading to double phosphorylation on PKMζ at sites T560 and T410. In the case of PKCζ, the mTORC2 may also play a critical role in T560 phosphorylation. Here, the base model does not account for any contribution by mTORC2 toward the PKMζ phosphorylation event at site T560 as the role of mTORC2 in PKMζat site T560 phosphorylation is not known. However, in the modified version of the model (Supplementary Figure S8), if incorporating a mTORC2-modulated T560 phosphorylation event along with autophosphorylation events, that is, PKMζP--PKMζP and PKMζP---PKMζPP, does not cause any change in the results, and the conclusions drawn from these results remain the same as those drawn from the basic version of the model.
One may wonder how this model compares with a previously published model of a local switch (Aslam et al., 2009). This model describes the autonomous synapse-specific mechanisms regulating PKMζ translation compared to the previous model of local CaMKII translation (Aslam et al., 2009). Although both models address the mechanisms of local protein synthesis and degradation, they have some differences: 1) the current model is not stimulated by a second messenger as PKMζ does not require a second messenger for its activation, whereas the previous model (Aslam et al., 2009) is stimulated with a second messenger pulse, that is, (Ca2+)4-CaM; 2) in the current model, all three forms of PKMζ have different degradation rates with the unphosphorylated enzyme degrading much faster, and the doubly phosphorylated form degrading much slowly from synapses; this feature is believed to be a contributing factor for bistable behavior. In contrast, all three forms of CaMKII degraded at the same rate in the previous model (Aslam et al., 2009); 3) the current model accounts for constitutive PKMζ activity by incorporating a constitutively active PDK1 and stable interactions between PDK1 and PKMζ, whereas, in the previous model, there is no constitutive activity mechanism of any of kinases involved; 4) this model also suggests that PKMζ phosphorylation levels before and after potentiation are almost the same (up and down states), suggesting they are not regulated, whereas the previous model (Aslam et al., 2009) indicates that CaMKII phosphorylation levels (up state) are elevated after potentiation compared to basal levels (down state), indicating a regulated event. Although there is direct and ample evidence that the PKMζ activity inhibitor ZIP can reverse potentiation late during maintenance, whereas the activity and protein synthesis inhibitors targeting CaMKII are unable to cause a reversal, the current model does not invalidate the previous CaMKII (Aslam et al., 2009) model. Rather, it is complementary because one key finding of the previous model (Aslam et al., 2009) suggests that the degree of inhibition required to cause a reversal in the maintenance phase is very high, and it is believed that the current class of CaMKII activity inhibitors are not able to generate that kind of blocking effects in the maintenance phase (wash out more quickly). Interestingly, through different molecules and different mechanisms of the local translational switch, the current model supports the findings of the previous model as the degree of inhibition required to reverse PKMζ-induced L-LTP is high, and the duration of inhibition is long. It could be that inhibitors may wash out, particularly protein synthesis inhibitors, whereas ZIP is effective enough to reverse L-LTP.
This model assumes that PDK1 is involved in stabilizing the newly synthesized PKMζ through a constitutive activation loop phosphorylation event. Intriguingly, previous observations (Tsokas et al., 2007) suggest that PDK1 may also be involved in new protein synthesis by regulating the activation of mTORC1. This means that during the induction phase, PDK1 may facilitate the stabilization of newly synthesized, non-phosphorylated enzymes and also contribute to the new synthesis of PKMζ, although this might only be a transient phenomenon. While this is not modeled here, it is possible that inhibiting PDK1 during induction may have a more pronounced influence on the model output.
Methods
Biochemical reactions
The biochemical reactions describing the local translation loop of PKMζ (Figure 1) are based on standard Michaelis–Menten type kinetics. The following set of reactions is used to describe the molecular interactions of this loop. The dynamical variables used are X represents PKMζ (both protein and transcript), a P subscript represents single phosphorylation, a PP subscript represents phosphorylation at two sites, and an M subscript represents PKMζ mRNA. The phosphatase P is approximated as a fixed parameter, not a dynamical variable, to simplify the bifurcation analysis. In simulations, this does not significantly alter the results. The signal U is a parameter that represents the signal from multiple upstream kinases to stimulate the synthesis of PKMζ protein from its messenger RNA. D1 represents the PDK1 concentration and is again a fixed parameter. Here, T represents the concentration of polyribosome. The reactions are:
The sequence of this loop starts with the loading of polyribosome T to PKMζ mRNA described by Eq. 1. This loaded transcript C1 is ready to undergo translation. During induction, the signal U from multiple upstream kinases acts on this loaded transcript and initiates the de novo synthesis of unphosphorylated PKMζ (X) from its RNA. This translation event is described by Eq. 2. The active forms of the PKMζ protein are the single, the form phosphorylated at the T410 site (XP) and the form that is doubly phosphorylated at sites T410 and T560 (XPP). Both these active forms also act on loaded transcript C1 and regulate the synthesis of X as shown in Eqs 3, 4. Once formed, X is unstable and rapidly undergoes maximum phosphorylation at site T410 through its strong binding with PDK1 as modeled by Eq. 5. The molecule XP initiates the neighbor–neighbor-dependent auto-phosphorylation and is phosphorylated at the auto-phosphorylation site T560. The autophosphorylation events are described by standard Michaelis–Menten kinetics as shown in Eqs 6, 7. The phosphatase enzyme regulates the dephosphorylation of XP and XPP molecules through Eqs 9, 8. The complete formulation in the form of differential equations and all the aforementioned reaction rates are described in the Supplementary Material.
Induction
In the simulations, L-LTP induction is simulated by a 15-min pulse of protein synthesis (basal protein synthesis signal U is set at .0001 [a.u], and for the initial 15 min, this concentration is increased by [100] [a.u]), which produces an upregulation in the total amount of PKMζ from a basal concentration of .0001 nM. The persistence of this upregulated state depends on the amplitude and duration of the protein synthesis pulse stimulus.
Temporal dynamics
These differential equations (Supplementary Material) were integrated through non-linear solvers (using Math-Works MATLAB). The dynamical coefficient values were estimated from limited experimental data. Unknown rate constants were scaled to obtain dynamics that are comparable to experimental values. All the molecular concentrations in the model are expressed as nano molars (nM), unless otherwise stated, and time is represented as minutes.
Bifurcation diagrams
The non-linear-coupled differential equations describing the aforementioned biochemical reactions are solved for their steady states. The steady-state solutions of these equations are determined by setting their right-hand side equal to zero and solving the corresponding system of algebraic equations through a global continuation-based solver (Aslam and Sunol, 2006). The steady states as a function of any model parameters are known as bifurcation diagrams. I tracked the bifurcation diagram of these non-linear algebraic equations through a continuation-based algorithm (Aslam and Sunol, 2006). To further verify the results, the bifurcation diagrams were reproduced with Matcont, a MATLAB-based package for numerical bifurcation analysis of ordinary differential equations (Dhooge et al., 2003).
Data availability statement
The original contributions presented in the study are included in the article/Supplementary Material, further inquiries can be directed to the corresponding author.
Author contributions
NA completed all this research on his own.
Conflict of interest
The author declares that the research was conducted in the absence of any commercial or financial relationships that could be construed as a potential conflict of interest.
Publisher’s note
All claims expressed in this article are solely those of the authors and do not necessarily represent those of their affiliated organizations, or those of the publisher, the editors, and the reviewers. Any product that may be evaluated in this article, or claim that may be made by its manufacturer, is not guaranteed or endorsed by the publisher.
Supplementary material
The Supplementary Material for this article can be found online at: https://www.frontiersin.org/articles/10.3389/fsysb.2022.933938/full#supplementary-material
References
Aslam, N., Kubota, Y., Wells, D., and Shouval, H. Z. (2009). Translational switch for long-term maintenance of synaptic plasticity. Mol. Syst. Biol. 5, 284. doi:10.1038/msb.2009.38
Aslam, N., and Sunol, A. K. (2006). Sensitivity of azeotropic states to activity coefficient model parameters and system variables. Fluid Phase Equilibria 240, 1–14. doi:10.1016/j.fluid.2005.11.019
Bear, M. F. (1996). A synaptic basis for memory storage in the cerebral cortex. Proc. Natl. Acad. Sci. U. S. A. 93, 13453–13459. doi:10.1073/pnas.93.24.13453
Bliss, T. V., and Lomo, T. (1973). Long-lasting potentiation of synaptic transmission in the dentate area of the anaesthetized rabbit following stimulation of the perforant path. J. Physiol. 232, 331–356. doi:10.1113/jphysiol.1973.sp010273
Dhooge, A., Govaerts, W., and Kuznetsov, Y. A. (2003). Matcont: A matlab package for numerical bifurcation analysis of ODEs. ACM TOMS 29, 141–164. doi:10.1145/779359.779362
Fonseca, R., Nägerl, U. V., and Bonhoeffer, T. (2006). Neuronal activity determines the protein synthesis dependence of long-term potentiation. Nat. Neurosci. 9, 478–480. doi:10.1038/nn1667
Frey, U., Krug, M., Reymann, K. G., and Matthies, H. (1988). Anisomycin, an inhibitor of protein synthesis, blocks late phases of LTP phenomena in the hippocampal CA1 region in vitro. Brain Res. 452, 57–65. doi:10.1016/0006-8993(88)90008-x
Frey, U., and Morris, R. G. (1997). Synaptic tagging and long-term potentiation. Nature 385, 533–536. doi:10.1038/385533a0
Gardiol, A., Racaa, C., and Triller, A. (1999). Dendritic and postsynaptic protein synthetic machinery. J. Neurosci. 19, 168–179. doi:10.1523/JNEUROSCI.19-01-00168.1999
Hernandez, A. I., Blace, N., Crary, J. F., Serrano, P. A., Leitges, M., Libien, J. M., et al. (2003). Protein kinase M zeta synthesis from a brain mRNA encoding an independent protein kinase C zeta catalytic domain Implications for the molecular mechanism of memory. J. Biol. Chem. 278 (41), 40305–40316. Epub 2003 Jul 11. doi:10.1074/jbc.M307065200
Hudmon, A., and Schulman, H. (2002). Neuronal ca2+/calmodulin-dependent protein kinase II: The role of structure and autoregulation in cellular function. Annu. Biochem. 71, 473–510. doi:10.1146/annurev.biochem.71.110601.135410
Kang, H., and Schuman, E. M. (1996). A requirement for local protein synthesis in neurotrophin-induced hippocampal synaptic plasticity. Science 273, 1402–1406. doi:10.1126/science.273.5280.1402
Kelly, M. T., Crary, J. F., and Sacktor, T. C. (2007). Regulation of protein kinase Mzeta synthesis by multiple kinases in long-term potentiation. J. Neurosci. 27 (31), 3439–3444. doi:10.1523/JNEUROSCI.5612-06.2007
Kholodenko, B. N. (2000). Negative feedback and ultrasensitivity can bring about oscillations in the mitogen-activated protein kinase cascades. Eur. J. Biochem. 267, 1583–1588. doi:10.1046/j.1432-1327.2000.01197.x
Le Good, J. A., Ziegler, W. H., Parekh, D. B., Alessi, D. R., Cohen, P., and Parker, P. J. (1998). Protein kinase C isotypes controlled by phosphoinositide 3-kinase through the protein kinase PDK1. Sci. (UNITED STATES) 281 (5385), 2042–2045. doi:10.1126/science.281.5385.2042
Lee Good, J., and Brindley, D. N. (2004). Molecular mechanisms regulating protein kinase Czeta turnover and cellular transformation. Biochem. J. 378, 83–92. doi:10.1042/BJ20031194
Levina, A., Fleming, K. D., Burke, J. C., and Leonard, T. A. (2022). Activation of the essential kinase PDK1 by phosphoinositide-driven trans-autophosphorylation. Nat. Commun. 13, 1874. doi:10.1038/s41467-022-29368-4
Ling, D. S., Benardo, L. S., Serrano, P. A., Blace, N., Kelly, M. T., Crary, J. F., et al. (2002). Protein kinase Mzeta is necessary and sufficient for LTP maintenance. Nat. Neurosci. 5, 295–296. doi:10.1038/nn829
Lisman, J. E., and Goldring, M. A. (1988). Feasibility of long-term storage of graded information by the Ca2+/calmodulin-dependent protein kinase molecules of the postsynaptic density. Proc. Natl. Acad. Sci. U. S. A. 85, 5320–5324. doi:10.1073/pnas.85.14.5320
Lisman, J. E., and Zhabotinsky, A. M. (2001). A model of synaptic memory: A CaMKII/PP1 switch that potentiates transmission by organizing an AMPA receptor anchoring assembly. Neuron 31, 191–201. doi:10.1016/s0896-6273(01)00364-6
Malinow, R., Schulman, H., and Tsien, R. W. (1989). Inhibition of postsynaptic PKC or CaMKII blocks induction but not expression of LTP. Science 245, 862–866. doi:10.1126/science.2549638
Miller, P., Zhabotinsky, A. M., Lisman, J. E., and Wang, X. J. (2005). The stability of a stochastic CaMKII switch: Dependence on the number of enzyme molecules and protein turnover. PLoS Biol. 3, e107–e717. doi:10.1371/journal.pbio.0030107
Morris, R. G. M., Martin, S. J., and Grimwood, P. D. (2000). Synaptic plasticity and memory: An evaluation of the hypothesis. Ann. Rev. Neuro 23, 649–711. doi:10.1146/annurev.neuro.23.1.649
Muslimov, I. A., Nimmrich, V., Hernandez, A. I., Tcherepanov, A., Sacktor, T. C., and Tiedge, H. (2004). Dendritic transport and localization of protein kinase mzeta mRNA: Implications for molecular memory consolidation. J. Biol. Chem. 279 (50), 52613–52622. doi:10.1074/jbc.M409240200
Naik, M. U., Benedikz, E., Hernandez, I., Libien, J., Hrabe, J., Valsamis, M., et al. (2000). Distribution of protein kinase Mzeta and the complete protein kinase C isoform family in rat brain. J. Comp. Neurol. 426, 243–258. doi:10.1002/1096-9861(20001016)426:2<243::aid-cne6>3.0.co;2-8
Osten, P., Valsamis, L., Harris, A., and Sacktor, T. C. (1996). Protein synthesis-dependent formation of protein kinase Mzeta in long-term potentiation. J. Neurosci. 16, 2444–2451. doi:10.1523/JNEUROSCI.16-08-02444.1996
Ostroff, L. E., Fiala, J. C., and Harris, K. M. (2002). Polyribosomes redistribute from dendritic shafts into spines with enlarged synapses during LTP in developing rat hippocampal slices. Neuron 35, 535–545. doi:10.1016/s0896-6273(02)00785-7
Otmakhov, N., Tao-Cheng, J. H., Carpenter, S., Asrican, B., Dosemeci, A., Reese, T. S., et al. (2004). Persistent accumulation of calcium/calmodulin-dependent protein kinase II in dendritic spines after induction of NMDA receptor-dependent chemical long-term potentiation. J. Neurosci. 24, 9324–9331. doi:10.1523/JNEUROSCI.2350-04.2004
Pastalkova, E., Serrano, P., Pinkhasova, D., Wallace, E., Fenton, A., and Sacktor, T. C. (2006). Storage of spatial information by the maintenance mechanism of LTP. Science 313 (5790), 1141–1144. doi:10.1126/science.1128657
Pierce, J. P., Van Leyen, K., and McCarthy, J. B. (2000). Translocation machinery for synthesis of integral membrane and secretory proteins in dendritic spines. Nat. Neurosci. 3, 311–313. doi:10.1038/73868
Sacktor, T. C., Osten, P., Valsamis, H., Jiang, X., Naik, M. U., and Sublette, E. (1993). Persistent activation of the zeta isoform of protein kinase C in the maintenance of long-term potentiation. Proc. Natl. Acad. Sci. U. S. A. 90, 8342–8346. doi:10.1073/pnas.90.18.8342
Sajikumar, S., Navakkode, S., Sacktor, T. C., and Frey, J. U. (2005). Synaptic tagging and cross-tagging: The role of protein kinase mzeta in maintaining long-term potentiation but not long-term depression. J. Neurosci. 25, 5750–5756. doi:10.1523/JNEUROSCI.1104-05.2005
Sanhueza, M., McIntyre, C. C., and Lisman, J. E. (2007). Reversal of synaptic memory by Ca2+/calmodulin-dependent protein kinase II inhibitor. J. Neurosci. 27, 5190–5199. doi:10.1523/JNEUROSCI.5049-06.2007
Serrano, P., Friedman, E. L., Kenney, J., Taubenfield, S. M., Zimmerman, J. M., Hanna, J., et al. (2008). PKMzeta maintains spatial, instrumental, and classically conditioned long-term memories. PLoS Biol. 6 (12), 2698–2706. doi:10.1371/journal.pbio.0060318
Serrano, P., Yao, Y., and Sacktor, T. C. (2005). Persistent phosphorylation by protein kinase Mzeta maintains late-phase long-term potentiation. J. Neurosci. 25 (8), 1979–1984. doi:10.1523/JNEUROSCI.5132-04.2005
Shema, R., Sacktor, T. C., and Dudai, Y. (2007). Rapid erasure of long-term memory associations in the cortex by an inhibitor of PKM zeta. Science 317 (5840), 951–953. doi:10.1126/science.1144334
Smolen, P., Baxter, D. A., and Byrne, J. H. (2006). A model of the roles of essential kinases in the induction and expression of late long-term potentiation. Biophys. J. 90, 2760–2775. doi:10.1529/biophysj.105.072470
Soderling, T. (2000). CaM-kinases: Modulators of synaptic plasticity. Curr. Opin. Neurobiol. 10, 375–380. doi:10.1016/s0959-4388(00)00090-8
Stanton, P. K., and Sarvey, J. M. (1984). Blockade of long-term potentiation in rat hippocampal CA1 region by inhibitors of protein synthesis. J. Neurosci. 4, 3080–3088. doi:10.1523/JNEUROSCI.04-12-03080.1984
Tang, S. J., Reis, G., Kang, H., Gingras, A. C., Sonenberg, N., and Schuman, E. M. (2002). A rapamycin-sensitive signaling pathway contributes to long-term synaptic plasticity in the hippocampus. Proc. Natl. Acad. Sci. U. S. A. 99, 467–472. doi:10.1073/pnas.012605299
Toker, A., and Newton, A. C. (2000). Cellular signaling: Pivoting around PDK1. Cell 103, 185–188. doi:10.1016/s0092-8674(00)00110-0
Tsokas, P., Ma, T., Iyengar, R., Landau, E. M., and Blitzer, R. D. (2007). Mitogen-activated protein kinase upregulates the dendritic translation machinery in long-term potentiation by controlling the mammalian target of rapamycin pathway. J. Neurosci. 27 (22), 5885–5894. doi:10.1523/JNEUROSCI.4548-06.2007
Tyson, J. J., Chen, K. C., and Novak, B. (2003). Sniffers, buzzers, toggles and blinkers: Dynamics of regulatory and signaling pathways in the cell. Curr Opin. Cell Biol. 15, 221–231. doi:10.1016/s0955-0674(03)00017-6
Watson, J. D., Baker, T. A., Bell, S. P., Gann, A., Levine, M., and Losick, R. (2008). Molecular Biology of the gene. New York: Cold Spring Harbor Laboratory Press Cold Spring Harbor.
Yao, Y., Kelly, M. T., Sajikumar, S., Serrano, P., Tian, D., Bergold, P. J., et al. (2008). PKM zeta maintains late long-term potentiation by N-ethylmaleimide-sensitive factor/GluR2-dependent trafficking of postsynaptic AMPA receptors. J. Neurosci. 28 (31), 7820–7827. doi:10.1523/JNEUROSCI.0223-08.2008
Keywords: PKMζ, protein synthesis, protein degradation, memory, synaptic specificity
Citation: Aslam N (2023) Maintenance of PKMζ-modulated synaptic efficacies despite protein turnover. Front. Syst. Biol. 2:933938. doi: 10.3389/fsysb.2022.933938
Received: 05 July 2022; Accepted: 13 December 2022;
Published: 09 January 2023.
Edited by:
Rishikesh Narayanan, Indian Institute of Science (IISc), IndiaReviewed by:
Todd C.Sacktor, Downstate Health Sciences University, United StatesPanayiotis Tsokas, Downstate Health Sciences University, United States
Copyright © 2023 Aslam. This is an open-access article distributed under the terms of the Creative Commons Attribution License (CC BY). The use, distribution or reproduction in other forums is permitted, provided the original author(s) and the copyright owner(s) are credited and that the original publication in this journal is cited, in accordance with accepted academic practice. No use, distribution or reproduction is permitted which does not comply with these terms.
*Correspondence: Naveed Aslam, bmF2ZWVkLmFzbGFtQGJpb3N5c3RvbWljcy5jb20=