- 1Department of Chemical Engineering, University of Virginia, Charlottesville, VA, United States
- 2Department of Biomedical Engineering, University of Virginia, Charlottesville, VA, United States
- 3Department of Chemistry, University of Virginia, Charlottesville, VA, United States
- 4Department of Chemical and Biomolecular Engineering, University of Maryland, College Park, MD, United States
Life exists at an interface. One of the key characteristics of biological cells is compartmentalization, which is facilitated by lipids that create a water-impenetrable barrier to control transport of materials across the hydrophilic-hydrophobic interface. Microbial systems utilize a rich diversity of surfactants beyond lipids to adapt to an environmental niche, modify the properties of an interface, facilitate solubilization of nutrients for metabolism and as antimicrobials. As such, they are a fascinating class of biomolecules to study in terms of how effectiveness in an application or niche environment depends on sequence, structure and chemical properties. Moreover, there is increasing appreciation of the negative health and environmental impacts petrochemical-based surfactants can have, such as soil erosion and toxicity to plants and aquatic life, as well as the carbon footprint and associated greenhouse gas emissions associated with petrochemical surfactant manufacturing. In this review, we discuss the properties of biosurfactants and applications, and highlight key glycolipid-, protein- and peptide-based surfactants described in literature as examples of biosurfactants with unique potential and applications. As society looks towards the transition to a circular bioeconomy, we are excited by the potential of synthetic biology to develop new materials such as biosurfactants to facilitate this important transition.
1 Introduction
Solubility and interfacial interactions are a chemical challenge that impact nearly every sector, including food, medicine, and the environment (Shaban et al., 2020; Konwar, 2022; Nagtode et al., 2023). In many cases, insolubility and limited surface interactions function to decrease or prevent reaction activity from occurring. This is true for the case of oil-soluble and water-soluble compounds. In order to maximize conversion of the reaction between compounds in these two immiscible phases, the surface area of interaction between the two must be maximized. With consideration to the hydrophilic (water) and hydrophobic (oil) properties of the two compounds, amphiphilic molecules combine the properties of both. An amphiphilic molecule has a hydrophilic head group and a hydrophobic tail, creating a bridge between the hydrophilic and hydrophobic compounds. These molecules are often called surfactants, which reduce surface tension by adsorbing to interfaces and displacing interfacial water molecules back to the bulk phase (West, 2018).
The name “surfactant” itself is a portmanteau of surface-active agent, highlighting the role of these molecules at interfaces. A household example is soap, commonly sodium stearate, whose amphiphilic character functions to solubilize greasy molecules to be washed away with water. Some specific examples of synthetic surfactants commonly used in scientific laboratories or in industry are sodium dodecyl sulfate (SDS), TWEEN, and Triton-X (Domínguez et al., 2011; Parra et al., 2020; Knoch et al., 2021).
The hydrophilic head and hydrophobic tail of surfactants are both variable in terms of chemical structure. For example, the head of the surfactant can have different ionic charges ranging from anionic, to cationic, to nonionic, or even zwitterionic (W.-C. Chen et al., 2015). In the case of sodium stearate, the negative charge associated deprotonated stearic acid means it is an anionic surfactant. Not only can surfactants align across a flat interface, they also commonly form micelles (Figure 1). A micelle is a spherical group of surfactants with hydrophilic heads facing outwards and the hydrophobic tails occluded within the sphere. The propensity for micelle formation, and a measure of surfactant activity, is quantified as critical micelle concentration, or CMC, which will vary depending on the specific surfactant (Czajka et al., 2015). When micelles form, the hydrophilic head groups form hydrogen bonds with aqueous solvent, stabilizing the solution (Rana et al., 2017). Although CMC is a commonly used metric for surfactants, there is some concern regarding the stringency of its definition (Knoch et al., 2021), however, in the absence of a better alternative, we will compare CMCs in our discussion of emerging biosurfactants.
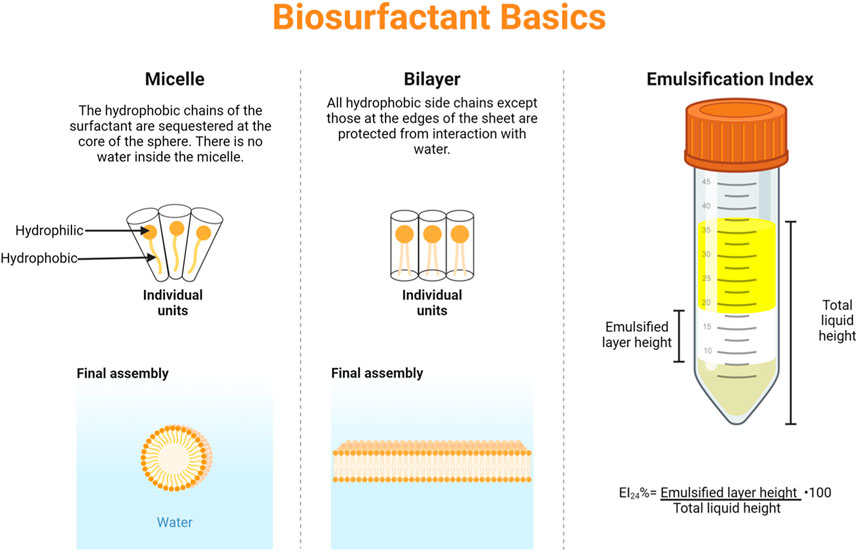
Figure 1. Biosurfactant basics–the common assemblies of micelles and bilayers are shown, along with a graphical explanation of emulsification index. Figure made with BioRender.
Currently, many synthetic surfactants are petroleum-derived, meaning that extensive chemistry with petroleum-based compounds is required to produce these small molecules (Czajka et al., 2015). Another class of common synthetic surfactants are poly- and perfluoroalkyl substances, which are nearly impossible to break down (Mann et al., 2022; Trinh et al., 2022). In both cases, the production and use of synthetic surfactants involve many hazardous and environmentally harmful chemicals at massive scales. A promising alternative is the use of biosurfactants, i.e., surfactants that are naturally occurring or derived from biology, which includes small-molecule, lipid- and protein-based molecules (Antonioli Júnior et al., 2022; Konwar, 2022). This review will present the current usage of biosurfactants including hydrophobin, and examine other promising emerging biosurfactants.
2 Current applications of biosurfactants
2.1 Research towards future food and consumer products
The food industry has an ever-growing need for surfactants as emulsifying agents, food additives, anti-adhesives, and antimicrobial agents, to improve food quality and extend shelf life. Synthetic surfactants may have all of these properties, but are often toxic, difficult to degrade, and lack stability in complex environments (Kiran et al., 2017). Therefore, biosurfactants are gaining popularity in many industries, including food and agriculture, due to their increased biodegradability, non-toxicity, stability in various temperatures, pHs, and salinity conditions, as well as their environmentally and economically friendly production methods (Kiran et al., 2017; Thraeib et al., 2022). Two popular biosurfactants are surfactin, a lipopeptide from Bacillus subtilis, and rhamnolipid, a glycolipid from Pseudomonas aeruginosa. Both have emulsifying properties, but more strikingly, also have the ability to inhibit adhesion of pathogenic bacteria, such as Listeria monocytogenes and Staphylococcus aureus which contributes to improvement of food quality and safety (Zezzi Do Valle Gomes and Nitschke, 2012). Additionally, biofilm-forming bacteria pose a large threat in the food industry; Kiran et al. describes lipopeptide MSA31 from Nesterenkonia sp. not only has anti-biofilm activity against S. aureus, but is also “halo-alkali and thermal tolerant” for optimal use in the food industry and is shown in the laboratory to improve the texture of muffins (Kiran et al., 2017). To add, another laboratory study has shown that an unidentified biosurfactant from Saccharomyces cerevisiae URM 6670 with emulsification and antioxidant properties was found to be a non-toxic and non-product altering food additive that has potential to serve as a replacement for egg yolk (Ribeiro et al., 2020). One challenge to the adoption of biosurfactants in the food industry may be uncertainty and fear of utilizing products from pathogenic bacteria such as P. aeruginosa. Addressing that concern, it has been discovered that non-pathogenic strains such as P. fluoroscens, P. chlororaphis, and Pseudomonas putida BD2 are also all able to produce rhamnolipds (Thangavel and Sridevi, 2015). Additionally, highlighting the use of rhamnolipids in industry, Evonik has recently begun commercial scale production of rhamnolipids for use in cleaning equipment used in food and consumer product manufacturing (Evonik Builds World’s First Industrial-Scale Production Plant for Rhamnolipids - Evonik Industries, 2024). Another way to alleviate the concerns surrounding biosurfactant production from pathogenic bacteria is to apply synthetic biology for the production of these biosurfactants in non-pathogenic hosts such as Escherichia coli. Interestingly, Kleetz, et al. show that E. coli can be engineered to produce non-native lipids via recombinant protein production (Kleetz et al., 2021). Alternatively, the protein-mediated production of biosurfactants via cell-free systems, such as those pioneered by the Jewett Lab, are promising for the high-throughput production of microbially-based molecules (Rasor et al., 2022). Biosurfactants serve as a natural, safe, and economically friendly approach to improving the needs of the food industry.
2.2 Medicine
The pharmaceutical industry similarly looks to biosurfactants for their desirable antimicrobial, anti-adhesive, antiviral, anticancer, anti-inflammatory, and immune system promoting properties that they possess (Inès and Dhouha, 2015; Bjerk et al., 2021; De Giani et al., 2021). Glycolipids, including the commonly known rhamnolipids produced by P. aeruginosa, have the ability to permeabilize bacterial cell membranes resulting in antibacterial effects, with the added benefit of likely being more biocompatible than synthetic surfactants (Inès and Dhouha, 2015). For example, Saravanakumari and Mani describe a xylolipid from probiotic bacteria Lactococcus lactis proven to be safe for oral and dermal use with antibacterial activity against pathogenic E. coli and S. aureus (Saravanakumari and Mani, 2010). De Giani, et al. provides a comprehensive review of other biosurfactants with antimicrobial activity that could be beneficial to human health (De Giani et al., 2021). In addition to antimicrobial use, some biosurfactants have shown promising anticancer effects. Sophorolipid, produced by yeast strain Wickerhamiella domercqiae, has been shown to cause morphological changes such as shrinkage and blebbing in H7402 human liver cells which ultimately leads to apoptosis supporting anticancer activity (Chen et al., 2006). With their aforementioned properties, biosurfactants offer a wide range of therapeutic potential and show promise for improving healthcare. As with the food industry, the origin of bacterially produced biosurfactants may be a barrier to their implementation and use. It is worth noting that recombinant protein expression, gene-knockouts, domestication of pathogenic bacteria, and other additional synthetic biology techniques may be helpful to increase acceptance and use of biosurfactants. For example, recombinant DNA technology and protein expression allows for the introduction of a biosurfactant gene sequence into a desired host organism for recombinant protein production (Jimoh et al., 2021). Studies have shown that incorporating the P. aeruginosa rhlAB operon into non-pathogenic hosts, such as P. putidia KT2440 and E. coli, serves as a safer production method for rhamnolipid (Setoodeh et al., 2014; Jimoh et al., 2021). To date, explicit implementation of synthetic biology to address these issues is a limited, but active area of research.
2.3 Environment
Pollutant sequestration with the use biosurfactants can be an effective method of bioremediation. Hydrocarbons that are difficult to degrade often accumulate in waste sites along with heavy metals. These pollutants can be extracted from soil with the use of biosurfactants, which effectively act to decrease surface tension and increase the solubility of contaminants (Ben Ayed et al., 2015). Some biosurfactants capable of pollutant degradation are produced by various bacteria including B. subtilis A21, P. aeruginosa PP3 and PP4, P. aeruginosa S5, B. subtilis B30, and others (Singh and Cameotra, 2013; Al-Wahaibi et al., 2014; Sun et al., 2019; Muthukumar et al., 2022; 2023). Biosurfactants including glycolipids, lipolipids, and rhamnolipids are particularly important for environmental applications as they reduce pollutants that would otherwise be added to existing contamination when using synthetic detergents or chemical solvents. This makes them environmentally sustainable solutions for removal of environmental contamination caused by hydrocarbons, heavy metals, oil, etc (Selva Filho et al., 2023).
Removal of polycyclic aromatic hydrocarbons (PAHs) from soil can be costly and ineffective due to the high hydrophobicity of PAHs and their strong affinity for soil (Singh and Cameotra, 2013). However, PAH removal can be facilitated by biosurfactants as discussed in Sun et al., where P. aeruginosa S5 was isolated from coking wastewater (Sun et al., 2019). Further examination of P. aeruginosa S5 showed the production of a glycolipid biosurfactant that decreased the surface tension enabling the enhanced the removal of PAHs (Ben Ayed et al., 2015). Similarly, Bezza and Chirwa identified biosurfactant producers Bacillus stratosphericus, B. subtilis, and Bacillus megaterium, whose biosurfactants increased the degradation potential of creosote in contaminated soil (Bezza and Chirwa, 2016). Conventional methods of remediation from oil spills generally yield a maximum of 15% degradation, but biosurfactants produced by P. aeruginosa often result in crude oil degradation of 50%–89% (Thavasi et al., 2011; Muthukumar et al., 2023). Likewise, Bacillus amyloliquefaciens An6 produces a biosurfactant with low toxicity that decreased the interfacial tension between diesel oil and water, enhancing bioavailability of diesel oil for degradation and making it a useful tool for bioremediation (Ben Ayed et al., 2015). Investigations of highly polluted soil continually reveal bacterial and fungal species that exhibit biosurfactant production (Singh and Cameotra, 2013; Janek et al., 2021; Yasmin et al., 2022), perhaps as a potential survival mechanism amongst hydrocarbon and heavy metal pollutants. Here, the evolution and adaptations of microbes for the natural production of biosurfactants may serve as a foundation for directed evolution or rationale-based design of improved or novel biosurfactants. This approach is discussed in further detail in Section 4, with directed evolution-derived biosurfactant MBSP1(Araújo et al., 2020).
3 Frequently used biosurfactant: hydrophobin
One family of protein biosurfactants called hydrophobins has been extensively studied for their wide-reaching applications (Berger and Sallada, 2019). Hydrophobins are small proteins (<20 kDa) with high surface activity secreted by filamentous fungi for a variety of vital functions (Wösten, 2001). Biosurfactant activity is created by an amphiphilic structure stabilized by four disulfide bonds among eight conserved cysteine residues in a characteristic pattern (Hakanpää et al., 2004). This family can be further divided into two classes based on self-assembly structures at air-water interfaces: Class I hydrophobins create amyloid-like fibrils independent of disulfide stability, while Class II hydrophobins create highly ordered films when disulfide bonds are intact (Sallada et al., 2018; Paananen et al., 2021). Naturally occurring hydrophobins within each class are very structurally similar with sequence variations indicative of uniquely evolved application (Hakanpää et al., 2004). This genetic flexibility has led to many engineered applications for hydrophobin in industry (Akanbi et al., 2010; Wang et al., 2017).
The pharmaceutical industry in particular has capitalized on the versatility of hydrophobins for drug delivery. Water-insoluble drugs such as cyclosporine A and nifedipine can be suspended in aqueous solution and made up to 500% more bioavailable by introduction of the hydrophobin SC3 (Akanbi et al., 2010). Fang et al. utilized Class I hydrophobin HPB to solubilize the lipophilic chemotherapy drug docetaxel, demonstrating high drug loading with delayed drug release (Fang et al., 2014). Valo et al. similarly suspended an insoluble corticosteroid in aqueous solution with a GFP-fused hydrophobin HFBII, allowing further study of the robust self-assembled micelle structure with fluorescence imaging (Valo et al., 2010). The retained functionality of these fusion proteins opens the door for various other surface modifications (Valo et al., 2010). For example, itraconazole was solubilized in micelles of synthesized hydrophobin HFBI fused to two cellulose binding domains and stabilized for 10 months by binding onto cellulose nanofibrils for storage (Valo et al., 2011). Chimeric hydrophobins have also been applied, seen in work by Vejnovic et al. improving permeability of terbinafine through human nails via a hydrophobin blending the N-terminal section of Class I hydrophobin SC3 with the C-terminal section of Class II hydrophobin HFBII (Vejnovic et al., 2010). Even without forming micelles, hydrophobins can be used to inhibit crystallization of drugs such as flufenamic acid (Sallada et al., 2021). The disulfide-dependent amphiphilic structure of hydrophobins can even be intended for intentional compromise in vivo as a drug release mechanism, as demonstrated by hydrophobin HFBII-stabilized gold nanoparticles created by Maiolo et al. to release drug payloads upon disulfide reduction by cytoplasmic glutathione (Maiolo et al., 2017).
The amphiphilic structure of hydrophobins has been utilized for wettability and dispersion applications outside of pharmaceuticals. Hydrophobins SC3 and HFBII, representatives of Class I and Class II respectively, were both shown to assemble on Teflon surfaces drastically reducing water contact angle, as well as facilitate wet-in of Teflon particles into water (Lumsdon et al., 2005). Reger and Hoffmann utilized hydrophobin HPB as an emulsifier, stabilizing boehmite particles in a toothpaste-like emulsion for use in cosmetics (Reger and Hoffmann, 2012). Water-dispersible suspensions of graphene and graphite can be created in one step for electrochemical applications with hydrophobins, and engineered hydrophobin variants can be substituted for modified surface properties (Laaksonen et al., 2010). As hydrophobin HFBII is not toxic, cytotoxic, or immunogenic (Shokribousjein et al., 2011), laboratory studies have shown that it can be used in food products to stabilize foams for significantly longer times than traditionally used emulsifying agents like milk proteins (Cox et al., 2009). This biocompatibility allowed Zhang et al. to design bioactive poly (ɛ-caprolactone) grafts coated in self-assembled hydrophobin HFBI films as a scaffold for immobilized anti-CD31 antibody to promote endothelialization (Zhang et al., 2011). Similar immobilization strategies were used on polydimethylsiloxane (PDMS) for microfluidics applications (Hou et al., 2009; Wang et al., 2007). Wang et al. utilized hydrophobin HFBI to reverse the hydrophobicity of PDMS, creating a bioactive surface which could then be robustly patterned with chicken IgG for immunoassays (Wang et al., 2007). Hou et al. iteratively improved the stability of this model, instead utilizing hydrophobin HGFI to create wetted PDMS surfaces able to withstand hot SDS washes (Hou et al., 2009). This immobilization strategy has been used to create a wide array of biosensors and antimicrobial coatings stable in various conditions by engineering or selecting hydrophobins with specific enzyme-binding properties (Wang et al., 2017; Zhao et al., 2009).
Production and purification methods of hydrophobins are as widely varied and interesting as their applications. Fungal host strains which naturally produce hydrophobins can be genetically engineered to overproduce, such as a Trichoderma reesei strain containing three additional copies of the HFBII gene fermented by Bailey et al. (Bailey et al., 2002). Grown in lactose-enriched media, this strain is capable of producing 240 mg/L HFBII in 92 h, a five-fold increase over the single-copy parent strain (Bailey et al., 2002). Both E. coli and yeast expression systems have been utilized as well (Kirkland and Keyhani, 2011; Sallada et al., 2019; Winterburn et al., 2011). Kirkland et al. designed a recombinant hydrophobin mHyd2 for expression in E. coli, and produced yields from 7–10 mg/L in less than 24 h (Kirkland and Keyhani, 2011). Although fermentation times increase from hours to days, S. cerevisiae yeast can also be used to express high yields of hydrophobins without need of structural modification (Winterburn et al., 2011). Sallada et al. introduced chaperone co-expression to a multi-copy HFBI Pichia pastoris strain, generating a 30-fold increase in protein production (Sallada et al., 2019). Regardless of production method, purification of interfacially-active hydrophobins is a challenge of its own, as necessary air supply can create large amounts of hydrophobin-sequestering foam (Winterburn et al., 2011). Winterburn et al. employed foam fractionation as a method to capitalize on this surface activity, continuously harvesting hydrophobin-rich foam throughout fermentation (Winterburn et al., 2011). Utilization of a biofilm reactor instead of traditional bioreactors and shake flasks can improve hydrophobin recovery by keeping the air inlet above the liquid volume (Khalesi et al., 2014). Hydrophobins can also be extracted from culture supernatants via phase separation utilizing nonionic surfactants, then recovered out of the non-ionic surfactant phase by addition of alcohol (Linder et al., 2001). Instead of extraction, cultures of purification tagged hydrophobins can be purified through immobilized metal affinity chromatography (IMAC) and eluted with imidazole (Sallada, 2020).
4 Promising emerging biosurfactants
Many diverse fungi produce glycolipid biosurfactants under harsh or starvation conditions, and in combination with fungal hydrolases, chitinases, and glutinases are effective for biocontrol (Jezierska et al., 2018; Zadeh et al., 2018; Da Silva et al., 2021). Yeast strain P. churashimaenius OK96, originally isolated from sugarcane, can produce mannosylerythritol lipid (MEL), whose hydrophilic head and hydrophobic tail impart surface active and self-assembling properties (Arutchelvi et al., 2008), resulting in activity as an antitumor agent and usefulness as an emulsifier in various cosmetics (Morita et al., 2013). P. churashimaenius OK96 has also shown adaptability to different sole carbon sources, including cuttlefish oil, for the production of MEL (Morita et al., 2013). This adaptability is promising as a method for sustainable and economical production of useful biosurfactants with alternate carbon sources. While these biosurfactants have shown effectiveness, the implementation of synthetic biology tool and approaches may further improve their activity.
Other fungal species, including Fusarium, Penicillium, and Trichoderma have been identified as biosurfactant producers through exploratory research in regions of high biodiversity, such as the Amazon rainforest (Sena et al., 2018; Piegza et al., 2021; Chotard et al., 2022). Along with Mucor, Candida, and Klebsiella species, these biosurfactants are compared in Table 1. In some cases, such as with M. circinelloide, the emulsification index (66%) suggests high surface activity, while the CMC does not (1.5%) (Zadeh et al., 2018; Marques et al., 2019), suggesting that the active biosurfactant has unique structure or specificity and that further studies will be imperative. Furthermore, it may be illuminating to consider other factors, such as ease of production, for which case Mucor circelloide has a biosurfactant yield of 6 g/L whereas C. sphaerica yields 4.5 g/L when cultured on corn steep liquor (Marques et al., 2019). Interestingly, genome editing of Mucor circinelloide with a plasmid-free CRISPR-Cas9 system for the modification of metabolic pathways shows differential production of M. circinelloide natural products, thus suggesting that CRISPR-Cas9 targeting of the biosurfactant production pathway is feasible to increase biosurfactant yields even further (Nagy et al., 2017).
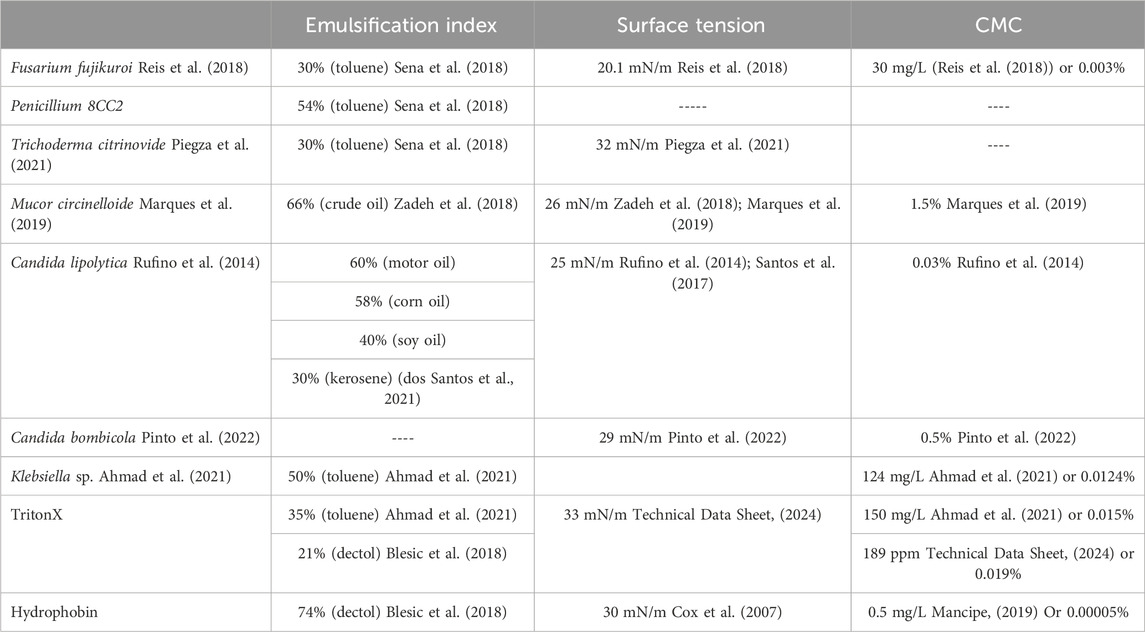
Table 1. A comparison of emulsification index, surface tension reduction, and CMC for emerging biosurfactants (most of which are unspecified as of yet), Triton-X, and hydrophobin. In cases where CMC was reported as mg/L, we performed a basic conversion to weight percent for ease of comparison.
Altogether, while many of these species have high biosurfactant activity in vivo, the targeted, recombinant production of biosurfactants in vitro may be faster and more economical.
One exciting biosurfactant that can be recombinantly produced in E. Coli., is the protein ranaspumin-2 (RSN-2). RSN-2 is one of six proteins that comprise foam nests of the túngara frog (Fleming et al., 2009). RSN-2 has an amphiphilic amino acid sequence and simple tertiary structure, where-in the C-terminal is analogous to the hydrophilic head of a surfactant, and the N-terminal analogous to the hydrophobic tail, protecting the eggs or sperm of the frog (Fleming et al., 2009). Work done by Morris et. al. confirmed a clam-shell model of adsorption at the interface and a two-step absorption process (Morris et al., 2016). Molecular dynamic simulations show that the removal of the hydrophobic N-terminus inhibits the protein from adsorbing to the interface while deleting the hydrophilic C-terminus only affected adsorption of RSN-2 in one scenario (Brandani et al., 2017). This suggests that the N-terminus is critical for adsorption while the C-terminus may aid in orienting the protein properly at the surface (Brandani et al., 2017). Another intriguing application of RSN-2 involves using it as a fusion tag for the foam fractionation of downstream enzyme processing (Krause et al., 2022).
Ranasmurfin is a protein similar to RSN-2, and is a biofoam produced by the Polypedates leucomystax tropical frog to protect their offspring (Oke et al., 2008; Cooper et al., 2017). The protein has a novel cross-link between two subunits of indophenol-like groups, which may cause the protein to have a blue color upon binding with zinc (Oke et al., 2008; Cooper et al., 2017). These groups could serve as targets for rational modification with the goal of modulating detection of other transition metals via coordinated cross-links. Oke, et al. postulate that RSN-2 and Ranasmurfin likely evolved in different and independent phylogenetic lineages due to their limited amino acid similarity. In spite of this, both these frog foams are able to protect embryonic staged eggs in harsh environments from many microbes, suggesting that these foams may have potential medical applications with anti-microbial properties.
Latherin is a mammalian produced biosurfactant protein found in the sweat and saliva of horses, that aids in evaporative cooling and acts as a microbial agent (McDonald et al., 2009). Latherin produces a significant reduction in water surface tension at low concentrations (less than 1 mg/mL). Structurally it has a predominantly polar outer surface with the non-polar residues buried. At the air-water interface the protein self-assembles to form a 10 Å layer which contributes its detergent-like properties (McDonald et al., 2009). Latherin represents the first mammalian surfactant protein with a known mechanism of action and structure, having potential uses in veterinary and medical science as well as nanotechnology processes (Vance et al., 2013).
In a completely genomic approach, MBSP1 is a biosurfactant protein derived from a metagenomic library derived from a soil sample taken from the Jundiaí River in Brazil. The protein of interest was shown to have a high similarity to hypothetical proteins from the Halobacteriaceae family. This protein has the ability to emulsify toluene and xylene, and yields positive results for drop collapse and oil dispersion assays (Araújo et al., 2020). The emulsification index, the height of the emulsion layer after a 24 h rest period in comparison to the original height of the liquid column (Figure 1), of purified MBSP1 with substrates including toluene, kerosene, and diesel was greater than 50%, with further studies showing long-term emulsion behavior even after 1 year (Araújo et al., 2020). MBSP1 is a promising example of implementing synthetic biology techniques to create novel biosurfactants, and similar strategies will likely have equally promising results.
As society continues to look for green alternatives to petrochemical surfactants, companies like Evonik, Locus Fermentation Solutions, BASF, AGAE Technologies, Glycosurf, Tensiogreen, Stepan Company, Holiferm, TeeGene Biotech Ltd., TARA Biologics Ltd., and Jeneil Biotech aim to produce biosurfactants as alternatives (BIOSURFACTANTS BY EVONIK ENTERING A NEW ERA OF SURFACTANTS, 2024; THE BIOSURFACTANT COMPANY ENABLING AN ECONOMIC TRANSITION TO A CLEANER WORLD, 2024). Additionally, as more biosurfactants are discovered and implemented in industrial processes, there is always room for improvement and diversification. Using synthetic biology tools, current biosurfactants can be adapted to improve their performance in their current roles as well as expanding their application to more diverse fields that also rely on chemical surfactants.
In conclusion, this mini-review has discussed the properties of key glycolipid-, protein-, and peptide-based biosurfactants described in literature along with their unique potential in a society moving towards a circular bioeconomy. This mini-review highlights the potential of synthetic biology to develop new materials such as biosurfactants to facilitate this important transition.
Author contributions
TV: Conceptualization, Data curation, Formal Analysis, Investigation, Project administration, Supervision, Writing–original draft, Writing–review and editing. SF: Conceptualization, Data curation, Formal Analysis, Investigation, Writing–original draft, Writing–review and editing. WF: Conceptualization, Data curation, Formal Analysis, Investigation, Writing–original draft, Writing–review and editing. MK: Conceptualization, Data curation, Formal Analysis, Investigation, Writing–original draft, Writing–review and editing. AV: Conceptualization, Data curation, Formal Analysis, Investigation, Writing–original draft, Writing–review and editing. AJ: Conceptualization, Data curation, Formal Analysis, Investigation, Writing–original draft, Writing–review and editing. SJ: Conceptualization, Data curation, Formal Analysis, Investigation, Writing–original draft, Writing–review and editing. BB: Conceptualization, Data curation, Formal Analysis, Funding acquisition, Investigation, Project administration, Supervision, Writing–original draft, Writing–review and editing.
Funding
The author(s) declare that financial support was received for the research, authorship, and/or publication of this article. This work was supported in part by the National Science Foundation (CBET 2211060, DMR 2050867), US Department of Agriculture (423671-19201) and research grants from Trulieve and the Jefferson Trust.
Acknowledgments
The authors wish to thank Madison Mann for feedback in preparing this manuscript.
Conflict of interest
The authors declare that the research was conducted in the absence of any commercial or financial relationships that could be construed as a potential conflict of interest.
The author(s) declared that they were an editorial board member of Frontiers, at the time of submission. This had no impact on the peer review process and the final decision.
Publisher’s note
All claims expressed in this article are solely those of the authors and do not necessarily represent those of their affiliated organizations, or those of the publisher, the editors and the reviewers. Any product that may be evaluated in this article, or claim that may be made by its manufacturer, is not guaranteed or endorsed by the publisher.
References
Ahmad, Z., Zhang, X., Imran, M., Zhong, H., Andleeb, S., Zulekha, R., et al. (2021). Production, functional stability, and effect of rhamnolipid biosurfactant from Klebsiella sp. on phenanthrene degradation in various medium systems. Ecotoxicol. Environ. Saf. 207, 111514. doi:10.1016/j.ecoenv.2020.111514
Akanbi, M. H. J., Post, E., Meter-Arkema, A., Rink, R., Robillard, G. T., Wang, X., et al. (2010). Use of hydrophobins in formulation of water insoluble drugs for oral administration. Colloids Surfaces. B, Biointerfaces 75 (2), 526–531. doi:10.1016/j.colsurfb.2009.09.030
Al-Wahaibi, Y., Joshi, S., Al-Bahry, S., Elshafie, A., Al-Bemani, A., and Shibulal, B. (2014). Biosurfactant production by Bacillus subtilis B30 and its application in enhancing oil recovery. Colloids Surfaces B Biointerfaces 114, 324–333. doi:10.1016/j.colsurfb.2013.09.022
Antonioli Júnior, R., Poloni, J. D. F., Pinto, É. S. M., and Dorn, M. (2022). Interdisciplinary overview of lipopeptide and protein-containing biosurfactants. Genes 14 (1), 76. doi:10.3390/genes14010076
Araújo, S. C. da S., Silva-Portela, R. C. B., de Lima, D. C., da Fonsêca, M. M. B., Araújo, W. J., da Silva, U. B., et al. (2020). MBSP1: a biosurfactant protein derived from a metagenomic library with activity in oil degradation. Sci. Rep. 10, 1340. doi:10.1038/s41598-020-58330-x
Arutchelvi, J. I., Bhaduri, S., Uppara, P. V., and Doble, M. (2008). Mannosylerythritol lipids: a review. J. Industrial Microbiol. Biotechnol. 35 (12), 1559–1570. doi:10.1007/s10295-008-0460-4
Bailey, M. J., Askolin, S., Hörhammer, N., Tenkanen, M., Linder, M., Penttilä, M., et al. (2002). Process technological effects of deletion and amplification of hydrophobins I and II in transformants of Trichoderma reesei. Appl. Microbiol. Biotechnol. 58 (6), 721–727. doi:10.1007/s00253-002-0966-z
Ben Ayed, H., Jemil, N., Maalej, H., Bayoudh, A., Hmidet, N., and Nasri, M. (2015). Enhancement of solubilization and biodegradation of diesel oil by biosurfactant from Bacillus amyloliquefaciens An6. Int. Biodeterior. Biodegrad. 99, 8–14. doi:10.1016/j.ibiod.2014.12.009
Berger, B. W., and Sallada, N. D. (2019). Hydrophobins: multifunctional biosurfactants for interface engineering. J. Biol. Eng. 13 (1), 10. doi:10.1186/s13036-018-0136-1
Bezza, F. A., and Chirwa, E. M. N. (2016). Biosurfactant-enhanced bioremediation of aged polycyclic aromatic hydrocarbons (PAHs) in creosote contaminated soil. Chemosphere 144, 635–644. doi:10.1016/j.chemosphere.2015.08.027
BIOSURFACTANTS BY EVONIK ENTERING A NEW ERA OF SURFACTANTS (2023). Evonik. Available at: https://household-care.evonik.com/en/products/biosurfactants (Retrieved September 12, 2023).
Bjerk, T. R., Severino, P., Jain, S., Marques, C., Silva, A. M., Pashirova, T., et al. (2021). Biosurfactants: properties and applications in drug delivery, biotechnology and ecotoxicology. Bioengineering 8 (8), 115. doi:10.3390/bioengineering8080115
Blesic, M., Dichiarante, V., Milani, R., Linder, M., and Metrangolo, P. (2018). Evaluating the potential of natural surfactants in the petroleum industry: the case of hydrophobins. Pure Appl. Chem. 90 (2), 305–314. doi:10.1515/pac-2017-0703
Brandani, G. B., Vance, S. J., Schor, M., Cooper, A., Kennedy, M. W., Smith, B. O., et al. (2017). Adsorption of the natural protein surfactant Rsn-2 onto liquid interfaces. Phys. Chem. Chem. Phys. 19 (12), 8584–8594. doi:10.1039/C6CP07261E
Chen, J., Song, X., Zhang, H., Qu, Y., and Miao, J. (2006). Sophorolipid produced from the new yeast strain Wickerhamiella domercqiae induces apoptosis in H7402 human liver cancer cells. Appl. Microbiol. Biotechnol. 72 (1), 52–59. doi:10.1007/s00253-005-0243-z
Chen, W.-C., Juang, R.-S., and Wei, Y.-H. (2015). Applications of a lipopeptide biosurfactant, surfactin, produced by microorganisms. Biochem. Eng. J. 103, 158–169. doi:10.1016/j.bej.2015.07.009
Chotard, M., Mounier, J., Meye, R., Padel, C., Claude, B., Nehmé, R., et al. (2022). Biosurfactant-producing mucor strains: selection, screening, and chemical characterization. Appl. Microbiol. 2 (1), 248–259. doi:10.3390/applmicrobiol2010018
Cooper, A., Vance, S. J., Smith, B. O., and Kennedy, M. W. (2017). Frog foams and natural protein surfactants. Colloids Surfaces A Physicochem. Eng. Aspects 534, 120–129. doi:10.1016/j.colsurfa.2017.01.049
Cox, A. R., Aldred, D. L., and Russell, A. B. (2009). Exceptional stability of food foams using class II hydrophobin HFBII. Food Hydrocoll. 23 (2), 366–376. doi:10.1016/j.foodhyd.2008.03.001
Cox, A. R., Cagnol, F., Russell, A. B., and Izzard, M. J. (2007). Surface properties of class II hydrophobins from Trichoderma reesei and influence on bubble stability. Langmuir 23 (15), 7995–8002. doi:10.1021/la700451g
Czajka, A., Hazell, G., and Eastoe, J. (2015). Surfactants at the design limit. Langmuir 31 (30), 8205–8217. doi:10.1021/acs.langmuir.5b00336
Da Silva, A. F., Banat, I. M., Giachini, A. J., and Robl, D. (2021). Fungal biosurfactants, from nature to biotechnological product: bioprospection, production and potential applications. Bioprocess Biosyst. Eng. 44 (10), 2003–2034. doi:10.1007/s00449-021-02597-5
De Giani, A., Zampolli, J., and Di Gennaro, P. (2021). Recent trends on biosurfactants with antimicrobial activity produced by bacteria associated with human health: different perspectives on their properties, challenges, and potential applications. Front. Microbiol. 12, 655150. doi:10.3389/fmicb.2021.655150
Domínguez, H. (2011). Structural transition of the sodium dodecyl sulfate (SDS) surfactant induced by changes in surfactant concentrations. J. Phys. Chem. B 115 (43), 12422–12428. doi:10.1021/jp202813b
dos Santos, J. C. V., Santos Emilia, M. da S., da Silva Yali, A., Lira, I. R. A. da S., Raianny Silva, R., Durval, I. J. B., et al. (2021). Application of Candida lipolytica biosurfactant for bioremediation of motor oil from contaminated environment. Chem. Eng. Trans. 86, 649–654. doi:10.3303/CET2186109
Evonik builds world’s first industrial-scale production plant for rhamnolipids—Evonik Industries (2023). Evonik builds world’s first industrial-scale production plant for rhamnolipids—Evonik Industries. Available at: https://corporate.evonik.com/en/investor-relations/evonik-builds-worlds-first-industrial-scale-production-plant-for-rhamnolipids-168524.html (Retrieved November 20, 2023).
Fang, G., Tang, B., Liu, Z., Gou, J., Zhang, Y., Xu, H., et al. (2014). Novel hydrophobin-coated docetaxel nanoparticles for intravenous delivery: in vitro characteristics and in vivo performance. Eur. J. Pharm. Sci. 60, 1–9. doi:10.1016/j.ejps.2014.04.016
Fleming, R. I., Mackenzie, C. D., Cooper, A., and Kennedy, M. W. (2009). Foam nest components of the túngara frog: a cocktail of proteins conferring physical and biological resilience. Proc. R. Soc. B Biol. Sci. 276 (1663), 1787–1795. doi:10.1098/rspb.2008.1939
Hakanpää, J., Paananen, A., Askolin, S., Nakari-Setälä, T., Parkkinen, T., Penttilä, M., et al. (2004). Atomic resolution structure of the HFBII hydrophobin, a self-assembling amphiphile. J. Biol. Chem. 279 (1), 534–539. doi:10.1074/jbc.M309650200
Hou, S., Li, X., Li, X., Feng, X.-Z., Wang, R., Wang, C., et al. (2009). Surface modification using a novel type I hydrophobin HGFI. Anal. Bioanal. Chem. 394 (3), 783–789. doi:10.1007/s00216-009-2776-y
Inès, M., and Dhouha, G. (2015). Glycolipid biosurfactants: potential related biomedical and biotechnological applications. Carbohydr. Res. 416, 59–69. doi:10.1016/j.carres.2015.07.016
Janek, T., Gudiña, E. J., Połomska, X., Biniarz, P., Jama, D., Rodrigues, L. R., et al. (2021). Sustainable surfactin production by Bacillus subtilis using crude glycerol from different wastes. Molecules 26 (12), 3488. doi:10.3390/molecules26123488
Jezierska, S., Claus, S., and Van Bogaert, I. (2018). Yeast glycolipid biosurfactants. FEBS Lett. 592 (8), 1312–1329. doi:10.1002/1873-3468.12888
Jimoh, A. A., Senbadejo, T. Y., Adeleke, R., and Lin, J. (2021). Development and genetic engineering of hyper-producing microbial strains for improved synthesis of biosurfactants. Mol. Biotechnol. 63 (4), 267–288. doi:10.1007/s12033-021-00302-1
Khalesi, M., Zune, Q., Telek, S., Riveros, S., Verachtert, H., Toye, D., et al. (2014). Fungal biofilm reactor improves the productivity of hydrophobin HFBII. Biochem. Eng. J. 88, 171–178. doi:10.1016/j.bej.2014.05.001
Kiran, G. S., Priyadharsini, S., Sajayan, A., Priyadharsini, G. B., Poulose, N., and Selvin, J. (2017). Production of lipopeptide biosurfactant by a marine Nesterenkonia sp. and its application in food industry. Front. Microbiol. 8, 1138. doi:10.3389/fmicb.2017.01138
Kirkland, B. H., and Keyhani, N. O. (2011). Expression and purification of a functionally active class I fungal hydrophobin from the entomopathogenic fungus Beauveria bassiana in E. coli. J. Industrial Microbiol. Biotechnol. 38 (2), 327–335. doi:10.1007/s10295-010-0777-7
Kleetz, J., Vasilopoulos, G., Czolkoss, S., Aktas, M., and Narberhaus, F. (2021). Recombinant and endogenous ways to produce methylated phospholipids in Escherichia coli. Appl. Microbiol. Biotechnol. 105 (23), 8837–8851. doi:10.1007/s00253-021-11654-8
Knoch, H., Ulbrich, M. H., Mittag, J. J., Buske, J., Garidel, P., and Heerklotz, H. (2021). Complex micellization behavior of the polysorbates tween 20 and tween 80. Mol. Pharm. 18 (8), 3147–3157. doi:10.1021/acs.molpharmaceut.1c00406
Konwar, B. K. (2022). Bacterial biosurfactants: isolation, purification, characterization, and industrial applications. 1st ed. Apple Academic Press. doi:10.1201/9781003188131
Krause, T., Keshavarzi, B., Dressel, J., Heitkam, S., and Ansorge-Schumacher, M. B. (2022). Rsn-2-mediated directed foam enrichment of β-lactamase. Biotechnol. J. 17 (12), 2200271. doi:10.1002/biot.202200271
Laaksonen, P., Kainlauri, M., Laaksonen, T., Shchepetov, A., Jiang, H., Ahopelto, J., et al. (2010). Interfacial engineering by proteins: exfoliation and functionalization of graphene by hydrophobins. Angew. Chem. Int. Ed. 49 (29), 4946–4949. doi:10.1002/anie.201001806
Linder, M., Selber, K., Nakari-Setälä, T., Qiao, M., Kula, M. R., and Penttilä, M. (2001). The hydrophobins HFBI and HFBII from Trichoderma reesei showing efficient interactions with nonionic surfactants in aqueous two-phase systems. Biomacromolecules 2 (2), 511–517. doi:10.1021/bm0001493
Lumsdon, S. O., Green, J., and Stieglitz, B. (2005). Adsorption of hydrophobin proteins at hydrophobic and hydrophilic interfaces. Colloids Surfaces B Biointerfaces 44 (4), 172–178. doi:10.1016/j.colsurfb.2005.06.012
Maiolo, D., Pigliacelli, C., Sánchez Moreno, P., Violatto, M. B., Talamini, L., Tirotta, I., et al. (2017). Bioreducible hydrophobin-stabilized supraparticles for selective intracellular release. ACS Nano 11 (9), 9413–9423. doi:10.1021/acsnano.7b04979
Mancipe, N. C. (2019). Design and Production of HighPerformance hydrophobin surfactant proteins Using a dual-domain fusion strategy [university of Minnesota]. Available at: https://hdl.handle.net/11299/202101.
Mann, M. M., Tang, J. D., and Berger, B. W. (2022). Engineering human liver fatty acid binding protein for detection of poly- and perfluoroalkyl substances. Biotechnol. Bioeng. 119 (2), 513–522. doi:10.1002/bit.27981
Marques, N. S. A. do A., Silva, T. A. L. e., Andrade, R. F. da S., Júnior, J. F. B., Okada, K., and Takaki, G. M. C. (2019). Lipopeptide biosurfactant produced by Mucor Circinelloides UCP/WFCC 0001 applied in the removal of crude oil and engine oil from soil. Acta Sci. Technol. 41. Available at: https://www.redalyc.org/journal/3032/303260200014/html/.
McDonald, R. E., Fleming, R. I., Beeley, J. G., Bovell, D. L., Lu, J. R., Zhao, X., et al. (2009). Latherin: a surfactant protein of horse sweat and saliva. PLoS ONE 4 (5), e5726. doi:10.1371/journal.pone.0005726
Morita, T., Kawamura, D., Morita, N., Fukuoka, T., Imura, T., Sakai, H., et al. (2013). Characterization of mannosylerythritol lipids containing hexadecatetraenoic acid produced from cuttlefish oil by Pseudozyma churashimaensis OK96. J. Oleo Sci. 62 (5), 319–327. doi:10.5650/jos.62.319
Morris, R. J., Brandani, G. B., Desai, V., Smith, B. O., Schor, M., and MacPhee, C. E. (2016). The conformation of interfacially adsorbed ranaspumin-2 is an arrested state on the unfolding pathway. Biophysical J. 111 (4), 732–742. doi:10.1016/j.bpj.2016.06.006
Muthukumar, B., Al Salhi, M. S., Narenkumar, J., Devanesan, S., Tentu, N. R., Kim, W., et al. (2022). Characterization of two novel strains of Pseudomonas aeruginosa on biodegradation of crude oil and its enzyme activities. Environ. Pollut. 304, 119223. doi:10.1016/j.envpol.2022.119223
Muthukumar, B., Surya, S., Sivakumar, K., AlSalhi, M. S., Rao, T. N., Devanesan, S., et al. (2023). Influence of bioaugmentation in crude oil contaminated soil by Pseudomonas species on the removal of total petroleum hydrocarbon. Chemosphere 310, 136826. doi:10.1016/j.chemosphere.2022.136826
Nagtode, V. S., Cardoza, C., Yasin, H. K. A., Mali, S. N., Tambe, S. M., Roy, P., et al. (2023). Green surfactants (biosurfactants): a petroleum-free substitute for Sustainability─Comparison, applications, market, and future prospects. ACS Omega 8 (13), 11674–11699. doi:10.1021/acsomega.3c00591
Nagy, G., Szebenyi, C., Csernetics, Á., Vaz, A. G., Tóth, E. J., Vágvölgyi, C., et al. (2017). Development of a plasmid free CRISPR-Cas9 system for the genetic modification of Mucor circinelloides. Sci. Rep. 7 (1), 16800. doi:10.1038/s41598-017-17118-2
Oke, M., Ching, R. T. Y., Carter, L. G., Johnson, K. A., Liu, H., McMahon, S. A., et al. (2008). Unusual chromophore and cross-links in Ranasmurfin: a blue protein from the foam nests of a tropical frog. Angew. Chem. Int. Ed. 47 (41), 7853–7856. doi:10.1002/anie.200802901
Paananen, A., Weich, S., Szilvay, G. R., Leitner, M., Tappura, K., and Ebner, A. (2021). Quantifying biomolecular hydrophobicity: single molecule force spectroscopy of class II hydrophobins. J. Biol. Chem. 296, 100728. doi:10.1016/j.jbc.2021.100728
Parra, J. G., Iza, P., Dominguez, H., Schott, E., and Zarate, X. (2020). Effect of Triton X-100 surfactant on the interfacial activity of ionic surfactants SDS, CTAB and SDBS at the air/water interface: a study using molecular dynamic simulations. Colloids Surfaces A Physicochem. Eng. Aspects 603, 125284. doi:10.1016/j.colsurfa.2020.125284
Piegza, M., Szura, K., and Łaba, W. (2021). Trichoderma citrinoviride: anti-fungal biosurfactants production characteristics. Front. Bioeng. Biotechnol. 9, 778701. doi:10.3389/fbioe.2021.778701
Pinto, M. I. S., Campos Guerra, J. M., Meira, H. M., Sarubbo, L. A., and De Luna, J. M. (2022). A biosurfactant from Candida bombicola: its synthesis, characterization, and its application as a food emulsions. Foods 11 (4), 561. doi:10.3390/foods11040561
Rana, S., Bhattacharjee, J., Barick, K., Verma, G., Hassan, P., and Yakhmi, J. (2017). Interfacial engineering of nanoparticles for cancer therapeutics. Nanostructures Cancer Ther., 177–209. doi:10.1016/b978-0-323-46144-3.00007-6
Rasor, B. J., Vögeli, B., Jewett, M. C., and Karim, A. S. (2022). “Cell-free protein synthesis for high-throughput biosynthetic pathway prototyping,” in Cell-free gene expression. Editors A. S. Karim,, and M. C. Jewett (Springer US), 2433, 199–215. doi:10.1007/978-1-0716-1998-8_12
Reger, M., and Hoffmann, H. (2012). Hydrophobin coated boehmite nanoparticles stabilizing oil in water emulsions. J. Colloid Interface Sci. 368 (1), 378–386. doi:10.1016/j.jcis.2011.10.050
Reis, C. B. L. D., Morandini, L. M. B., Bevilacqua, C. B., Bublitz, F., Ugalde, G., Mazutti, M. A., et al. (2018). First report of the production of a potent biosurfactant with α,β-trehalose by Fusarium fujikuroi under optimized conditions of submerged fermentation. Braz. J. Microbiol. 49, 185–192. doi:10.1016/j.bjm.2018.04.004
Ribeiro, B. G., Guerra, J. M. C., and Sarubbo, L. A. (2020). Potential food application of a biosurfactant produced by Saccharomyces cerevisiae URM 6670. Front. Bioeng. Biotechnol. 8, 434. doi:10.3389/fbioe.2020.00434
Rufino, R. D., De Luna, J. M., De Campos Takaki, G. M., and Sarubbo, L. A. (2014). Characterization and properties of the biosurfactant produced by Candida lipolytica UCP 0988. Electron. J. Biotechnol. 17 (1), 34–38. doi:10.1016/j.ejbt.2013.12.006
Sallada, N., Li, Y., Berger, B., and Lamm, M. S. (2021). Engineered hydrophobin as a crystallization inhibitor for flufenamic acid. ACS Appl. Bio Mater. 4 (8), 6441–6450. doi:10.1021/acsabm.1c00612
Sallada, N. D. (2020). Overproduction and initial characterization of a class II hydrophobin fusion protein heterologously produced in Pichia pastoris.
Sallada, N. D., Dunn, K. J., and Berger, B. W. (2018). A structural and functional role for disulfide bonds in a class II hydrophobin. Biochemistry 57 (5), 645–653. doi:10.1021/acs.biochem.7b01166
Sallada, N. D., Harkins, L. E., and Berger, B. W. (2019). Effect of gene copy number and chaperone coexpression on recombinant hydrophobin HFBI biosurfactant production in Pichia pastoris. Biotechnol. Bioeng. 116 (8), 2029–2040. doi:10.1002/bit.26982
Santos, D. K. F., Meira, H. M., Rufino, R. D., Luna, J. M., and Sarubbo, L. A. (2017). Biosurfactant production from Candida lipolytica in bioreactor and evaluation of its toxicity for application as a bioremediation agent. Process Biochem. 54, 20–27. doi:10.1016/j.procbio.2016.12.020
Saravanakumari, P., and Mani, K. (2010). Structural characterization of a novel xylolipid biosurfactant from Lactococcus lactis and analysis of antibacterial activity against multi-drug resistant pathogens. Bioresour. Technol. 101 (22), 8851–8854. doi:10.1016/j.biortech.2010.06.104
Selva Filho, A. A. P., Converti, A., Soares Da Silva, R. D. C. F., and Sarubbo, L. A. (2023). Biosurfactants as multifunctional remediation agents of environmental pollutants generated by the petroleum industry. Energies 16 (3), 1209. doi:10.3390/en16031209
Sena, H. H., Sanches, M. A., Rocha, D. F. S., Segundo, W. O. P. F., de Souza, É. S., and de Souza, J. V. B. (2018). Production of biosurfactants by soil fungi isolated from the Amazon forest. Int. J. Microbiol. 2018, 5684261. doi:10.1155/2018/5684261
Setoodeh, P., Jahanmiri, A., Eslamloueyan, R., Niazi, A., Ayatollahi, S. S., Aram, F., et al. (2014). Statistical screening of medium components for recombinant production of Pseudomonas aeruginosa ATCC 9027 rhamnolipids by nonpathogenic cell factory Pseudomonas putida KT2440. Mol. Biotechnol. 56 (2), 175–191. doi:10.1007/s12033-013-9693-1
Shaban, S. M., Kang, J., and Kim, D.-H. (2020). Surfactants: recent advances and their applications. Compos. Commun. 22, 100537. doi:10.1016/j.coco.2020.100537
Shokribousjein, Z., Deckers, S. M., Gebruers, K., Lorgouilloux, Y., Baggerman, G., Verachtert, H., et al. (2011). Hydrophobins, beer foaming and gushing. Cerevisia 35 (4), 85–101. doi:10.1016/j.cervis.2010.12.001
Singh, A. K., and Cameotra, S. S. (2013). Efficiency of lipopeptide biosurfactants in removal of petroleum hydrocarbons and heavy metals from contaminated soil. Environ. Sci. Pollut. Res. 20 (10), 7367–7376. doi:10.1007/s11356-013-1752-4
Sun, S., Wang, Y., Zang, T., Wei, J., Wu, H., Wei, C., et al. (2019). A biosurfactant-producing Pseudomonas aeruginosa S5 isolated from coking wastewater and its application for bioremediation of polycyclic aromatic hydrocarbons. Bioresour. Technol. 281, 421–428. doi:10.1016/j.biortech.2019.02.087
Technical Data Sheet (2024). Triton-X 100 surfactant [dataset]. Midland, Michigan: The Dow Chemical Company.
Thangavel, P.,, and Sridevi, G. (2015). Environmental sustainability: role of green Technologies (India: Springer). doi:10.1007/978-81-322-2056-5
Thavasi, R., Jayalakshmi, S., and Banat, I. M. (2011). Effect of biosurfactant and fertilizer on biodegradation of crude oil by marine isolates of Bacillus megaterium, Corynebacterium kutscheri and Pseudomonas aeruginosa. Bioresour. Technol. 102 (2), 772–778. doi:10.1016/j.biortech.2010.08.099
THE BIOSURFACTANT COMPANY ENABLING AN ECONOMIC TRANSITION TO A CLEANER WORLD (2023). Locus fermentation solutions. Available at: https://locusfs.com/(Retrieved September 12, 2023).
Thraeib, J. Z., Altemimi, A. B., Jabbar Abd Al-Manhel, A., Abedelmaksoud, T. G., El-Maksoud, A. A. A., Madankar, C. S., et al. (2022). Production and characterization of a bioemulsifier derived from microorganisms with potential application in the food industry. Life 12 (6), 924. doi:10.3390/life12060924
Trinh, V., Malloy, C. S., Durkin, T. J., Gadh, A., and Savagatrup, S. (2022). Detection of PFAS and fluorinated surfactants using differential behaviors at interfaces of complex droplets. ACS Sensors 7 (5), 1514–1523. doi:10.1021/acssensors.2c00257
Valo, H. K., Kovalainen, M., Laaksonen, P., Häkkinen, M., Auriola, S., Peltonen, L., et al. (2011). Immobilization of protein-coated drug nanoparticles in nanofibrillar cellulose matrices—enhanced stability and release. J. Control. Release 156 (3), 390–397. doi:10.1016/j.jconrel.2011.07.016
Valo, H. K., Laaksonen, P. H., Peltonen, L. J., Linder, M. B., Hirvonen, J. T., and Laaksonen, T. J. (2010). Multifunctional hydrophobin: toward functional coatings for drug nanoparticles. ACS Nano 4 (3), 1750–1758. doi:10.1021/nn9017558
Vance, S. J., McDonald, R. E., Cooper, A., Smith, B. O., and Kennedy, M. W. (2013). The structure of latherin, a surfactant allergen protein from horse sweat and saliva. J. R. Soc. Interface 10 (85), 20130453. doi:10.1098/rsif.2013.0453
Vejnovic, I., Huonder, C., and Betz, G. (2010). Permeation studies of novel terbinafine formulations containing hydrophobins through human nails in vitro. Int. J. Pharm. 397, 67–76. doi:10.1016/j.ijpharm.2010.06.051
Wang, R., Yang, Y.-L., Qin, M., Wang, L.-K., Yu, L., Shao, B., et al. (2007). Biocompatible hydrophilic modifications of poly(dimethylsiloxane) using self-assembled hydrophobins. Chem. Mater. 19 (13), 3227–3231. doi:10.1021/cm070445n
Wang, X., Mao, J., Chen, Y., Song, D., Gao, Z., Zhang, X., et al. (2017). Design of antibacterial biointerfaces by surface modification of poly (ε-caprolactone) with fusion protein containing hydrophobin and PA-1. Colloids Surfaces B Biointerfaces 151, 255–263. doi:10.1016/j.colsurfb.2016.12.019
West, A. (2018). “Intermolecular forces and solvation,” in Interface science and technology (Elsevier), 21, 49–130. doi:10.1016/B978-0-12-801970-2.00002-1
Winterburn, J. B., Russell, A. B., and Martin, P. J. (2011). Integrated recirculating foam fractionation for the continuous recovery of biosurfactant from fermenters. Biochem. Eng. J. 54 (2), 132–139. doi:10.1016/j.bej.2011.02.011
Wösten, H. A. B. (2001). Hydrophobins: multipurpose proteins. Annu. Rev. Microbiol. 55 (1), 625–646. doi:10.1146/annurev.micro.55.1.625
Yasmin, A., Aslam, F., and Fariq, A. (2022). Genetic evidences of biosurfactant production in two Bacillus subtilis strains MB415 and MB418 isolated from oil contaminated soil. Front. Bioeng. Biotechnol. 10, 855762. doi:10.3389/fbioe.2022.855762
Zadeh, P. H., Moghimi, H., and Hamedi, J. (2018). Biosurfactant production by Mucor circinelloides: environmental applications and surface-active properties. Eng. Life Sci. 18 (5), 317–325. doi:10.1002/elsc.201700149
Zezzi Do Valle Gomes, M., and Nitschke, M. (2012). Evaluation of rhamnolipid and surfactin to reduce the adhesion and remove biofilms of individual and mixed cultures of food pathogenic bacteria. Food control. 25 (2), 441–447. doi:10.1016/j.foodcont.2011.11.025
Zhang, M., Wang, Z., Wang, Z., Feng, S., Xu, H., Zhao, Q., et al. (2011). Immobilization of anti-CD31 antibody on electrospun poly(ɛ-caprolactone) scaffolds through hydrophobins for specific adhesion of endothelial cells. Colloids Surfaces B Biointerfaces 85 (1), 32–39. doi:10.1016/j.colsurfb.2010.10.042
Keywords: biosurfactant, lipoprotein, hydrophobin, emulsification, formulation, foam fractionation, antimicrobial, sustainability
Citation: Vigil TN, Felton SM, Fahy WE, Kinkeade MA, Visek AM, Janiga AR, Jacob SG and Berger BW (2024) Biosurfactants as templates to inspire new environmental and health applications. Front. Synth. Biol. 2:1303423. doi: 10.3389/fsybi.2024.1303423
Received: 28 September 2023; Accepted: 28 March 2024;
Published: 12 April 2024.
Edited by:
Pattanathu K. S. M. Rahman, Liverpool John Moores University, United KingdomReviewed by:
Stephen Robert Euston, Heriot-Watt University, United KingdomRajasekar Aruliah, Thiruvalluvar University, India
Copyright © 2024 Vigil, Felton, Fahy, Kinkeade, Visek, Janiga, Jacob and Berger. This is an open-access article distributed under the terms of the Creative Commons Attribution License (CC BY). The use, distribution or reproduction in other forums is permitted, provided the original author(s) and the copyright owner(s) are credited and that the original publication in this journal is cited, in accordance with accepted academic practice. No use, distribution or reproduction is permitted which does not comply with these terms.
*Correspondence: Bryan W. Berger, YndiMmtAdmlyZ2luaWEuZWR1