- Department of Pharmacology and Chemical Biology, University of Pittsburgh School of Medicine, Pittsburgh, PA, United States
Synaptic plasticity is a critical process that regulates neuronal activity by allowing neurons to adjust their synaptic strength in response to changes in activity. Despite the high proximity of excitatory glutamatergic and inhibitory GABAergic postsynaptic zones and their functional integration within dendritic regions, concurrent plasticity has historically been underassessed. Growing evidence for pathological disruptions in the excitation and inhibition (E/I) balance in neurological and neurodevelopmental disorders indicates the need for an improved, more “holistic” understanding of synaptic interplay. There continues to be a long-standing focus on the persistent strengthening of excitation (excitatory long-term potentiation; eLTP) and its role in learning and memory, although the importance of inhibitory long-term potentiation (iLTP) and depression (iLTD) has become increasingly apparent. Emerging evidence further points to a dynamic dialogue between excitatory and inhibitory synapses, but much remains to be understood regarding the mechanisms and extent of this exchange. In this mini-review, we explore the role calcium signaling and synaptic crosstalk play in regulating postsynaptic plasticity and neuronal excitability. We examine current knowledge on GABAergic and glutamatergic synapse responses to perturbances in activity, with a focus on postsynaptic plasticity induced by short-term pharmacological treatments which act to either enhance or reduce neuronal excitability via ionotropic receptor regulation in neuronal culture. To delve deeper into potential mechanisms of synaptic crosstalk, we discuss the influence of synaptic activity on key regulatory proteins, including kinases, phosphatases, and synaptic structural/scaffolding proteins. Finally, we briefly suggest avenues for future research to better understand the crosstalk between glutamatergic and GABAergic synapses.
Introduction
Ligand-gated ion channel GABA type A receptors (GABAARs) mediate the majority of fast inhibition in the central nervous system, while glutamatergic AMPA receptors (AMPARs) and NMDA receptors (NMDARs) collectively mediate fast excitatory neurotransmission. NMDARs particularly play a unique role in synaptic plasticity due to high calcium permeability and voltage-dependent Mg2+ block typically relieved by AMPAR-mediated depolarization. Slow inhibition and excitation are generated by G protein-coupled, GABA type B (GABABRs) and metabotropic glutamate receptors (mGluRs), respectively. The concerted action of these receptors balances neuronal excitability. A close and coordinated spatial relationship between glutamatergic and GABAergic synapses on dendrites (Megías et al., 2001; Bleckert et al., 2013; Iascone et al., 2020), sometimes as near as on the same spine (Chen et al., 2012), facilitates synaptic input integration, dynamic calcium regulation, synaptic crosstalk, and coregulation.
Synaptic plasticity describes the ability of synapses to adapt their relative strength based on the overall level of activity or specific activity patterns, often by dynamic regulation of receptor-synaptic scaffold interactions or through trafficking. During development, it is heavily involved in dendritic growth, synaptogenesis, and the formation of neural circuits (reviewed in Akgül and McBain, 2016; Ismail et al., 2017; Jenks et al., 2021). In mature neurons, synaptic plasticity is responsible for synapse remodeling during experience. Genetic mutations or pathology leading to altered excitatory or inhibitory neurotransmission or impaired synaptogenesis typically result in deficits in synaptic plasticity, a common feature in neurodevelopmental and neurological disorders (Rudolph and Möhler, 2014; Mele et al., 2019), including autism (Hansel, 2019; Sohal and Rubenstein, 2019), down syndrome (Galdzicki et al., 2001; Schulz et al., 2019), schizophrenia (Lewis and Moghaddam, 2006; Gao and Penzes, 2015), epilepsy (Needs et al., 2019), and neurodegenerative disorders (Smith-Dijak et al., 2019; Bi et al., 2020). Uncovering the mechanisms regulating synaptic plasticity will help illuminate how disruptions in GABAergic and glutamatergic function influence the pathophysiology of these disorders, identify new therapeutic targets, and reveal potential impacts of pharmacologically targeting these receptors.
Homeostatic and Hebbian plasticity constitute two major forms of activity-dependent regulation of synaptic transmission (Kavalali and Monteggia, 2020; reviewed in Galanis and Vlachos, 2020). During Hebbian plasticity, synapses rapidly respond to a stimulus in the same direction as the applied stimulus. These Hebbian mechanisms typically result in a persistent strengthening or weakening of synapses, termed long-term potentiation (LTP) and long-term depression (LTD), respectively. NMDAR-dependent LTP of excitatory synapses in the hippocampus is the most studied experimental model for investigating the synaptic basis of plasticity, learning, and memory in vertebrates (Collingridge et al., 1983; Bliss and Collingridge, 1993; Martin et al., 2000; Neves et al., 2008; Stuchlik, 2014). On the other hand, during homeostatic plasticity, synapses respond in the opposite direction and on a slower timescale from the applied stimulus, compensating for the shift in activity. For example, 24-h treatment with the GABAAR competitive antagonist bicuculline overall upregulates inhibition, increasing GABAAR surface clustering and reducing miniature excitatory postsynaptic currents (mEPSCs) (Turrigiano et al., 1998; Pribiag et al., 2014). Although the importance of inhibitory synapse plasticity has become more apparent in recent years, research has largely continued to divide focus between excitation or inhibition, studying one or the other in isolation. However, increasing evidence indicates a coordination between GABAergic and glutamatergic synapses to maintain an optimal orchestrated balance of neuronal activity (Higley, 2014; Jedlicka et al., 2018; Chiu et al., 2019). Activity-dependent functioning of proteins in calcium-dependent signaling pathways appears to be the primary mechanism for synaptic crosstalk during plasticity, with important examples including voltage-gated calcium channels; the kinases CaMKII and PKC; the phosphatase calcineurin; and the protease calpain. However, there is a lack of sufficient understanding of these mechanisms. Further studies which simultaneously investigate excitatory and inhibitory synaptic responses under various plasticity-inducing protocols are needed, with a particular focus at the receptor level and mechanisms of crosstalk. Pharmacological manipulation provides valuable insight into the plasticity of synaptic ionotropic receptors under differential states of activity. In this mini-review, we focus primarily on brief to intermediate (30-min–48-h) pharmacologically-induced postsynaptic plasticity of GABAergic and glutamatergic synapses studied using the long-standing model of rodent neuron cultures and discuss potential key proteins involved in mediating synaptic crosstalk.
GABA Type a Receptor Synaptic Plasticity Induced by Pharmacological Treatments
Receptor trafficking, particularly lateral diffusion between synaptic and extrasynaptic sites, rapidly regulates excitatory or inhibitory synaptic strength (reviewed in Bard and Groc, 2011; Ladépêche et al., 2014; Petrini and Barberis, 2014; Maynard and Triller, 2019). The postsynaptic scaffolds gephyrin and PSD95 help tether ionotropic receptors at inhibitory and excitatory synapses, respectively, while diffusion to extrasynaptic regions facilitates receptor internalization (Blanpied et al., 2002; Rácz et al., 2004; Thomas et al., 2005; Bogdanov et al., 2006). As discussed below, pharmacologically altering neuronal activity influences receptor trafficking and synaptic localization to dynamically modulate synaptic strength.
Acute Changes in Neuronal Activity
Overall, moderate-to-high increases in neuronal activity on an acute timescale induce iLTD through enhanced GABAAR diffusion and reduced postsynaptic clustering. For example, dramatically increasing activity with the potassium channel blocker 4-aminopyridine (4AP, 100 μM) or NMDAR stimulation (NMDA + co-agonist glycine + tetrodotoxin) for <30-min immediately destabilizes inhibitory synapses via reduced synaptic GABAAR confinement and decreases the amplitude of miniature inhibitory postsynaptic currents (mIPSCs) (Niwa et al., 2012; Lévi et al., 2015). Pharmacological receptor stabilization by the benzodiazepine (BZD) diazepam (DZP), a GABAAR positive allosteric modulator, prevents the 4AP-induced mobility increase (Lévi et al., 2015). Enhanced GABAAR diffusion is similarly observed during NMDAR activation by co-application of glutamate and glycine (Muir et al., 2010) or when GABAAR activity is reduced by the negative allosteric modulator (NAM), methyl-6,7-dimethoxyl-4-ethyl- β-carboline-3-carboxylate (DMCM) (Lévi et al., 2015). These rapid effects rely on calcineurin activation and phosphorylation of the γ2 subunit present in the majority of synaptic GABAARs and appear to be independent of receptor internalization; inhibition of endocytosis with dynasore did not impact the 4AP- or glutamate/glycine-induced effects (Bannai et al., 2009; Muir et al., 2010). In contrast, Saliba et al. (2012) observed a CaMKII-dependent increase in surface β3-GABAAR with 30-min 4AP (50 μM) along with enhanced tonic current. eLTD/iLTP protocols with brief, moderate NMDA stimulation (2-min NMDA + AMPAR antagonist CNQX) followed by a short recovery period (10–20 min) also enhanced CaMKII-mediated cell-surface insertion of β2/3-GABAAR, increased mIPSC amplitude, and stabilized synapse-specific GABAAR (Marsden et al., 2007; Petrini et al., 2014). CaMKII-mediated receptor insertion may also represent a potential mechanism for GABAAR recovery in response to dramatic increases in activity. GABAAR synaptic cluster loss following brief NMDAR activation is recovered within 40-min via either new receptor insertion or reclustering of existing surface receptors (Muir et al., 2010). Thus, it appears opposing effects on GABAAR plasticity may reflect how specific experimental conditions produce localized time-dependent calcium concentration dynamics activating either calcineurin at high [Ca2+] or CaMKII at moderate-to-low [Ca2+].
Based on these observations, acute pharmacological enhancement of inhibition might be anticipated to promote GABAAR synapse stabilization. Contrary to this expectation, 30-min treatment with the GABAAR agonist muscimol increases GABAAR diffusivity and reduces receptor and scaffold clustering (Gouzer et al., 2014; Brady et al., 2018). Intriguingly, there is an overall rearrangement of distinct populations of receptors, whereby γ2-GABAARs shift to extrasynaptic sites and non-γ2-GABAARs increase in the synapse (Brady et al., 2018). These iLTD-like responses to a GABAAR agonist are reminiscent to those observed with acute enhancement of excitation. While GABAAR agonists destabilize synapses, 30-min application of GABAAR antagonists such as gabazine or picrotoxin increase GABAAR synaptic prevalence in an iLTP-like fashion (Gouzer et al., 2014). In contrast to muscimol-destabilization of inhibitory synapses, muscimol and DZP co-treatment stabilizes GABAergic synapses. Interestingly, DZP stabilizes receptors independent of activity and calcium (Gouzer et al., 2014), with similar reductions in both muscimol and 4AP-mediated GABAAR diffusion. GABAAR subtypes exhibit differential clustering, synaptic localization, drug binding sites, and intracellular protein interactors, thus potentially invoking different plasticity mechanisms. In support of this, the effects of DZP on receptor dynamics was dependent on the gephyrin binding motif (Gouzer et al., 2014).
Chronic Changes in Neuronal Activity
Compared to changes observed with acute activity modulation, homeostatic synaptic plasticity in response to 24–48-h pharmacological manipulation functions to re-establish neuronal activity balance. For example, 48-h-4AP-enhanced activity increases synaptic gephyrin and α2-GABAARs with concurrent reduced α2-GABAAR diffusion (Battaglia et al., 2018). Additionally, global depletion of neuronal activity for 24–48-h with tetrodotoxin (TTX) substantially restructures both glutamatergic and GABAergic synapses. This is characterized by reduced surface/total levels and clustering of the major synaptic γ2-GABAARs (Gouzer et al., 2014), reduced presynaptic GAD65, and diminished GABAergic neurotransmission (Kilman et al., 2002; Swanwick et al., 2006). Meanwhile, TTX leads to increased mEPSCs, indicating upregulated excitatory transmission (O’Brien et al., 1998; Turrigiano et al., 1998; Watt et al., 2000). The effects of TTX on inhibitory synapses were replicated by pharmacological blockade of either AMPARs by DNQX or NMDARs by APV (Swanwick et al., 2006), implicating a role for these receptors in inhibitory plasticity induction. Furthermore, 24–48-h treatment with the GABAAR competitive antagonist bicuculline overall upregulates inhibition by increasing GABAAR surface clustering and reducing mEPSCs (Turrigiano et al., 1998; Pribiag et al., 2014). As early as 4-h of bicuculline treatment increases the expression of GAD65 and VGAT presynaptically and α1-GABAAR postsynaptically, accompanied by a corresponding functional enhancement of inhibition (Peng et al., 2010). Co-application of the AMPAR antagonist NBQX blocks these responses. Bicuculline-induced increases in surface α5-GABAARs and tonic inhibition after 24–48 h are also blocked by APV or the GluN2A-preferring antagonist NVP (Wu et al., 2021a). Activity-dependent scaling of inhibition was recently demonstrated in detail using super-resolution microscopy. Specifically, 24-h bicuculline increases the number of subsynaptic domains per synapse and increases the compartment volume each of gephyrin, GABAARs, and the inhibitory postsynaptic density (Crosby et al., 2019). Such homeostatic responses equilibrate E/I balance throughout prolonged modulation of neuronal excitability.
Despite the intrinsic utility of homeostasis, chronic therapeutic targeting of these receptors can prompt homeostatic responses that are detrimental to maintaining the desired response. For example, BZDs are clinically used in treatment of seizures, anxiety, and insomnia, but tolerance and dependence develop with long-term use. Withdrawal symptoms occur upon discontinuation and are characterized by hallmarks of hyperexcitability, including increased risk of seizures, insomnia, and anxiety. Correspondingly, prolonged (12–48-h) BZD treatment downregulates inhibition through multiple interconnected mechanisms. This includes through reduced transcription of α1 subunits (Foitzick et al., 2020); increased degradation of BZD-sensitive α2 and γ2 subunits (Jacob et al., 2012; Lorenz-Guertin et al., 2019); reduced gephyrin clustering via increased activity of the calcium-activated protease calpain (Vlachos et al., 2013; Lorenz-Guertin et al., 2019); and enhanced surface mobility of γ2-GABAAR and increased inhibitory synaptic turnover (Lorenz-Guertin et al., 2019). Together these events likely contribute to a functional reduction of DZP potentiation and inhibition overall (Zeng and Tietz, 1999; Jacob et al., 2012; Vlachos et al., 2013). Moving forward, fundamental studies focused on concurrent basal synaptic plasticity and translational efforts centered on how chronic use of therapeutic agents modifies plasticity at excitatory and inhibitory synapses will be invaluable.
Interplay Between Glutamatergic and Gabaergic Synapses
Research has generally separated its focus between excitation or inhibition, despite the ever-present, dynamic coordination and integration between both synapse types necessary for orchestrating appropriate neuronal activity. This is largely due to the greater experimental load and range of required reagents, time, and expertise this necessitates. Developmental studies have thus far provided important insight into the interplay between glutamatergic and GABAergic synapses (reviewed in Jenks et al., 2021). However, insufficient research has been aimed at understanding crosstalking mechanisms at the receptor level in mature neurons. Studies which previously investigated both synapse types have often evaluated plasticity responses only on a macroscopic level (population field potentials), which lends difficulty in discerning what underlying changes in excitation and/or inhibition contribute to the net result. Accumulating evidence points to complex mechanisms at play involving a convergence of signaling cascades, facilitating crosstalk between synapse types.
Effect of Glutamate Receptor Signaling on Inhibitory Synaptic Plasticity
Much of what is known about synaptic crosstalk resulting in inhibitory plasticity is based on excitatory protocols activating NMDARs or mGluRs. Exposure to NMDA or aspartate activates NMDARs and subsequently suppresses GABAAR current (Stelzer and Shi, 1994; Chisari et al., 2012) in a GABA concentration-dependent manner (Cong et al., 2011), and vice versa—NMDAR currents can be suppressed by GABAAR pre-activation (Cong et al., 2011). Recent GluN2A vs. GluN2B NMDAR subtype-specific crosstalk effects were identified; 24-h antagonism of GluN2A in cultured neurons at days in vitro 14 (DIV14) with NVP-AAM077 decreased surface α5-GABAAR and tonic inhibition, while blockade of GluN2B with ifenprodil led to an increase (Wu et al., 2021a). In contrast to moderate-to-high level NMDAR activation that destabilizes GABAAR, a low-level, brief NMDAR activation results in enhanced spontaneous IPSCs (Xue et al., 2011) and α5-GABAAR-mediated tonic current (Wyroślak et al., 2021) while simultaneously inducing eLTD (Rajgor et al., 2020). This NMDA-induced iLTP is further characterized by increased synaptic gephyrin accumulation and receptor insertion (Petrini and Barberis, 2014) and associated with the formation of subsynaptic nanodomains that stabilize IPSP amplitude (Pennacchietti et al., 2017). 90-min following NMDA stimulation, reduced microRNA-mediated gene silencing of α1- and γ2-GABAARs is responsible for increased surface receptor expression in iLTP, which occurs alongside enhanced silencing of the AMPAR GluA1 gene (Rajgor et al., 2020). NMDAR antagonism by APV blocks iLTP, confirming the impact of glutamatergic activity on inhibitory plasticity (Ouardouz and Sastry, 2000). In addition to ionotropic NMDARs, mGluR activation also regulates synaptic inhibition via inositol 1,4,5-trisphosphate receptor (IP3R)-dependent intracellular calcium store release and subsequent PKC-mediated stabilization of GABAARs at synapses (Bannai et al., 2015). Interestingly, eLTP is potentiated by pharmacological inhibition of mGluR or IP3R (Taufiq et al., 2005) or IP3R genetic deletion, likely due to the lower decay rate of calcium levels observed (Yoshioka et al., 2010).
Effect of GABA Type A Receptor Signaling on Excitatory Synaptic Plasticity
Just as glutamatergic activity modulates inhibitory plasticity, GABAAR activity modulates excitatory plasticity. Pharmacological enhancement of GABAARs, as with the BZD flunitrazepam or 4,5,6,7-tetrahydroisoxazolo[5,4-c]pyridin-3-ol (THIP), a δ-GABAAR-preferring agonist, blocks eLTP (Seabrook et al., 1997; Whissell et al., 2013). Furthermore, potentiation of GABAARs by the anesthetics isoflurane, sevoflurane, and etomidate inhibits eLTP in a dose-dependent manner (Haseneder et al., 2009), where the effects of etomidate are mediated by β2-GABAARs (Figueroa et al., 2021). Several mechanisms for this dampening of excitation during potentiation of inhibition have been demonstrated. For example, isoflurane application for 6-h specifically enhances endocytosis of GluN2B-NMDARs (Dong et al., 2013), while co-application of muscimol with glutamate functionally reduces glutamate-induced calcium rise (Brady et al., 2018). Alcohol potentiates GABAARs and inhibits NMDARs (reviewed in Roberto and Varodayan, 2017). At physiologically relevant concentrations (Wallner et al., 2006; Olsen et al., 2007), a 30 min–1 h withdrawal from single-dose ethanol induces rapid subunit alterations in GABAARs. Most notably, extrasynaptic α4βδ-containing receptors are rapidly internalized, resulting in overall reduced tonic current and diminished ethanol-potentiation of tonic current (Liang et al., 2007; Shen et al., 2011; Suryanarayanan et al., 2011; Chen et al., 2018). Interestingly, α4 and γ2 subunit expression increase at later timepoints after withdrawal, beginning at 4-h and persisting for up to 48-h (Liang et al., 2007; Shen et al., 2011; Werner et al., 2011), potentially due to increased α4βγ2-GABAARs. A 15-min ethanol administration to hippocampal slices blocks tetanic-induced eLTP via a GABAAR-dependent mechanism (Ramachandran et al., 2015). Surprisingly, this increases expression of γ2-GABAAR along with AMPAR subunits GluA1 and GluA2, NMDAR subunit GluN2A, and PSD95. Ethanol treatment also increases production of allopregnanolone, a neurosteroid which potentiates GABAARs, contributing to the ethanol-dependent inhibition of eLTP (Ramachandran et al., 2015). While potentiating inhibition blocks eLTP, stifling inhibition with the GABAAR NAM DMCM (Seabrook et al., 1997) or the competitive antagonist bicuculline (Matsuyama et al., 2008) potentiates eLTP, which is associated with specific alterations in NMDAR expression. In immature DIV14 hippocampal neurons, 48-h inhibition of α5-GABAARs with the NAM L-655,708 decreased GluN2A, but increased synaptic GluN2B (Nuwer et al., 2021). Interestingly, as neurons mature to DIV21, L-655,708 had the opposite effect—synaptic GluN2A increased while synaptic GluN2B decreased. Additionally, increased neuronal activity uncouples α5-GABAAR from its extrasynaptic scaffold radixin (Hausrat et al., 2015), allowing α5-GABAARs to diffuse into inhibitory synapses, whereby increased synaptic α5 conductance prevents runaway LTP and freezes excitatory synaptic strength (Davenport et al., 2021).
Interplay Between Synapses
Further supporting the interplay between glutamatergic and GABAergic plasticity, iLTP induced by low-frequency stimulation (LFS) causes eLTD, reducing EPSC amplitudes (Ravasenga et al., 2022). Using photo-stimulation to induce eLTP at a single spine, Ravasenga et al. (2022) further demonstrated differential plasticity of GABAAR synapses based on their relative spatial localization to the potentiating spine, such that inhibitory synapses <3 μm of the potentiating spine underwent iLTD and synapses >3 μm away underwent iLTP. These are examples of heterosynaptic plasticity, in which unstimulated synapses undergo plasticity in response to stimulation of a separate synapse. High-frequency glutamatergic stimulation at dendritic spines increases surface AMPARs at the potentiated synapse while nearby (<3.4 μm) unstimulated spines show a decrease in surface AMPARs and eLTD, with the degree of spine shrinkage inversely proportional to stimulated spine enlargement (Oh et al., 2015; Tong et al., 2021). Although these studies did not specifically examine inhibitory synapses, GABAergic inhibition can suppress bulk cytosolic Ca2+ increases and allow the preservation of NMDAR-generated Ca2+ nanodomains to induce spine shrinkage (Hayama et al., 2013) and control postsynaptic Ca2+ signals within an individual dendritic spine (Chiu et al., 2013). In support of this, heterosynaptic plasticity induced by spike time-dependent plasticity protocols is regulated by Ca2+-induced Ca2+ release to selectively adjust the synaptic strength from populations of inputs onto mouse auditory cortex (Field et al., 2020). Likewise, treatment with the group 1 mGluR agonist (S)-3,5-Dihydroxyphenylglycine (DHPG) triggers LTD at both excitatory and inhibitory synapses by distinct mechanisms in the lateral habenula (Valentinova and Mameli, 2016), and olfactory discrimination learning results in a CaMKII-dependent twofold balanced increase in GABAAR and AMPAR channel conductance in a subset of pyramidal cells in the piriform cortex (Reuveni et al., 2017). Simultaneous structural remodeling of inhibitory and excitatory synapses is also observed in vivo under different plasticity protocols. Monocular deprivation results in clustered remodeling of inhibitory synapses and dendritic spines within a restricted dendritic region of ∼10 μm (Chen et al., 2012). Interestingly, electron microscopy studies reveal that TBS-LTP spinogenesis in mature hippocampal CA1 dendrites consists of initial loss of small excitatory and inhibitory synapses with subsequent balanced enlargement of both synapses by 2-h (Bourne and Harris, 2011).
These studies collectively point to convergent glutamatergic and GABAergic signaling that allows activity-dependent receptor coordination for regulation and tuning of excitation/inhibition balance. Identifying crosstalking proteins and mechanisms that coordinate these signaling pathways are an important current and future area of investigation.
Calcium Acts as a Master Regulator of Synaptic Crosstalk
Considerable evidence supports divergent calcium signaling pathways as the primary mechanism for mediating crosstalk between excitatory and inhibitory synapses (Figure 1). Calcium entry via NMDARs plays a central role in excitatory synapse strengthening, offset by GABAAR-regulated dampening of Ca2+ signaling. Induction of iLTP showed dependence on glutamatergic NMDAR (Ouardouz and Sastry, 2000) or mGluR signaling (Morales-Weil et al., 2020), both of which trigger a rise in intracellular calcium, such that calcium chelation prevents iLTP (Ghafouri et al., 2019; Ravasenga et al., 2022). Bannai et al. (2015) demonstrate the presence of two distinct, non-overlapping mechanisms by which mGluRs and NMDARs trigger differential calcium-signaling pathways with opposing effects on hippocampal inhibitory synapses. In this model, group 1 mGluRs promote IP3R-dependent intracellular calcium store release from the endoplasmic reticulum, leading to PKC-mediated GABAAR stabilization. Conversely, NMDAR-induced calcium influx activates the phosphatase calcineurin, which dephosphorylates γ2-GABAARs at S327, resulting in enhanced GABAAR mobility required for the rapid induction of iLTD during elevated activity (Lu Y. M. et al., 2000; Wang et al., 2003; Muir et al., 2010; Bannai et al., 2015; Garcia et al., 2021). Pharmacological inhibition of NMDAR (APV), group 1 mGluR (MPEP and CPCCOEt), IP3R (Xestospongin C), or calcineurin (FK506) all prevent heterosynaptic eLTD, strongly supporting the role of these calcium signaling pathways in eLTD (Oh et al., 2015; Tong et al., 2021).
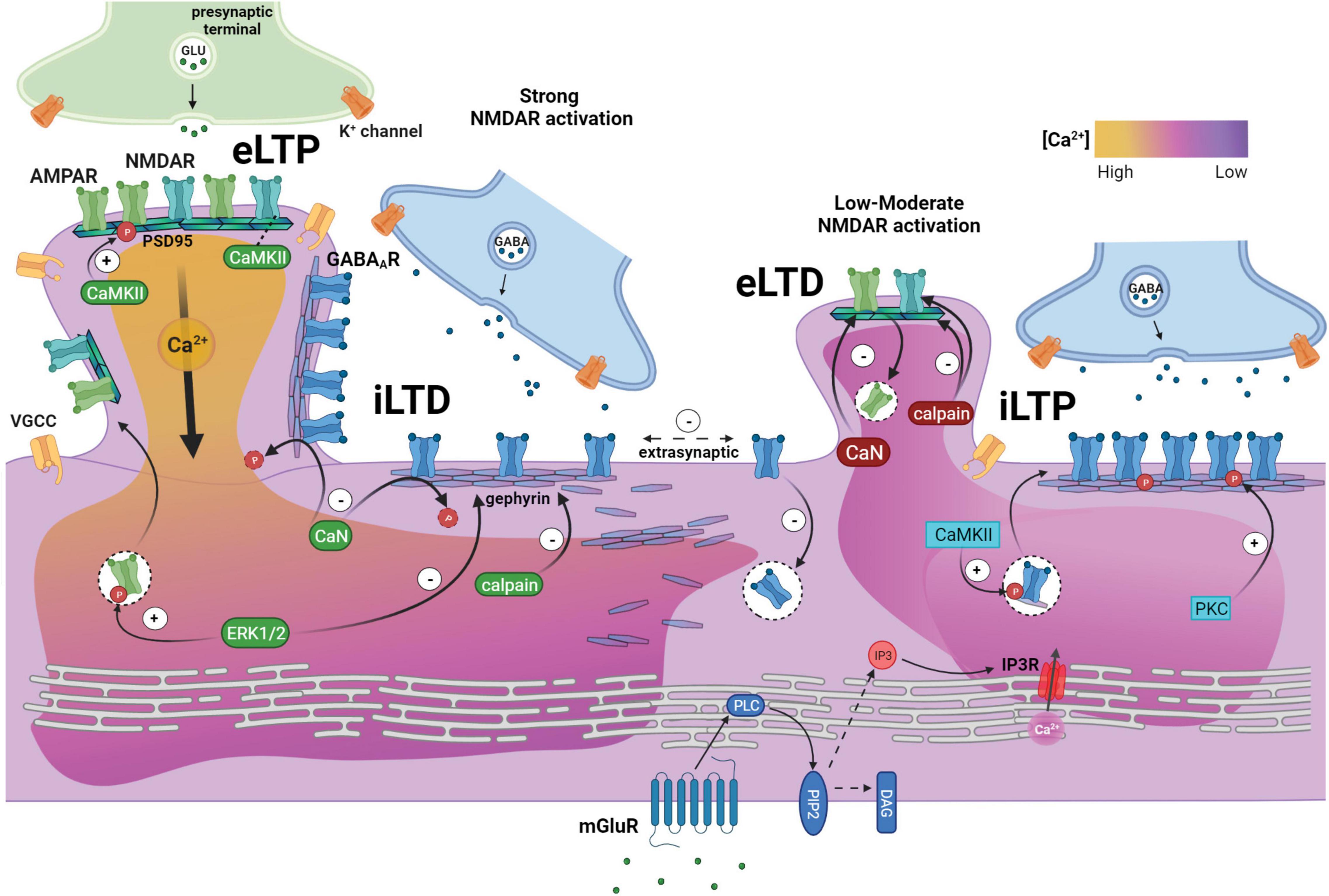
Figure 1. Relative local calcium levels facilitate glutamatergic and GABAergic synaptic crosstalk and plasticity responses through an intersection of downstream signaling pathways. Left: Strong glutamatergic activation and high calcium influx (yellow) triggers excitatory long-term potentiation (eLTP) associated with ERK1/2-mediated insertion of synaptic AMPARs, which is facilitated by translocation of CaMKIIα to excitatory synapses and interaction with NMDARs. CaMKIIα phosphorylation additionally stabilizes synaptic AMPARs. eLTP also induces heterosynaptic long-term depression of nearby inhibitory synapses (iLTD). During iLTD, calcineurin-mediated dephosphorylation of γ2-GABAARs increases receptor mobility and diffusion to extrasynaptic sites, while ERK1/2-mediated gephyrin phosphorylation and calpain protease activity disassembles the gephyrin scaffold. Right: During low-moderate NMDAR activation, CaMKIIα translocation to inhibitory synapses facilitates inhibitory long-term potentiation (iLTP) through synaptic gephyrin recruitment and enhanced β3-GABAAR forward trafficking and membrane insertion. Simultaneously, moderate NMDAR stimulation triggers a calcineurin-mediated reduction in surface AMPARs and calpain proteolytic degradation of NMDAR-GluN2B subunit and glutamatergic PSD95 scaffold (excitatory long-term depression, eLTD). eLTD and spine shrinkage is also observed in response to eLTP of proximal spines, while iLTP occurs at synapses distant from the potentiating spine. Bottom: Activation of group I metabotropic mGluR induces downstream IP3 receptor activation and release of calcium stores from the endoplasmic reticulum. The moderate increase in calcium concentration (pink) prompts PKC-mediated GABAAR phosphorylation and stabilization, contributing to the strengthening of inhibitory synapses during iLTP. Created with BioRender.com.
Voltage-gated calcium channels (VGCCs) permit calcium influx in response to membrane depolarization. This functional control over intracellular calcium makes VGCCs important contributors to multiple forms of plasticity at both synapse types (Nanou and Catterall, 2018; Gravielle, 2021). An overall dependence on NMDARs and L-VGCCs during homeostatic decreases in excitability is observed (Lee and Chung, 2014). Additionally, activation of L- and N-type VGCCs is necessary for LFS-induced iLTP. L-VGCCs also mediate heterosynaptic iLTD in response to single-spine eLTP (Ravasenga et al., 2022). Mechanistically, VGCCs can impact downstream receptor trafficking and receptor expression levels. L-VGCCs regulate GABAAR synaptic abundance by reducing proteosomal degradation and enhancing exocytosis of newly translated GABAAR (Saliba et al., 2009) via CaMKII phosphorylation of β3 at S383 (Saliba et al., 2012). On the other hand, L-VGCCs reduce nascent transcription of α1-GABAARs with 48-h DZP (Foitzick et al., 2020) and mediate enhanced GABAAR diffusion during chronic depolarization at the axon initial segment (Muir and Kittler, 2014).
Overall, these studies demonstrate the substantial impact that changes in local calcium concentrations can have on both glutamatergic and GABAergic synapses through the concerted action of key calcium-signaling proteins.
Post-translational Modifications and Crosstalking Proteins in Synaptic Plasticity
PTM-dependent regulation of interactions between receptors, sub-membrane scaffolds, and other synaptic structural proteins is central to plasticity, with many synaptic-targeting kinases and phosphatases activated by rising intracellular calcium, including calcineurin, CaMKII, and PKC. The crosstalking protein discussion provided here focuses on gephyrin, CaMKII, KCC2, and calpain; see Table 1 for a more comprehensive list of proteins indicated in plasticity at excitatory and inhibitory synapses.
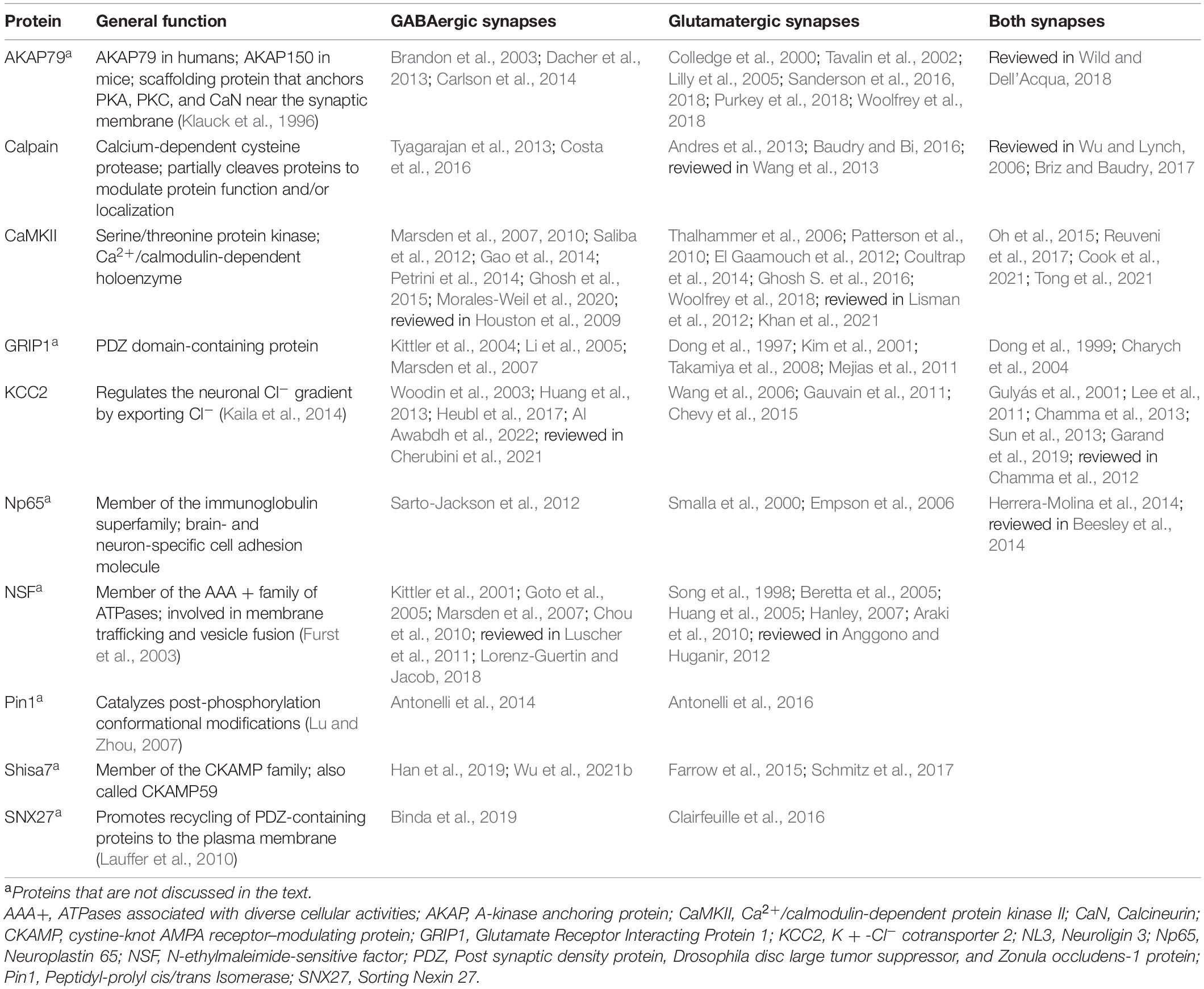
Table 1. Potential crosstalk proteins implicated in plasticity at both GABAergic and glutamatergic synapses.
GABAAR or NMDAR phosphorylation has distinct functional consequences depending on the subunit and site of phosphorylation (for review, see Chen and Roche, 2007; Nakamura et al., 2015). Gephyrin itself is also highly regulated by a multitude of PTMs (Zacchi et al., 2014; Ghosh H. et al., 2016; Battaglia et al., 2018). Chronic increases in activity (48-h 4AP treatment) result in gephyrin phosphorylation and synaptic accumulation, followed by a decrease in GABAAR diffusion (Battaglia et al., 2018). This contrasts with the effects of acutely increasing neuronal activity; here, gephyrin scaffold loss occurs subsequent to the increase in GABAAR diffusion triggered by receptor dephosphorylation (Bannai et al., 2009; Niwa et al., 2012). Using gephyrin phosphomutants, Battaglia et al. (2018) showed that chronic activity-induced phosphorylation of gephyrin by CaMKII, PKA, and GSK3β regulate synaptic GABAAR activity-dependent diffusion, while phosphorylation of gephyrin by GSK3β alone regulates extrasynaptic GABAAR activity-dependent diffusion. During acute periods of elevated activity, ERK1/2 phosphorylates gephyrin at S268 to activate calpain-mediated disassembly of the gephyrin scaffold and restrict inhibitory synaptic clustering (Tyagarajan et al., 2013). While ERK1/2 is not involved in activity-dependent regulation of gephyrin and GABAAR diffusion during chronic periods of elevated activity, it is involved in the activity-dependent regulation of extrasynaptic GABAAR mobility independent of gephyrin (Battaglia et al., 2018). Increased calpain-mediated gephyrin cleavage is also observed with 24-h DZP treatment (Lorenz-Guertin et al., 2019) and is necessary for iLTD of synapses close to spines undergoing eLTP (Ravasenga et al., 2022). Thus, gephyrin phosphorylation and calcium-activated calpain-mediated gephyrin cleavage are key regulators of inhibitory synaptic strength. Calpain also functions at excitatory synapses, where it similarly regulates PSD95 and GluN2B-NMDAR (Lu X. et al., 2000; Vinade et al., 2001; Hawasli et al., 2007; Doshi and Lynch, 2009).
CaMKII activation is required for both eLTP and eLTD (Coultrap et al., 2014; Cook et al., 2021), heterosynaptic plasticity (Oh et al., 2015; Tong et al., 2021), and iLTP (Morales-Weil et al., 2020). CaMKII also plays an important role in the long-lasting morphological changes in dendritic spines that coincide with the functional expression of plasticity (Matsuzaki et al., 2004; Lee et al., 2009). Both eLTP and eLTD/iLTP require CaMKII autophosphorylation at T286, while eLTD/iLTP requires subsequent CaMKII phosphorylation at T305/306 (Cook et al., 2021). Moderate NMDAR activation results in the translocation of CaMKIIα to inhibitory synapses (Marsden et al., 2010), which then recruits gephyrin to the synapse and increases GABAAR forward trafficking and synaptic stabilization through CaMKII-mediated phosphorylation of β3 at S383 (Petrini et al., 2014). Furthermore, earlier work by Marsden et al. (2007) revealed that moderate NMDAR activation can increase GABAAR membrane insertion via the activity of N-ethylmaleimide-sensitive factor (NSF), GABAAR-associated protein (GABARAP), and glutamate receptor interacting protein 1 (GRIP1). This same degree of activation also reduces surface AMPAR via activation of calcineurin and PP1 (Mulkey and Malenka, 1992; Beattie et al., 2000; Marsden et al., 2007). Conversely, strong NMDAR activation leads to CaMKIIα translocation to excitatory synapses (Strack et al., 1997; Shen and Meyer, 1999; Thalhammer et al., 2006) and increases AMPAR membrane insertion (Lemieux et al., 2012). Phosphorylation of AMPARs by ERK1/2, but not CaMKII, is required for activity-dependent AMPAR exocytosis (Patterson et al., 2010); interestingly, activation of GluN2B-containing, but not GluN2A-containing NMDARs results in ERK1/2 phosphorylation through a direct interaction between GluN2B and CaMKIIα (El Gaamouch et al., 2012).
Gephyrin scaffolding interactions with non-receptor proteins provides an additional mechanism of synaptic regulation. For example, gephyrin directly interacts with and regulates the surface expression of the neuronal chloride extruder K+-Cl– cotransporter KCC2 (Al Awabdh et al., 2022). KCC2 is expressed near both inhibitory and excitatory synapses (Gulyás et al., 2001; Chamma et al., 2013) and is critical for generating the hyperpolarizing chloride gradient that allows for GABAAR inhibition in mature neurons. In addition to its chloride regulation function, KCC2 promotes dendritic spine development through a structural, ion-transport-independent manner via cytoskeletal interactions (reviewed in Virtanen et al., 2021). Increased activity reduces KCC2 clustering and promotes internalization via PP1-mediated dephosphorylation and calpain-dependent cleavage (Chamma et al., 2013), while enhanced GABAAR inhibition confines KCC2 to the plasma membrane via the Cl– sensing kinase WNK1 (Heubl et al., 2017). Suppression of KCC2 precludes eLTP by preventing activity-dependent AMPAR membrane insertion in a KCC2 Cl–-transport-independent manner (Gauvain et al., 2011; Chevy et al., 2015). Interestingly, gephyrin may have further non-canonical function at excitatory synapses through interactions with neuroligins (NLGs), synaptogenic postsynaptic cell adhesion molecules which are implicated in cognition and contribute to autism (reviewed in Südhof, 2008). Co-immunoprecipitation experiments identified an association of gephyrin with both NLG2, exclusively expressed at inhibitory synapses, and NLG1, preferentially found at excitatory synapses (Varley et al., 2011). Further supporting evidence showed that hampering gephyrin function reduced VGLUT expression and mEPSC frequency.
In summary, subcellular localized domains for PTM-dependent regulation of receptors and other synaptic structural proteins allows for different forms of plasticity.
Conclusion
Pharmacological manipulation of neuronal activity, largely targeted at GABAAR or NMDAR, has provided fundamental insights into mechanisms of glutamatergic and GABAergic synaptic plasticity. However, much remains to be understood on the interplay occurring between excitatory and inhibitory synapses. Outside of the postsynaptic molecular mechanisms described here, metabotropic GABABRs, glutamatergic kainate and AMPA receptors, presynaptic signaling, and local translation all contribute to the modulation of neuronal excitability and synaptic plasticity and are important areas of ongoing research for understanding synaptic crosstalk (see Castillo et al., 2011 for general iLTP/iLTD review). Further complexity is revealed upon investigating activity-induced changes in subpopulations of synapses that arise from different types of GABAergic interneurons (Yap et al., 2020; and reviewed in Chiu et al., 2019). Future investigations should strive to understand the concurrent impact of pharmacological manipulation and pathology on both excitatory and inhibitory transmission. In addition, more standardized treatment protocols for stimulation or inhibition of neuronal activity would facilitate cross-publication comparisons of results. Furthermore, few studies have investigated GABAAR plasticity with other GABAAR subtype-specific drugs, many of which are either current clinical therapeutics or are in development. Moving forward from examining receptor-focused plasticity events, identifying the localization and plasticity contributions of crosstalking proteins at each synapse type will be valuable. Although in vivo and slice studies are included throughout this mini-review, discussion focused on plasticity studied with primary rodent neuronal cultures. While this model is invaluable for elucidating molecular mechanisms at high resolution, neuronal cultures do not provide the same complex cellular, three-dimensional, and layer specific environment found with ex vivo or in vivo models. Thus, concerted efforts using cultured-neuron, slice, and in vivo studies will be needed to piece together a comprehensive understanding of the interplay between inhibitory and excitatory synapses under different states of neuronal activity or drug treatment. This knowledge will provide insights to target impaired synaptic plasticity in neurodevelopmental and neurological disorders.
Author Contributions
CC, JN, and TJ wrote and edited the manuscript. CC prepared the figure. JN prepared the table with input from all authors.
Funding
This work was supported by the funding from U.S. National Institutes of Health grants (R01MH114908 to TJ, T32GM133332 to CC, NINDS T32NS086749 to JN).
Conflict of Interest
The authors declare that the research was conducted in the absence of any commercial or financial relationships that could be construed as a potential conflict of interest.
Publisher’s Note
All claims expressed in this article are solely those of the authors and do not necessarily represent those of their affiliated organizations, or those of the publisher, the editors and the reviewers. Any product that may be evaluated in this article, or claim that may be made by its manufacturer, is not guaranteed or endorsed by the publisher.
References
Akgül, G., and McBain, C. J. (2016). Diverse roles for ionotropic glutamate receptors on inhibitory interneurons in developing and adult brain. J. Physiol. 594, 5471–5490. doi: 10.1113/JP271764
Al Awabdh, S., Donneger, F., Goutierre, M., Séveno, M., Vigy, O., Weinzettl, P., et al. (2022). Gephyrin Interacts with the K-Cl Cotransporter KCC2 to regulate its surface expression and function in cortical neurons. J. Neurosci. 42, 166–182. doi: 10.1523/JNEUROSCI.2926-20.2021
Andres, A. L., Regev, L., Phi, L., Seese, R. R., Chen, Y., Gall, C. M., et al. (2013). NMDA receptor activation and calpain contribute to disruption of dendritic spines by the stress neuropeptide CRH. J. Neurosci. 33, 16945–16960. doi: 10.1523/JNEUROSCI.1445-13.2013
Anggono, V., and Huganir, R. L. (2012). Regulation of AMPA receptor trafficking and synaptic plasticity. Curr. Opin. Neurobiol. 22, 461–469. doi: 10.1016/j.conb.2011.12.006
Antonelli, R., De Filippo, R., Middei, S., Stancheva, S., Pastore, B., Ammassari-Teule, M., et al. (2016). Pin1 Modulates the Synaptic Content of NMDA Receptors via Prolyl-Isomerization of PSD-95. J. Neurosci. 36, 5437–5447. doi: 10.1523/JNEUROSCI.3124-15.2016
Antonelli, R., Pizzarelli, R., Pedroni, A., Fritschy, J.-M., Del Sal, G., Cherubini, E., et al. (2014). Pin1-dependent signalling negatively affects GABAergic transmission by modulating neuroligin2/gephyrin interaction. Nat. Commun. 5:5066. doi: 10.1038/ncomms6066
Araki, Y., Lin, D.-T., and Huganir, R. L. (2010). Plasma membrane insertion of the AMPA receptor GluA2 subunit is regulated by NSF binding and Q/R editing of the ion pore. Proc. Natl. Acad. Sci. USA 107, 11080–11085. doi: 10.1073/pnas.1006584107
Bannai, H., Lévi, S., Schweizer, C., Inoue, T., Launey, T., Racine, V., et al. (2009). Activity-dependent tuning of inhibitory neurotransmission based on GABAAR diffusion dynamics. Neuron 62, 670–682. doi: 10.1016/j.neuron.2009.04.023
Bannai, H., Niwa, F., Sherwood, M. W., Shrivastava, A. N., Arizono, M., Miyamoto, A., et al. (2015). Bidirectional control of synaptic GABAAR clustering by glutamate and calcium. Cell Rep. 13, 2768–2780. doi: 10.1016/j.celrep.2015.12.002
Bard, L., and Groc, L. (2011). Glutamate receptor dynamics and protein interaction: lessons from the NMDA receptor. Mol. Cell. Neurosci. 48, 298–307. doi: 10.1016/j.mcn.2011.05.009
Battaglia, S., Renner, M., Russeau, M., Côme, E., Tyagarajan, S. K., and Lévi, S. (2018). Activity-dependent inhibitory synapse scaling is determined by gephyrin phosphorylation and subsequent regulation of GABAA Receptor Diffusion. eNeuro 5:17. doi: 10.1523/ENEURO.0203-17.2017
Baudry, M., and Bi, X. (2016). Calpain-1 and Calpain-2: The Yin and Yang of Synaptic Plasticity and Neurodegeneration. Trends Neurosci. 39, 235–245. doi: 10.1016/j.tins.2016.01.007
Beattie, E. C., Carroll, R. C., Yu, X., Morishita, W., Yasuda, H., von Zastrow, M., et al. (2000). Regulation of AMPA receptor endocytosis by a signaling mechanism shared with LTD. Nat. Neurosci. 3, 1291–1300. doi: 10.1038/81823
Beesley, P. W., Herrera-Molina, R., Smalla, K.-H., and Seidenbecher, C. (2014). The Neuroplastin adhesion molecules: key regulators of neuronal plasticity and synaptic function. J. Neurochem. 131, 268–283. doi: 10.1111/jnc.12816
Beretta, F., Sala, C., Saglietti, L., Hirling, H., Sheng, M., and Passafaro, M. (2005). NSF interaction is important for direct insertion of GluR2 at synaptic sites. Mol. Cell. Neurosci. 28, 650–660. doi: 10.1016/j.mcn.2004.11.008
Bi, D., Wen, L., Wu, Z., and Shen, Y. (2020). GABAergic dysfunction in excitatory and inhibitory (E/I) imbalance drives the pathogenesis of Alzheimer’s disease. Alzheimers Dement 16, 1312–1329. doi: 10.1002/alz.12088
Binda, C. S., Nakamura, Y., Henley, J. M., and Wilkinson, K. A. (2019). Sorting nexin 27 rescues neuroligin 2 from lysosomal degradation to control inhibitory synapse number. Biochem. J. 476, 293–306. doi: 10.1042/BCJ20180504
Blanpied, T. A., Scott, D. B., and Ehlers, M. D. (2002). Dynamics and regulation of clathrin coats at specialized endocytic zones of dendrites and spines. Neuron 36, 435–449. doi: 10.1016/s0896-6273(02)00979-0
Bleckert, A., Parker, E. D., Kang, Y., Pancaroglu, R., Soto, F., Lewis, R., et al. (2013). Spatial relationships between GABAergic and glutamatergic synapses on the dendrites of distinct types of mouse retinal ganglion cells across development. PLoS One 8:e69612. doi: 10.1371/journal.pone.0069612
Bliss, T. V., and Collingridge, G. L. (1993). A synaptic model of memory: long-term potentiation in the hippocampus. Nature 361, 31–39. doi: 10.1038/361031a0
Bogdanov, Y., Michels, G., Armstrong-Gold, C., Haydon, P. G., Lindstrom, J., Pangalos, M., et al. (2006). Synaptic GABAA receptors are directly recruited from their extrasynaptic counterparts. EMBO J. 25, 4381–4389. doi: 10.1038/sj.emboj.7601309
Bourne, J. N., and Harris, K. M. (2011). Coordination of size and number of excitatory and inhibitory synapses results in a balanced structural plasticity along mature hippocampal CA1 dendrites during LTP. Hippocampus 21, 354–373. doi: 10.1002/hipo.20768.
Brady, M. L., Pilli, J., Lorenz-Guertin, J. M., Das, S., Moon, C. E., Graff, N., et al. (2018). Depolarizing, inhibitory GABA type A receptor activity regulates GABAergic synapse plasticity via ERK and BDNF signaling. Neuropharmacology 128, 324–339. doi: 10.1016/j.neuropharm.2017.10.022
Brandon, N. J., Jovanovic, J. N., Colledge, M., Kittler, J. T., Brandon, J. M., Scott, J. D., et al. (2003). A-kinase anchoring protein 79/150 facilitates the phosphorylation of GABAA receptors by cAMP-dependent protein kinase via selective interaction with receptor β subunits. Mol. Cell. Neurosci. 22, 87–97. doi: 10.1016/S1044-7431(02)00017-9
Briz, V., and Baudry, M. (2017). Calpains: master regulators of synaptic plasticity. Neuroscientist 23, 221–231. doi: 10.1177/1073858416649178
Carlson, S. L., O’Buckley, T. K., Thomas, R., Thiele, T. E., and Morrow, A. L. (2014). Altered GABAA receptor expression and seizure threshold following acute ethanol challenge in mice lacking the RIIβ subunit of PKA. Neurochem. Res. 39, 1079–1087. doi: 10.1007/s11064-013-1167-0
Castillo, P. E., Chiu, C. Q., and Carroll, R. C. (2011). Long-term plasticity at inhibitory synapses. Curr. Opin. Neurobiol. 21, 328–338. doi: 10.1016/j.conb.2011.01.006
Chamma, I., Chevy, Q., Poncer, J. C., and Lévi, S. (2012). Role of the neuronal K-Cl co-transporter KCC2 in inhibitory and excitatory neurotransmission. Front. Cell. Neurosci. 6:5. doi: 10.3389/fncel.2012.00005
Chamma, I., Heubl, M., Chevy, Q., Renner, M., Moutkine, I., Eugène, E., et al. (2013). Activity-dependent regulation of the K/Cl transporter KCC2 membrane diffusion, clustering, and function in hippocampal neurons. J. Neurosci. 33, 15488–15503. doi: 10.1523/JNEUROSCI.5889-12.2013
Charych, E. I., Yu, W., Li, R., Serwanski, D. R., Miralles, C. P., Li, X., et al. (2004). A four PDZ domain-containing splice variant form of GRIP1 is localized in GABAergic and glutamatergic synapses in the brain. J. Biol. Chem. 279, 38978–38990. doi: 10.1074/jbc.M405786200
Chen, B.-S., and Roche, K. W. (2007). Regulation of NMDA receptors by phosphorylation. Neuropharmacology 53, 362–368. doi: 10.1016/j.neuropharm.2007.05.018
Chen, J., He, Y., Wu, Y., Zhou, H., Su, L.-D., Li, W.-N., et al. (2018). Single ethanol withdrawal regulates extrasynaptic δ-GABAA Receptors Via PKCδ Activation. Front. Mol. Neurosci. 11:141. doi: 10.3389/fnmol.2018.00141
Chen, J. L., Villa, K. L., Cha, J. W., So, P. T. C., Kubota, Y., and Nedivi, E. (2012). Clustered dynamics of inhibitory synapses and dendritic spines in the adult neocortex. Neuron 74, 361–373. doi: 10.1016/j.neuron.2012.02.030
Cherubini, E., Di Cristo, G., and Avoli, M. (2021). Dysregulation of GABAergic signaling in neurodevelomental disorders: targeting cation-chloride co-transporters to re-establish a Proper E/I Balance. Front. Cell. Neurosci. 15:813441. doi: 10.3389/fncel.2021.813441
Chevy, Q., Heubl, M., Goutierre, M., Backer, S., Moutkine, I., Eugène, E., et al. (2015). KCC2 Gates Activity-Driven AMPA Receptor Traffic through Cofilin Phosphorylation. J. Neurosci. 35, 15772–15786. doi: 10.1523/JNEUROSCI.1735-15.2015
Chisari, M., Zorumski, C. F., and Mennerick, S. (2012). Cross talk between synaptic receptors mediates NMDA-induced suppression of inhibition. J. Neurophysiol. 107, 2532–2540. doi: 10.1152/jn.01145.2011
Chiu, C. Q., Barberis, A., and Higley, M. J. (2019). Preserving the balance: diverse forms of long-term GABAergic synaptic plasticity. Nat. Rev. Neurosci. 20, 272–281. doi: 10.1038/s41583-019-0141-5
Chiu, C. Q., Lur, G., Morse, T. M., Carnevale, N. T., Ellis-Davies, G. C. R., and Higley, M. J. (2013). Compartmentalization of GABAergic inhibition by dendritic spines. Science 340, 759–762. doi: 10.1126/science.1234274
Chou, W.-H., Wang, D., McMahon, T., Qi, Z.-H., Song, M., Zhang, C., et al. (2010). GABAA receptor trafficking is regulated by protein kinase C(epsilon) and the N-ethylmaleimide-sensitive factor. J. Neurosci. 30, 13955–13965. doi: 10.1523/JNEUROSCI.0270-10.2010
Clairfeuille, T., Mas, C., Chan, A. S. M., Yang, Z., Tello-Lafoz, M., Chandra, M., et al. (2016). A molecular code for endosomal recycling of phosphorylated cargos by the SNX27-retromer complex. Nat. Struct. Mol. Biol. 23, 921–932. doi: 10.1038/nsmb.3290
Colledge, M., Dean, R. A., Scott, G. K., Langeberg, L. K., Huganir, R. L., and Scott, J. D. (2000). Targeting of PKA to glutamate receptors through a MAGUK-AKAP complex. Neuron 27, 107–119. doi: 10.1016/s0896-6273(00)00013-1
Collingridge, G. L., Kehl, S. J., and McLennan, H. (1983). Excitatory amino acids in synaptic transmission in the Schaffer collateral-commissural pathway of the rat hippocampus. J. Physiol. 334, 33–46. doi: 10.1113/jphysiol.1983.sp014478
Cong, D., Tang, Z., Li, L., Huang, Y., Wang, J., and Chen, L. (2011). Cross-talk between NMDA and GABA(A) receptors in cultured neurons of the rat inferior colliculus. Sci. China Life Sci. 54, 560–566. doi: 10.1007/s11427-011-4178-6
Cook, S. G., Buonarati, O. R., Coultrap, S. J., and Bayer, K. U. (2021). CaMKII holoenzyme mechanisms that govern the LTP versus LTD decision. Sci. Adv. 7:2300. doi: 10.1126/sciadv.abe2300
Costa, J. T., Mele, M., Baptista, M. S., Gomes, J. R., Ruscher, K., Nobre, R. J., et al. (2016). Gephyrin cleavage in in vitro brain ischemia decreases GABAA receptor clustering and contributes to neuronal death. Mol. Neurobiol. 53, 3513–3527. doi: 10.1007/s12035-015-9283-2
Coultrap, S. J., Freund, R. K., O’Leary, H., Sanderson, J. L., Roche, K. W., Dell’Acqua, M. L., et al. (2014). Autonomous CaMKII mediates both LTP and LTD using a mechanism for differential substrate site selection. Cell Rep. 6, 431–437. doi: 10.1016/j.celrep.2014.01.005
Crosby, K. C., Gookin, S. E., Garcia, J. D., Hahm, K. M., Dell’Acqua, M. L., and Smith, K. R. (2019). Nanoscale subsynaptic domains underlie the organization of the inhibitory synapse. Cell Rep. 26, 3284–3297. doi: 10.1016/j.celrep.2019.02.070
Dacher, M., Gouty, S., Dash, S., Cox, B. M., and Nugent, F. S. (2013). A-kinase anchoring protein-calcineurin signaling in long-term depression of GABAergic synapses. J. Neurosci. 33, 2650–2660. doi: 10.1523/JNEUROSCI.2037-12.2013
Davenport, C. M., Rajappa, R., Katchan, L., Taylor, C. R., Tsai, M.-C., Smith, C. M., et al. (2021). Relocation of an extrasynaptic GABAA receptor to inhibitory synapses freezes excitatory synaptic strength and preserves memory. Neuron 109, 123–134. doi: 10.1016/j.neuron.2020.09.037
Dong, H., O’Brien, R. J., Fung, E. T., Lanahan, A. A., Worley, P. F., and Huganir, R. L. (1997). GRIP: a synaptic PDZ domain-containing protein that interacts with AMPA receptors. Nature 386, 279–284. doi: 10.1038/386279a0
Dong, H., Zhang, P., Song, I., Petralia, R. S., Liao, D., and Huganir, R. L. (1999). Characterization of the glutamate receptor-interacting proteins GRIP1 and GRIP2. J. Neurosci. 19, 6930–6941.
Dong, Y., Wu, X., Zhang, G., Xu, Z., Zhang, Y., Gautam, V., et al. (2013). Isoflurane facilitates synaptic NMDA receptor endocytosis in mice primary neurons. Curr. Mol. Med. 13, 488–498. doi: 10.2174/1566524011313040003
Doshi, S., and Lynch, D. R. (2009). Calpain and the glutamatergic synapse. Front. Biosci. 1:466–476.
El Gaamouch, F., Buisson, A., Moustié, O., Lemieux, M., Labrecque, S., Bontempi, B., et al. (2012). Interaction between αCaMKII and GluN2B controls ERK-dependent plasticity. J. Neurosci. 32, 10767–10779. doi: 10.1523/JNEUROSCI.5622-11.2012
Empson, R. M., Buckby, L. E., Kraus, M., Bates, K. J., Crompton, M. R., Gundelfinger, E. D., et al. (2006). The cell adhesion molecule neuroplastin-65 inhibits hippocampal long-term potentiation via a mitogen-activated protein kinase p38-dependent reduction in surface expression of GluR1-containing glutamate receptors. J. Neurochem. 99, 850–860. doi: 10.1111/j.1471-4159.2006.04123.x
Farrow, P., Khodosevich, K., Sapir, Y., Schulmann, A., Aslam, M., Stern-Bach, Y., et al. (2015). Auxiliary subunits of the CKAMP family differentially modulate AMPA receptor properties. eLife 4:e09693. doi: 10.7554/eLife.09693
Field, R. E., D’amour, J. A., Tremblay, R., Miehl, C., Rudy, B., Gjorgjieva, J., et al. (2020). Heterosynaptic plasticity determines the set point for cortical excitatory-inhibitory balance. Neuron 106, 842–854. doi: 10.1016/j.neuron.2020.03.002
Figueroa, A. G., Benkwitz, C., Surges, G., Kunz, N., Homanics, G. E., and Pearce, R. A. (2021). Hippocampal β2-GABAA receptors mediate LTP suppression by etomidate and contribute to long-lasting feedback but not feedforward inhibition of pyramidal neurons. J. Neurophysiol. 126, 1090–1100. doi: 10.1152/jn.00303.2021
Foitzick, M. F., Medina, N. B., Iglesias García, L. C., and Gravielle, M. C. (2020). Benzodiazepine exposure induces transcriptional down-regulation of GABAA receptor α1 subunit gene via L-type voltage-gated calcium channel activation in rat cerebrocortical neurons. Neurosci. Lett. 721:134801. doi: 10.1016/j.neulet.2020.134801
Furst, J., Sutton, R. B., Chen, J., Brunger, A. T., and Grigorieff, N. (2003). Electron cryomicroscopy structure of N-ethyl maleimide sensitive factor at 11 A resolution. EMBO J. 22, 4365–4374. doi: 10.1093/emboj/cdg420
Galanis, C., and Vlachos, A. (2020). Hebbian and homeostatic synaptic plasticity-do alterations of one reflect enhancement of the other? Front. Cell. Neurosci. 14:50. doi: 10.3389/fncel.2020.00050
Galdzicki, Z., Siarey, R., Pearce, R., Stoll, J., and Rapoport, S. I. (2001). On the cause of mental retardation in Down syndrome: extrapolation from full and segmental trisomy 16 mouse models. Brain Res. Brain Res. Rev. 35, 115–145. doi: 10.1016/S0926-6410(00)00074-4
Gao, R., and Penzes, P. (2015). Common mechanisms of excitatory and inhibitory imbalance in schizophrenia and autism spectrum disorders. Curr. Mol. Med. 15, 146–167. doi: 10.2174/1566524015666150303003028
Gao, Z., van Woerden, G. M., Elgersma, Y., De Zeeuw, C. I., and Hoebeek, F. E. (2014). Distinct roles of α- and βCaMKII in controlling long-term potentiation of GABAA-receptor mediated transmission in murine Purkinje cells. Front. Cell. Neurosci. 8:16. doi: 10.3389/fncel.2014.00016
Garand, D., Mahadevan, V., and Woodin, M. A. (2019). Ionotropic and metabotropic kainate receptor signalling regulates Cl- homeostasis and GABAergic inhibition. J. Physiol. 597, 1677–1690. doi: 10.1113/JP276901
Garcia, J. D., Gookin, S. E., Crosby, K. C., Schwartz, S. L., Tiemeier, E., Kennedy, M. J., et al. (2021). Stepwise disassembly of GABAergic synapses during pathogenic excitotoxicity. Cell Rep. 37:110142. doi: 10.1016/j.celrep.2021.110142
Gauvain, G., Chamma, I., Chevy, Q., Cabezas, C., Irinopoulou, T., Bodrug, N., et al. (2011). The neuronal K-Cl cotransporter KCC2 influences postsynaptic AMPA receptor content and lateral diffusion in dendritic spines. Proc. Natl. Acad. Sci. USA 108, 15474–15479. doi: 10.1073/pnas.1107893108
Ghafouri, S., Fathollahi, Y., Semnanian, S., Shojaei, A., Asgari, A., Ebrahim Amini, A., et al. (2019). Deep brain stimulation restores the glutamatergic and GABAergic synaptic transmission and plasticity to normal levels in kindled rats. PLoS One 14:e0224834. doi: 10.1371/journal.pone.0224834
Ghosh, H., Auguadri, L., Battaglia, S., Simone Thirouin, Z., Zemoura, K., Messner, S., et al. (2016). Several posttranslational modifications act in concert to regulate gephyrin scaffolding and GABAergic transmission. Nat. Commun. 7:13365. doi: 10.1038/ncomms13365
Ghosh, S., Reuveni, I., Barkai, E., and Lamprecht, R. (2016). Calcium/calmodulin-dependent kinase II activity is required for maintaining learning-induced enhancement of α-amino-3-hydroxy-5-methyl-4-isoxazolepropionic acid receptor-mediated synaptic excitation. J. Neurochem. 136, 1168–1176. doi: 10.1111/jnc.13505
Ghosh, S., Reuveni, I., Lamprecht, R., and Barkai, E. (2015). Persistent CaMKII activation mediates learning-induced long-lasting enhancement of synaptic inhibition. J. Neurosci. 35, 128–139. doi: 10.1523/JNEUROSCI.2123-14.2015
Goto, H., Terunuma, M., Kanematsu, T., Misumi, Y., Moss, S. J., and Hirata, M. (2005). Direct interaction of N-ethylmaleimide-sensitive factor with GABA(A) receptor beta subunits. Mol. Cell. Neurosci. 30, 197–206. doi: 10.1016/j.mcn.2005.07.006
Gouzer, G., Specht, C. G., Allain, L., Shinoe, T., and Triller, A. (2014). Benzodiazepine-dependent stabilization of GABA(A) receptors at synapses. Mol. Cell. Neurosci. 63, 101–113. doi: 10.1016/j.mcn.2014.10.004
Gravielle, M. C. (2021). Regulation of GABAA receptors induced by the activation of L-Type voltage-gated calcium channels. Membranes 11:486. doi: 10.3390/membranes11070486
Gulyás, A. I., Sík, A., Payne, J. A., Kaila, K., and Freund, T. F. (2001). The KCl cotransporter, KCC2, is highly expressed in the vicinity of excitatory synapses in the rat hippocampus. Eur. J. Neurosci. 13, 2205–2217. doi: 10.1046/j.0953-816x.2001.01600.x
Han, W., Li, J., Pelkey, K. A., Pandey, S., Chen, X., Wang, Y.-X., et al. (2019). Shisa7 is a GABAA receptor auxiliary subunit controlling benzodiazepine actions. Science 366, 246–250. doi: 10.1126/science.aax5719
Hanley, J. G. (2007). NSF binds calcium to regulate its interaction with AMPA receptor subunit GluR2. J. Neurochem. 101, 1644–1650. doi: 10.1111/j.1471-4159.2007.04455.x
Hansel, C. (2019). Deregulation of synaptic plasticity in autism. Neurosci. Lett. 688, 58–61. doi: 10.1016/j.neulet.2018.02.003
Haseneder, R., Kratzer, S., von Meyer, L., Eder, M., Kochs, E., and Rammes, G. (2009). Isoflurane and sevoflurane dose-dependently impair hippocampal long-term potentiation. Eur. J. Pharmacol. 623, 47–51. doi: 10.1016/j.ejphar.2009.09.022
Hausrat, T. J., Muhia, M., Gerrow, K., Thomas, P., Hirdes, W., Tsukita, S., et al. (2015). Radixin regulates synaptic GABAA receptor density and is essential for reversal learning and short-term memory. Nat. Commun. 6:6872. doi: 10.1038/ncomms7872
Hawasli, A. H., Benavides, D. R., Nguyen, C., Kansy, J. W., Hayashi, K., Chambon, P., et al. (2007). Cyclin-dependent kinase 5 governs learning and synaptic plasticity via control of NMDAR degradation. Nat. Neurosci. 10, 880–886. doi: 10.1038/nn1914
Hayama, T., Noguchi, J., Watanabe, S., Takahashi, N., Hayashi-Takagi, A., Ellis-Davies, G. C. R., et al. (2013). GABA promotes the competitive selection of dendritic spines by controlling local Ca2+ signaling. Nat. Neurosci. 16, 1409–1416. doi: 10.1038/nn.3496
Herrera-Molina, R., Sarto-Jackson, I., Montenegro-Venegas, C., Heine, M., Smalla, K.-H., Seidenbecher, C. I., et al. (2014). Structure of excitatory synapses and GABAA receptor localization at inhibitory synapses are regulated by neuroplastin-65. J. Biol. Chem. 289, 8973–8988. doi: 10.1074/jbc.M113.514992
Heubl, M., Zhang, J., Pressey, J. C., Al Awabdh, S., Renner, M., Gomez-Castro, F., et al. (2017). GABAA receptor dependent synaptic inhibition rapidly tunes KCC2 activity via the Cl–sensitive WNK1 kinase. Nat. Commun. 8:1776. doi: 10.1038/s41467-017-01749-0
Higley, M. J. (2014). Localized GABAergic inhibition of dendritic Ca(2+) signalling. Nat. Rev. Neurosci. 15, 567–572. doi: 10.1038/nrn3803
Houston, C. M., He, Q., and Smart, T. G. (2009). CaMKII phosphorylation of the GABA(A) receptor: receptor subtype- and synapse-specific modulation. J. Physiol. 587, 2115–2125. doi: 10.1113/jphysiol.2009.171603
Huang, Y., Man, H.-Y., Sekine-Aizawa, Y., Han, Y., Juluri, K., Luo, H., et al. (2005). S-nitrosylation of N-ethylmaleimide sensitive factor mediates surface expression of AMPA receptors. Neuron 46, 533–540. doi: 10.1016/j.neuron.2005.03.028
Huang, Y., Wang, J.-J., and Yung, W.-H. (2013). Coupling between GABA-A receptor and chloride transporter underlies ionic plasticity in cerebellar Purkinje neurons. Cerebellum 12, 328–330. doi: 10.1007/s12311-013-0453-3
Iascone, D. M., Li, Y., Sümbül, U., Doron, M., Chen, H., Andreu, V., et al. (2020). Whole-Neuron synaptic mapping reveals spatially precise excitatory/inhibitory balance limiting dendritic and somatic spiking. Neuron 106, 566–578. doi: 10.1016/j.neuron.2020.02.015
Ismail, F. Y., Fatemi, A., and Johnston, M. V. (2017). Cerebral plasticity: windows of opportunity in the developing brain. Eur. J. Paediatr. Neurol. 21, 23–48. doi: 10.1016/j.ejpn.2016.07.007
Jacob, T. C., Michels, G., Silayeva, L., Haydon, J., Succol, F., and Moss, S. J. (2012). Benzodiazepine treatment induces subtype-specific changes in GABA(A) receptor trafficking and decreases synaptic inhibition. Proc. Natl. Acad. Sci. USA 109, 18595–18600. doi: 10.1073/pnas.1204994109
Jedlicka, P., Muellerleile, J., and Schwarzacher, S. W. (2018). Synaptic plasticity and excitation-inhibition balance in the dentate gyrus: insights from in Vivo Recordings in Neuroligin-1, Neuroligin-2, and Collybistin Knockouts. Neural. Plast. 2018:6015753. doi: 10.1155/2018/6015753
Jenks, K. R., Tsimring, K., Ip, J. P. K., Zepeda, J. C., and Sur, M. (2021). Heterosynaptic plasticity and the experience-dependent refinement of developing neuronal circuits. Front. Neural Circ. 15:803401. doi: 10.3389/fncir.2021.803401
Kaila, K., Price, T. J., Payne, J. A., Puskarjov, M., and Voipio, J. (2014). Cation-chloride cotransporters in neuronal development, plasticity and disease. Nat. Rev. Neurosci. 15, 637–654. doi: 10.1038/nrn3819
Kavalali, E. T., and Monteggia, L. M. (2020). Targeting homeostatic synaptic plasticity for treatment of mood disorders. Neuron 106, 715–726. doi: 10.1016/j.neuron.2020.05.015
Khan, R., Kulasiri, D., and Samarasinghe, S. (2021). Functional repertoire of protein kinases and phosphatases in synaptic plasticity and associated neurological disorders. Neural Regen. Res. 16, 1150–1157. doi: 10.4103/1673-5374.300331
Kilman, V., van Rossum, M. C. W., and Turrigiano, G. G. (2002). Activity deprivation reduces miniature IPSC amplitude by decreasing the number of postsynaptic GABA(A) receptors clustered at neocortical synapses. J. Neurosci. 22, 1328–1337.
Kim, C. H., Chung, H. J., Lee, H. K., and Huganir, R. L. (2001). Interaction of the AMPA receptor subunit GluR2/3 with PDZ domains regulates hippocampal long-term depression. Proc. Natl. Acad. Sci. USA 98, 11725–11730. doi: 10.1073/pnas.211132798
Kittler, J. T., Arancibia-Carcamo, I. L., and Moss, S. J. (2004). Association of GRIP1 with a GABA(A) receptor associated protein suggests a role for GRIP1 at inhibitory synapses. Biochem. Pharmacol. 68, 1649–1654. doi: 10.1016/j.bcp.2004.07.028
Kittler, J. T., Rostaing, P., Schiavo, G., Fritschy, J. M., Olsen, R., Triller, A., et al. (2001). The subcellular distribution of GABARAP and its ability to interact with NSF suggest a role for this protein in the intracellular transport of GABA(A) receptors. Mol. Cell. Neurosci. 18, 13–25. doi: 10.1006/mcne.2001.1005
Klauck, T. M., Faux, M. C., Labudda, K., Langeberg, L. K., Jaken, S., and Scott, J. D. (1996). Coordination of three signaling enzymes by AKAP79, a mammalian scaffold protein. Science 271, 1589–1592. doi: 10.1126/science.271.5255.1589
Ladépêche, L., Dupuis, J. P., and Groc, L. (2014). Surface trafficking of NMDA receptors: gathering from a partner to another. Semin. Cell Dev. Biol. 27, 3–13. doi: 10.1016/j.semcdb.2013.10.005
Lauffer, B. E. L., Melero, C., Temkin, P., Lei, C., Hong, W., Kortemme, T., et al. (2010). SNX27 mediates PDZ-directed sorting from endosomes to the plasma membrane. J. Cell Biol. 190, 565–574. doi: 10.1083/jcb.201004060
Lee, H. H. C., Deeb, T. Z., Walker, J. A., Davies, P. A., and Moss, S. J. (2011). NMDA receptor activity downregulates KCC2 resulting in depolarizing GABAA receptor-mediated currents. Nat. Neurosci. 14, 736–743. doi: 10.1038/nn.2806
Lee, K. Y., and Chung, H. J. (2014). NMDA receptors and L-type voltage-gated Ca2+ channels mediate the expression of bidirectional homeostatic intrinsic plasticity in cultured hippocampal neurons. Neuroscience 277, 610–623. doi: 10.1016/j.neuroscience.2014.07.038
Lee, S.-J. R., Escobedo-Lozoya, Y., Szatmari, E. M., and Yasuda, R. (2009). Activation of CaMKII in single dendritic spines during long-term potentiation. Nature 458, 299–304. doi: 10.1038/nature07842
Lemieux, M., Labrecque, S., Tardif, C., Labrie-Dion, E., Lebel, E., and De Koninck, P. (2012). Translocation of CaMKII to dendritic microtubules supports the plasticity of local synapses. J. Cell Biol. 198, 1055–1073. doi: 10.1083/jcb.201202058
Lévi, S., Le Roux, N., Eugène, E., and Poncer, J. C. (2015). Benzodiazepine ligands rapidly influence GABAA receptor diffusion and clustering at hippocampal inhibitory synapses. Neuropharmacology 88, 199–208. doi: 10.1016/j.neuropharm.2014.06.002
Lewis, D. A., and Moghaddam, B. (2006). Cognitive dysfunction in schizophrenia: convergence of gamma-aminobutyric acid and glutamate alterations. Arch. Neurol. 63, 1372–1376. doi: 10.1001/archneur.63.10.1372
Li, R.-W., Serwanski, D. R., Miralles, C. P., Li, X., Charych, E., Riquelme, R., et al. (2005). GRIP1 in GABAergic synapses. J. Comp. Neurol. 488, 11–27. doi: 10.1002/cne.20566
Liang, J., Suryanarayanan, A., Abriam, A., Snyder, B., Olsen, R. W., and Spigelman, I. (2007). Mechanisms of reversible GABAA receptor plasticity after ethanol intoxication. J. Neurosci. 27, 12367–12377. doi: 10.1523/JNEUROSCI.2786-07.2007
Lilly, S. M., Alvarez, F. J., and Tietz, E. I. (2005). Synaptic and subcellular localization of A-kinase anchoring protein 150 in rat hippocampal CA1 pyramidal cells: Co-localization with excitatory synaptic markers. Neuroscience 134, 155–163. doi: 10.1016/j.neuroscience.2005.03.039
Lisman, J., Yasuda, R., and Raghavachari, S. (2012). Mechanisms of CaMKII action in long-term potentiation. Nat. Rev. Neurosci. 13, 169–182. doi: 10.1038/nrn3192
Lorenz-Guertin, J. M., Bambino, M. J., Das, S., Weintraub, S. T., and Jacob, T. C. (2019). Diazepam accelerates GABAAR synaptic exchange and alters intracellular trafficking. Front. Cell. Neurosci. 13:163. doi: 10.3389/fncel.2019.00163
Lorenz-Guertin, J. M., and Jacob, T. C. (2018). GABA type a receptor trafficking and the architecture of synaptic inhibition. Dev. Neurobiol. 78, 238–270. doi: 10.1002/dneu.22536
Lu, K. P., and Zhou, X. Z. (2007). The prolyl isomerase PIN1: a pivotal new twist in phosphorylation signalling and disease. Nat. Rev. Mol. Cell Biol. 8, 904–916. doi: 10.1038/nrm2261
Lu, X., Rong, Y., and Baudry, M. (2000). Calpain-mediated degradation of PSD-95 in developing and adult rat brain. Neurosci. Lett. 286, 149–153. doi: 10.1016/s0304-3940(00)01101-0
Lu, Y. M., Mansuy, I. M., Kandel, E. R., and Roder, J. (2000). Calcineurin-mediated LTD of GABAergic inhibition underlies the increased excitability of CA1 neurons associated with LTP. Neuron 26, 197–205. doi: 10.1016/s0896-6273(00)81150-2
Luscher, B., Fuchs, T., and Kilpatrick, C. L. (2011). GABAA receptor trafficking-mediated plasticity of inhibitory synapses. Neuron 70, 385–409. doi: 10.1016/j.neuron.2011.03.024
Marsden, K. C., Beattie, J. B., Friedenthal, J., and Carroll, R. C. (2007). NMDA receptor activation potentiates inhibitory transmission through GABA receptor-associated protein-dependent exocytosis of GABA(A) receptors. J. Neurosci. 27, 14326–14337. doi: 10.1523/JNEUROSCI.4433-07.2007
Marsden, K. C., Shemesh, A., Bayer, K. U., and Carroll, R. C. (2010). Selective translocation of Ca2+/calmodulin protein kinase IIalpha (CaMKIIalpha) to inhibitory synapses. Proc. Natl. Acad. Sci. USA 107, 20559–20564. doi: 10.1073/pnas.1010346107
Martin, S. J., Grimwood, P. D., and Morris, R. G. (2000). Synaptic plasticity and memory: an evaluation of the hypothesis. Annu. Rev. Neurosci. 23, 649–711. doi: 10.1146/annurev.neuro.23.1.649
Matsuyama, S., Taniguchi, T., Kadoyama, K., and Matsumoto, A. (2008). Long-term potentiation-like facilitation through GABAA receptor blockade in the mouse dentate gyrus in vivo. Neuroreport 19, 1809–1813. doi: 10.1097/WNR.0b013e328319ab94
Matsuzaki, M., Honkura, N., Ellis-Davies, G. C. R., and Kasai, H. (2004). Structural basis of long-term potentiation in single dendritic spines. Nature 429, 761–766. doi: 10.1038/nature02617
Maynard, S. A., and Triller, A. (2019). Inhibitory receptor diffusion dynamics. Front. Mol. Neurosci. 12:313. doi: 10.3389/fnmol.2019.00313
Megías, M., Emri, Z., Freund, T. F., and Gulyás, A. I. (2001). Total number and distribution of inhibitory and excitatory synapses on hippocampal CA1 pyramidal cells. Neuroscience 102, 527–540. doi: 10.1016/s0306-4522(00)00496-6
Mejias, R., Adamczyk, A., Anggono, V., Niranjan, T., Thomas, G. M., Sharma, K., et al. (2011). Gain-of-function glutamate receptor interacting protein 1 variants alter GluA2 recycling and surface distribution in patients with autism. Proc. Natl. Acad. Sci. USA 108, 4920–4925. doi: 10.1073/pnas.1102233108
Mele, M., Costa, R. O., and Duarte, C. B. (2019). Alterations in GABAA-Receptor Trafficking and Synaptic Dysfunction in Brain Disorders. Front. Cell. Neurosci. 13:77. doi: 10.3389/fncel.2019.00077
Morales-Weil, K., Moreno, M., Ahumada, J., Arriagada, J., Fuentealba, P., Bonansco, C., et al. (2020). Priming of GABAergic long-term potentiation by muscarinic receptors. Neuroscience 428, 242–251. doi: 10.1016/j.neuroscience.2019.12.033
Muir, J., Arancibia-Carcamo, I. L., MacAskill, A. F., Smith, K. R., Griffin, L. D., and Kittler, J. T. (2010). NMDA receptors regulate GABAA receptor lateral mobility and clustering at inhibitory synapses through serine 327 on the γ2 subunit. Proc. Natl. Acad. Sci. USA 107, 16679–16684. doi: 10.1073/pnas.1000589107
Muir, J., and Kittler, J. T. (2014). Plasticity of GABAA receptor diffusion dynamics at the axon initial segment. Front. Cell. Neurosci. 8:151. doi: 10.3389/fncel.2014.00151
Mulkey, R. M., and Malenka, R. C. (1992). Mechanisms underlying induction of homosynaptic long-term depression in area CA1 of the hippocampus. Neuron 9, 967–975. doi: 10.1016/0896-6273(92)90248-c
Nakamura, Y., Darnieder, L. M., Deeb, T. Z., and Moss, S. J. (2015). Regulation of GABAARs by phosphorylation. Adv. Pharmacol. 72, 97–146. doi: 10.1016/bs.apha.2014.11.008
Nanou, E., and Catterall, W. A. (2018). Calcium channels, synaptic plasticity, and neuropsychiatric disease. Neuron 98, 466–481. doi: 10.1016/j.neuron.2018.03.017
Needs, H. I., Henley, B. S., Cavallo, D., Gurung, S., Modebadze, T., Woodhall, G., et al. (2019). Changes in excitatory and inhibitory receptor expression and network activity during induction and establishment of epilepsy in the rat Reduced Intensity Status Epilepticus (RISE) model. Neuropharmacology 158:107728. doi: 10.1016/j.neuropharm.2019.107728
Neves, G., Cooke, S. F., and Bliss, T. V. P. (2008). Synaptic plasticity, memory and the hippocampus: a neural network approach to causality. Nat. Rev. Neurosci. 9, 65–75. doi: 10.1038/nrn2303
Niwa, F., Bannai, H., Arizono, M., Fukatsu, K., Triller, A., and Mikoshiba, K. (2012). Gephyrin-independent GABA(A)R mobility and clustering during plasticity. PLoS One 7:e36148. doi: 10.1371/journal.pone.0036148
Nuwer, J. L., Brady, M. L., Povysheva, N. V., Coyne, A., and Jacob, T. C. (2021). Sustained treatment with an α5 GABA A receptor negative allosteric modulator delays excitatory circuit development while maintaining GABAergic neurotransmission. Neuropharmacology 197:108724. doi: 10.1016/j.neuropharm.2021.108724
O’Brien, R. J., Kamboj, S., Ehlers, M. D., Rosen, K. R., Fischbach, G. D., and Huganir, R. L. (1998). Activity-dependent modulation of synaptic AMPA receptor accumulation. Neuron 21, 1067–1078. doi: 10.1016/s0896-6273(00)80624-8
Oh, W. C., Parajuli, L. K., and Zito, K. (2015). Heterosynaptic structural plasticity on local dendritic segments of hippocampal CA1 neurons. Cell Rep. 10, 162–169. doi: 10.1016/j.celrep.2014.12.016
Olsen, R. W., Hanchar, H. J., Meera, P., and Wallner, M. (2007). GABAA receptor subtypes: the “one glass of wine” receptors. Alcohol 41, 201–209. doi: 10.1016/j.alcohol.2007.04.006
Ouardouz, M., and Sastry, B. R. (2000). Mechanisms underlying LTP of inhibitory synaptic transmission in the deep cerebellar nuclei. J. Neurophysiol. 84, 1414–1421. doi: 10.1152/jn.2000.84.3.1414
Patterson, M. A., Szatmari, E. M., and Yasuda, R. (2010). AMPA receptors are exocytosed in stimulated spines and adjacent dendrites in a Ras-ERK-dependent manner during long-term potentiation. Proc. Natl. Acad. Sci. USA 107, 15951–15956. doi: 10.1073/pnas.0913875107
Peng, Y.-R., Zeng, S.-Y., Song, H.-L., Li, M.-Y., Yamada, M. K., and Yu, X. (2010). Postsynaptic spiking homeostatically induces cell-autonomous regulation of inhibitory inputs via retrograde signaling. J. Neurosci. 30, 16220–16231. doi: 10.1523/JNEUROSCI.3085-10.2010
Pennacchietti, F., Vascon, S., Nieus, T., Rosillo, C., Das, S., Tyagarajan, S. K., et al. (2017). Nanoscale molecular reorganization of the inhibitory postsynaptic density is a determinant of gabaergic synaptic potentiation. J. Neurosci. 37, 1747–1756. doi: 10.1523/JNEUROSCI.0514-16.2016
Petrini, E. M., and Barberis, A. (2014). Diffusion dynamics of synaptic molecules during inhibitory postsynaptic plasticity. Front. Cell. Neurosci. 8:300. doi: 10.3389/fncel.2014.00300
Petrini, E. M., Ravasenga, T., Hausrat, T. J., Iurilli, G., Olcese, U., Racine, V., et al. (2014). Synaptic recruitment of gephyrin regulates surface GABAA receptor dynamics for the expression of inhibitory LTP. Nat. Commun. 5:3921. doi: 10.1038/ncomms4921
Pribiag, H., Peng, H., Shah, W. A., Stellwagen, D., and Carbonetto, S. (2014). Dystroglycan mediates homeostatic synaptic plasticity at GABAergic synapses. Proc. Natl. Acad. Sci. USA 111, 6810–6815. doi: 10.1073/pnas.1321774111
Purkey, A. M., Woolfrey, K. M., Crosby, K. C., Stich, D. G., Chick, W. S., Aoto, J., et al. (2018). AKAP150 Palmitoylation Regulates Synaptic Incorporation of Ca2+-Permeable AMPA Receptors to Control LTP. Cell Rep. 25, 974–987. doi: 10.1016/j.celrep.2018.09.085
Rácz, B., Blanpied, T. A., Ehlers, M. D., and Weinberg, R. J. (2004). Lateral organization of endocytic machinery in dendritic spines. Nat. Neurosci. 7, 917–918. doi: 10.1038/nn1303
Rajgor, D., Purkey, A. M., Sanderson, J. L., Welle, T. M., Garcia, J. D., Dell’Acqua, M. L., et al. (2020). Local miRNA-Dependent Translational Control of GABAAR Synthesis during Inhibitory Long-Term Potentiation. Cell Rep. 31:107785. doi: 10.1016/j.celrep.2020.107785
Ramachandran, B., Ahmed, S., Zafar, N., and Dean, C. (2015). Ethanol inhibits long-term potentiation in hippocampal CA1 neurons, irrespective of lamina and stimulus strength, through neurosteroidogenesis. Hippocampus 25, 106–118. doi: 10.1002/hipo.22356
Ravasenga, T., Ruben, M., Regio, V., Polenghi, A., Petrini, E. M., and Barberis, A. (2022). Spatial regulation of coordinated excitatory and inhibitory synaptic plasticity at dendritic synapses. Cell Rep. 38:110347. doi: 10.1016/j.celrep.2022.110347
Reuveni, I., Ghosh, S., and Barkai, E. (2017). Real Time Multiplicative Memory Amplification Mediated by Whole-Cell Scaling of Synaptic Response in Key Neurons. PLoS Comput. Biol. 13:e1005306. doi: 10.1371/journal.pcbi.1005306
Roberto, M., and Varodayan, F. P. (2017). Synaptic targets: chronic alcohol actions. Neuropharmacology 122, 85–99. doi: 10.1016/j.neuropharm.2017.01.013
Rudolph, U., and Möhler, H. (2014). GABAA receptor subtypes: therapeutic potential in Down syndrome, affective disorders, schizophrenia, and autism. Annu. Rev. Pharmacol. Toxicol. 54, 483–507. doi: 10.1146/annurev-pharmtox-011613-135947
Saliba, R. S., Gu, Z., Yan, Z., and Moss, S. J. (2009). Blocking L-type voltage-gated Ca2+ channels with dihydropyridines reduces gamma-aminobutyric acid type A receptor expression and synaptic inhibition. J. Biol. Chem. 284, 32544–32550. doi: 10.1074/jbc.M109.040071
Saliba, R. S., Kretschmannova, K., and Moss, S. J. (2012). Activity-dependent phosphorylation of GABAA receptors regulates receptor insertion and tonic current. EMBO J. 31, 2937–2951. doi: 10.1038/emboj.2012.109
Sanderson, J. L., Gorski, J. A., and Dell’Acqua, M. L. (2016). NMDA Receptor-Dependent LTD requires transient synaptic incorporation of Ca2+-Permeable AMPARs Mediated by AKAP150-Anchored PKA and Calcineurin. Neuron 89, 1000–1015. doi: 10.1016/j.neuron.2016.01.043
Sanderson, J. L., Scott, J. D., and Dell’Acqua, M. L. (2018). Control of Homeostatic Synaptic Plasticity by AKAP-Anchored Kinase and Phosphatase Regulation of Ca2+-Permeable AMPA Receptors. J. Neurosci. 38, 2863–2876. doi: 10.1523/JNEUROSCI.2362-17.2018
Sarto-Jackson, I., Milenkovic, I., Smalla, K.-H., Gundelfinger, E. D., Kaehne, T., Herrera-Molina, R., et al. (2012). The cell adhesion molecule neuroplastin-65 is a novel interaction partner of γ-aminobutyric acid type A receptors. J. Biol. Chem. 287, 14201–14214. doi: 10.1074/jbc.M111.293175
Schmitz, L. J. M., Klaassen, R. V., Ruiperez-Alonso, M., Zamri, A. E., Stroeder, J., Rao-Ruiz, P., et al. (2017). The AMPA receptor-associated protein Shisa7 regulates hippocampal synaptic function and contextual memory. eLife 6:192. doi: 10.7554/eLife.24192
Schulz, J. M., Knoflach, F., Hernandez, M.-C., and Bischofberger, J. (2019). Enhanced dendritic inhibition and impaired NMDAR activation in a mouse model of down syndrome. J. Neurosci. 39, 5210–5221. doi: 10.1523/JNEUROSCI.2723-18.2019
Seabrook, G. R., Easter, A., Dawson, G. R., and Bowery, B. J. (1997). Modulation of long-term potentiation in CA1 region of mouse hippocampal brain slices by GABAA receptor benzodiazepine site ligands. Neuropharmacology 36, 823–830. doi: 10.1016/s0028-3908(97)00040-3
Shen, K., and Meyer, T. (1999). Dynamic control of CaMKII translocation and localization in hippocampal neurons by NMDA receptor stimulation. Science 284, 162–166. doi: 10.1126/science.284.5411.162
Shen, Y., Lindemeyer, A. K., Spigelman, I., Sieghart, W., Olsen, R. W., and Liang, J. (2011). Plasticity of GABAA receptors after ethanol pre-exposure in cultured hippocampal neurons. Mol. Pharmacol. 79, 432–442. doi: 10.1124/mol.110.068650
Smalla, K. H., Matthies, H., Langnäse, K., Shabir, S., Böckers, T. M., Wyneken, U., et al. (2000). The synaptic glycoprotein neuroplastin is involved in long-term potentiation at hippocampal CA1 synapses. Proc. Natl. Acad. Sci. USA 97, 4327–4332. doi: 10.1073/pnas.080389297
Smith-Dijak, A. I., Sepers, M. D., and Raymond, L. A. (2019). Alterations in synaptic function and plasticity in Huntington disease. J. Neurochem. 150, 346–365. doi: 10.1111/jnc.14723
Sohal, V. S., and Rubenstein, J. L. R. (2019). Excitation-inhibition balance as a framework for investigating mechanisms in neuropsychiatric disorders. Mol. Psychiatry 24, 1248–1257. doi: 10.1038/s41380-019-0426-0
Song, I., Kamboj, S., Xia, J., Dong, H., Liao, D., and Huganir, R. L. (1998). Interaction of the N-ethylmaleimide-sensitive factor with AMPA receptors. Neuron 21, 393–400. doi: 10.1016/s0896-6273(00)80548-6
Stelzer, A., and Shi, H. (1994). Impairment of GABAA receptor function by N-methyl-D-aspartate-mediated calcium influx in isolated CA1 pyramidal cells. Neuroscience 62, 813–828. doi: 10.1016/0306-4522(94)90479-0
Strack, S., Choi, S., Lovinger, D. M., and Colbran, R. J. (1997). Translocation of autophosphorylated calcium/calmodulin-dependent protein kinase II to the postsynaptic density. J. Biol. Chem. 272, 13467–13470. doi: 10.1074/jbc.272.21.13467
Stuchlik, A. (2014). Dynamic learning and memory, synaptic plasticity and neurogenesis: an update. Front. Behav. Neurosci. 8:106. doi: 10.3389/fnbeh.2014.00106
Südhof, T. C. (2008). Neuroligins and neurexins link synaptic function to cognitive disease. Nature 455, 903–911. doi: 10.1038/nature07456
Sun, C., Zhang, L., and Chen, G. (2013). An unexpected role of neuroligin-2 in regulating KCC2 and GABA functional switch. Mol. Brain 6:23. doi: 10.1186/1756-6606-6-23
Suryanarayanan, A., Liang, J., Meyer, E. M., Lindemeyer, A. K., Chandra, D., Homanics, G. E., et al. (2011). Subunit compensation and plasticity of synaptic GABA(A) receptors induced by ethanol in α4 subunit knockout mice. Front. Neurosci. 5:110. doi: 10.3389/fnins.2011.00110
Swanwick, C. C., Murthy, N. R., and Kapur, J. (2006). Activity-dependent scaling of GABAergic synapse strength is regulated by brain-derived neurotrophic factor. Mol. Cell. Neurosci. 31, 481–492. doi: 10.1016/j.mcn.2005.11.002
Takamiya, K., Mao, L., Huganir, R. L., and Linden, D. J. (2008). The glutamate receptor-interacting protein family of GluR2-binding proteins is required for long-term synaptic depression expression in cerebellar Purkinje cells. J. Neurosci. 28, 5752–5755. doi: 10.1523/JNEUROSCI.0654-08.2008
Taufiq, A. M., Fujii, S., Yamazaki, Y., Sasaki, H., Kaneko, K., Li, J., et al. (2005). Involvement of IP3 receptors in LTP and LTD induction in guinea pig hippocampal CA1 neurons. Learn. Mem. 12, 594–600. doi: 10.1101/lm.17405
Tavalin, S. J., Colledge, M., Hell, J. W., Langeberg, L. K., Huganir, R. L., and Scott, J. D. (2002). Regulation of GluR1 by the A-kinase anchoring protein 79 (AKAP79) signaling complex shares properties with long-term depression. J. Neurosci. 22, 3044–3051.
Thalhammer, A., Rudhard, Y., Tigaret, C. M., Volynski, K. E., Rusakov, D. A., and Schoepfer, R. (2006). CaMKII translocation requires local NMDA receptor-mediated Ca2+ signaling. EMBO J. 25, 5873–5883. doi: 10.1038/sj.emboj.7601420
Thomas, P., Mortensen, M., Hosie, A. M., and Smart, T. G. (2005). Dynamic mobility of functional GABAA receptors at inhibitory synapses. Nat. Neurosci. 8, 889–897. doi: 10.1038/nn1483
Tong, R., Chater, T. E., Emptage, N. J., and Goda, Y. (2021). Heterosynaptic cross-talk of pre- and postsynaptic strengths along segments of dendrites. Cell Rep. 34:108693. doi: 10.1016/j.celrep.2021.108693
Turrigiano, G. G., Leslie, K. R., Desai, N. S., Rutherford, L. C., and Nelson, S. B. (1998). Activity-dependent scaling of quantal amplitude in neocortical neurons. Nature 391, 892–896. doi: 10.1038/36103
Tyagarajan, S. K., Ghosh, H., Yévenes, G. E., Imanishi, S. Y., Zeilhofer, H. U., Gerrits, B., et al. (2013). Extracellular signal-regulated kinase and glycogen synthase kinase 3β regulate gephyrin postsynaptic aggregation and GABAergic synaptic function in a calpain-dependent mechanism. J. Biol. Chem. 288, 9634–9647. doi: 10.1074/jbc.M112.442616
Valentinova, K., and Mameli, M. (2016). mGluR-LTD at Excitatory and Inhibitory Synapses in the Lateral Habenula Tunes Neuronal Output. Cell Rep. 16, 2298–2307. doi: 10.1016/j.celrep.2016.07.064
Varley, Z. K., Pizzarelli, R., Antonelli, R., Stancheva, S. H., Kneussel, M., Cherubini, E., et al. (2011). Gephyrin regulates GABAergic and glutamatergic synaptic transmission in hippocampal cell cultures. J. Biol. Chem. 286, 20942–20951. doi: 10.1074/jbc.M111.234641
Vinade, L., Petersen, J. D., Do, K., Dosemeci, A., and Reese, T. S. (2001). Activation of calpain may alter the postsynaptic density structure and modulate anchoring of NMDA receptors. Synapse 40, 302–309. doi: 10.1002/syn.1053
Virtanen, M. A., Uvarov, P., Mavrovic, M., Poncer, J. C., and Kaila, K. (2021). The multifaceted roles of KCC2 in cortical development. Trends Neurosci. 44, 378–392. doi: 10.1016/j.tins.2021.01.004
Vlachos, A., Reddy-Alla, S., Papadopoulos, T., Deller, T., and Betz, H. (2013). Homeostatic regulation of gephyrin scaffolds and synaptic strength at mature hippocampal GABAergic postsynapses. Cereb. Cortex 23, 2700–2711. doi: 10.1093/cercor/bhs260
Wallner, M., Hanchar, H. J., and Olsen, R. W. (2006). Low dose acute alcohol effects on GABA A receptor subtypes. Pharmacol. Ther. 112, 513–528. doi: 10.1016/j.pharmthera.2006.05.004
Wang, J., Liu, S., Haditsch, U., Tu, W., Cochrane, K., Ahmadian, G., et al. (2003). Interaction of calcineurin and type-A GABA receptor gamma 2 subunits produces long-term depression at CA1 inhibitory synapses. J. Neurosci. 23, 826–836.
Wang, W., Gong, N., and Xu, T.-L. (2006). Downregulation of KCC2 following LTP contributes to EPSP-spike potentiation in rat hippocampus. Biochem. Biophys. Res. Commun. 343, 1209–1215. doi: 10.1016/j.bbrc.2006.03.038
Wang, Y., Briz, V., Chishti, A., Bi, X., and Baudry, M. (2013). Distinct roles for μ-calpain and m-calpain in synaptic NMDAR-mediated neuroprotection and extrasynaptic NMDAR-mediated neurodegeneration. J. Neurosci. 33, 18880–18892. doi: 10.1523/JNEUROSCI.3293-13.2013
Watt, A. J., van Rossum, M. C., MacLeod, K. M., Nelson, S. B., and Turrigiano, G. G. (2000). Activity coregulates quantal AMPA and NMDA currents at neocortical synapses. Neuron 26, 659–670. doi: 10.1016/s0896-6273(00)81202-7
Werner, D. F., Kumar, S., Criswell, H. E., Suryanarayanan, A., Fetzer, J. A., Comerford, C. E., et al. (2011). PKCγ is required for ethanol-induced increases in GABA(A) receptor α4 subunit expression in cultured cerebral cortical neurons. J. Neurochem. 116, 554–563. doi: 10.1111/j.1471-4159.2010.07140.x
Whissell, P. D., Eng, D., Lecker, I., Martin, L. J., Wang, D.-S., and Orser, B. A. (2013). Acutely increasing δGABA(A) receptor activity impairs memory and inhibits synaptic plasticity in the hippocampus. Front. Neural Circ. 7:146. doi: 10.3389/fncir.2013.00146
Wild, A. R., and Dell’Acqua, M. L. (2018). Potential for therapeutic targeting of AKAP signaling complexes in nervous system disorders. Pharmacol. Ther. 185, 99–121. doi: 10.1016/j.pharmthera.2017.12.004
Woodin, M. A., Ganguly, K., and Poo, M. (2003). Coincident pre- and postsynaptic activity modifies GABAergic synapses by postsynaptic changes in Cl- transporter activity. Neuron 39, 807–820. doi: 10.1016/s0896-6273(03)00507-5
Woolfrey, K. M., O’Leary, H., Goodell, D. J., Robertson, H. R., Horne, E. A., Coultrap, S. J., et al. (2018). CaMKII regulates the depalmitoylation and synaptic removal of the scaffold protein AKAP79/150 to mediate structural long-term depression. J. Biol. Chem. 293, 1551–1567. doi: 10.1074/jbc.M117.813808
Wu, K., Castellano, D., Tian, Q., and Lu, W. (2021a). Distinct regulation of tonic GABAergic inhibition by NMDA receptor subtypes. Cell Rep. 37:109960. doi: 10.1016/j.celrep.2021.109960
Wu, K., Han, W., Tian, Q., Li, Y., and Lu, W. (2021b). Activity- and sleep-dependent regulation of tonic inhibition by Shisa7. Cell Rep. 34:108899. doi: 10.1016/j.celrep.2021.108899
Wyroślak, M., Lebida, K., and Mozrzymas, J. W. (2021). Induction of inhibitory synaptic plasticity enhances tonic current by increasing the content of α5-Subunit Containing GABAA Receptors in Hippocampal Pyramidal Neurons. Neuroscience 467, 39–46. doi: 10.1016/j.neuroscience.2021.05.020
Xue, J.-G., Masuoka, T., Gong, X.-D., Chen, K.-S., Yanagawa, Y., Law, S. K. A., et al. (2011). NMDA receptor activation enhances inhibitory GABAergic transmission onto hippocampal pyramidal neurons via presynaptic and postsynaptic mechanisms. J. Neurophysiol. 105, 2897–2906. doi: 10.1152/jn.00287.2010
Yap, E. L., Pettit, N. L., Davis, C. P., Nagy, M. A., Harmin, D. A., Golden, E., et al. (2020). Bidirectional perisomatic inhibitory plasticity of a Fos neuronal network. Nature 590, 115–121. doi: 10.1038/s41586-020-3031-0
Yoshioka, M., Yamazaki, Y., Fujii, S., Kaneko, K., Kato, H., and Mikoshiba, K. (2010). Intracellular calcium ion dynamics involved in long-term potentiation in hippocampal CA1 neurons in mice lacking the IP3 type 1 receptor. Neurosci. Res. 67, 149–155. doi: 10.1016/j.neures.2010.03.002
Zacchi, P., Antonelli, R., and Cherubini, E. (2014). Gephyrin phosphorylation in the functional organization and plasticity of GABAergic synapses. Front. Cell. Neurosci. 8:103. doi: 10.3389/fncel.2014.00103
Keywords: synaptic plasticity, GABA receptor (GABA-R), glutamate, NMDA receptor, LTP (long term potentiation), LTD (long term depression), calcium, activity
Citation: Chapman CA, Nuwer JL and Jacob TC (2022) The Yin and Yang of GABAergic and Glutamatergic Synaptic Plasticity: Opposites in Balance by Crosstalking Mechanisms. Front. Synaptic Neurosci. 14:911020. doi: 10.3389/fnsyn.2022.911020
Received: 01 April 2022; Accepted: 26 April 2022;
Published: 19 May 2022.
Edited by:
Leonardo Pignataro, The City University of New York, United StatesReviewed by:
Andrea Barberis, Italian Institute of Technology (IIT), ItalyCarlos Fernando Valenzuela, University of New Mexico, United States
Copyright © 2022 Chapman, Nuwer and Jacob. This is an open-access article distributed under the terms of the Creative Commons Attribution License (CC BY). The use, distribution or reproduction in other forums is permitted, provided the original author(s) and the copyright owner(s) are credited and that the original publication in this journal is cited, in accordance with accepted academic practice. No use, distribution or reproduction is permitted which does not comply with these terms.
*Correspondence: Tija C. Jacob, dGNqMTFAcGl0dC5lZHU=
†These authors have contributed equally to this work and share first authorship