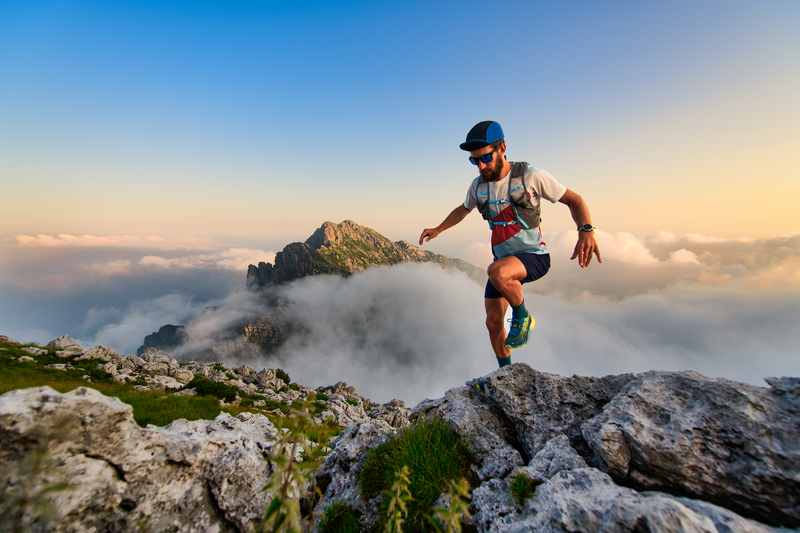
95% of researchers rate our articles as excellent or good
Learn more about the work of our research integrity team to safeguard the quality of each article we publish.
Find out more
MINI REVIEW article
Front. Synaptic Neurosci. , 06 January 2023
Volume 14 - 2022 | https://doi.org/10.3389/fnsyn.2022.1087042
This article is part of the Research Topic Synaptic Plasticity and Dysfunction, Friend or Foe? View all 10 articles
Sensory loss leads to widespread adaptation of neural circuits to mediate cross-modal plasticity, which allows the organism to better utilize the remaining senses to guide behavior. While cross-modal interactions are often thought to engage multisensory areas, cross-modal plasticity is often prominently observed at the level of the primary sensory cortices. One dramatic example is from functional imaging studies in humans where cross-modal recruitment of the deprived primary sensory cortex has been observed during the processing of the spared senses. In addition, loss of a sensory modality can lead to enhancement and refinement of the spared senses, some of which have been attributed to compensatory plasticity of the spared sensory cortices. Cross-modal plasticity is not restricted to early sensory loss but is also observed in adults, which suggests that it engages or enables plasticity mechanisms available in the adult cortical circuit. Because adult cross-modal plasticity is observed without gross anatomical connectivity changes, it is thought to occur mainly through functional plasticity of pre-existing circuits. The underlying cellular and molecular mechanisms involve activity-dependent homeostatic and Hebbian mechanisms. A particularly attractive mechanism is the sliding threshold metaplasticity model because it innately allows neurons to dynamically optimize their feature selectivity. In this mini review, I will summarize the cellular and molecular mechanisms that mediate cross-modal plasticity in the adult primary sensory cortices and evaluate the metaplasticity model as an effective framework to understand the underlying mechanisms.
Cross-modal plasticity refers to changes in brain function following a sensory loss that allows the spared senses to be used more effectively to guide behavior. There are two main changes: cross-modal recruitment of the brain areas that serve the lost sensory modality and compensatory plasticity of the brain areas that process the spared senses (Figure 1). Cross-modal recruitment has been observed in blind subjects where braille reading activates the deprived visual cortex (Sadato et al., 1996; Buchel et al., 1998; Burton et al., 2002b) and in deaf subjects where visual stimuli activate the deprived auditory cortex (Sandmann et al., 2012). This functional cross-modal recruitment of the deprived cortices is thought to enhance the processing of the remaining senses by increasing the cortical territory. This idea stems from the notion that cortical circuits are functionally equivalent and can process any sensory information presented to them. One of the supporting evidence for the functional equivalence of cortical circuits comes from a study, which rewired visual inputs to the primary auditory cortex (A1) of ferrets during early development causing visually guided behavior to become dependent on A1 (von Melchner et al., 2000). However, primary sensory cortices can be quite specialized in their anatomical organization, for example, barrel cortex in rodents and ocular dominance columns present in primary visual cortex (V1) of some carnivores and primates. Whether such anatomical specializations may affect the functional equivalence of cortical processing is unclear. In addition to the cross-modal recruitment, compensatory plasticity of the spared sensory areas is thought to allow refinement and increase the sensitivity of the spared sensory systems (Pascual-Leone and Torres, 1993; Sterr et al., 1998a,b; Roder et al., 1999; Elbert et al., 2002).
Figure 1. Synaptic changes associated with adult cross-modal plasticity. Sensory loss produces compensatory plasticity in the spared sensory cortices and cross-modal recruitment plasticity in the deprive sensory cortex. Lower panels show compilation of synaptic plasticity data from adult mice after vision loss (Petrus et al., 2014, 2015; Whitt et al., 2022). (Lower right panel) In the deprived primary visual cortex (V1), there is no plasticity of the feedforward (thalamocortical inputs to L4 and L4–L2/3) or the thalamic circuit, but lateral inputs to L2/3 potentiate. There is no change in parvalbumin (PV) inhibition onto L4 or L2/3 neurons. Corticothalamic synapses from V1 to thalamic reticular nucleus (TRN) or the primary visual thalamus [dorsal lateral geniculate nucleus (dLGN)] did not change. Adaptation of V1 circuit could allow cross-modal recruitment of V1 for processing other sensory inputs arriving through the potentiated lateral inputs to L2/3. (Lower left panel) In the spared primary auditory cortex (A1), there is potentiation of the feedforward synapses, including thalamocortical synapses and L4–L2/3 synapses. The strength of lateral inputs to L2/3 is weakened. Cortical inhibition mediated by PV neurons becomes stronger in L4 but does not change in L2/3. In addition, there is plasticity in the thalamic circuit, where TRN inhibition to the primary auditory thalamic nucleus [ventral portion of medial geniculate body (MGBv)] is reduced. Corticothalamic synapses to MGBv were not altered. Such adaptation of A1 is expected to amplify auditory signals and allow sharpening of tuning properties to mediate compensatory plasticity. Plasticity of synaptic strength following vision loss is color coded as shown in the insets. Pink, potentiated synapses; Blue, depressed synapses; Yellow: no change in synaptic strength. Triangles, excitatory (Ex) synapses; Circles, inhibitory (In) synapses.
In animal models, early sensory loss results in cross-modal anatomical changes to the primary sensory cortices, especially of thalamocortical inputs (Henschke et al., 2018; Dooley and Krubitzer, 2019), but in adults, most of the cross-modal plasticity likely occurs through functional plasticity of pre-existing circuits (Lee and Whitt, 2015; Ewall et al., 2021). Cross-modal functional plasticity is observed in both the deprived and the spared primary sensory cortices (Figure 1). Evidence from animal studies suggests that these involve experience-dependent plasticity mechanisms based on Hebbian, both long-term potentiation (LTP) and long-term depression (LTD), and homeostatic mechanisms (Lee and Whitt, 2015; Ewall et al., 2021). In this mini-review, I will summarize the synaptic plasticity mechanisms of adult cross-modal plasticity and explain how utilizing the framework of the metaplasticity model can easily account for the global cortical adaptation in adults. To do this, I will first provide a brief introduction to the sliding threshold metaplasticity model.
Metaplasticity refers to the regulation of synaptic plasticity (Abraham and Bear, 1996) and often refers to the sliding threshold model proposed by Bienenstock, Cooper, and Monroe (BCM theory); a theoretical model that can provide stability to Hebbian plasticity (Bienenstock et al., 1982; Bear et al., 1987; Cooper and Bear, 2012). It was recognized that synaptic plasticity solely based on Hebbian mechanisms is limited and is unable to provide network stability that is necessary for neural function on its own. This is due to the fact that correlation based synaptic plasticity mechanisms, such as LTP and LTD, have in-built positive feedback. Strengthening synapse by LTP causes postsynaptic neurons to respond to inputs more strongly, which increases the coincidence of pre- and post-synaptic activity. This in turn promotes further LTP leading to over-excitability when left unchecked. Similar positive feedback occurs upon weakening synapses via LTD, but in the opposite direction that ultimately leads to inactivity. Such bistable property of Hebbian plasticity on neural network activity suggests a need for homeostatic control to provide stability. The sliding threshold model allows such homeostatic control by postulating that the induction threshold for LTP and LTD, referred to as the synaptic modification threshold (θm), slides as a function of the integrated past activity of a neuron (Figure 2). This key property of the sliding threshold allows neurons to dynamically tune their feature selectivity to the dominant input at the moment as a function of the history of overall activity (Bienenstock et al., 1982; Bear et al., 1987; Cooper and Bear, 2012). Here I will discuss the two main properties of the BCM model: the generation of feature selectivity and its dynamic regulation by past activity that endows homeostasis.
Figure 2. Metaplasticity sliding threshold model to explain adult cross-modal plasticity. Sliding threshold model can account for synaptic plasticity observed in the deprived sensory cortex (cross-modal recruitment) and the spared sensory cortices (compensatory plasticity). (Lower right panel) The sliding threshold model states that when past activity is low, the synaptic modification threshold (θm) slides down (from θM–θM′). This is expected to be the case in primary visual cortex (V1) with vision loss. Lower θm (θM′) now allows the second strongest input (cyan, 2), which carries non-visual input, to potentiate. This may recruit V1 to process other sensory inputs in the absence of vision. The weakest input (green, 3) is still below the lowered θm (θM′), hence stay in the LTD zone. This allows the neurons to maintain selectivity for the newly adopted feature (cyan, 2). (Lower left panel) According to the sliding threshold model, an increase in past activity slides up θm (from θM–θM″) to limit LTP induction and increase the range in which LTD can be induced. Vision loss in adults potentiates feedforward synapses in primary auditory cortex (A1) and reduces inhibition in the auditory thalamus (Figure 1), which is predicted to increase input activity to A1 L2/3 leading to a higher θm (θM″). This causes activity from only the strongest input (pink, 1) to exceed the new θm (θM″) to produce LTP, while the second strongest input (cyan, 2) now falls below the new θm (θM″) and undergoes LTD. This in essence makes A1 neurons more selective, which would aid in the discrimination of auditory signals. Because the sliding threshold maintains a range for LTP and LTD, it is ideally suited to dynamically allow neurons to adopt new features in the absence of their main input to mediate cross-modal recruitment or become more feature selective when there is an increase in overall activity as would occur during compensatory plasticity. Filled upward pointing arrows, inputs with activity that surpasses θm, which will undergo LTP. Open downward pointing arrows, inputs with activity that falls below θm, which will undergo LTD.
One of the main features of the sliding threshold model is the development of feature selectivity by LTP and LTD. Stronger inputs that exceed the θm undergo LTP, while weaker inputs that fail to produce sufficient postsynaptic activity are depressed via LTD. Thus neurons “select” the dominant input, at the expense of other weaker inputs. The ability of sensory cortical neurons to express feature selectivity is critical for sensory processing by enabling discrimination of distinct inputs to generate a percept of a certain feature in the sensory environment. While the initial setup of feature selectivity arises from the developmental organization of axonal projections based on guidance cues (Crowley and Katz, 1999, 2000), the refinement of feature selectivity is known to depend on the activity and in particular sensory experience (Sengpiel and Kind, 2002; Hooks and Chen, 2020). An essential feature of the sliding threshold is its ability to provide network stability, which is endowed by the regulation of θm as a function of the integrated past activity. If the neuron has been under high activity regime for a duration of time, θm increases (i.e., slides up) to make LTP more difficult to induce and promote LTD across the majority of synapses. Weakening the majority of the inputs via LTD causes the overall postsynaptic activity to decrease, hence providing homeostatic control of neural activity. In contrast, if the postsynaptic neuronal activity is low, θm is reduced to promote LTP and decreases the range of activity that produces LTD. While stabilizing the neural activity, the sliding threshold model preserves feature selectivity because stronger inputs will surpass θm to strengthen their connections at the expense of weaker inputs whether the θm is high or low. Implementing the sliding threshold to computational neural networks successfully explains the development of feature selectivity based on LTP/LTD while maintaining network stability (Bienenstock et al., 1982). As will be discussed later sliding threshold also enables dynamic regulation of the neuronal feature selectivity when there are changes to the type of inputs available to the neurons (Figure 2). The sliding threshold model was first experimentally demonstrated in V1, where reducing overall neural activity by dark rearing from birth promotes LTP and reduces LTD (Kirkwood et al., 1996). Since then, it has been confirmed across many brain regions (Lebel et al., 2001; Krucker et al., 2002; Solger et al., 2004; Muller et al., 2007; Narayanan and Johnston, 2010; Hulme et al., 2012) and malfunctioning of the sliding threshold has been reported in various neurological disease models (Hulme et al., 2013; Megill et al., 2015; Jang and Chung, 2016) suggesting its critical role in normal brain function.
At a molecular level, the sliding threshold is implemented by alterations in either the induction or the expression mechanisms of LTP/LTD. The initial postulate of the sliding threshold model stated a horizontal shift of the synaptic modification function (Figure 2), but now there is evidence that the synaptic modification function could shift vertically (Seol et al., 2007; Huang et al., 2012; Hong et al., 2020). Both the horizontal and vertical shift in the synaptic modification function slides the θm in the same manner, but the molecular mechanisms underlying the two are distinct. The horizontal shift in synaptic modification occurs by changes in the induction mechanisms of LTP/D, which in molecular terms has been demonstrated as changes in N-methyl-D-aspartate (NMDA) receptor (NMDAR) subunit composition. Low neuronal activity upregulates the expression of NMDAR GluN2B (or NR2B) subunit (Quinlan et al., 1999; Philpot et al., 2001, 2003), which confers longer current duration compared to GluN2A (or NR2A) containing NMDARs (Chen et al., 1999; Rumbaugh and Vicini, 1999). This in essence allows larger intracellular Ca2+ increase through NMDARs to promote LTP. With heightened neuronal activity, GluN2A becomes the dominant subunit for NMDARs, which increases the θm to make LTP induction more stringent. In contrast, the vertical shift in synaptic modification (also referred to as the Pull-Push model) has been shown to occur with neuromodulators acting on LTP/LTD expression mechanisms (Seol et al., 2007; Huang et al., 2012; Hong et al., 2020; Mihalas et al., 2021). Neuromodulators linked to cyclic adenosine 3′,5′-monophosphate (cAMP) signaling promote LTP by increasing phosphorylation of α-amino-3-hydroxy-5-methyl-4-isoxazolepropionic acid receptors (AMPARs), especially on the protein kinase A target serine-845 (S845) residue of the GluA1 subunit to promote LTP (Seol et al., 2007; Qian et al., 2012). GluA1-S845 phosphorylation has been shown to increase the content of cell surface AMPARs (Oh et al., 2006; He et al., 2011) and prime AMPARs for synaptic recruitment by LTP (Lee and Kirkwood, 2011; Diering and Huganir, 2018). In contrast, neuromodulators linked to phospholipase C (PLC) slide up the θm by promoting LTD and preventing LTP expression (Huang et al., 2012). The main difference between the horizontal and vertical shift in synaptic modification function is that the latter puts synapses into an LTP-only or an LTD-only mode while the former preserves the full range of LTP and LTD (Figure 2).
Next, I will review the synaptic mechanisms of cross-modal plasticity in the adult primary sensory cortices and discuss how the horizontal shift in synaptic modification function could account for the observed findings.
In the adult primary sensory cortices, the superficial layers (L2/3) are likely a major substrate for adult cross-modal plasticity. L2/3 synapses retain their ability to undergo LTP and LTD as well as homeostatic synaptic plasticity into adulthood (Goel and Lee, 2007; Jiang et al., 2007). This contrasts plasticity in L4, where thalamocortical LTP/LTD and homeostatic synaptic plasticity display a short early critical period for plasticity (Crair and Malenka, 1995; Feldman et al., 1998; Desai et al., 2002; Jiang et al., 2007; Barkat et al., 2011; Rodriguez et al., 2018). Moreover, the functional connectivity of the L2/3 neurons is well poised to integrate top-down contextual information with the bottom-up sensory responses from thalamorecipient neurons in L4. Besides feedforward inputs from L4, L2/3 principal neurons receive long-range inputs from multisensory association cortices and higher order sensory cortices, direct connections from other primary sensory cortices, as well as inputs from higher order thalamic nuclei that carry multisensory information (Ewall et al., 2021).
Even a short duration of sensory loss impacts normal development (Sengpiel and Kind, 2002; Hooks and Chen, 2020), but there is some degree of plasticity in adult sensory cortices (Lee and Whitt, 2015; Ribic, 2020), especially in the superficial layers. For example, a couple of days of complete visual deprivation leads to homeostatic strengthening of excitatory synapses measured as miniature excitatory postsynaptic currents (mEPSCs) in adult V1 L2/3 neurons (Goel and Lee, 2007). These changes can be interpreted in the framework of synaptic scaling (Turrigiano and Nelson, 2004; Turrigiano, 2008) or sliding threshold (Ewall et al., 2021). Synaptic scaling model would allow network activity (i.e., firing rate) homeostasis that can prevent overall silencing of the cortical network caused by lost inputs, while sliding threshold would enable dynamic remapping of neuronal features in addition to activity homeostasis. Synaptic scaling and sliding threshold likely operate across different activity regimes to maintain network homeostasis (Lee and Kirkwood, 2019).
Key distinguishing features of synaptic scaling and sliding threshold model are dependence on activity, especially of NMDARs, and input-specific nature of plasticity. Inactivity-driven synaptic scaling was first demonstrated in neuronal cultures upon pharmacological blockade of activity using tetrodotoxin (TTX) or AMPAR blockers (O’Brien et al., 1998; Turrigiano et al., 1998). Subsequent studies demonstrated that NMDAR blockade can accelerate the rate of scaling up synapses with TTX (Sutton et al., 2006). In contrast, according to the sliding threshold model, while the sliding of the θm can happen in the absence of activity, the manifestation of changes in AMPAR-mediated synaptic transmission requires LTP or LTD that is dependent on NMDAR activity (Cooper and Bear, 2012). It is demonstrated that the potentiation of mEPSCs in V1 L2/3 following visual deprivation is blocked by NMDAR antagonist application (Rodriguez et al., 2019) and in particular by blockers of GluN2B (Bridi et al., 2018). These data support the sliding threshold model and are at odds with the synaptic scaling model. Furthermore, the potentiation of mEPSCs in V1 L2/3 neurons following visual deprivation is not multiplicative across all synapses in adults (Goel and Lee, 2007) and is specific to lateral inputs but not observed at feedforward inputs from L4 (Petrus et al., 2015; Chokshi et al., 2019; Figure 1). These results corroborate that in intact cortical circuits with distinct inputs that carry different patterns of activity, homeostatic adaptation is not uniform across all the synapses. Such input-specific and NMDAR-dependent plasticity supports the sliding threshold model, but we cannot exclude the role of synaptic scaling especially with extreme changes in activity (Lee and Kirkwood, 2019).
According to the sliding threshold model, loss of a major input, such as visual input to V1 neurons, is expected to decrease the overall neuronal activity (Figure 2). If this persists, the theory states that θm will slide down, which allows a subset of previously weak inputs to cross the lowered θm and strengthen via LTP. This allows V1 neurons, devoid of visual inputs, to adopt these newly potentiated inputs as their main driver. Inputs with activity below the new θm will still undergo LTD, which permits V1 neurons to maintain selectivity to the newly adopted inputs. Therefore, the sliding threshold model enables neurons to dynamically adopt new features based on the changes in the landscape of sensory experience. Particularly for cross-modal plasticity, the metaplasticity model allows V1 neurons to respond to non-visual activity carried by the newly potentiated inputs. This could serve as a substrate for cross-modal recruitment observed in blind subjects (Sadato et al., 1996; Buchel et al., 1998; Burton et al., 2002a; Sandmann et al., 2012). While the main function of the primary sensory cortices is to process their respective sensory information, multisensory information is readily available at this early stage of sensory processing. Indeed, in vivo whole-cell recordings demonstrate subthreshold functional connections between primary sensory cortices (Iurilli et al., 2012). Furthermore, these subthreshold cortico-cortical connections provide multisensory modulation of the primary inputs. For example, loud sound sharpens the orientation tuning of L2/3 V1 neurons via direct input from A1 L5 neurons (Ibrahim et al., 2016; Deneux et al., 2019). Conceivable then, visual deprivation-induced sliding down of θm in V1 L2/3 neurons could allow these subthreshold A1 inputs to potentiate, thus enabling recruitment of V1 for auditory processing.
Plasticity of the spared primary sensory cortices is thought to refine the processing of the spared sensory inputs, improving discrimination, and detection. At a cellular level, cross-modal sensory loss strengthens feedforward connections of spared primary sensory cortices (Figure 1). For example, depriving vision of adult mice potentiates the thalamocortical synapses in L4 and L4–L2/3 synapses in A1 (Petrus et al., 2014, 2015) and potentiates L4–L2/3 synapses in the rat barrel cortex (Jitsuki et al., 2011). Similarly, deafening adult mice strengthens thalamocortical synapses in V1 L4 (Petrus et al., 2014; Rodriguez et al., 2018). The potentiation of thalamocortical synapses in adults is of interest because both thalamocortical LTP and LTD were shown to be restricted to an early postnatal critical period (Crair and Malenka, 1995; Feldman et al., 1998; Desai et al., 2002; Jiang et al., 2007; Barkat et al., 2011). Cross-modal potentiation of thalamocortical synapses is likely to occur via recovery of NMDAR-dependent thalamocortical LTP in adults (Rodriguez et al., 2018), but the mechanism is currently unknown.
L4–L2/3 synapses, unlike thalamocortical synapses, retain plasticity in adults (Goel and Lee, 2007; Jiang et al., 2007). The potentiation of the feedforward inputs in the spared sensory cortices suggests that feedforward activity increases to a level above θm to produce LTP. For cross-modal plasticity in A1, the evidence suggests a central mechanism that may amplify auditory activity. This may be achieved by a targeted reduction in thalamic inhibition (Whitt et al., 2022). Specifically, depriving vision of adult mice caused a selective reduction of thalamic reticular nucleus (TRN) inhibition to the auditory thalamus [ventral portion of medial geniculate body (MGBv)] but not to the visual thalamus [dorsal lateral geniculate nucleus (dLGN)] (Figure 1). Such reduction in inhibition is expected to increase feedforward activity to A1, which would exceed the θm to induce LTP of feedforward synapses (Figure 2).
Concomitantly, cross-modal sensory deprivation reduces the strength of lateral intracortical synapses to L2/3 neurons (Petrus et al., 2015; Figure 1). The cross-modal synaptic depression of the lateral inputs in L2/3 of the spared sensory cortices can be explained by an increase in θm that results from enhanced feedforward activity (Figure 2). Cross-modal sensory deprivation-induced increase in θm would induce LTD in the majority of inputs as their activity will now fall within the LTD range below the new θm. This theoretically can explain the observed depression of the lateral excitatory inputs (Petrus et al., 2015), which by definition will produce weaker activity compared to the feedforward inputs. At a functional level, the decrease in the θm results in enhanced feature selectivity of the neurons in the spared sensory cortices, because only the few highly active inputs will cross the θm to remain strengthened to drive postsynaptic firing (Figure 2). The spared cortical circuit also exhibits increased inhibition from parvalbumin (PV) interneurons (Petrus et al., 2015), which will aid in the sharpening of the feature selectivity.
The sliding threshold model is compatible with experimental observations of synaptic plasticity related to both cross-modal recruitment and compensatory plasticity. Viewing cross-modal plasticity in the framework of sliding threshold presents testable predictions. Because the synaptic plasticity is ultimately driven by NMDAR-dependent Hebbian modification in accordance with the newly defined synaptic modification threshold, it suggests that cross-modal plasticity will be dependent on sensory experience in the spared modalities. Such requirement of experience may explain the varied observations of the outcome of cross-modal plasticity in human subjects, especially when a sensory modality is lost later in life (Frasnelli et al., 2011; Lazzouni and Lepore, 2014). Furthermore, observations that pre-existing functional connections across the sensory cortices remain plasticity even in adults suggest that cross-modal plasticity mechanisms could be used for enhancing adult brain function.
A1, primary auditory cortex; AMPAR, α-amino-3-hydroxy-5-methyl-4-isoxazolepropionic acid receptor; cAMP, cyclic adenosine 3′,5′-monophosphate; dLGN, dorsal lateral geniculate nucleus; GluN2A, glutamate ionotropic receptor NMDA type 2A; GluN2B, glutamate ionotropic receptor NMDA type subunit 2B; L2/3, layer 2/3; L4, layer 4; L5, layer 5; LTD, long-term depression; LTP, long-term potentiation; mEPSCs, miniature excitatory postsynaptic currents; MGBv, ventral portion of medial geniculate body; NMDAR, N-methyl-D-aspartate (NMDA) receptor; NR2A, NMDAR 2A subunit; NR2B, NMDAR 2B subunit; PLC, phospholipase C; TRN, thalamic reticular nucleus; TTX, tetrodotoxin; V1, primary visual cortex; θm, synaptic modification threshold.
H-KL synthesized and conceptualized the ideas and wrote the manuscript.
This work was supported by the NIH grant R01-EY014882 to H-KL.
We thank Dr. Alfredo Kirkwood for helpful discussions.
The author declares that the research was conducted in the absence of any commercial or financial relationships that could be construed as a potential conflict of interest.
All claims expressed in this article are solely those of the authors and do not necessarily represent those of their affiliated organizations, or those of the publisher, the editors and the reviewers. Any product that may be evaluated in this article, or claim that may be made by its manufacturer, is not guaranteed or endorsed by the publisher.
Abraham, W. C., and Bear, M. F. (1996). Metaplasticity: The plasticity of synaptic plasticity. Trends Neurosci. 19, 126–130. doi: 10.1016/S0166-2236(96)80018-X
Barkat, T. R., Polley, D. B., and Hensch, T. K. (2011). A critical period for auditory thalamocortical connectivity. Nat. Neurosci. 14, 1189–1194. doi: 10.1038/nn.2882
Bear, M. F., Cooper, L. N., and Ebner, F. F. (1987). A physiological basis for a theory of synapse modification. Science 237, 42–48. doi: 10.1126/science.3037696
Bienenstock, E. L., Cooper, L. N., and Munro, P. W. (1982). Theory for the development of neuron selectivity: Orientation specificity and binocular interaction in visual cortex. J. Neurosci. 2, 32–48. doi: 10.1523/JNEUROSCI.02-01-00032.1982
Bridi, M. C. D., De Pasquale, R., Lantz, C. L., Gu, Y., Borrell, A., Choi, S. Y., et al. (2018). Two distinct mechanisms for experience-dependent homeostasis. Nat. Neurosci. 21, 843–850. doi: 10.1038/s41593-018-0150-0
Buchel, C., Price, C., Frackowiak, R. S., and Friston, K. (1998). Different activation patterns in the visual cortex of late and congenitally blind subjects. Brain 121(Pt 3), 409–419. doi: 10.1093/brain/121.3.409
Burton, H., Snyder, A. Z., Diamond, J. B., and Raichle, M. E. (2002b). Adaptive changes in early and late blind: A FMRI study of verb generation to heard nouns. J. Neurophysiol. 88, 3359–3371. doi: 10.1152/jn.00129.2002
Burton, H., Snyder, A. Z., Conturo, T. E., Akbudak, E., Ollinger, J. M., and Raichle, M. E. (2002a). Adaptive changes in early and late blind: A fMRI study of Braille reading. J. Neurophysiol. 87, 589–607. doi: 10.1152/jn.00285.2001
Chen, N., Luo, T., and Raymond, L. A. (1999). Subtype-dependence of NMDA receptor channel open probability. J. Neurosci. 19, 6844–6854. doi: 10.1523/JNEUROSCI.19-16-06844.1999
Chokshi, V., Gao, M., Grier, B. D., Owens, A., Wang, H., Worley, P. F., et al. (2019). Input-specific metaplasticity in the visual cortex requires Homer1a-mediated mGluR5 signaling. Neuron 104, 736–748.e6. doi: 10.1016/j.neuron.2019.08.017
Cooper, L. N., and Bear, M. F. (2012). The BCM theory of synapse modification at 30: Interaction of theory with experiment. Nat. Rev. Neurosci. 13, 798–810. doi: 10.1038/nrn3353
Crair, M. C., and Malenka, R. C. (1995). A critical period for long-term potentiation at thalamocortical synapses. Nature 375, 325–328. doi: 10.1038/375325a0
Crowley, J. C., and Katz, L. C. (1999). Development of ocular dominance columns in the absence of retinal input. Nat. Neurosci. 2, 1125–1130. doi: 10.1038/16051
Crowley, J. C., and Katz, L. C. (2000). Early development of ocular dominance columns. Science 290, 1321–1324. doi: 10.1126/science.290.5495.1321
Deneux, T., Harrell, E. R., Kempf, A., Ceballo, S., Filipchuk, A., and Bathellier, B. (2019). Context-dependent signaling of coincident auditory and visual events in primary visual cortex. Elife 8:e44006. doi: 10.7554/eLife.44006
Desai, N. S., Cudmore, R. H., Nelson, S. B., and Turrigiano, G. G. (2002). Critical periods for experience-dependent synaptic scaling in visual cortex. Nat. Neurosci. 5, 783–789. doi: 10.1038/nn878
Diering, G. H., and Huganir, R. L. (2018). The AMPA receptor code of synaptic plasticity. Neuron 100, 314–329. doi: 10.1016/j.neuron.2018.10.018
Dooley, J. C., and Krubitzer, L. A. (2019). Alterations in cortical and thalamic connections of somatosensory cortex following early loss of vision. J. Comp. Neurol. 527, 1675–1688. doi: 10.1002/cne.24582
Elbert, T., Sterr, A., Rockstroh, B., Pantev, C., Muller, M. M., and Taub, E. (2002). Expansion of the tonotopic area in the auditory cortex of the blind. J. Neurosci. 22, 9941–9944. doi: 10.1523/JNEUROSCI.22-22-09941.2002
Ewall, G., Parkins, S., Lin, A., Jaoui, Y., and Lee, H.-K. (2021). Cortical and subcortical circuits for cross-modal plasticity induced by loss of vision. Front. Neural Circuits 15:665009. doi: 10.3389/fncir.2021.665009
Feldman, D. E., Nicoll, R. A., Malenka, R. C., and Isaac, J. T. (1998). Long-term depression at thalamocortical synapses in developing rat somatosensory cortex. Neuron 21, 347–357. doi: 10.1016/S0896-6273(00)80544-9
Frasnelli, J., Collignon, O., Voss, P., and Lepore, F. (2011). Crossmodal plasticity in sensory loss. Prog. Brain Res. 191, 233–249. doi: 10.1016/B978-0-444-53752-2.00002-3
Goel, A., and Lee, H. K. (2007). Persistence of experience-induced homeostatic synaptic plasticity through adulthood in superficial layers of mouse visual cortex. J. Neurosci. 27, 6692–6700. doi: 10.1523/JNEUROSCI.5038-06.2007
He, K., Goel, A., Ciarkowski, C. E., Song, L., and Lee, H. K. (2011). Brain area specific regulation of synaptic AMPA receptors by phosphorylation. Commun. Integr. Biol. 4, 569–572. doi: 10.4161/cib.15890
Henschke, J. U., Oelschlegel, A. M., Angenstein, F., Ohl, F. W., Goldschmidt, J., Kanold, P. O., et al. (2018). Early sensory experience influences the development of multisensory thalamocortical and intracortical connections of primary sensory cortices. Brain Struct. Funct. 223, 1165–1190. doi: 10.1007/s00429-017-1549-1
Hong, S. Z., Huang, S., Severin, D., and Kirkwood, A. (2020). Pull-push neuromodulation of cortical plasticity enables rapid bi-directional shifts in ocular dominance. Elife 9:e54455. doi: 10.7554/eLife.54455
Hooks, B. M., and Chen, C. (2020). Circuitry underlying experience-dependent plasticity in the mouse visual system. Neuron 106, 21–36. doi: 10.1016/j.neuron.2020.01.031
Huang, S., Trevino, M., He, K., Ardiles, A., Pasquale, R., Guo, Y., et al. (2012). Pull-push neuromodulation of LTP and LTD enables bidirectional experience-induced synaptic scaling in visual cortex. Neuron 73, 497–510. doi: 10.1016/j.neuron.2011.11.023
Hulme, S. R., Jones, O. D., and Abraham, W. C. (2013). Emerging roles of metaplasticity in behaviour and disease. Trends Neurosci. 36, 353–362. doi: 10.1016/j.tins.2013.03.007
Hulme, S. R., Jones, O. D., Ireland, D. R., and Abraham, W. C. (2012). Calcium-dependent but action potential-independent BCM-like metaplasticity in the hippocampus. J. Neurosci. 32, 6785–6794. doi: 10.1523/JNEUROSCI.0634-12.2012
Ibrahim, L. A., Mesik, L., Ji, X. Y., Fang, Q., Li, H. F., Li, Y. T., et al. (2016). Cross-modality sharpening of visual cortical processing through layer-1-mediated inhibition and disinhibition. Neuron 89, 1031–1045. doi: 10.1016/j.neuron.2016.01.027
Iurilli, G., Ghezzi, D., Olcese, U., Lassi, G., Nazzaro, C., Tonini, R., et al. (2012). Sound-driven synaptic inhibition in primary visual cortex. Neuron 73, 814–828. doi: 10.1016/j.neuron.2011.12.026
Jang, S. S., and Chung, H. J. (2016). Emerging link between Alzheimer’s disease and homeostatic synaptic plasticity. Neural Plast. 2016:7969272. doi: 10.1155/2016/7969272
Jiang, B., Trevino, M., and Kirkwood, A. (2007). Sequential development of long-term potentiation and depression in different layers of the mouse visual cortex. J. Neurosci. 27, 9648–9652. doi: 10.1523/JNEUROSCI.2655-07.2007
Jitsuki, S., Takemoto, K., Kawasaki, T., Tada, H., Takahashi, A., Becamel, C., et al. (2011). Serotonin mediates cross-modal reorganization of cortical circuits. Neuron 69, 780–792. doi: 10.1016/j.neuron.2011.01.016
Kirkwood, A., Rioult, M. C., and Bear, M. F. (1996). Experience-dependent modification of synaptic plasticity in visual cortex. Nature 381, 526–528. doi: 10.1038/381526a0
Krucker, T., Siggins, G. R., Mcnamara, R. K., Lindsley, K. A., Dao, A., Allison, D. W., et al. (2002). Targeted disruption of RC3 reveals a calmodulin-based mechanism for regulating metaplasticity in the hippocampus. J. Neurosci. 22, 5525–5535. doi: 10.1523/JNEUROSCI.22-13-05525.2002
Lazzouni, L., and Lepore, F. (2014). Compensatory plasticity: Time matters. Front. Hum. Neurosci. 8:340. doi: 10.3389/fnhum.2014.00340
Lebel, D., Grossman, Y., and Barkai, E. (2001). Olfactory learning modifies predisposition for long-term potentiation and long-term depression induction in the rat piriform (olfactory) cortex. Cereb. Cortex 11, 485–489. doi: 10.1093/cercor/11.6.485
Lee, H. K., and Kirkwood, A. (2011). AMPA receptor regulation during synaptic plasticity in hippocampus and neocortex. Semin Cell Dev. Biol. 22, 514–520. doi: 10.1016/j.semcdb.2011.06.007
Lee, H. K., and Kirkwood, A. (2019). Mechanisms of homeostatic synaptic plasticity in vivo. Front. Cell. Neurosci. 13:520. doi: 10.3389/fncel.2019.00520
Lee, H. K., and Whitt, J. L. (2015). Cross-modal synaptic plasticity in adult primary sensory cortices. Curr. Opin. Neurobiol. 35, 119–126. doi: 10.1016/j.conb.2015.08.002
Megill, A., Tran, T., Eldred, K., Lee, N. J., Wong, P. C., Hoe, H. S., et al. (2015). Defective age-dependent metaplasticity in a mouse model of Alzheimer’s disease. J. Neurosci. 35, 11346–11357. doi: 10.1523/JNEUROSCI.5289-14.2015
Mihalas, S., Ardiles, A., He, K., Palacios, A., and Kirkwood, A. (2021). A multisubcellular compartment model of AMPA receptor trafficking for neuromodulation of Hebbian synaptic plasticity. Front. Synaptic Neurosci. 13:703621. doi: 10.3389/fnsyn.2021.703621
Muller, J. F., Orekhov, Y., Liu, Y., and Ziemann, U. (2007). Homeostatic plasticity in human motor cortex demonstrated by two consecutive sessions of paired associative stimulation. Eur. J. Neurosci. 25, 3461–3468. doi: 10.1111/j.1460-9568.2007.05603.x
Narayanan, R., and Johnston, D. (2010). The h current is a candidate mechanism for regulating the sliding modification threshold in a BCM-like synaptic learning rule. J. Neurophysiol. 104, 1020–1033. doi: 10.1152/jn.01129.2009
O’Brien, R. J., Kamboj, S., Ehlers, M. D., Rosen, K. R., Fischbach, G. D., and Huganir, R. L. (1998). Activity-dependent modulation of synaptic AMPA receptor accumulation. Neuron 21, 1067–1078. doi: 10.1016/S0896-6273(00)80624-8
Oh, M. C., Derkach, V. A., Guire, E. S., and Soderling, T. R. (2006). Extrasynaptic membrane trafficking regulated by GluR1 serine 845 phosphorylation primes AMPA receptors for long-term potentiation. J. Biol. Chem. 281, 752–758. doi: 10.1074/jbc.M509677200
Pascual-Leone, A., and Torres, F. (1993). Plasticity of the sensorimotor cortex representation of the reading finger in Braille readers. Brain 116(Pt 1), 39–52. doi: 10.1093/brain/116.1.39
Petrus, E., Isaiah, A., Jones, A. P., Li, D., Wang, H., Lee, H. K., et al. (2014). Crossmodal induction of thalamocortical potentiation leads to enhanced information processing in the auditory cortex. Neuron 81, 664–673. doi: 10.1016/j.neuron.2013.11.023
Petrus, E., Rodriguez, G., Patterson, R., Connor, B., Kanold, P. O., and Lee, H. K. (2015). Vision loss shifts the balance of feedforward and intracortical circuits in opposite directions in mouse primary auditory and visual cortices. J. Neurosci. 35, 8790–8801. doi: 10.1523/JNEUROSCI.4975-14.2015
Philpot, B. D., Espinosa, J. S., and Bear, M. F. (2003). Evidence for altered NMDA receptor function as a basis for metaplasticity in visual cortex. J. Neurosci. 23, 5583–5588. doi: 10.1523/JNEUROSCI.23-13-05583.2003
Philpot, B. D., Sekhar, A. K., Shouval, H. Z., and Bear, M. F. (2001). Visual experience and deprivation bidirectionally modify the composition and function of NMDA receptors in visual cortex. Neuron 29, 157–169. doi: 10.1016/S0896-6273(01)00187-8
Qian, H., Matt, L., Zhang, M., Nguyen, M., Patriarchi, T., Koval, O. M., et al. (2012). beta2-Adrenergic receptor supports prolonged theta tetanus-induced LTP. J. Neurophysiol. 107, 2703–2712. doi: 10.1152/jn.00374.2011
Quinlan, E. M., Olstein, D. H., and Bear, M. F. (1999). Bidirectional, experience-dependent regulation of N-methyl-D-aspartate receptor subunit composition in the rat visual cortex during postnatal development. Proc. Natl. Acad. Sci. U.S.A. 96, 12876–12880. doi: 10.1073/pnas.96.22.12876
Ribic, A. (2020). Stability in the face of change: Lifelong experience-dependent plasticity in the sensory cortex. Front. Cell. Neurosci. 14:76. doi: 10.3389/fncel.2020.00076
Roder, B., Teder-Salejarvi, W., Sterr, A., Rosler, F., Hillyard, S. A., and Neville, H. J. (1999). Improved auditory spatial tuning in blind humans. Nature 400, 162–166. doi: 10.1038/22106
Rodriguez, G., Chakraborty, D., Schrode, K. M., Saha, R., Uribe, I., Lauer, A. M., et al. (2018). Cross-modal reinstatement of thalamocortical plasticity accelerates ocular dominance plasticity in adult mice. Cell Rep. 24, 3433–3440.e4. doi: 10.1016/j.celrep.2018.08.072
Rodriguez, G., Mesik, L., Gao, M., Parkins, S., Saha, R., and Lee, H. K. (2019). Disruption of NMDA receptor function prevents normal experience-dependent homeostatic synaptic plasticity in mouse primary visual cortex. J. Neurosci. 39, 7664–7673. doi: 10.1523/JNEUROSCI.2117-18.2019
Rumbaugh, G., and Vicini, S. (1999). Distinct synaptic and extrasynaptic NMDA receptors in developing cerebellar granule neurons. J. Neurosci. 19, 10603–10610. doi: 10.1523/JNEUROSCI.19-24-10603.1999
Sadato, N., Pascual-Leone, A., Grafman, J., Ibanez, V., Deiber, M. P., Dold, G., et al. (1996). Activation of the primary visual cortex by Braille reading in blind subjects. Nature 380, 526–528. doi: 10.1038/380526a0
Sandmann, P., Dillier, N., Eichele, T., Meyer, M., Kegel, A., Pascual-Marqui, R. D., et al. (2012). Visual activation of auditory cortex reflects maladaptive plasticity in cochlear implant users. Brain 135, 555–568. doi: 10.1093/brain/awr329
Sengpiel, F., and Kind, P. C. (2002). The role of activity in development of the visual system. Curr. Biol. 12, R818–R826. doi: 10.1016/S0960-9822(02)01318-0
Seol, G. H., Ziburkus, J., Huang, S., Song, L., Kim, I. T., Takamiya, K., et al. (2007). Neuromodulators control the polarity of spike-timing-dependent synaptic plasticity. Neuron 55, 919–929. doi: 10.1016/j.neuron.2007.08.013
Solger, J., Wozny, C., Manahan-Vaughan, D., and Behr, J. (2004). Distinct mechanisms of bidirectional activity-dependent synaptic plasticity in superficial and deep layers of rat entorhinal cortex. Eur. J. Neurosci. 19, 2003–2007. doi: 10.1111/j.1460-9568.2004.03292.x
Sterr, A., Muller, M. M., Elbert, T., Rockstroh, B., Pantev, C., and Taub, E. (1998a). Changed perceptions in Braille readers. Nature 391, 134–135. doi: 10.1038/34322
Sterr, A., Muller, M. M., Elbert, T., Rockstroh, B., Pantev, C., and Taub, E. (1998b). Perceptual correlates of changes in cortical representation of fingers in blind multifinger Braille readers. J. Neurosci. 18, 4417–4423. doi: 10.1523/JNEUROSCI.18-11-04417.1998
Sutton, M. A., Ito, H. T., Cressy, P., Kempf, C., Woo, J. C., and Schuman, E. M. (2006). Miniature neurotransmission stabilizes synaptic function via tonic suppression of local dendritic protein synthesis. Cell 125, 785–799. doi: 10.1016/j.cell.2006.03.040
Turrigiano, G. G. (2008). The self-tuning neuron: Synaptic scaling of excitatory synapses. Cell 135, 422–435. doi: 10.1016/j.cell.2008.10.008
Turrigiano, G. G., and Nelson, S. B. (2004). Homeostatic plasticity in the developing nervous system. Nat. Rev. Neurosci. 5, 97–107. doi: 10.1038/nrn1327
Turrigiano, G. G., Leslie, K. R., Desai, N. S., Rutherford, L. C., and Nelson, S. B. (1998). Activity-dependent scaling of quantal amplitude in neocortical neurons. Nature 391, 892–896. doi: 10.1038/36103
von Melchner, L., Pallas, S. L., and Sur, M. (2000). Visual behaviour mediated by retinal projections directed to the auditory pathway. Nature 404, 871–876. doi: 10.1038/35009102
Keywords: cross-modal plasticity, cortical plasticity, sensory experience, homeostatic synaptic plasticity, sliding threshold, LTP, LTD, adult plasticity
Citation: Lee H-K (2023) Metaplasticity framework for cross-modal synaptic plasticity in adults. Front. Synaptic Neurosci. 14:1087042. doi: 10.3389/fnsyn.2022.1087042
Received: 01 November 2022; Accepted: 19 December 2022;
Published: 06 January 2023.
Edited by:
Fereshteh S. Nugent, Uniformed Services University, United StatesReviewed by:
Arianna Maffei, Stony Brook University, United StatesCopyright © 2023 Lee. This is an open-access article distributed under the terms of the Creative Commons Attribution License (CC BY). The use, distribution or reproduction in other forums is permitted, provided the original author(s) and the copyright owner(s) are credited and that the original publication in this journal is cited, in accordance with accepted academic practice. No use, distribution or reproduction is permitted which does not comply with these terms.
*Correspondence: Hey-Kyoung Lee, aGV5a3lvdW5nbGVlQGpodS5lZHU=
Disclaimer: All claims expressed in this article are solely those of the authors and do not necessarily represent those of their affiliated organizations, or those of the publisher, the editors and the reviewers. Any product that may be evaluated in this article or claim that may be made by its manufacturer is not guaranteed or endorsed by the publisher.
Research integrity at Frontiers
Learn more about the work of our research integrity team to safeguard the quality of each article we publish.