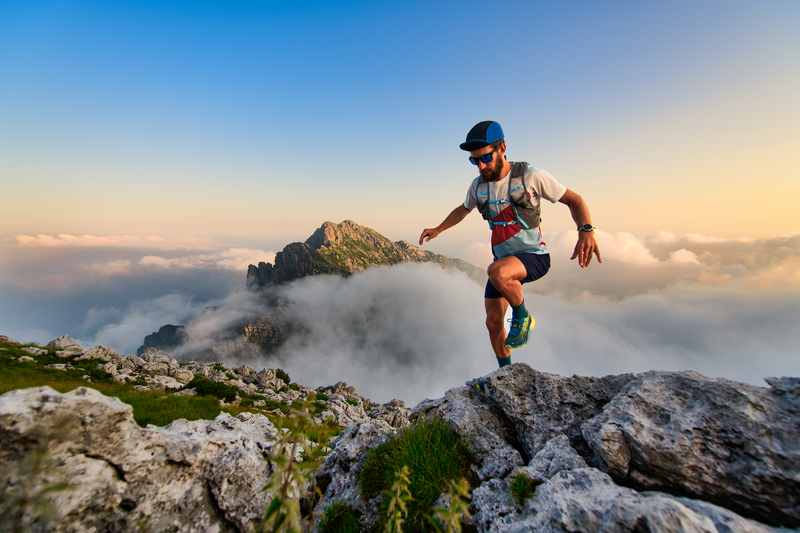
95% of researchers rate our articles as excellent or good
Learn more about the work of our research integrity team to safeguard the quality of each article we publish.
Find out more
ORIGINAL RESEARCH article
Front. Synaptic Neurosci. , 06 October 2022
Volume 14 - 2022 | https://doi.org/10.3389/fnsyn.2022.1021832
This article is part of the Research Topic Synaptic Plasticity and Dysfunction, Friend or Foe? View all 10 articles
Inhibitor-2 (I-2) is a prototypic inhibitor of protein phosphatase-1 (PP1), a major serine-threonine phosphatase that regulates synaptic plasticity and learning and memory. Although I-2 is a potent inhibitor of PP1 in vitro, our previous work has elucidated that, in vivo, I-2 may act as a positive regulator of PP1. Here we show that I-2 and PP1γ, but not PP1α, positively regulate synaptic transmission in hippocampal neurons. Moreover, we demonstrated that I-2 enhanced PP1γ interaction with its major synaptic scaffold, neurabin, by Förster resonance energy transfer (FRET)/Fluorescence lifetime imaging microscopy (FLIM) studies, while having a limited effect on PP1 auto-inhibitory phosphorylation. Furthermore, our study indicates that the effect of I-2 on PP1 activity in vivo is dictated by I-2 threonine-72 phosphorylation. Our work thus demonstrates a molecular mechanism by which I-2 positively regulates PP1 function in synaptic transmission.
Inhibitor-2 (I-2) is a prototypic inhibitor of protein phosphatase-1 (PP1), a major serine-threonine phosphatase which plays a critical role in synaptic functions (Foley et al., 2021b). The mechanism of inhibition of PP1 by I-2 has been extensively studied in vitro for decades since its purification in 1976 (Cohen, 1989; Lemaire and Bollen, 2020). Based on the crystal structure of the PP1–I-2 complex (Hurley et al., 2007) and other biochemical studies, PP1 binds to I-2 in a 1:1 stoichiometry, and an α-helix of I-2 (130–169) covers the active site of PP1, thereby inhibiting catalytic activity.
PP1 is inactive within the in vitro PP1–I-2 complex, but can be quickly (<1 min) activated when I-2 is phosphorylated at threonine 72 (pT72) by GSK3β (Cohen, 1989), MAPK or CDK5 (Li et al., 2006), presumably moving the I-2 α-helix away from the active site of PP1. However, I-2 pT72 acts as an intramolecular substrate for active PP1, and therefore must undergo dephosphorylation before PP1 is active toward other substrates. While PP1 is active, dephosphorylated I-2 T72 will lead to another slow (∼30 min) conformational change such that the α-helix of I-2 (residues 130–169) swings back into the active site, eventually leading to full inhibition of phosphatase activity (Cohen, 1989), completing the PP1 activation-inhibition cycle. Mutation of I-2 at T72 (T72A) blocks the activation step in the cycle and should be a constitutive PP1 inhibitor (Park et al., 1994; Huang et al., 1999).
While in vitro studies predominate, studies of I-2 function in intact cells and model organisms suggest more complex I-2 regulation and function (Wang et al., 2008a,b). Increasing evidence suggests that I-2 can function as a positive regulator of PP1 in addition to its role in PP1 inhibition (Tung et al., 1995; Lemaire and Bollen, 2020). For example, we found that I-2 knockdown, but not I-1 knockdown, in primary cortical neurons decreases PP1 activity based on increased PP1 inhibitory phosphorylation at T320 (Hou et al., 2013). Further, PP1 activity is required for long-term depression (LTD), but chemical LTD is defective in I-2 knockdown neurons (Hou et al., 2013). Similarly, PP1 constrains learning and memory and acts as a molecule of forgetfulness (Genoux et al., 2002), but global knockout (KO) of I-2 in mice, and knockdown of I-2 in rats, decreased memory formation as assayed by novel object recognition, contextual fear conditioning, and Morris water maze (Yang et al., 2015).
While we have found that I-2 plays an important role in LTD (Hou et al., 2013), synaptic downscaling (Siddoway et al., 2013a), and memory formation (Yang et al., 2015), whether I-2 plays a role in regulating synaptic transmission has not been examined. Additionally, the mechanism by which I-2 can promote PP1 function in the central nervous system is not clear, but, like other PP1 regulatory proteins, likely involves changing PP1 interaction with other proteins. Previous research has shown that I-2 can form a heterotrimeric complex with neurabin and PP1 (Terry-Lorenzo et al., 2002b; Dancheck et al., 2011), presenting an enticing model by which I-2 could regulate synaptic PP1.
Neurabin is a major PP1 regulatory protein that binds F-actin and targets PP1 to synaptic spines. Neurabin binds to PP1 via its RvXF460 motif as well as adjacent disordered regions (Ragusa et al., 2010). Mutating F460 in the RvXF460 motif leads to robust and significant decrease of neurabin-PP1 binding (Hu et al., 2006; Ragusa et al., 2010), as well as a decrease in synaptic transmission, suggesting that PP1 bound to neurabin promotes synaptic transmission (Hu et al., 2007). Neurabin binds PP1γ preferentially, PP1α to a lesser extent, and PP1β minimally (Terry-Lorenzo et al., 2002a; Carmody et al., 2008), suggesting PP1γ is most likely the PP1 isoform that promotes synaptic transmission. However, no direct test has validated this. Moreover, I-2, PP1, and neurabin all localize to excitatory synapses (Siddoway and Xia, 2011; Foley et al., 2021b), suggesting that they could act together in regulating synaptic transmission.
In this study, we employed over-expression of I-2 in combination with PP1α, PP1γ, and I-2 KO studies and found that I-2 and PP1γ both promote basal synaptic transmission. By introducing a phospho-null mutation at T72 in I-2, thereby disrupting the activation-inhibition cycle of PP1–I-2, we abolished the positive effect of I-2 on PP1 activity. Lastly, we found that I-2 promotes PP1γ targeting to neurabin, a critical synaptic PP1γ scaffolding protein for synaptic transmission. Our current work elucidates an important function of I-2 in promoting synaptic transmission and provides a mechanism of how I-2 positively regulates PP1 function.
Nestin-cre and CaMKIIα-cre (T29-1) were purchased from Jackson Lab. PP1α and PP1γ conditional KO mouse were generated as described previously (Liu et al., 2015). I2 floxed mice, in which exon 1 and exon 2 were flanked by Cre recombinase-dependent loxP recognition sequences, were generated by the University of Rochester Medical Center Transgenic and Genome Editing Core.
Primary cortical neurons were prepared from mixed male/female E18 Sprague Dawley (SD) rat embryos as previously described (Siddoway et al., 2013b,2014). ∼DIV21 neurons were used in our study. CFP, CFP-I-2(WT), and CFP-I-2(T72A) constructs and recombinant Sindbis virus generation and infection have been described previously (Hou et al., 2013). In brief, pSinRep5 (nsP2S) vector was used for CFP/I-2 subcloning, and targeted recombinant constructs were linearized, in vitro translated and electroplated into BHK21 cells, along with helper DHBB. Supernatant containing recombinant viruses were collected, concentrated via centrifugation before being applied to cultured neurons for 24 h. Medium from the 12-well neuronal plates were aspirated quickly, before 1X SDS gel loading buffer (contains protease and phosphatase inhibitor) was added to each well for about 10 min on ice before the cell lysates were harvested and heated for 10 min at 95°C followed by SDS-PAGE and immunoblotting. Anti-PP1 pT320 (1:1,000; Cell Signaling Technology), anti-PP1 (1:1,000; E-9, Santa Cruz Biotechnology, Inc.), and anti-GFP (1:1,000, 0.4 μg/ml; Roche).
Whole-cell patch-clamp recordings on cultured cortical neurons were recorded at ∼DIV21, as described (Siddoway et al., 2013a). Neurons were transfected with CFP, CFP-I-2WT or CFP-I-2T72A by calcium phosphate precipitation method 3 days prior. Pipettes were filled with (in mM): 117 Cs-methylsulfonate, 20 HEPES, 1 EGTA, 0.1 CaCl2, 5 CsCl, 2.5 MgATP, 0.25 Na3GTP, pH 7.4. External solution consisted of (in mM): 135 NaCl, 3.5 KCl, 2 CaCl2, 1.3 MgCl2, 10 HEPES, 20 glucose, supplemented with 200 nM TTX, 25 μM D-AP5, and 50 μM picrotoxin. mEPSCs were detected using template fitting in Clampfit 10.3 with a 5 pA threshold. Cumulative distributions were generated by histogram cumulative distribution of all mEPSC events for CFP (981 events) and I2T72A (932 events) groups. An equivalent number of events was randomly sampled from each I-2WT cell, yielding 946 events. mEPSC amplitude distributions were separately compared for the CFP vs. I2 (WT) groups, and the CFP vs. I2 (T72A) groups using non-parametric Kolmogorov-Smirnov test in GraphPad Prism 9.4.1.
Acute hippocampal slices were prepared from 1 to 2-month old mice bred as previously described (Foley et al., 2021a). Four hundred micrometer thick slices were prepared after decapitation and rapid extraction of the brains into ice-cold artificial cerebrospinal fluid (ACSF). Slices were then recovered in room temperature (RT) ACSF for at least 1 h prior to field recordings. Recordings were conducted at Schaffer collateral-CA1 synapses in RT ACSF at a flow rate of 2–3 mL/min. A borosilicate recording electrode (1–3 MΩ) filled with 1 M NaCl was placed in CA1 stratum radiatum and a monopolar borosilicate filled with ACSF (Figure 2) or tungsten concentric bipolar stimulating electrode (FHC) (Supplementary Figure 1) placed on Schaffer collaterals between CA3 and CA1. Responses were elicited every 15 s, with stimulation delivered by an ISO-Flex stimulus isolator (AMPI). The ACSF solution consisted of (in mM): 126.0 NaCl; 2.5 KCl; 2.5 CaCl2; 1.3 MgSO4; 1.25 NaH2PO4; 26.0 NaHCO3; and 10.0 D-glucose. ACSF was continuously aerated with carbogen (95% O2, 5% CO2) during incubation and recordings. Basal synaptic transmission was assessed by input-output (IO) curves. Short-term pre-synaptic plasticity was assayed using paired-pulse facilitation (PPF) of varying inter-pulse intervals. Recordings were collected with a MultiClamp 700A amplifier (Axon Instruments), PCI-6221 data acquisition device (National Instruments), and Igor Pro 7 (Wavemetrics) with a customized software package (Recording Artist)1. All experimental protocols for live animals were approved by the University Committee on Animal Resources of the University of Rochester Medical Center.
HEK293 cells were transfected in 35 mm culture dishes and imaging was performed 2 days later. Images were captured on an Olympus BX51WI upright microscope using a water-immersion 25x objective (Olympus XLPlan N). Two-photon 850 nm excitation was achieved with a Mai Tai Ti:Sapphire multi-photon laser (Spectra Physics), using a repetition rate of 80 MHz and a pulse width of approximately 100 fs, and emission was filtered with a 480-20 filter. Emission was measured by a H72422P Hamamatsu hybrid avalanche photodiode. Time-correlated single photon counting was performed using a Becker and Hickl module with a 25 ps resolution. VistaVision software (ISS) was used for lifetime analysis. Donor fluorescence from individual cells was binned and fit with a double exponential function, consistent with the lifetime properties of CFP.
GraphPad Prism (9.3.0) (in mEPSC it was 9.4.1) was used for statistical analyses and data visualization. Statistical significance between means was calculated using unpaired, two-tailed t-tests or ANOVAs. Repeated-measure (RM)-ANOVAs were used for IO and PPF comparisons. Tukey and Bonferroni post hoc comparisons were performed for one- and two-way ANOVAs, respectively. The arithmetic mean and standard error of the mean are displayed in all figures unless otherwise specified.
In order to study the potential role of I-2 in regulating basal synaptic transmission, we expressed CFP (control), CFP-tagged wild type (WT) I-2, or CFP-tagged I-2T72A in primary cortical neurons and performed whole-cell recordings 3 days after transfection (Figure 1A). We found that mEPSC amplitude, but not frequency, was robustly increased in CFP-I-2-expressing neurons compared to control neurons (Figures 1B–D). Interestingly, neither mEPSC amplitude nor mEPSC frequency showed significant change in CFP-I-2T72A-expressing neurons (Figures 1B–D). Our data thus suggest that I-2 promotes synaptic transmission, but this effect depends on the T72-mediated activation-inhibition cycle. While the mean mEPSC frequency was not significantly different overall, a subset of CFP-I-2WT-expressing neurons showed a robust increase in mEPSC frequency, though this potential effect was not further pursued in this study.
Figure 1. I-2 promotes synaptic transmission in primary cortical neurons. (A) Example traces of mEPSC recordings in primary cortical neurons (∼DIV21) expressing CFP, CFP-I2, or CFP-I2(T72A). (B,C) Quantification of mEPSC amplitude (B) and frequency (C). I-2 overexpression significantly increases mEPSC amplitude, but not frequency [one-way ANOVAs, F(2, 32) = 5.81, p < 0.01; F(2, 32) = 1.62, p = 0.21, respectively]. (D) Cumulative probability of mEPSC amplitude distribution [Kolmogorov-Smirnov test, p < 0.0001 between CFP and I-2(WT)-expressed neurons. P = 0.48 for between CFP and I2(T72A)]. Data in (B–D) are from the following number of cells, CFP: 12; I2: 12; I2(T72A): 11. (E) Western blot derived from ∼DIV21 primary cortical neurons 24 h after infection with CFP-, CFP- I2-, or CFP-I2(T72A)-expressing Sindbis virus. (F) Quantification of western blot results from five sets of neuronal cultures. pT320 was first normalized to total PP1, then normalized to CFP control culture [one-way ANOVA, F(2, 12) = 6.01, p < 0.05]. Tukey post hoc comparisons following one-way ANOVAs are displayed: *p < 0.05, *p < 0.01, ns, not significant.
Figure 2. I-2 and PP1γ promote synaptic transmission in acute hippocampal slices. (A) Successful knockout of PP1γ protein in nestin-cre;PP1γf/f mice. There is a slight upregulation of PP1β protein. (B–E) Results from field recordings in acute hippocampal slices at Sch-CA1 synapses. (B,C) There is a decrease in basal synaptic transmission in PP1γKO and I-2KO mice [two-way RM-ANOVAs, genotype: F(1, 16) = 14.39, p < 0.01; F(1, 8) = 6.987, p < 0.05, respectively]. (D,E) There is no change in paired pulse facilitation (PPF) in PP1γKO or I-2KO mice [two-way RM-ANOVAs, genotype: F(1, 12) = 0.280, p = 0.606; F(1, 8) = 0.068, p = 0.801, respectively]. Data are from the following number of mice/slices: (B), control 3/9, knockout 3/9; (C), control 3/6, knockout 2/4; (D), control 3/7, knockout 3/7; (E), control 3/6, knockout 2/4.
In order to determine the effect of overexpressed I-2WT and I-2T72A on PP1 activity, we examined the status of endogenous PP1’s inhibitory phosphorylation at threonine 320 (pT320) (Hou et al., 2013). Consistent with I-2T72A as a constitutive PP1 inhibitor, we found that overexpressed CFP-I-2T72A robustly (∼30 fold) and significantly increased pT320 (Figures 1E,F), an inverse marker of PP1 activity (Dohadwala et al., 1994). Surprisingly, there was no significant difference in pT320 between CFP-I-2WT and CFP (one-way ANOVA, Tukey post hoc, n = 5 per group, adjusted p = 0.9927) (Figures 1E,F), a drastic difference from the effect of CFP-I-2T72A on pT320. This result is contrary to the simple interpretation of I-2 as a PP1 inhibitor from in vitro studies, but consistent with our previous report of robust PP1 activity in I-2 immunoprecipitate (Yang et al., 2015). This is also consistent with the model of PP1 inhibition-activation by I-2: the rate-limiting step in the PP1 activation-inhibition cycle is the inhibition step (Cohen, 1989) and the robust I-2 T72 kinase activity seen in neurons (Hou et al., 2013) should favor the activation state. On the other hand, I-2T72A blocks the activation step and potently inhibits PP1 (Figures 1E,F).
Since I-2WT (Figure 1) and the neurabin-PP1 holoenzyme (Hu et al., 2007) both promote basal synaptic transmission in neuronal cultures, we next sought to directly compare the function of I-2 and PP1 on synaptic transmission in acute hippocampal slices via genetic ablation. As neurabin has minimal binding to PP1β, and PP1β is not as enriched in dendritic spines (Bordelon et al., 2005), we focused our study on PP1α and PP1γ. While mice with PP1α or PP1γ KO in neural progenitor cells (NPCs; Nestin-Cre, JAX) were viable, I-2 NPC KO was embryonic lethal. We therefore generated I-2 conditional KO mice with a hippocampus-specific Cre (CaMKII-creT–29, JAX). We then measured basal synaptic transmission via input-output (I/O) curves at hippocampal CA3-CA1 Schaffer-collateral synapses in each transgenic mouse line. We found that synaptic transmission was significantly decreased in both I-2 and PP1γ KO mice compared to control littermates (Figures 2B,C and Supplementary Figure 1). On the other hand, the I/O curve from PP1α KO mice was not significantly different compared to control littermates (Supplementary Figure 1), consistent with its lower binding affinity to neurabin. We did not observe a difference in paired-pulse facilitation (PPF) in any mouse model (Figures 2D,E and Supplementary Figure 1), indicating no change in glutamate release by CA3 neurons. These results thus suggest that I-2 and PP1γ, but not PP1α, promote AMPAR-mediated basal synaptic transmission in CA1 pyramidal neurons.
Our data so far suggest that PP1γ and I-2WT, but not I-2T72A, promote excitatory synaptic transmission. Since we did not observe a change in PP1 activity by overexpressed I-2WT (Figure 1E), we next sought to determine the effect of I-2 on PP1 interaction with its regulatory proteins. We first established direct I-2–PP1 interaction via fluorescence lifetime imaging microscopy (FLIM). The fluorescence lifetime of CFP (ƬCFP) on CFP-I-2 was robustly decreased when YFP-PP1γ was co-expressed in HEK 293 cells (Figures 3A1–A3). Moreover, we found that the decrease of ƬCFP–I–2 was attenuated if YFP-PP1γH125Q was co-expressed, a PP1 mutant made to disrupt the binding interface between I-2 and PP1 (Hurley et al., 2007; Figure 3A). This suggests that the FRET observed between CFP-I-2 and YFP-PP1γ is derived from I-2–PP1 interaction. We next examined the interaction between neurabin and I-2. We observed a robust decrease of ƬCFP of CFP-neurabin1–490 when YFP-I-2 was co-expressed (Figures 3B1–B3). This decrease was not observed with a PP1-binding-deficient neurabin, CFP-neurabin1–490, F460A (Figures 3B1–B3). Because the RvXF motif on neurabin does not participate in interaction with I-2 (Dancheck et al., 2011), this result suggests that the FRET between CFP- neurabin1–490 and YFP-I-2 involves a trimeric complex with endogenous PP1. We did not observe FRET between CFP-I-2 and neurabin1–490-YFP (Figures 3C1–C3), demonstrating that FRET between fluorescently tagged I-2 and neurabin is sensitive to N- vs. C-terminal positioning of the fluorophores. However, we observed significant FRET between CFP-I-2T72A and neurabin1–490-YFP (Figures 3C1–C3), supporting the idea that T72A mutation leads to a conformational change in I-2, large enough to position the fluorophores close enough and/or in optimal orientation for FRET to occur.
Figure 3. Regulation of I-2 interactions with PP1γ and neurabin in vivo as assessed by FLIM. (A1–C1) Intensity (left) and lifetime (right) of example cells. Lifetime expressed in nanoseconds (ns), with a color scale shown on the right. (A2–C2) Time-correlated single photon counting (TCSPC) histograms from example fields of view. (A3–C3) Mean lifetimes and SEM from the indicated constructs. (A3) YFP-PP1γ, but not YFP-PP1γH125Q, co-expression decreases CFP-I2 lifetime [one-way ANOVA, F(4, 289) = 417.9, p < 0.0001]. (B3) YFP-I2 co-expression decreases CFP-Nrb lifetime. The interaction between CFP-Nrb1–490 and YFP-I2 is attenuated by PP1-binding-deficient Nrb, CFP-Nrb1–490, F60A [one-way ANOVA, F(3, 216) = 35.60, p < 0.0001]. (C3) Nrb1–490-YFP does not affect CFP-I2 lifetime, but decreases CFP-I2T72A lifetime [one-way ANOVA, F(2, 352) = 27.86, p < 0.0001]. Tukey post hoc comparisons following one-way ANOVAs are displayed: *p < 0.05, **p < 0.01, ***p < 0.001, ****p < 0.0001, ns, not significant. Number of cultures and number of cells are shown within bars. All data represent independent experiments; lifetime data was not reused between subfigures.
We next determined whether I-2 could promote neurabin-PP1γ holoenzyme formation. We observed a robust decrease of ƬCFP on CFP-neurabin1–490 when it was co-expressed with YFP-PP1γ (Figure 4A). Moreover, the decrease of ƬCFP was significantly reduced when YFP-PP1γ was co-expressed with PP1-binding deficient CFP-neurabin1–490, F460A (Figure 4A). This suggests that the FRET observed between CFP-neurabin1–490 and YFP-PP1γ is derived from their interaction. Notably, we found that co-expressing rLuc-I-2WT with CFP-neurabin (1-490) and YFP-PP1γ significantly further decreased the ƬCFP on CFP-neurabin1–490, relative to CFP-neurabin1–490 and YFP-PP1γ double transfection (Figure 4B). This suggests that I-2 promotes neurabin-PP1γ holoenzyme formation, thus regulating PP1γ function in a positive manner. This finding is consistent with previous in vitro binding data showing I-2 promotes neurabin-PP1 binding (Terry-Lorenzo et al., 2002b). A similar study of I-2 function in tobacco leaves showed that I-2 promotes PP1 binding to its scaffolding protein SNF1-related protein kinase 2 (SnRK2) (Hou et al., 2016). Overall, our study suggests that overexpressed I-2WT increases synaptic transmission, not via promoting the enzymatic activity of individual PP1 molecules (Figures 1D,E), rather via increasing the number of PP1 molecules targeted by neurabin.
Figure 4. I-2 promotes neurabin-PP1 interaction. (A1,B1) Intensity (left) and lifetime (right) of example cells. Lifetime expressed in ns. (A2,B2) Time-correlated single photon counting (TCSPC) histograms from example fields of view. (A3,B3) Mean lifetimes and SEM from the indicated constructs. (A3) YFP-PP1γ co-expression decreases CFP-Nrb1–490 lifetime. CFP-Nrb1–490 F460A significantly attenuates the YFP-PP1γ-mediated decrease in lifetime [one-way ANOVA, F(7, 694) = 190.4, p < 0.0001]. (B3) rLuc-I2 co-expression promotes CFP-Nrb1–490 interaction with YFP-PP1γ, further decreasing lifetime [two-tailed t-test, t(177) = 3.443, p < 0.001]. t-test results (B3) or Tukey post hoc comparisons following one-way ANOVAs (A3) are displayed: *p < 0.05, **p < 0.01, ***p < 0.001, ****p < 0.0001, ns, not significant. Number of cultures and number of cells are shown within bars. All data represent independent experiments; lifetime data was not reused between subfigures.
In summary, we found that I-2, PP1γ, but not PP1α, promotes basal synaptic transmission, and that I-2 positively regulates PP1γ function via enhancing neurabin-PP1γ holoenzyme formation, without affecting PP1γ enzymatic activity. Our study provides a fundamental mechanism by which I-2 can positively regulate PP1 function. This mechanism may regulate PP1 in vivo function beyond synaptic transmission.
The datasets generated and/or analyzed during the current study are available from the corresponding author on reasonable request.
The animal study was reviewed and approved by UCAR committee of the University of Rochester Medical Center.
HX: conceptualization, supervision, writing – original draft, and project administration. KF: investigation, formal analysis, and writing – original draft. HA: investigation, formal analysis, and writing – review and editing. HH and HY: investigation. YZ and DM: investigation and formal analysis. CM: formal analysis and writing – review and editing. MP-S: investigation and writing – review and editing. AM: formal analysis. AN: resources and writing – review and editing. DS: supervision and writing–review and editing. All authors contributed to the article and approved the submitted version.
This work was supported by the National Institutes of Health (NIH) R01 MH109719 and National Science Foundation (NSF) IOS-1457336 to HX; NIH DA10044 to AN, NIH F30 MH122046 to KF, NIH R35GM137951 to DM, the Heart and Stroke Foundation, Canada, to HA, and the Natural Sciences and Engineering Research Council of Canada to DS. We have institution start-up for covering the publication fee; however, HX was an associate editor for the Frontier in Molecular Neuroscience and thus enjoy one free publication per year.
The authors declare that the research was conducted in the absence of any commercial or financial relationships that could be construed as a potential conflict of interest.
All claims expressed in this article are solely those of the authors and do not necessarily represent those of their affiliated organizations, or those of the publisher, the editors and the reviewers. Any product that may be evaluated in this article, or claim that may be made by its manufacturer, is not guaranteed or endorsed by the publisher.
The Supplementary Material for this article can be found online at: https://www.frontiersin.org/articles/10.3389/fnsyn.2022.1021832/full#supplementary-material
Supplementary Figure 1 | PP1α does not play a role in synaptic transmission. (A) Successful knockout of PP1α protein in nestin-cre;PP1αf/f mice. (B–E) Results from field recordings in acute hippocampal slices at Sch-CA1 synapses. (B,C) There is no difference in basal synaptic transmission in PP1αKO mice, but a significant decrease in PP1γKO mice [two-way RM-ANOVAs, genotype: F(1, 10) = 0.02, p = 0.899; F(1, 14) = 4.79, p < 0.05, respectively). (D,E) There is no change in paired pulse facilitation (PPF) at the Sch-CA1 pyramidal synapses in PP1αKO or PP1γKO mice [two-way RM-ANOVAs, genotype: F(1, 10) = 0.172, p = 0.687; F(1, 14) = 0.006, p = 0.938, respectively). Data are from the following number of mice/slices: (B), control 2/7, knockout 2/5; (C), control 3/7, knockout 3/9; (D), control 2/6, knockout 2/6; (E), control 3/6, knockout 3/10.
Bordelon, J. R., Smith, Y., Nairn, A. C., Colbran, R. J., Greengard, P., and Muly, E. C. (2005). Differential localization of protein phosphatase-1alpha, beta and gamma1 isoforms in primate prefrontal cortex. Cereb. Cortex 15, 1928–1937. doi: 10.1093/cercor/bhi070
Carmody, L. C., Baucum, A. J. II., Bass, M. A., and Colbran, R. J. (2008). Selective targeting of the gamma1 isoform of protein phosphatase 1 to F-actin in intact cells requires multiple domains in spinophilin and neurabin. Faseb J. 22, 1660–1671. doi: 10.1096/fj.07-092841
Cohen, P. (1989). The structure and regulation of protein phosphatases. Annu. Rev. Biochem. 58, 453–508.
Dancheck, B., Ragusa, M. J., Allaire, M., Nairn, A. C., Page, R., and Peti, W. (2011). Molecular investigations of the structure and function of the protein phosphatase 1-spinophilin-inhibitor 2 heterotrimeric complex. Biochemistry 50, 1238–1246. doi: 10.1021/bi101774g
Dohadwala, M., da Cruz e Silva, E. F., Hall, F. L., Williams, R. T., Carbonaro-Hall, D. A., Nairn, A. C., et al. (1994). Phosphorylation and inactivation of protein phosphatase 1 by cyclin-dependent kinases. Proc. Natl. Acad. Sci. U. S. A. 91, 6408–6412.
Foley, K. F. W., Barnett, D., Cory-Slechta, D. A., and Xia, H. (2021a). Early low-level arsenic exposure impacts post-synaptic hippocampal function in juvenile mice. Toxics 9:206. doi: 10.3390/toxics9090206
Foley, K., McKee, C., Nairn, A. C., and Xia, H. (2021b). Regulation of synaptic transmission and plasticity by protein phosphatase 1. J. Neurosci. 41, 3040–3050.
Genoux, D., Haditsch, U., Knobloch, M., Michalon, A., Storm, D., and Mansuy, I. M. (2002). Protein phosphatase 1 is a molecular constraint on learning and memory. Nature 418, 970–975. doi: 10.1038/nature00928
Hou, H., Sun, L., Siddoway, B. A., Petralia, R. S., Yang, H., Gu, H., et al. (2013). Synaptic NMDA receptor stimulation activates PP1 by inhibiting its phosphorylation by Cdk5. J. Cell Biol. 203, 521–535. doi: 10.1083/jcb.201303035
Hou, Y. J., Zhu, Y., Wang, P., Zhao, Y., Xie, S., Batelli, G., et al. (2016). Type one protein phosphatase 1 and its regulatory protein inhibitor 2 negatively regulate ABA signaling. PLoS Genet. 12:e1005835. doi: 10.1371/journal.pgen.1005835
Hu, X. D., Huang, Q., Roadcap, D. W., Shenolikar, S. S., and Xia, H. (2006). Actin-associated neurabin-protein phosphatase-1 complex regulates hippocampal plasticity. J. Neurochem. 98, 1841–1851. doi: 10.1111/j.1471-4159.2006.04070.x
Hu, X. D., Huang, Q., Yang, X., and Xia, H. (2007). Differential regulation of AMPA receptor trafficking by neurabin-targeted synaptic protein phosphatase-1 in synaptic transmission and long-term depression in hippocampus. J. Neurosci. 27, 4674–4686. doi: 10.1523/JNEUROSCI.5365-06.2007
Huang, H. B., Horiuchi, A., Watanabe, T., Shih, S. R., Tsay, H. J., Li, H. C., et al. (1999). Characterization of the inhibition of protein phosphatase-1 by DARPP-32 and inhibitor-2. J. Biol. Chem. 274, 7870–7878.
Hurley, T. D., Yang, J., Zhang, L., Goodwin, K. D., Zou, Q., Cortese, M., et al. (2007). Structural basis for regulation of protein phosphatase 1 by inhibitor-2. J. Biol. Chem. 282, 28874–28883. doi: 10.1074/jbc.M703472200
Lemaire, S., and Bollen, M. (2020). Protein phosphatase-1: Dual activity regulation by inhibitor-2. Biochem. Soc. Trans. 48, 2229–2240.
Li, M., Stefansson, B., Wang, W., Schaefer, E. M., and Brautigan, D. L. (2006). Phosphorylation of the Pro-X-Thr-Pro site in phosphatase inhibitor-2 by cyclin-dependent protein kinase during M-phase of the cell cycle. Cell Signal 18, 1318–1326. doi: 10.1016/j.cellsig.2005.10.020
Liu, R., Correll, R. N., Davis, J., Vagnozzi, R. J., York, A. J., Sargent, M. A., et al. (2015). Cardiac-specific deletion of protein phosphatase 1beta promotes increased myofilament protein phosphorylation and contractile alterations. J. Mol. Cell Cardiol. 87, 204–213. doi: 10.1016/j.yjmcc.2015.08.018
Park, I. K., Roach, P., Bondor, J., Fox, S. P., and DePaoli-Roach, A. A. (1994). Molecular mechanism of the synergistic phosphorylation of phosphatase inhibitor-2. Cloning, expression, and site-directed mutagenesis of inhibitor-2. J. Biol. Chem. 269, 944–954.
Ragusa, M. J., Dancheck, B., Critton, D. A., Nairn, A. C., Page, R., and Peti, W. (2010). Spinophilin directs protein phosphatase 1 specificity by blocking substrate binding sites. Nat. Struct. Mol. Biol. 17, 459–464. doi: 10.1038/nsmb.1786
Siddoway, B. A., Altimimi, H. F., Hou, H., Petralia, R. S., Xu, B., Stellwagen, D., et al. (2013a). An essential role for inhibitor-2 regulation of protein phosphatase-1 in synaptic scaling. J. Neurosci. 33, 11206–11211. doi: 10.1523/JNEUROSCI.5241-12.2013
Siddoway, B. H. H., and Xia, H. (2011). Glutamatergic synapses: Molecular organisation. eLS doi: 10.1002/9780470015902.a0000235.pub2 [Epub ahead of print].
Siddoway, B., Hou, H., Yang, H., Petralia, R., and Xia, H. (2014). Synaptic activity bidirectionally regulates a novel sequence-specific S-Q phosphoproteome in neurons. J. Neurochem. 128, 841–851. doi: 10.1111/jnc.12487
Siddoway, B., Hou, H., Yang, J., Sun, L., Yang, H., Wang, G. Y., et al. (2013b). Potassium channel Kv2.1 is regulated through protein phosphatase-1 in response to increases in synaptic activity. Neurosci. Lett. 583, 142–147. doi: 10.1016/j.neulet.2014.08.051
Terry-Lorenzo, R. T., Carmody, L. C., Voltz, J. W., Connor, J. H., Li, S., Smith, F. D., et al. (2002a). The neuronal actin-binding proteins, neurabin I and neurabin II, recruit specific isoforms of protein phosphatase-1 catalytic subunits. J. Biol. Chem. 277, 27716–27724. doi: 10.1074/jbc.M203365200
Terry-Lorenzo, R. T., Elliot, E., Weiser, D. C., Prickett, T. D., Brautigan, D. L., and Shenolikar, S. (2002b). Neurabins recruit protein phosphatase-1 and inhibitor-2 to the actin cytoskeleton. J. Biol. Chem. 277, 46535–46543. doi: 10.1074/jbc.M206960200
Tung, H. Y., Wang, W., and Chan, C. S. (1995). Regulation of chromosome segregation by Glc8p, a structural homolog of mammalian inhibitor 2 that functions as both an activator and an inhibitor of yeast protein phosphatase 1. Mol. Cell Biol. 15, 6064–6074. doi: 10.1128/MCB.15.11.6064
Wang, W., Cronmiller, C., and Brautigan, D. L. (2008a). Maternal phosphatase inhibitor-2 is required for proper chromosome segregation and mitotic synchrony during Drosophila embryogenesis. Genetics 179, 1823–1833. doi: 10.1534/genetics.108.091959
Wang, W., Stukenberg, P. T., and Brautigan, D. L. (2008b). Phosphatase inhibitor-2 balances protein phosphatase 1 and aurora B kinase for chromosome segregation and cytokinesis in human retinal epithelial cells. Mol. Biol. Cell 19, 4852–4862. doi: 10.1091/mbc.e08-05-0460
Keywords: protein phosphatase-1, scaffolding protein, regulatory subunit, synaptic transmission, FRET-Förster resonance energy transfer, hippocampus, inhibitor-2
Citation: Foley K, Altimimi H, Hou H, Zhang Y, McKee C, Papasergi-Scott MM, Yang H, Mayer A, Ward N, MacLean DM, Nairn AC, Stellwagen D and Xia H (2022) Protein phosphatase-1 inhibitor-2 promotes PP1γ positive regulation of synaptic transmission. Front. Synaptic Neurosci. 14:1021832. doi: 10.3389/fnsyn.2022.1021832
Received: 17 August 2022; Accepted: 09 September 2022;
Published: 06 October 2022.
Edited by:
Lu Chen, Stanford University, United StatesReviewed by:
Yan Dong, University of Pittsburgh, United StatesCopyright © 2022 Foley, Altimimi, Hou, Zhang, McKee, Papasergi-Scott, Yang, Mayer, Ward, MacLean, Nairn, Stellwagen and Xia. This is an open-access article distributed under the terms of the Creative Commons Attribution License (CC BY). The use, distribution or reproduction in other forums is permitted, provided the original author(s) and the copyright owner(s) are credited and that the original publication in this journal is cited, in accordance with accepted academic practice. No use, distribution or reproduction is permitted which does not comply with these terms.
*Correspondence: Houhui Xia, SG91aHVpX3hpYUB1cm1jLnJvY2hlc2VyLmVkdQ==
Disclaimer: All claims expressed in this article are solely those of the authors and do not necessarily represent those of their affiliated organizations, or those of the publisher, the editors and the reviewers. Any product that may be evaluated in this article or claim that may be made by its manufacturer is not guaranteed or endorsed by the publisher.
Research integrity at Frontiers
Learn more about the work of our research integrity team to safeguard the quality of each article we publish.