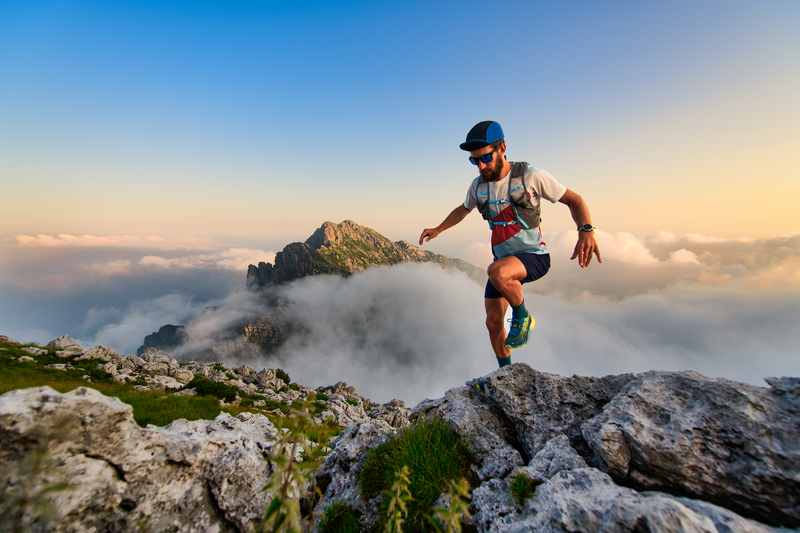
95% of researchers rate our articles as excellent or good
Learn more about the work of our research integrity team to safeguard the quality of each article we publish.
Find out more
BRIEF RESEARCH REPORT article
Front. Synaptic Neurosci. , 07 February 2020
Volume 12 - 2020 | https://doi.org/10.3389/fnsyn.2020.00004
This article is part of the Research Topic The Key Role of Calcium in Synaptic Neuroscience View all 4 articles
Calcium-calmodulin-dependent protein kinase (CaMKII) is a molecule involved in several cell processes including plasticity related to learning and memory. Activation of NMDA-type glutamate receptors results in translocation of CaMKII to synapses. However, there are at least two distinct mechanisms by which glutamate-dependent CaMKII translocation occurs: one well-studied process resulting from whole-cell glutamate stimulation and one resulting from brief, local glutamate application. Unlike the relatively fast CaMKII translocation seen following whole-cell glutamate delivery (seconds), local application results in CaMKII translocation that occurs gradually within 6–10 min. This locally-induced translocation of CaMKII requires L-type Ca2+ channel co-activation but does not rely on GluN2B receptor subunit expression, unlike translocation following whole-cell application of glutamate. The current study examined if nucleotide binding is necessary for locally-induced CaMKII translocation, similar to CaMKII translocation resulting from whole-cell glutamate application. Three different mechanisms of inhibition were employed: staurosporine (ATP inhibitor), CaMKII(281–302) peptide inhibitor and expression of the K42M mutation. Locally-induced CaMKII translocation was moderately suppressed in the presence of either the broad-spectrum kinase inhibitor staurosporine (100 nm) or the CaMKII(281–302) peptide inhibitor. However, expression of the catalytically dead K42M mutation that prevents ATP-binding to CaMKII, significantly inhibited locally-induced translocation. Thus, CaMKII translocation following brief, local glutamate application requires nucleotide binding, providing support for future research into the molecular mechanisms of this distinct form of CaMKII translocation.
Calcium-calmodulin-dependent protein kinase (CaMKII) is involved in several cellular processes and is abundantly expressed in neurons (Erondu and Kennedy, 1985). It is well-established that whole-neuron activation with glutamate or NMDA results in rapid translocation of CaMKII to synapses (within seconds) resulting from calcium influx through activated NMDA receptor channels (Shen and Meyer, 1999; Shen et al., 2000; Thalhammer et al., 2006). Whole-cell glutamate-induced CaMKII translocation requires nucleotide binding, Ca2+/CaM activation of the kinase and binding of CaMKII to NMDA receptor GluN2B subunits, a process that increases the ATP-binding affinity of CaMKII (Shen and Meyer, 1999; Shen et al., 2000; Bayer et al., 2001, 2006; Cheriyan et al., 2011; O’Leary et al., 2011; Halt et al., 2012). However, CaMKII translocation resulting from whole-cell activation does not require voltage-gated Ca2+ channel activation and further does not rely on CaMKII autophosphorylation at the T286 site (Shen and Meyer, 1999; Bayer et al., 2006; Thalhammer et al., 2006; Rose et al., 2009; O’Leary et al., 2011).
Weaker glutamate excitation protocols (e.g., single puff of glutamate via local pipette) can also activate CaMKII translocation that occurs on a much longer time scale (on the order of minutes; Zhang et al., 2008; Rose et al., 2009; She et al., 2012). Similar to whole-cell activation protocols, CaMKII translocation resulting from a single local puff of glutamate similarly requires Ca2+/CaM activation and calcium influx via NMDA receptor channels (Rose et al., 2009). However, unlike CaMKII translocation following whole-cell stimulation, locally-induced CaMKII translocation requires L-type calcium channel activation but not CaMKII-GluN2B binding (Rose et al., 2009; She et al., 2012) indicating that the molecular mechanisms of locally-induced synaptic CaMKII translocation differ somewhat from those involved in CaMKII translocation resulting from whole-cell activation.
Given the consistent finding that nucleotide binding is required for whole-cell glutamate-induced CaMKII translocation, it remains of interest to determine if locally-induced CaMKII translocation also requires nucleotide binding. To test this, a combination of approaches was performed. Local stimulation was delivered in the presence of either staurosporine (a competitive ATP inhibitor) or a peptide inhibitor (CaMKII281–302). As well, locally-induced CaMKII translocation trials were performed following expression of the K42M mutation with knockdown of endogenous CaMKII. Significant inhibition of CaMKII translocation resulted with K42M expression while only moderate inhibition was noted in the other conditions. The results from these trials contribute to uncovering the basic mechanisms of locally-induced synaptic CaMKII translocation.
All experiments involving the use of rats and the procedures were approved by the Western Washington University Institutional Animal Care and Use Committee and were in strict accordance with the Guide for the Care and Use of Laboratory Animals described by the National Institutes of Health. E18 rat hippocampal neurons were isolated and cultured as described by Harms and Craig (2005) and Kaech and Banker (2006). Briefly, dissociated hippocampal neurons were plated onto poly-D-lysine-coated (MW >100,000; Sigma) glass coverslips at a density of 1.5 × 105 cells per 60 mm dish in Neurobasal medium containing B27 (Gibco), 0.6 mM glutamine and 100 U ml−1/100 μg ml−1 penicillin/streptomycin. Neurons were maintained at 37°C and 5% CO2 and cultured with a glial feeder layer. One-third of the medium was exchanged weekly and 5 μM AP5 was added twice weekly. All experiments were performed on neurons 14–16 days in vitro. Neuron transfection occurred either ~72 h prior to experiments via Lipofectamine 3000 (Invitrogen) or by electronucleoporation just prior to plating using an Amaxa Nucleofector II (program O-005). Expression plasmids employed were either GFP-CaMKIIα (gift from A.M. Craig) or GFP-CaMKIIα with K42M mutation combined with shRNA for endogenous CaMKII knockdown (gift from K.U. Bayer; see Barcomb et al., 2014 for details).
Glass micropipettes were shaped with a 3–5 μm opening similar to that used in Rose et al. (2009) using a micropipette puller (Model P-97, Sutter) just prior to imaging and loaded with glutamate plus glycine in ECS solution (10 μM glycine, 100 μM glutamate; ECS: 168 mM NaCl, 2.4 mM KCl, 10 mM HEPES, 10 mM D-glucose, 1.3 CaCl2, 1.3 MgCl2, pH 7.3). Drug conditions included 100 nM staurosporine (Sigma, CA, USA), a concentration previously reported to block CaMKII activity (Yanagihara et al., 1991) and peptide inhibitors CaMKII(290–309; sc3037, 1 μM, sequence: LKKFNARRKLKGAILTTMLA) and CaMKII(281–302; sc3039, 1 μM, sequence: MHRQEAVDCLKKFNARRKLKGA; Santa Cruz Biotechnology, Dallas, TX, USA) to inhibit calmodulin- or ATP-binding, respectively (Malinow et al., 1989). Staurosporine or peptide inhibitors were bath applied to neuron cultures at least 20 min prior to local glutamate stimulation and were included in the stimulation pipette. A locally-induced stimulation trial with an ECS vehicle control bath always preceded inhibitor/mutation trials.
Live-imaging of neurons was conducted on an Olympus IX-81 inverted microscope with epifluorescence. All images were collected with a Hamamatsu CCD camera using Metamorph software (version 7.6.5.0; Molecular Devices Inc., San Jose, CA, USA). Individual coverslips were mounted in an open chamber with a continuous circulating ECS bath. Once a single neuron was in focus, a DIC and a GFP fluorescence image were captured (Figure 1A). A micropipette connected to an air injector (microINJECTOR with MINJ-2 pulse-length control module; Tritech) was then lowered within ~5–10 μm of a dendrite (Figure 1B). Glutamate (plus glycine) solution was delivered via micropipette (100 ms at 100 psi). Within 1–2 s following stimulation, the micropipette was raised away from the neuron and time-series collection began with GFP images captured (500 ms exposure duration) once every 30 s (Figure 1C). Following the conclusion of the time series, an additional post-translocation DIC image was captured. Morphology filters were employed in Metamorph to produce a consistent measure of relative fluorescence over time. A correction function was employed in FIJI software (overall fluorescence level of each frame was compared to the first frame in a time series to determine a normalizing ratio; Schindelin et al., 2012) to account for any potential bleaching.
Figure 1. GFP-calcium-calmodulin-dependent protein kinase (CaMKII) expression and translocation after stimulation with 100 μM glutamate with 10 μM glycine via pipette. (A) GFP-CaMKII expression prior to stimulation. (B) DIC image of the same neuron with measured dendrites highlighted in red (right) and blue (left) and an arrow indicating the position of the micropipette. Scale bar = 50 μm. (C) Time-lapse subtraction images (current fluorescence level minus initial fluorescence level; increases in fluorescence appear whiter while decreases are darker/black). Hollow white arrows indicate dendrite locations that show increased relative fluorescence over time, solid white arrow indicates the location where relative fluorescence level decreases. (D) Linescan measures of the same neuron shown in (C) indicating fluorescence levels for respective dendrites, red (top) and blue (bottom), starting proximal to the cell body. Brackets denote regions that correspond to boxes shown in (A,B). Black linescan trace indicates fluorescence levels measured at first post-stimulation image capture for comparison. Arrowhead indicates the location of the stimulation pipette.
Images were analyzed using Metamorph software (version 7.6.5.0; Molecular Devices Inc., San Jose, CA, USA). Using the linescan function, a multipoint line with a width of 18 pixels was drawn along dendrites and fluorescence intensity was captured (Figure 1D). Linescan data processing was performed using a custom R script (see Supplementary Data Sheet 1) whereby fluorescence-expressing puncta (i.e., fluorescence peaks) were determined mathematically to avoid selection biases. Briefly, two moving averages for fluorescence intensity were computed along each dendrite; one a moving average of five pixels (approximate minimum size of fluorescence puncta; the first value was the average fluorescence intensity of the first five pixels, the next value being the average of the second to sixth-pixel intensity values, and so on) and the other a moving average of 31 pixels (fluorescence for short dendrite region). Each punctal fluorescence average (five pixels) was then divided by the corresponding 31-pixel average for that specific dendrite region with quotients greater than 1.1 being flagged as peaks (a point of fluorescence brightness as an indication of puncta). For confirmation that the mathematical determination reliably identified GFP-expressing puncta along dendrites, locations of flagged puncta were mapped and compared to the original fluorescence data.
Once puncta were identified, the fluorescence of puncta was calculated by dividing punctal fluorescence intensity by the total fluorescence for each frame (accounting for background and bleaching). An increase in punctal fluorescence intensity served as an indicator of CaMKII translocation and was calculated as the average relative peak fluorescence level for each dendrite normalized to initial peak fluorescence intensity. In the vehicle group, it was noted that GFP-CaMKII translocation was seen in all dendrites, both adjacent and non-adjacent to the stimulation site, with no significant difference in time of translocation initiation (F(48,2) = 0.34, p > 0.10; Figures 1C,D). Thus, data were pooled across dendrites for each experimental condition.
The density of GFP-CaMKII puncta was calculated by dividing the number of identified puncta by the length of the dendrite measured using the MetaMorph linescan function yielding a value in puncta/μm. To compare relative GFP-CaMKII fluorescence intensity as well as GFP-CaMKII punctal density, a repeated measures mixed-design ANOVA was employed (with Greenhouse-Geisser correction) followed by tests of simple main effects with Šidák adjustment for multiple tests. According to Levene’s test, groups had equal variances over time. All statistics were performed using SPSS (version 25) and graphs were designed in Excel 2016.
For GFP-CaMKII expressing neurons co-transfected with T286A, live-cell imaging methods were as described above except imaging was performed on a Nikon TE300 microscope with a 40× 1.0 NA oil objective and Retiga EXi or Diagnostics Instruments SPOT cooled CCD camera using Metamorph software, as reported in Rose et al. (2009). As well, in these experiments, local pipette stimulation was delivered via a single pressure ejection of 10–20 psi and 10–25 ms via a General Valve Picospritzer as reported in Rose et al. (2009). Images were acquired at equal gain and contrast settings. Subtraction images (e.g., Figures 3C,D) were obtained by subtracting the pre-stimulus image from images captured at either an early (60 s) or late (360 s) time point. Images were analyzed for punctal fluorescence intensity and representative images were compiled for figures using FIJI.
Figure 2. Effects of CaMKII inhibition on GFP-CaMKII translocation. Fluorescence levels (A–C) [Mean (SEM)] of GFP-CaMKII-expressing puncta relative to initial GFP-CaMKII fluorescence levels across post-stimulation time points captured at 30-s intervals. (A) GFP-CaMKII expression in control (ECS; n = 10) and in the presence of the CaMKII(290–309) peptide inhibitor (n = 10). (B) GFP-CaMKII in control (ECS, n = 14), staurosporine (n = 12) or in the presence of the CaMKII(281–302) peptide inhibitor (n = 10). (C) CaMKII knockdown and re-expression of GFP-CaMKII (n = 6) or GFP-CaMKII(K42M; n = 16). (D) Mean (±SEM) relative GFP-CaMKII punctal fluorescence levels at early (90 s), mid (240 s) and late (360 s) translocation time points by condition. ECS and GFP-CaMKII knockdown and re-expression conditions combined as there was no significant difference between these control conditions (F(18,38) = 1.008, p > 0.40). *p < 0.05, **p < 0.01.
Figure 3. GFP-CaMKII translocation following local stimulation in neurons co-transfected with T286A. (A) Phase image of representative neuron showing micropipette captured prior to local stimulation. (B) GFP-CaMKII expression in the same neuron in (A) prior to stimulation. (C) Subtraction image (current fluorescence level minus initial fluorescence level; increases in fluorescence appear whiter while decreases are darker/black) captured within 60 s of local stimulation (early). (D) Subtraction image at 360 s post-stimulation (late). (E) Fluorescence intensity measured at an early (60 s) vs. late (360 s) time point following local stimulation. **p < 0.01.
CaMKII translocation was measured as an increase in fluorescence intensity of GFP-CaMKII expressing puncta following stimulation. Brief delivery of 100 μM glutamate plus 10 μM glycine via micropipette was sufficient to initiate a progressive translocation of GFP-CaMKII that reached maximum punctal fluorescence intensity within 5–6 min (Figures 1C,D). In dendritic regions where pre-stimulation GFP-CaMKII expression was diffuse, local glutamate plus glycine stimulation resulted in the gradual appearance of GFP-CaMKII expressing puncta while previously bright puncta frequently showed an increase in fluorescence intensity, although occasional decreases in fluorescence at these early-observable puncta were also seen (Figure 1C). Although an increase in puncta number was anticipated, the density of GFP-CaMKII expressing puncta did not show a significant difference between groups (F(4,69) = 0.878, p > 0.40, with Greenhouse–Geisser sphericity correction).
The presence of inhibitors produced differential effects on locally-induced CaMKII translocation resulting in a significant interaction between experimental conditions across time (F(12.25,211.24) = 3.31, p < 0.001, with Greenhouse–Geisser sphericity correction; Figures 2A–C). Simple main effects tests confirmed that inhibition of calmodulin blocked CaMKII translocation; the CaMKII(290–309) peptide inhibitor was sufficient to block translocation measured at early (90 s), mid (240 s) and late (360 s) time points (Figure 2D). Specifically inhibiting nucleotide binding to CaMKII with the K42M mutation did block locally-induced CaMKII translocation but only at the mid- and late time points (Figure 2D). The staurosporine and the CaMKII(281–302) peptide inhibitor conditions produced only moderate CaMKII translocation that was not statistically significant from translocation seen in the vehicle condition or the inhibited translocation in the calmodulin peptide inhibitor condition (p > 0.20). These results demonstrate that both CaMKII activation and nucleotide-binding are necessary for locally-induced CaMKII translocation.
The results confirm that a single brief (100 ms) application of 100 μM glutamate plus 10 μM glycine via local pipette is sufficient to trigger gradual CaMKII translocation by 6 min post-stimulation (Figure 2A). As expected, inhibition of calmodulin with the CaMKII(290–309) peptide inhibitor effectively prevented locally-induced CaMKII translocation (Figure 2A) similar to what has been previously reported with another calmodulin activation inhibitor of CaMKII, KN93 (Rose et al., 2009).
Overall, results from ATP inhibitor conditions were mixed; however, notably the K42M mutation that prevents nucleotide binding to CaMKII inhibited locally-induced CaMKII translocation (Figure 2C). This result was predicted as previous reports have shown that K42M prevents CaMKII translocation or aggregation following whole-cell stimulation with glutamate (Hudmon et al., 2005; Vest et al., 2009; O’Leary et al., 2011). However, in the current study inhibition of CaMKII translocation was not immediately notable as the K42M condition only reached significance compared to the vehicle condition after ~4 min post-stimulation (Figure 2D). Given the small increase in CaMKII punctal expression seen in the vehicle condition at the early time point (90 s), it is difficult to ascertain if the lack of a significant difference from the K42M condition at the early time point is meaningful. However, others have reported that although K42M prevents ATP-binding and kinase activation, it does not prevent association with F-actin or microtubules (O’Leary et al., 2011; Lemieux et al., 2012; Khan et al., 2016). Thus, it is possible that the lack of significant difference at the early time point for the K42M condition may be due to some weak initial ATP-independent peri-synaptic translocation of CaMKII.
Unlike the K42M condition, the staurosporine and the CaMKII(281–302) peptide inhibitor conditions resulted in moderate CaMKII translocation levels that were not significantly different from either vehicle or the CaMKII(290–309) calmodulin inhibitor condition (Figures 2B,D). There are several possible explanations for this outcome, including the possible role(s) of other kinases. In the current study, both staurosporine and the CaMKII(281–302) peptide inhibitor were employed at concentrations previously reported to be effective to inhibit CaMKII activation and CaMKII-ATP binding (Yanagihara et al., 1991; Smith et al., 1992; Barcomb et al., 2013). Given that ATP inhibition in these conditions is non-specific, perhaps some negative regulator of locally-induced CaMKII translocation was also inhibited. For example, phosphorylation at CaMKII site T305/T306 (α and β subunits, respectively) inhibits activation of CaMKII by calmodulin, predisposes neurons to long-term depression and decreases synaptic localization of CaMKII when co-expressed with the constitutively active T286D mutation (Patton et al., 1990; Hanson et al., 1994; Pi et al., 2010; Barcomb et al., 2014). As well, CaMKII translocation to microtubules resulting from whole-cell KCl stimulation is inhibited by the constitutively active T305D mutation (Lemieux et al., 2012). Thus, it is possible that the moderate decrease in locally-induced CaMKII translocation noted in the present study is the result of some ATP-dependent process that normally inhibits CaMKII translocation.
In addition to ATP inhibition, both staurosporine and the CaMKII(281–302) peptide inhibitor block the catalytic activity of the CaMKII molecule (Malinow et al., 1989; Barcomb et al., 2013). Thus, it is possible that the moderate effects of these inhibitors reflect some requirement of autophosphorylation for locally-induced translocation. However, co-expression of T286A (Figures 3A–B), a modification that impairs autophosphorylation (Giese et al., 1998), has little to no effect on locally-induced translocation (Figures 3C–E) similar to what has been previously reported with T286A for CaMKII translocation following global bath stimulation (Shen and Meyer, 1999).
Staurosporine alone has previously been reported to result in CaMKII translocation and potentially facilitates CaMKII binding to GluN2B receptor subunits (Barcomb et al., 2013). For the current study, it is possible that staurosporine itself somehow primed neurons for CaMKII translocation while inhibiting any ATP-dependent mechanisms. It is interesting that in the current study, bathing neurons for several minutes in staurosporine resulted in a moderate level of CaMKII translocation that developed gradually and appeared to be initiated by brief, local glutamate application. This is different to the seemingly maximal CaMKII translocation produced by staurosporine alone as reported in Barcomb et al. (2013); however, this difference may be a reflection of staurosporine concentrations as the current study used a staurosporine concentration of 100 nM while Barcomb et al. (2013) reported CaMKII translocation comparable to whole-cell glutamate-induced levels following bath delivery of 2 μM staurosporine. Taken together, the variability of results noted here is consistent with the equivocal outcomes others have reported with ATP inhibitors in whole-cell glutamate stimulation trials providing evidence that mode of action for inhibitors similarly must be considered for locally-induced CaMKII translocation.
Results from this study confirmed that ATP binding is necessary for locally-induced CaMKII translocation. Additional research is needed to identify phosphorylation sites involved. One possibility is autophosphorylation of the T253 site as this develops over a similar time scale of minutes (Migues et al., 2006). An alternative possibility is that local stimulation affects some part of the basal exchange of CaMKII between dendritic spines and shafts (a process that normally occurs between 1–5 min; Sharma et al., 2006; Lee et al., 2009; see Hell, 2014), thus resulting in a gradual accumulation of the molecule at spines. In addition to phosphorylation sites, the synaptic binding partner(s) of CaMKII following locally-induced translocation remains unknown. Studies into mechanisms of neuroplasticity have identified the K42 site as important for long-term potentiation and correlated spine enlargement (Yamagata et al., 2009; Kabakov and Lisman, 2015). Thus, it is of interest to identify mechanisms affected by brief, local glutamate stimulation and discern the functional purpose of this form of CaMKII translocation.
The datasets generated for this study are available on request to the corresponding author.
The animal study was reviewed and approved by Western Washington University Institutional Animal Care and Use Committee in strict accordance with the Guide for the Care and Use of Laboratory Animals described by the National Institutes of Health.
ZF performed all experiments, prepared dissociated hippocampal neuron cultures and designed manuscript figures under the direction and guidance of JR. Image processing and data extraction performed by ZF and JR. Initial and ongoing instruction and direction of experimental procedures, experiment conceptualization, statistical analyses and manuscript text composition performed by JR.
Research in this report was supported by the National Institute of Neurological Disorders and Stroke of the National Institutes of Health under award number 1R15NS070733 to JR. Publication funding provided by Western Washington University Office of Research and Sponsored Programs. The content is solely the responsibility of the authors and does not necessarily represent the official views of the National Institutes of Health.
The authors declare that the research was conducted in the absence of any commercial or financial relationships that could be construed as a potential conflict of interest.
We would like to thank Robert Bragg and Andrew Galbraith-Records for technical support.
The Supplementary Material for this article can be found online at: https://www.frontiersin.org/articles/10.3389/fnsyn.2020.00004/full#supplementary-material.
Barcomb, K., Buard, I., Coultrap, S. J., Kulbe, J. R., O’Leary, H., Benke, T. A., et al. (2014). Autonomous CaMKII requires further stimulation by Ca2+/calmodulin for enhancing synaptic strength. FASEB J. 28, 3810–3819. doi: 10.1096/fj.14-250407
Barcomb, K., Coultrap, S. J., and Bayer, K. U. (2013). Enzymatic activity of CaMKII is not required for its interaction with the glutamate receptor subunit GluN2B. Mol. Pharmacol. 84, 834–843. doi: 10.1124/mol.113.089045
Bayer, K.-U., De Koninck, P., Leonard, A. S., Hell, J. W., and Schulman, H. (2001). Interaction with the NMDA receptor locks CaMKII in an active conformation. Nature 411, 801–805. doi: 10.1038/35081080
Bayer, K.-U., LeBel, E., McDonald, G. L., O’Leary, H., Schulman, H., and De Koninck, P. (2006). Transition from reversible to persistent binding of CaMKII to postsynaptic sites and NR2B. J. Neurosci. 26, 1164–1174. doi: 10.1523/JNEUROSCI.3116-05.2006
Cheriyan, J., Kumar, P., Mayadevi, M., Surolia, A., and Omkumar, R. V. (2011). Calcium/calmodulin dependent protein kinase II bound to NMDA receptor 2B subunit exhibits increased ATP affinity and attenuated dephosphorylation. PLoS One 6:e16495. doi: 10.1371/journal.pone.0016495
Erondu, N. E., and Kennedy, M. B. (1985). Regional distribution of type II Ca2+/calmodulin-dependent protein kinase in rat brain. J. Neurosci. 5, 3270–3277. doi: 10.1523/JNEUROSCI.05-12-03270.1985
Giese, K. P., Fedorov, N. B., Filipkowski, R. K., and Silva, A. J. (1998). Autophosphorylation at Thr286 of the α calcium-calmodulin kinase II in LTP and learning. Science 279, 870–873. doi: 10.1126/science.279.5352.870
Halt, A. R., Dallapiazza, R. F., Zhou, Y., Stein, I. S., Qian, H., Juntti, S., et al. (2012). CaMKII binding to GluN2B is critical during memory consolidation. EMBO J. 31, 1203–1216. doi: 10.1038/emboj.2011.482
Hanson, P. I., Meyer, T., Stryer, L., and Schulman, H. (1994). Dual role of calmodulin in autophosphorylation of multifunctional CaM kinase may underlie decoding of calcium signals. Neuron 12, 943–956. doi: 10.1016/0896-6273(94)90306-9
Harms, K. J., and Craig, A. M. (2005). Synapse composition and organization following chronic activity blockade in cultured hippocampal neurons. J. Comp. Neurol. 490, 72–84. doi: 10.1002/cne.20635
Hell, J. W. (2014). CaMKII: claiming center stage in postsynaptic function and organization. Neuron 81, 249–265. doi: 10.1016/j.neuron.2013.12.024
Hudmon, A., Schulman, H., Kim, J., Maltez, J. M., Tsien, R. W., and Pitt, G. S. (2005). CaMKII tethers to L-type Ca2+ channels, establishing a local and dedicated integrator of Ca2+ signals for facilitation. J. Cell Biol. 171, 537–547. doi: 10.1083/jcb.200505155
Kabakov, A. Y., and Lisman, J. E. (2015). Catalytically dead αCaMKII K42M mutant acts as a dominant negative in the control of synaptic strength. PLoS One 10:e0123718. doi: 10.1371/journal.pone.0123718
Kaech, S., and Banker, G. (2006). Culturing hippocampal neurons. Nat. Protoc. 1, 2406–2415. doi: 10.1038/nprot.2006.356
Khan, S., Conte, I., Carter, T., Bayer, K. U., and Molloy, J. E. (2016). Multiple CaMKII binding modes to the actin cytoskeleton revealed by single-molecule imaging. Biophys. J. 111, 395–408. doi: 10.1016/j.bpj.2016.06.007
Lee, S.-J. R., Escobedo-Lozoya, Y., Szatmari, E. M., and Yasuda, R. (2009). Activation of CaMKII in single dendritic spines during long-term potentiation. Nature 458, 299–304. doi: 10.1038/nature07842
Lemieux, M., Labrecque, S., Tardif, C., Labrie-Dion, É., LeBel, É. and De Koninck, P. (2012). Translocation of CaMKII to dendritic microtubules supports the plasticity of local synapses. J. Cell Biol. 198, 1055–1073. doi: 10.1083/jcb.201202058
Malinow, R., Schulman, H., and Tsien, R. W. (1989). Inhibition of postsynaptic PKC or CaMKII blocks induction but not expression of LTP. Science 245, 862–866. doi: 10.1126/science.2549638
Migues, P. V., Lehmann, I. T., Fluechter, L., Cammarota, M., Gurd, J. W., Sim, A. T. R., et al. (2006). Phosphorylation of CaMKII at Thr253 occurs in vivo and enhances binding to isolated postsynaptic densities. J. Neurochem. 98, 89–299. doi: 10.1111/j.1471-4159.2006.03876.x
O’Leary, H., Liu, W. H., Rorabaugh, J. M., Coultrap, S. J., and Bayer, K. U. (2011). Nucleotides and phosphorylation bi-directionally modulate Ca2+/calmodulin-dependent protein kinase II (CaMKII) binding to the N-methyl-D-aspartate (NMDA) receptor subunit GluN2B. J. Biol. Chem. 286, 31272–31281. doi: 10.1074/jbc.m111.233668
Patton, B. L., Miller, S. G., and Kennedy, M. B. (1990). Activation of type II calcium/calmodulin-dependent protein kinase by Ca2+/calmodulin is inhibited by autophosphorylation of threonine within the calmodulin-binding domain. J. Biol. Chem. 265, 11204–11212.
Pi, H. J., Otmakhov, N., Lemelin, D., De Koninck, P., and Lisman, J. (2010). Autonomous CaMKII can promote either long-term potentiation or long-term depression, depending on the state of T305/T306 phosphorylation. J. Neurosci. 30, 8704–8709. doi: 10.1523/JNEUROSCI.0133-10.2010
Rose, J., Jin, S.-X., and Craig, A. M. (2009). Heterosynaptic molecular dynamics: locally induced propagating synaptic accumulation of CaM kinase II. Neuron 61, 351–358. doi: 10.1016/j.neuron.2008.12.030
Schindelin, J., Arganda-Carreras, I., Frise, E., Kaynig, V., Longair, M., Pietzsch, T., et al. (2012). Fiji: an open-source platform for biological-image analysis. Nat. Methods 9, 676–682. doi: 10.1038/nmeth.2019
Sharma, K., Fong, D. K., and Craig, A. M. (2006). Postsynaptic protein mobility in dendritic spines: long-term regulation by synaptic NMDA receptor activation. Mol. Cell. Neurosci. 31, 702–712. doi: 10.1016/j.mcn.2006.01.010
She, K., Rose, J. K., and Craig, A. M. (2012). Differential stimulus-dependent synaptic recruitment of CaMKIIα by intracellular determinants of GluN2B. Mol. Cell. Neurosci. 51, 68–78. doi: 10.1016/j.mcn.2012.08.001
Shen, K., and Meyer, T. (1999). Dynamic control of CaMKII translocation and localization in hippocampal neurons by NMDA receptor stimulation. Science 284, 162–167. doi: 10.1126/science.284.5411.162
Shen, K., Teruel, M. N., Connor, J. H., Shenolikar, S., and Meyer, T. (2000). Molecular memory by reversible translocation of calcium/calmodulin-dependent protein kinase II. Nat. Neurosci. 3, 881–886. doi: 10.1038/78783
Smith, M. K., Colbran, R. J., Brickey, D. A., and Soderling, T. R. (1992). Functional determinants in the autoinhibitory domain of calcium/calmodulin-dependent protein kinase II. Role of His282 and multiple basic residues. J. Biol. Chem. 267, 1761–1768.
Thalhammer, A., Rudhard, Y., Tigaret, C. M., Volynski, K. E., Rusakov, D. A., and Schoepfer, R. (2006). CaMKII translocation requires local NMDA receptor-mediated Ca2+ signaling. EMBO J. 25, 5873–5883. doi: 10.1038/sj.emboj.7601420
Vest, R. S., O’Leary, H., and Bayer, K. U. (2009). Differential regulation by ATP versus ADP further links CaMKII aggregation to ischemic conditions. FEBS Lett. 583, 3577–3581. doi: 10.1016/j.febslet.2009.10.028
Yamagata, Y., Kobayashi, S., Umeda, T., Inoue, A., Sakagami, H., Fukaya, M., et al. (2009). Kinase-dead knock-in mouse reveals an essential role of kinase activity of Ca2+/calmodulin-dependent protein kinase IIα in dendritic spine enlargement, long-term potentiation, and learning. J. Neurosci. 29, 7607–7618. doi: 10.1523/JNEUROSCI.0707-09.2009
Yanagihara, N., Tachikawa, E., Izumi, F., Yasugawa, S., Yamamoto, H., and Miyamoto, E. (1991). Staurosporine: an effective inhibitor for Ca2+/calmodulin-dependent protein kinase II. J. Neurochem. 56, 294–298. doi: 10.1111/j.1471-4159.1991.tb02595.x
Keywords: CaMKII, ATP—adenosine triphosphate, glutamate, synaptic, catalytic activity, translocation, kinase activation
Citation: Fitzgerald ZT and Rose JK (2020) Locally-Induced CaMKII Translocation Requires Nucleotide Binding. Front. Synaptic Neurosci. 12:4. doi: 10.3389/fnsyn.2020.00004
Received: 17 September 2019; Accepted: 17 January 2020;
Published: 07 February 2020.
Edited by:
Anis Contractor, Northwestern University, United StatesReviewed by:
Antonio Sanz-Clemente, Northwestern University, United StatesCopyright © 2020 Fitzgerald and Rose. This is an open-access article distributed under the terms of the Creative Commons Attribution License (CC BY). The use, distribution or reproduction in other forums is permitted, provided the original author(s) and the copyright owner(s) are credited and that the original publication in this journal is cited, in accordance with accepted academic practice. No use, distribution or reproduction is permitted which does not comply with these terms.
*Correspondence: Jacqueline K. Rose, amFja2llLnJvc2VAd3d1LmVkdQ==
Disclaimer: All claims expressed in this article are solely those of the authors and do not necessarily represent those of their affiliated organizations, or those of the publisher, the editors and the reviewers. Any product that may be evaluated in this article or claim that may be made by its manufacturer is not guaranteed or endorsed by the publisher.
Research integrity at Frontiers
Learn more about the work of our research integrity team to safeguard the quality of each article we publish.