- 1Neurobiology of Stress Laboratory, Facultad de Ciencias, Universidad Autónoma de San Luis Potosí, San Luis Potosí, Mexico
- 2School for Behavior and Brain Sciences, University of Texas at Dallas, Richardson, TX, USA
- 3Department of Psychiatry, University of Texas Southwestern, Dallas, TX, USA
- 4Laboratory of Cortical Plasticity and Learning, Universidad de Guadalajara, Guadalajara, Mexico
- 5Electrophysiology Laboratory, Centro de Investigaciones Regionales “Dr. Hideyo Noguchi”, Universidad Autónoma de Yucatán, Mérida, Mexico
Norepinephrine (NE) is synthesized in the Locus Coeruleus (LC) of the brainstem, from where it is released by axonal varicosities throughout the brain via volume transmission. A wealth of data from clinics and from animal models indicates that this catecholamine coordinates the activity of the central nervous system (CNS) and of the whole organism by modulating cell function in a vast number of brain areas in a coordinated manner. The ubiquity of NE receptors, the daunting number of cerebral areas regulated by the catecholamine, as well as the variety of cellular effects and of their timescales have contributed so far to defeat the attempts to integrate central adrenergic function into a unitary and coherent framework. Since three main families of NE receptors are represented—in order of decreasing affinity for the catecholamine—by: α2 adrenoceptors (α2Rs, high affinity), α1 adrenoceptors (α1Rs, intermediate affinity), and β adrenoceptors (βRs, low affinity), on a pharmacological basis, and on the ground of recent studies on cellular and systemic central noradrenergic effects, we propose that an increase in LC tonic activity promotes the emergence of four global states covering the whole spectrum of brain activation: (1) sleep: virtual absence of NE, (2) quiet wake: activation of α2Rs, (3) active wake/physiological stress: activation of α2- and α1-Rs, (4) distress: activation of α2-, α1-, and β-Rs. We postulate that excess intensity and/or duration of states (3) and (4) may lead to maladaptive plasticity, causing—in turn—a variety of neuropsychiatric illnesses including depression, schizophrenic psychoses, anxiety disorders, and attention deficit. The interplay between tonic and phasic LC activity identified in the LC in relationship with behavioral response is of critical importance in defining the short- and long-term biological mechanisms associated with the basic states postulated for the CNS. While the model has the potential to explain a large number of experimental and clinical findings, a major challenge will be to adapt this hypothesis to integrate the role of other neurotransmitters released during stress in a centralized fashion, like serotonin, acetylcholine, and histamine, as well as those released in a non-centralized fashion, like purines and cytokines.
Berserk
“Early 19th century (originally as a noun denoting a wild Norse warrior who fought with frenzy): from Old Norse berserkr (noun), probably from birn-, bjorn (bear) + serkr “coat,” but also possibly from berr “bare” (i.e., without armor).” Oxford Dictionary.
“His (Odin's) men rushed forwards without armor, were as mad as dogs or wolves, bit their shields, and were strong as bears or wild oxen, and killed people at a blow, but neither fire nor iron told upon them. This was called Berserkergang.” Ynglinga saga and Laing Samuel (1889). The Heimskringla or the Sagas of the Norse Kings. London: John. C. Nimo. p. 276.
“If a soldier survives the berserk state, it imparts emotional deadness and vulnerability to explosive rage to his psychology and permanent hyperarousal to his physiology—hallmarks of post-traumatic stress disorder in combat veterans. My clinical experience with Vietnam combat veterans prompts me to place the berserk state at the heart of their most severe psychological and psycho-physiological injuries.” Shay Jonathan (1994). Achilles in Vietnam. New York: Scribner. p. 98. ISBN 0-689-12182-2.
Introduction
Neurotransmitters Controlling the Spatio-Temporal Brain Activation Patterns
Evolution has shaped the mammalian brain during millions of years, endowing it with redundant and inter-related neurotransmitter networks to manage and administer stress. The characteristics of the “berserk,” the ultimate warrior—superhuman physical strength, insensitivity to pain, lack of concern for the consequences of his actions—are possibly the display of an extreme state, an upper limit of human physical and mental condition at the core of norepinephrine (NE)-induced states.
Although a variety of hormones may turn on neuronal circuits for the execution of energetically demanding behavioral tasks, only a fistful of neurotransmitters have the capability to actually regulate the global state of activation of the whole brain, managing effectively and parsimoniously the necessarily limited energy/power capability of the brain and of the whole organism. The NE-releasing Locus Ceruleus (LC) is anatomically and functionally intertwined with the brain area which is arguably the major recipient of stress-related information: the paraventricular nucleus of the hypothalamus (PVN, Figure 1). Other hypothalamic nuclei also impinge upon the LC. Among them the hypocretin-expressing nuclei in the lateral hypothalamus (Henny et al., 2010; Carter et al., 2012). The hypothalamus-LC axis controls input and output information from and to the autonomic system through the brainstem, to and from the neuroendocrine system through the pituitary gland and numerous gland-to-brain biochemical feedback loops, as well as all the rest of the central nervous system (CNS), through brain and spinal cord volume transmission (Figure 1).
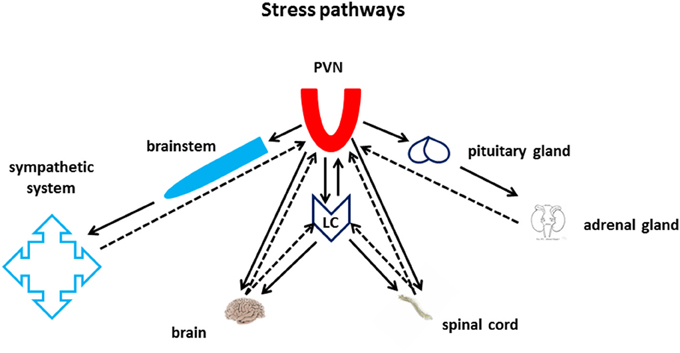
Figure 1. Stress pathways. The Nucleus Paraventricularis of the Hypothalamus (PVN) and the Locus Ceruleus lie at the core of the CNS stress pathways. Both areas are at the center of an intense bi-directional information exchange with multiple targets in the periphery, within the brain, and with each other. The PVN sends and receives information to and from the autonomic nervous system through the brain stem, and from and to the periphery through the neuroendocrine axes. The LC sends and receives information to and from the spinal cord and the whole brain. Furthermore, PVN and LC also share monosynaptic bi-directional communication through the medial forebrain bundle.
Further hints of the biological importance and pervasiveness of central adrenergic function come from the analgesic properties of NE and its agonists (Simpson and Lin, 2007), by its important role in the control of body temperature, like during inflammatory response (Bencsics et al., 1995; Ordway et al., 2007; Osaka, 2009), as well as from the observation that other relevant input to the LC originate from nuclei coordinating vital functions like sex/reproduction (Nucleus Paragigantocellularis), respiration (Parabrachial and Solitary Tract Nuclei), and vestibular balance control (Simpson and Lin, 2007).
The control of the above functions is likely retained in the evolution from lower to higher mammals (Tohyama et al., 1974), but the increase in brain size and complexity associated with its disproportionate anatomical development makes the mammalian CNS particularly vulnerable to sudden surges in energy consumption caused by stressful situations. This latter evolutionary purpose might have further strengthened the importance of LC as master energy hub (Berridge and Waterhouse, 2003; O'Donnell et al., 2012), enhancing—particularly in humans—its role in the etiology of stress-related conditions.
Functional and Anatomical Peculiarity of the LC/NE System
Many hormones have a potential for global control of energy expenditure and activity regulation. Among them—for instance—the corticosteroid system is well placed for exerting a global and sophisticated biochemical regulation of energy demand and distribution (De Kloet, 2004), but lacks the property of anatomical and functional contiguity that the LC possesses. For similar reasons, the cytokine network, which also has the potential to control the brain (and bodily) global energy distribution (Guijarro et al., 2006), also does not seem to qualify as “central energy master.”
Central cholinergic fibers made up a highly divergent and almost ubiquitous release system (McKinney and Jacksonville, 2005; Smythies, 2005a). However, the existence of a large number of nuclei and brain areas that independently control the release of acetylcholine (Ach; Nucleus Basalis of Meynert, medial septum, latero-dorsal tegmentum, etc.), each toward or within their respective anatomical targets suggests that the cholinergic system hardly exerts a genuinely centralized control of energy expenditure.
The central histaminergic system stemming from the tuberomammillary nucleus of the hypothalamus appears to play a powerful and genuinely centralized role in triggering an emergency and alert maintenance response (Wada et al., 1991; Sakata et al., 1997; Shan et al., 2015). To our present knowledge, though, the histaminergic system does not appear to display a repertoire of cellular and synaptic actions paralleling the complexity and flexibility of the LC/adrenergic system, which is perhaps rivaled in its pervasiveness and variety of effects only by the Raphe/5HT system (Heisler et al., 2003; Zhou et al., 2005). In this respect, only serotonergic projections from the Raphe nucleus and the cholinergic fibers from the basal forebrain (Nucleus Basalis of Meynert) reach the extent and density of LC adrenergic projections throughout the CNS (Smythies, 2005b). The importance of the serotoninergic system in the coordination of the stress response has been reviewed elsewhere (Waselus et al., 2011).
While we highlight the importance of developing a comprehensive theory integrating the roles of the many neurotransmitters involved in the stress response, we will henceforth limit our discussion on the role of NE. In the following sections we will discuss experimental evidence relating central NE function to activities related to stress (stress perception, elaboration, and execution of a stress-ridding plan, as well as storage—or deletion—of related memories), and will make an attempt to integrate previous literature into a qualitative model in which increasing levels of NE co-ordinate the activity of different brain areas, inducing global brain states with increasing energy consumption and stress levels. We will only briefly mention the effects of NE on long-term processes, which we have recently reviewed elsewhere (Salgado et al., 2016).
It is worthwhile emphasizing the genuinely global nature of NE, differing from its chemical precursor dopamine, whose cortical projecting axons target more selectively the prefrontal cortex (Robbins and Arnsten, 2009). For the sake of clarity, we would like to highlight that stress activates two distinct pools of NE: a central one and a peripheral one, the latter associated with sympathetic nervous system activation. While the interaction between the two pools is essential to the understanding of the systemic effects of stress, only the former will be considered in the present discussion.
Stress, HPA Axis, and LC Activation
For the purpose of this discussion, we will broadly define stress as any situation in which an organism increases its energy consumption beyond an expected or biologically bearable range (which greatly varies even among individuals of the same species), and as stressor its objective or perceived source. We will get back to this definition of stress in Section LC-CNS Interactions. In the presence of most types of stressors the brain carries out the following (conscious or subconscious) functions: (1) evaluation of the stressor characteristics (short- or long-term intensity, duration, and consequences), (2) elaboration of a strategy to eliminate the stress(or), (3) execution of such plan, and (4) long-term storage (or in some case erasing) of stressor-related memories. Among these memories are the inner representations of the stress as a measure of potential danger, as well as the representation of one or more actual stress exit strategies, and their perceived effectiveness (or lack thereof).
In the historical context of the studies of the stress response, a critical element of adrenergic effects had been recognized early in the interaction between the hypothalamus-pituitary-adrenal gland (HPA) neuroendocrine axis and LC reviewed in Gold and Chrousos (2002) and Gold (2015). In fact, NE-releasing neurons of the LC are important targets of corticotropin-releasing hormone (CRH)-producing hypothalamic neurons from the PVN (Nicolaides et al., 2015), as well as from other limbic areas including the amygdala (Ordway et al., 2007), whose activity in turn stimulates LC leading to NE release. The importance of the PVN-LC axis is underscored by the observation that the inactivation of glucocorticoid receptors in the LC induces depression-like symptoms in a mouse model (Chmielarz et al., 2013).
A critical feature of the well-studied HPA response to stress is the negative feedback between the production of glucocorticoids and the activation of the HPA axis, which occurs both at the level of CRH-producing neurons in the PVN of the hypothalamus as well as in pituitary ACTH-producing corticotrophs (Figure 2). An energetically meaningful consequence of the elevation of glucocorticoid levels is the parallel shut-down or at least decrease of the high-energy consuming immune adaptive system, which in turn may increase the chance of infection and cancer in chronically stressed individuals (Reiche et al., 2004).
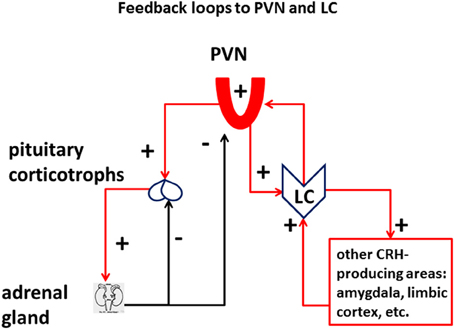
Figure 2. Feedback loops to PVN and LC: Vulnerability of the LC in the stress axes. The LC is integral part of the stress response, in addition to the HPA axis. Different from the HPA axis, which receives a double negative-feedback (minus signs) of corticosteroids from the suprarenal gland, both at the level of the pituitary and the paraventricular nucleus of the hypothalamus (PVN), PVN, and other CRH-releasing cells in the CNS are connected to the LC through a positive-feedback loop (plus signs), which has the potential to derange the energy equilibrium of the system.
Importantly—unlike the glucocorticoid negative-feedback on HPA axis—the activation of the PVN/CRH/NE/LC branch of the stress response not only does not produce a negative feedback (Gold, 2015), but produces a positive feedback which opposes and jeopardizes the closure of the HPA loop associated with glucocorticoids (Figure 2). The presence of a feedforward loop between CRH-producing areas of the cortex and of the hypothalamus and the LC is a risk factor in the induction of maladaptive plasticity of the stress system, which greatly enhances its vulnerability to intense and/or chronic challenge. Systemic inflammation can be considered as the opposite phenomenon, whereby a combined action of pro-inflammatory cytokines induces a temporary state of physical apathy and inaction (Haroon et al., 2012; Miller et al., 2013). A consequence of such “sick response” is to spare systemic energy and promote a prompt recovery of the organism affected by a viral or bacterial infection.
Decade-long seminal work from the group of Aston-Jones and Waterhouse provides a solid ground for assessing the basic functions and activity dynamics of the LC (Rajkowski et al., 1994; Aston-Jones et al., 1998; Usher et al., 1999; Aston-Jones and Cohen, 2005; Aston-Jones and Waterhouse, 2016). Using mostly in vivo electrophysiological recordings from both primate and rodent models, this body of work has shown that LC displays virtually no activity during the sleep phase, whereas during the wake state it displays two emergent firing patterns: a tonic one, associated with the arousal level of the animal, and a phasic one, related with decision making and attention. Importantly, the extent of LC phasic firing appears to follow an inverse-U shape function with respect to the levels of tonic LC firing. In fact, while low levels of tonic firing—as during low arousal level—are insufficient to elicit a consistent behavioral response, intermediate tonic levels yield optimal phasic firing, whereas high levels of tonic firing—associated with high arousal and limbic activation—yield low phasic response as LC units firing activity gets closer to saturation. The eventual overall effect of NE depends on the spatio-temporal pattern of its volume release (Fuxe et al., 2010), on the nature of the cellular and synaptic targets affected, and on the type of receptor activated (summarized in Figure 3).
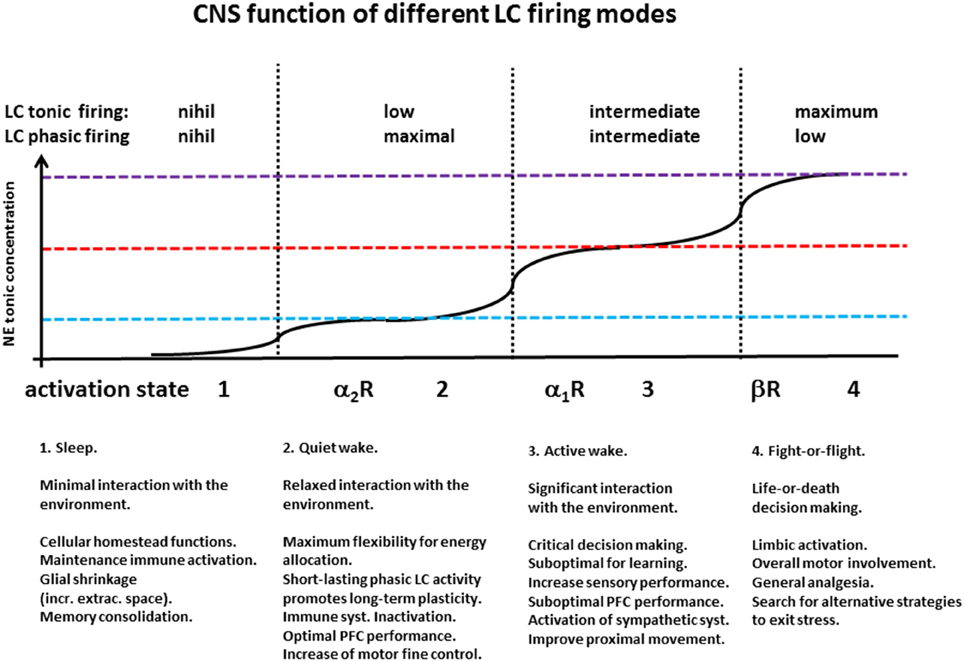
Figure 3. CNS function of different LC firing modes. Seminal work from Aston-Jones groups has shown the existence of a relationship between behavioral states and LC tonic and phasic firing patterns: During sleep, LC cells display low or no activity (vertical axis in arbitrary units—A.U.); during quiet wake they display modest tonic firing, and phasic responses to behavioral stimuli; in conditions of intermediate tonic release, associated with moderate stress and energy demand, LC presents its highest phasic response during biologically relevant behavioral responses; the highest level of LC tonic firing occurs in situations of arousal and fight-or-flight response, and is associated with the lowest levels of phasic LC activity.
Molecular, Cellular, and Synaptic Effects of NE
NE is released in the brain by axonal varicosities via volume transmission (Grzanna, 1980; Jones and Yang, 1985; Agnati et al., 1995). NE receptors have been first studied in the periphery and subsequently identified throughout the whole CNS, with different densities and regional specializations as reviewed earlier (Ramos and Arnsten, 2007). In terms of molecular effects, they can be categorized into three main groups, in descending order of affinity: α2Rs, (≈50 nM), α1Rs (≈300 nM), and βRs (≈0.7–0.8 μM) reviewed in Ramos and Arnsten (2007). Both α2- and β-Rs are known to activate guanosine-dependent (G–)protein receptors, each affecting adenylyl cyclase in opposite directions, namely by decreasing (α2Rs) or increasing (βRs) the intracellular concentrations of cyclic adenosine monophosphate (cAMP). In contrast, α1Rs activate phospholipase C (PLC), thus triggering the synthesis of intracellular diacylglycerol and activation of protein kinase C as well as of inositol phosphate, which in turn releases Ca2+ from intracellular stores (Ramos and Arnsten, 2007).
The existence of widespread families of high-affinity neurotransmitter receptors (NE α2Rs, M2 muscarinic, D2 dopaminergic) whose activation decreases adenylyl cyclase activity (Gi) suggests that basal (tonic) cytosolic levels of cAMP are not zero, and that they concur to the regular maintenance cellular processes active during cell rest. As a corollary, we hypothesize that the inactivity of the CNS noradrenergic system—similar to that of the other monoaminergic and the cholinergic system—during sleep is associated with a non-zero level of cAMP (Figure 4), and a tonic level of cellular energy expenditure. Such ground-level of cellular metabolic activity is possibly necessary to carry out a number of functions including a temporary enhancement of immune function during the resting phase (Kamath et al., 2015) and memory consolidation (Wilson and McNaughton, 1994; Barnes and Wilson, 2014; Figure 3). A slight increase in the concentration of neurotransmitters activating a Gi (in the case of NE up to 100 nM) could be sufficient to shut down such sleep-associated maintenance cellular processes and re-direct cellular metabolic energy to the quiet-wake related activities. Only relatively higher NE concentrations (around or above 0.4 μM) would be able to solidly activate the PLC cascade and increase cAMP levels above its resting levels (Figure 3), increasing the cellular supply for more energetically demanding biological activities (Figure 4).
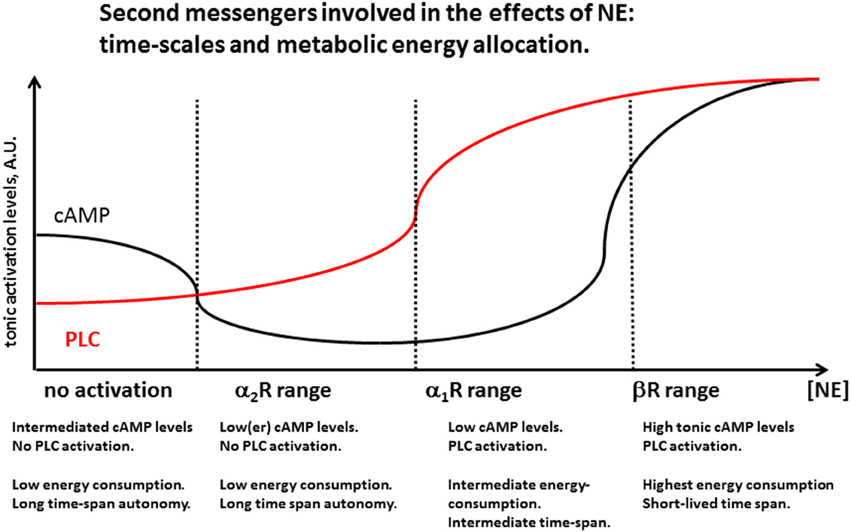
Figure 4. Second messengers involved in the effects of NE: time-scales and metabolic energy allocation. Increasing levels of NE activate noradrenergic receptors by first decreasing cAMP levels by activation of α2Rs, probably reducing homestead maintenance cellular function active during sleep. A further increase in NE concentration activates α1R, activating the phospholipase C (PLC) cascade while cAMP levels are still low. For still higher levels both PLC and cAMP levels are heightened, consistent with highest levels of cellular activation. Energetic considerations suggest that this high-demand state need to be associated with decreased function in at least some brain areas, and has necessarily to be short-lasting, in order to prevent depletion of organismic energy stores and desensitization of membrane receptor mechanisms. Periods of brief and intense LC activation like during its phasic release may induce temporary activation of βRs associated with memory and learning. Prolonged high LC activity may be detrimental for learning and memory as it would necessarily reduce phasic LC activity and reduce the spatial and temporal specificity of βR synaptic effects.
Cellular and synaptic adrenergic modulation (Salgado et al., 2016) suggests that the activation of the cortical branch of the LC/adrenergic system could simultaneously perform two tasks: (1) single neuron activation by modulation of intrinsic conductances, with consequent local mobilization of large amounts of metabolic energy, and (2) temporary shut down or depression of the activity of other cortical areas that are unnecessary or irrelevant to the resolution of a particular behavioral contingence. This end could be achieved by a combination of selective depression of excitatory and enhancement of inhibitory synaptic transmission (Waterhouse et al., 1991; Sessler et al., 1995; Salgado et al., 2016).
Heterogeneous mechanisms of adrenergic modulation in different cortical areas like sensory vs. prefrontal cortices (Salgado et al., 2011, 2012b; Roychowdhury et al., 2014) together with activity-dependent modulation may concur to a functional selective enhancement or depression of neuronal activity in specific areas (Hains and Arnsten, 2008; Arnsten et al., 2012; Edeline, 2012; Roychowdhury et al., 2014). In the following sections we will review experimental evidence of effects of the noradrenergic receptor families mentioned above, from clinics and animal models, in an attempt to condense the related information in an integrated view. In many cases it will be hard to guess how a particular cellular or synaptic phenomenon participates into a behavioral function. In the tables we will either report the author's interpretation of their experimental finding, or will formulate a plausible one, keeping in mind that the same cellular experimental data may play different roles in a systemic function.
α2Rs Central Modulation
α2Rs are present in many brain areas in both pre- and post-synaptic membranes, as well as in glia (Lee et al., 1998).
Alertness and Anxiolytic Effects
Based on pharmacological observations in clinics and in animal models, the activation of α2Rs is deemed necessary for optimal performance in working memory and other tasks carried out with a strong prefrontal cortex (PFC) component (Gamo and Arnsten, 2011; Arnsten and Jin, 2012, 2014). Along with promoting working memory—and possibly related to it—α2Rs also appear to contribute to a plethora of functions such as attention and impulse inhibition (Brennan and Arnsten, 2008; Robbins and Arnsten, 2009). The activation of α2Rs decreases the inhibitory synaptic drive onto the tuberomammillary nucleus of the hypothalamus, contributing to alertness (Nakamura et al., 2013). α2R activation in the medial septum and hippocampus increases theta rhythm (Kitchigina et al., 2003), presumably enhancing cognition. The increase in spontaneous inhibitory postsynaptic currents (sIPSCs) in the PVN following α2R agonist application (Chong et al., 2004), together with the α2R-induced decrease in glutamatergic drive onto the ventral tegmental area (VTA) may contribute at least part to α2Rs anxiolytic properties.
PFC Activity Modulation
Ample evidence exists that α2Rs directly modulate PFC activity (Kovács and Hernádi, 2003; Wang et al., 2010), reviewed in Arnsten and Li (2005). Most of this literature indicates a beneficial effect of α2R activation for working memory, although in some studies beneficial effects of α2R blockers have been described (Brown et al., 2012; Bari and Robbins, 2013). The α2R-induced block of N-methyl-D aspartate receptor (NMDAR)-mediated current (Liu et al., 2006), would corroborate a role in PFC learning for this receptor, but it could also represent a faster “clearance” of PFC reverberant circuits. Particularly remarkable is the evidence from multiple studies, of the beneficial effects of α2R-induced block of dendritic hyperpolarization-activated cyclic nucleotide (HCN) channels (Wang et al., 2011; Zhang Z. et al., 2013), whose age-related decline is considered an important cause of cognitive deterioration (Wang et al., 2011).
Motor and Sensory Activity
α2R activation does modulate motor activity (Villégier et al., 2003), although not always in the same direction (Cathala et al., 2002; Carey and Regehr, 2009). α2R activation appears to modulate cerebellar activity, necessary for fine timing and control of distal movement (Hirono and Obata, 2006; Di Mauro et al., 2013; Lippiello et al., 2015). Little evidence is reported of α2R modulation of sensory areas, mainly in auditory (Leão and Von Gersdorff, 2002; Salgado et al., 2011) and olfactory (Nai et al., 2009) areas.
Clinical and Pre-clinical Studies
Different—sometimes contradictory—evidence about the global effects elicited by α2R ligands may perhaps be explained as the result of two contrasting actions on excitatory (pro-convulsive) presynaptic α2Rs and inhibitory (anti-convulsive) postsynaptic α2Rs, as revealed by an epilepsy study (Szot et al., 2004). It is worth mentioning that the use of tricyclic medication, used as antidepressant, may induce α2R internalization (Cottingham et al., 2015), perhaps indicating that depression may be associated with or even caused by an increase in α2R expression, possibly elicited by a high NE tone associated with prolonged stress.
Overall, the activation of α2Rs by an increased but moderate NE tone (possibly ≤ 100 nM), appears to increase alertness, improve working memory, attention, PFC function in general, and enhance fine motor control and sensory processing, possibly acting on pre- and post-synaptic receptors carrying out opposite functions. Table 1 reports a series of α2R-mediated effects grouped per putative function.
α1Rs Central Modulation
A somehow controversial picture emerges from the literature concerning the roles of α1Rs, reporting either facilitatory or detrimental effects of cognitive function following the activation of α1Rs, depending on the assay used. Remarkable information comes from studies of different subtypes of α1R in constitutively activated mutant (CAM) mice, reviewed elsewhere recently (Nalepa et al., 2013). These studies suggest complex—sometime opposite—interplay of the different subtypes of α1Rs. CAM mice overexpressing α1BRs display neurodegeneration and grand mal-like seizures, probably caused by an imbalance between excitatory and inhibitory synaptic currents. Behavioral assays on these animals suggest a role for α1BRs in memory consolidation and fear-driven exploratory behavior (Knauber and Müller, 2000). On the other hand, α1ARs CAMs live 10% longer than controls, and display improved memory and learning (Doze et al., 2011), opposite to α1BRs CAMs (Collette et al., 2014). Another subtype of α1Rs, the α1DR appears to be inversely related to motor control, as α1DR KO mice perform better in the rotarod test (Mishima et al., 2004).
General Activation and Emotion Regulation
In general, α1Rs activation promotes wake and activity by directly affecting neurons (Schmeichel and Berridge, 2013; Igata et al., 2014), and possibly also by activating astrocytes (Pankratov and Lalo, 2015). Activation of α1Rs also concurs to the anorexigenic effect of NE and amphetamines (da Silva et al., 2014).
A specific and consequential effect of α1R activation is emotion control. The decrease of the inhibitory drive onto the VTA may indicate an increase in motivation (Velásquez-Martínez et al., 2015). α1Rs are also clearly involved in the stress response, as revealed by acute restraint stress (Alves et al., 2014), predatory stress (Rajbhandari et al., 2015), and maternal separation (Coccurello et al., 2014) studies. In agreement with its role in the stress response, block of α1Rs impairs HPA activation (Yang et al., 2012).
Working Memory and Motor Control
α1R positive modulation of working memory and other PFC function also seems to be solidly established by a wealth of data. For instance, α1R activation improves working memory deficit induced by applications of the GABAAR agonist muscimol (Hvoslef-Eide et al., 2015), while α1R block disrupts the “go” performance in a “go-no-go” task (Bari and Robbins, 2013). At the synaptic basis of these effects could lay an enhancement in glutamatergic function (Luo et al., 2014b, 2015a), which may, in turn, yield a general increase in firing frequency in the PFC (Zhang Z. et al., 2013). Other cellular and synaptic effects of α1R activation in the PFC, like an increase in inhibitory drive (Luo et al., 2015b) or a specific decrease in NMDAR-mediated response are not necessarily prone to similarly straightforward interpretations.
Motor effects of α1R activation appear to be associated with a generalized increase in motor activity (Villégier et al., 2003), accompanied by a reduced fine motor control (Aono et al., 2015), suggested also by improved rotarod performance of KO α1R mice (Mishima et al., 2004). A decreased glutamatergic cerebellar drive may concur to a reduced distal motor control (Lippiello et al., 2015).
Sensory Modulation, Memory, and Learning
Even more puzzling are the effects of α1R activation on sensory activity. While the effectiveness of α1R activation on sensory areas appears to be well established, its overall function remains enigmatic, possibly due to opposite effects on excitatory and inhibitory synaptic systems, as well as to a genuine heterogeneity of the response to different sensory modalities. For instance, α1R activation increases firing in the somatosensory cortex (Devilbiss and Waterhouse, 2000), but decreases firing frequency and responses to glutamate in the visual (Terakado, 2014) and in the auditory cortex (Manunta and Edeline, 1997; Dinh et al., 2009). In the latter—in turn—activation of α1Rs elicits opposite responses on electrically-evoked GABAergic transmission originating from different cortical layers (Salgado et al., 2011, 2012a). αRs (possibly α1Rs) are involved in auditory cortex activity-dependent plasticity evoked by electric or optogenetic stimulation of LC (Martins and Froemke, 2015).
The olfactory bulb is also not exempt from displaying apparently contrasting α1R-induced effects, like an increase of GABAergic response (Zimnik et al., 2013) and membrane depolarization (Nai et al., 2009). The effects of α1R activation on sensory areas may be related to sensory modality selection after adrenergic activation, and/or maintenance of the excitatory/inhibitory balance following intense activation.
On the other hand, α1R activation elicits clearly positive effects on memory and learning (Doze et al., 2011), as corroborated by studies on constitutively active α1AR mentioned earlier (Collette et al., 2014), and by a worsened learning and working-memory related performance in KO α1R mice (Spreng et al., 2001). An increase in rebound excitation and neuronal ensemble synchronization associated with an α1R–mediated increase in GABA release in the entorhinal cortex (Lei et al., 2007; Cilz et al., 2014) may be at the root of at least some of the α1R–induced improvements in learning and memory.
Clinical Data
Depression, psychosis, and numerous treatments for stress-related neuropsychiatric disease appear to modulate importantly α1Rs expression and function, although the direction of such modulation is not always consistent with illness or therapeutic effects. For instance, long-term administration of imipramine or electroconvulsive therapy increase the expression of α1Rs (Nalepa et al., 2002), but the antidepressant effects of other tricyclic antidepressants (TCAs) (Ramakrishna and Subhash, 2012) or quetiapine (Nikiforuk, 2013) reduce α1Rs expression. The interpretation of these results is further complicated by the age-dependence of α1Rs function (Deupree et al., 2007). Table 2 summarizes some of the systemic and cellular effects associated with α1Rs activation.
βRs Central Modulation
Similar to α2Rs and α1Rs, the distribution of the various subtypes of βRs in the brain is almost ubiquitous in the mammalian brain (Paschalis et al., 2009; Ursino et al., 2009). βRs are, in fact, expressed in both excitatory and inhibitory cells in the cortex as well as in subcortical nuclei (Cox et al., 2008; Salgado et al., 2011; Liu et al., 2014). Among the latter, the amygdala is endowed with an especially high βRs density (Abraham et al., 2008).
Alertness, Wake, and Metabolism
Many functions identified for αRs are also brought about by βRs activation. One of them is wake and alertness (Schmeichel and Berridge, 2013). Especially interesting is the effect of βRs activation on astrocytes (Song et al., 2015; Dienel and Cruz, 2016; Sherpa et al., 2016), which induces a decrease in extracellular brain volume. βR are also neuroprotective (Laureys et al., 2014), and decrease endotoxin-induced toxicity (Jiang et al., 2015), possibly by eliciting process retraction in resting microglia (Gyoneva and Traynelis, 2013), in contrast with the induction of neurite growth in cultured cortical primary neurons (Day et al., 2014).
Cognition and Sensory Areas
βRs exert their effects in many sensory areas including the somatosensory cortex (Devilbiss and Waterhouse, 2000), the visual cortex (Terakado, 2014), the auditory cortex (Manunta and Edeline, 2004; Salgado et al., 2011), cochlear nucleus, lateral lemniscus, inferior colliculus (Wanaka et al., 1989), and the olfactory bulb (Shakhawat et al., 2015). Activation of βRs impairs sustained attention (Bari and Robbins, 2013), and increases the power (but not the frequency) of γ-oscillations (Haggerty et al., 2013), apparently without impairing cognitive flexibility (Steenbergen et al., 2015).
Similar to the sensory effects of αRs described in the previous sections, the effects of βRs do not necessarily appear to converge onto an unequivocal single function, representing either genuine differences between sensory areas, or recovery of the excitation/inhibition balance through adjustment of synaptic strength or other cellular mechanisms.
Limbic and Motor Function
The body of knowledge concerning the effects of βRs on a variety of limbic functions is remarkably consistent with the hypothesis that a high concentration of tonic NE is critical for eliciting or modulating emotion. Fear memory—for instance—is impaired after administration of βR blockers (Fitzgerald et al., 2015; Zhou et al., 2015), and βR activation interferes with fear extinction induced by novel stimuli (Liu et al., 2015). Interestingly, social stress generates microRNA which decreases fear response acting on βRs (Volk et al., 2014). These data indicate that βRs activation is unequivocally associated with fear and fear memory, most likely because of their high expression in the amygdala. βRs are also causally related to anxiety generation, as suggested by the anxiogenic effect of βR-agonist administration (Hecht et al., 2014), and corroborated by elegant experiments where βRs were activated via optogenetic means (Siuda et al., 2015). Interestingly, βR blockers also reduce the anxiogenic effect of cocaine intake (Wenzel et al., 2014), while βR agonist administration within the pre-Botzinger complex increases spontaneous sigh frequency (Viemari et al., 2013).
Perhaps not surprisingly, βRs are important mediators of stress effects. For instance, restraint stress reduces dopaminergic effects but not after blocking βRs (Chang and Grace, 2013). Along the same line, stress elevates LC release of NE, leading to desensitization of βRs, an effect that—if chronic—may give rise to a depressive behavioral phenotype (Porterfield et al., 2012). Motor function appears to be improved by βR activation. βR agonists increase cerebellar GABA input (Di Mauro et al., 2013), increase excitatory input to Purkinje cells, and decrease the threshold for cerebellar LTD (Lippiello et al., 2015), although worsening spatial orientation performance (Robinson et al., 2015).
Memory and Learning
A wide experimental database supports a positive effect of βRs in learning and memory (Salgado et al., 2016). For instance, βR activation increases long-term potentiation (LTP) in the hippocampus and in the neocortex (Laing and Bashir, 2014; Hansen and Manahan-Vaughan, 2015; O'Dell et al., 2015) and memory retrieval, possibly by shutting down an after hyperpolarization activated (AHP) current (Zhang L. et al., 2013; Zhou et al., 2013), by “unsilencing” of silent synapses (Rozas et al., 2015), but also by inducing hippocampal long term depression (LTD; Goh and Manahan-Vaughan, 2013; Lethbridge et al., 2014).
βRs involvement in long-term synaptic plasticity is further indicated by the increased predisposition to long-term changes in both GABAergic synapses (Inoue et al., 2013) and glutamatergic synapses (Maity et al., 2015) following exposure to βRs or stress, in a βR-dependent manner (Grigoryan and Segal, 2013; Grigoryan et al., 2015).
Neuropsychiatric Disease
The role of βRs in neurologic and psychiatric disease is an example of bell-shaped curve: on one hand, a βR deficit is associated with CNS malfunction and impairment, on the other one, it is a βRs hyperactivation causes distress and neurodegeneration. The former instance is epitomized by the βRs deficits associated with decreased LC-adrenergic function in aging (Santulli and Iaccarino, 2013). The finding of antibodies against βRs in the plasma of chronic fatigue syndrome (Loebel et al., 2016), and the improvement in memory (Dang et al., 2014) and cognitive performance (Phillips et al., 2016) in Down syndrome patients treated with βR agonist highlight the global importance of the βR-dependent component of noradrenergic transmission. The involvement of βRs in Alzheimer disease (AD) symptomatology is somehow controversial. For instance, βR activation appears to increase tau-protein phosphorylation—one of the hallmarks of AD (Wang et al., 2013), while routine presentation of novel stimuli is reported to protect from the toxicity from β-amyloid—another important AD marker—through βR activation (Li et al., 2013).
A βR-dependent increase in excitatory transmission in the bed nucleus stria terminalis (BNST) has been interpreted as distress factor in drug addiction seeking behavior (Egli et al., 2005), while βR block has been proposed as treatment for depression-related allodynia (Barrot et al., 2009). A possible general interpretation of the body of work related to the function of βRs in the context of stress is that short-term, acute, activation of βRs promotes demanding performances, whereas their chronic stimulation may lead to detrimental consequences of the same functions promoted by βRs short-term action. Table 3 summarizes recent experimental work on βR central function.
Use of β-Blockers in the Treatment of Psychiatric Disease
The high expression and high functional relevance of βRs in the amygdala and overall in the initiation of stress response would prompt them as target for pharmacological intervention in the treatment of stress-related psychiatric illness. Administration of the βR blockers, indeed, does decrease the behavioral and biochemical effects of social stress (Wohleb et al., 2011), of restraint stress (Tamburella et al., 2010), and shock-probe defensive burying response (Bondi et al., 2007), possibly by inhibiting cytokine release from microglia, among other effects (Wohleb et al., 2011). Promising results in the treatment of acute effects of stress come from the development of the blood-brain permeable β3R agonist amibegron (Stemmelin et al., 2008). Activation of βRs may be an important therapeutic component of the antidepressant effect of mirtazapine (Rauggi et al., 2005).
An old hypothesis positing that the therapeutic effect of antidepressant was due to downregulation of βRs (as elicited by TCA treatment; Peet and Yates, 1981) has long been discarded (Charney et al., 1986). βRs agonists have been proposed also in the treatment of the memory impairment associated with psychotic schizophrenia, but the detrimental effects of βR-agonists on working memory and general cognitive flexibility, prevent their routine use (Friedman et al., 2004). In spite of a clear involvement of βRs (and CRH receptors) in amygdala activation in the etiology of PTSD, less or no effective has been the use of βR blocker in the long-term treatment of post-traumatic stress disorder (PTSD; Amos et al., 2014) and schizophrenia (Wahlbeck et al., 2000). βR blockers lack of effectiveness may perhaps be explained by the occurrence of βR internalization induced by their persistent activation. βR internalization was one of the first β-arrestin mediated processes to be described (reviewed in DeWire et al., 2007). Neurons have among the highest expression of non-visual β-arrestin in the whole mammalian body (Gainetdinov et al., 2004). Stress activates β-arrestin mediated internalization of βRs as well as internalization of corticotropin-releasing hormone (CRH) type 1 receptors (Hauger et al., 2009). Either process is an important mechanism of neuronal desensitization to stress response. A related third neuronal desensitization process is the G-protein receptor kinase (GRK)—mediated switch of G-protein functioning from its classic pathway (adenylyl cyclase activation through Gs, in the case of βRs) to a ERK-only pathway (Hauger et al., 2009). This process prevents short-term action of βRs (Gs-induced activation of adenylyl cyclase) but potentially triggers longer-term mechanism like mitogen activated protein kinase/extracellular signal-regulated kinase (MAPK/ERK), synaptogenesis, and, possibly, maladaptive synaptic plasticity.
LC-CNS Interactions
As discussed earlier, the activation of the PFC-LC-PFC axis is critically important in the stress response (Itoi and Sugimoto, 2010). The extent of the involvement of the LC/central adrenergic system in the coordination of organism sensory input, decision-making, and motor execution suggests that the LC/NE system plays a critical role in the coordination of all stages of the spatio-temporal pattern of brain activation from quiet wake to periods of intense metabolic demand/stress. At the high end of metabolic demand, detrimental consequences of stress-evoked release of NE may derive from multiple factors, including the simultaneous abnormal release of cytokines—particularly interleukin 6 (IL-6; Li et al., 2015)—which by itself may lead to a wide array of psychiatric consequences from depression to psychosis and anxiety disorders (Atzori et al., 2012). Not surprisingly, an increase in the adrenergic (as well as dopaminergic) tone is an essential component of drug-induced “high” (Weinshenker and Schroeder, 2007; Sofuoglu and Sewell, 2009; Fitzgerald, 2013), similar to catecholamine hyper-function during psychotic episodes (Fitzgerald, 2014). An altered sensitivity of adrenergic receptors, or their abnormal function, may thus be a factor shared by a variety of stress-related psychiatric diseases, including post-traumatic stress disorder (George et al., 2013), generalized anxiety (Goddard et al., 2010), fibromyalgia (Clauw, 2014), as well as attention-deficit disorder (Chandler, 2015; Sterley et al., 2016).
We speculate that in a similar fashion, other areas showing differential adrenergic modulation are the motor cortex/cerebellum/striatum complex—responsible for commencing, coordinating, and carrying out rehearsed motor routines and impulsive behavior—as well as the heterogeneous group of brain areas labeled as Limbic System, which generate a variety of positive, negative, and mixed emotional states. The presence of strong anatomical projections from corticotropin-releasing hormone (CRH)-producing limbic areas to the LC is consistent with the hypothesis that negative mood may be a strong trigger for LC activity increase, at least in a physiological functioning brain.
We previously defined as stress any circumstance that raises the energy demand above an expected or biologically bearable threshold. Keeping in mind this idea, the steady-state energetic need of different fully-activated cortical areas varies greatly. For instance, limbic areas appear to be active even during sleep (default network; Buckner et al., 2008), with minimal energy demand. Purely sensory tasks, accompanied by sensory cortex activation, are likely to be the next least energetically demanding areas, as they are endowed with inbuilt circuitry for passive activity during the wake state. On the contrary, effective motor activity requires a combination of intention and sensory-motor coordination, which sets the motor circuitry to a relatively high-energy-demanding position. The highest energy need is requested by the prefrontal cortex, whose “working memory” juggles between multiple tasks including attention, planning future actions based on the retrieval of behavioral rules and sensory information stored earlier, and inhibition of momentary impulse. In addition, a high tone of limbic areas may drive high energy consumption from the PFC, to which is anatomically and functionally bi-directionally connected. It is likely, then, that stress affects to different extents brain areas with different stress-imposed additional energy requirement. Cortical areas in Figure 5 are numbered (1–4) in order of increasing energy need (anticlockwise), starting from limbic areas (1, lower right), sensory areas (2, upper right), motor areas (3, upper left), up to the prefrontal cortex (4, lower left).
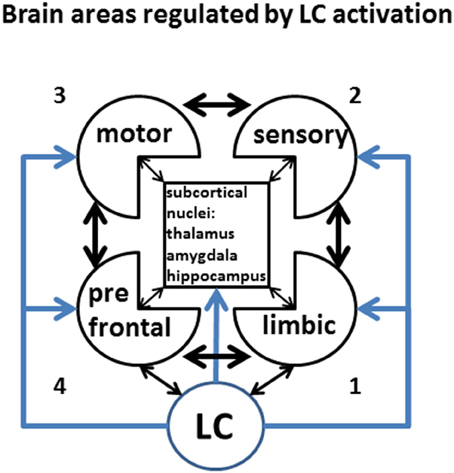
Figure 5. Brain areas regulated by LC activation. LC activity controls in a centralized fashion the level of activity and functional connectivity among of virtually all brain area. Keeping in mind that the effects of LC might have regionally specific effects, for the purpose of this discussion we will only consider differential LC effects onto prefrontal, motor, sensory, and limbic cortices, and lump together the activity subcortical nuclei. Different levels of activity are indicated by increasing color intensity, while the strength of inter-regional connectivity will be represented by the thickness of the arrows. This figure represents the legend for the Figures 6–8. The number (1–4) on the side of each sketch represents the putative resting energy demand of each activated state, from the least-demanding (LA) to the most demanding (PFC).
LC-NE Induced Activation States
Good evidence exists for gap-junction mediated synchronous LC activation (Ishimatsu and Williams, 1996; Rash et al., 2007). Computational modeling supports the hypothesis of simultaneous activation of LC neurons, and simultaneous increase in brain NE (Gao and Holmes, 2007; Patel and Joshi, 2015), although alternative hypothesis have been proposed (Chandler et al., 2014a,b; Chandler, 2015). These observations suggest that different brain states may be elicited by increasing NE concentrations progressively activating ARs from high to low affinity for NE. In each of these states a different combination of tonic and phasic NE levels would give rise to regional differences in the brain activity, as well as to specific patterns of global function. In this section we will describe a largely speculative proposal for a sequence of cortical states in the order of progressively higher energy demand (Constantinople and Bruno, 2011), higher CNS NE levels, and progressive binding to the sequence of adrenoceptors α2R, α1R, and βRs in the order of affinity from the highest to the lowest (Figure 6). The intensity of gray indicates the level of physiological (non-pathological) activation, the thickness of the arrows indicates the strength of the connectivity between areas.
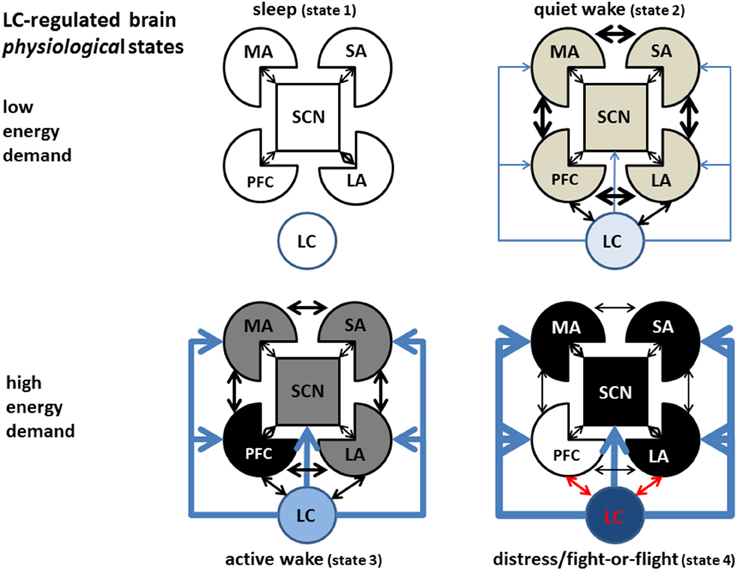
Figure 6. LC-regulated brain activation states. Refer to Figure 5 as legend for the representation of different brain areas. While many intermediate states are likely to exist, we depict in the sketch only four of them, in order of energy demand. During the sleep state (upper left) the LC is inactive, all cortices (except possibly limbic cortices) are virtually inactive, maintenance processes (like memory consolidation and basal immune activity) are on-going, while cellular energy content is restored. During quiet wake(upper right), LC is moderately active in the tonic mode, maximizing phasic release of NE which allows optimal intracortical communication and flexible behavioral and decision-making strategies and memory and learning associated with high phasic LC activation and βR-mediated activation. During high-energy demand (stress, lower left), an increased drive in the limbic cortex induces higher LC activation and hyperactivity in other cortical areas relevant to the specific stressor (most often the PFC, but on occasion could be other areas like motor or sensory cortices could be over-activated to carry out specific behavioral tasks). Extreme stress induces hyperactivity in parts of the limbic system, fight- or-flight response (lower right), overdrive and functional shut-down of the PFC, and hyper-activation of motor areas and subcortical nuclei (symbolized by the grid lines, MA: motor areas, SA sensory areas, SCN: subcortical nuclei, PFC: prefrontal cortex, LA: limbic areas, LC: locus ceruleus).
Sleep is the lowest-energy state, corresponding to a virtual absence of adrenergic tone. The whole organism—particularly the CNS—refills metabolic energy stores depleted during the previous wake phases. During this metabolic stage the organism is able to carry out important low-energy demand functions which do not require behavioral performance, like maintenance immune functions, rehearsal of mnemonic segments (Foster and Wilson, 2006) or motor sequence silent replay (Barnes and Wilson, 2014), aimed to synaptic stabilization and episodic or procedural memory consolidation.
The second state in the energy-demand ladder (quiet wake) is associated to low LC tonic firing, an active reward system, and mostly positive emotion in limbic areas. In this circumstance, the VTA releases dopamine that stabilizes the motivation axis represented by LC-Nucleus Accumbens-PFC receiving further input from the limbic cortices, and producing an optimal balance in the activity and reciprocal interaction among cortical areas and between cortical and subcortical regions. This state is characterized by optimal and flexible exchange of information between cortical areas and a relatively low physical and mental energetic load. In terms of LC activation/NE release, is associated with low but not nihil LC tonic firing and a high dynamic range for phasic LC responses to novel or salient stimuli, while, in terms of adrenoceptor activation, corresponds to tonic activation of α2 adrenoceptors, sporadic activation of α1Rs, and memory-promoting activation of βRs during phasic LC activation.
Tonic NE concentrations in the α1R activation range would promote a third condition (active wake) represented by a series of states characterized by selective activity-dependent enhancement of energy consumption in particular cortical areas. Such areas would be selected depending on the specific demands of the circumstance, driven by a relatively high emotional tone in limbic areas. For instance, during strenuous physical activity, strong sensory engagement, or critical behavioral planning, NE released from LC would selectively depress the activity in non-critical cortical areas in an α1R-dependent fashion, through depression of glutamatergic synapses (Dinh et al., 2009; Roychowdhury et al., 2014). At the same time, NE release would enhance energy consumption in critical cortical area(s) (i.e., motor controlling, sensory areas, working memory, or other brain areas) in an activity-dependent fashion, through a combination of phasically activated α1- and β-AR activation. This state would be associated with a relatively high tonic level of LC firing, a limited range of phasic LC responses to salient or new stimuli, and a decreased but still functional communication between different cortical areas. In the “active wake” state, an in-built circuitry, prepared by evolution for automatic processing, would promote strong but not overwhelming activity in sensory areas, motor areas, as well as in the PFC. The latter would elaborate variable strategies to resolve the specific demands of the contingency for which automatic processing is not effective. In social mammals, extinguishing a stressor may require an additional inter-individual interaction (social) component that overburdens the limbic system and is therefore especially vexing on the individual.
At the physiological highest level of energy consumption, the LC would display the strongest tonic activation, high tonic NE release, and a limited or inexistent range of phasic NE release, corresponding to the “fight-or-flight” (FoF) response described in the pioneering work of Cannon reviewed in Fee and Brown (2002), at the extreme of which could lay the berserk condition. This state is characterized by strong activation of cortical βARs, strong negative or positive emotion, impulsive response, deficient sensory activity, shut-down of the planning (PFC) areas, and scant or inefficient intra-cortical communication (Holmes and Wellman, 2009). This condition would be terminated with either of two outcomes: (1) the elimination of the stressor, resetting of LC activity to low tonic state, reactivation of a temporarily inhibited dopaminergic system, and return of the system to a low-energy state (sleep or idle wake), or, on the opposite end (2) failure to eliminate the stressor, depletion of organic energy reserve, and, possibly onset of long-term deficit or even death. In humans, this condition may give rise to neuropsychiatric disorder including epilepsy, burn-out syndrome, psychosis, depression, or anxiety, depending on the stressor pattern, individual genetic predisposition, and previous life history.
The maximum duration and intensity of high-energy states (active wake and distress/FoF) bearable by a specific individual would display significant inter-individual differences related to genetics, previous training/experience, and motivational state, and strongly depends on the history of the subject, to the point that periodic and controlled incursions into the stress state may be beneficial to increase the probability of successfully extinguishing unexpected future stressors. The hypothesis is graphically summarized by the sketches in Figures 5, 6.
An oft found bell-shaped dependence of specific PFC-dependent performance on adrenergic activation (Roychowdhury et al., 2012; Sapolsky, 2015) could be interpreted as a transition from a low-energy state (state 2: quiet wake), to state 3 (active wake), associated with a larger energy mobilization, stronger engagement, and improved cognitive performance (left part of the bell shape curve). At the right end of the curve would lay the transition between state 3 (stress) and state 4 (FoF, right part of the bell shape), with a massive engagement of βRs in the cortex as well as in subcortical nuclei—particularly the amygdala—and consequent dysfunctional working memory, in favor of an optimal impulsive, automatic, motor response and full-fledged autonomic sympathetic response (Bouret and Sara, 2005; Hains and Arnsten, 2008; Gamo and Arnsten, 2011). Needless to say, a comprehensive theory of energy mobilization in high-demanding states (active wake and distress/FoF) should include the role of other global transmitters, particularly acetylcholine, histamine, and 5HT. Such discussion is left for important future work, and falls outside the scope of this review.
It is tempting to speculate further that the regional pattern of energy consumption in this condition may have changed in the course of mammalian evolution, and possibly along mankind history, such that the effects of stress on motor and sensory cortices used to be a lot more severe during early history/evolution/developmental stages, compared with the effects on the PFC, while the latter has become (is becoming) the major subject—and potential victim—of stress in modern society, particularly for adolescent and adult humans.
Clinical Consequences of Stress-Induced Maladaptive Plasticity
Stress notoriously impairs the dynamic balance between sympathetic and parasympathetic autonomic branches, affecting sleep, digestion, endocrine function, by altering the balance between peripheral parasympathetic and sympathetic tones (Grippo and Johnson, 2009; Silvani et al., 2016). Even more consequential, in the CNS, physiological stress of high intensity and/or prolonged duration may lead to β arrestin-mediated internalization of adrenergic receptors, studied in detail for βRs (Stone and Quartermain, 1999), leading in turn to a de-sensitization of βR-mediated central adrenergic pathways (Fu and Xiang, 2015). A possible consequence of intense or prolonged stress could be a decrease in effectiveness of the LC adrenergic system, leading to a decrease in the expression of the β1R type (Porterfield et al., 2012) in limbic areas, and to a change in the expression of βR-related effectors in other parts of the limbic system like the hippocampus (Benes et al., 2004).
We represented the two poles of LC/NE function with two examples each, in Figure 7 (LC hypofunction) and Figure 8 (LC hyperfunction). In these figures, red and yellow represent pathologically hyper- or hypo-active areas, respectively. The intensity of the blue stripes inside LC sketch (light in Figure 7 and strong in Figure 8) represents the level of tonic LC activation. As example of LC hypofunction we represented attention deficit disorder with hyperactivity (ADHD), in which a monoaminergic hypofunction yields a hypofunctional PFC, which in turn fails to exert a satisfactory inhibitory control on automatic motor activity, associated with sensory distractibility (Figure 7, left). While other monoaminergic deficits (principally dopaminergic) are most likely also involved in ADHD, the efficacy of the NE-reuptake inhibitors like atomoxetine in ADHD treatment corroborates the notion that an LC/NE deficit is a critical component of this condition.
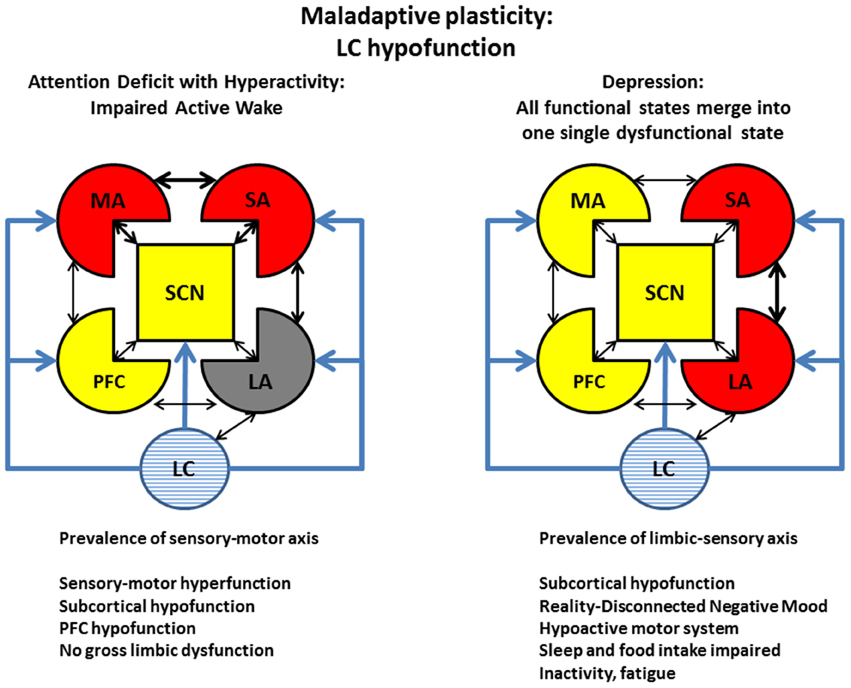
Figure 7. Maladaptive plasticity: Examples of LC hypofunction. Left: ADHD. Attention deficit disorder with hyperactivity (ADHD) is treated clinically with pro-monoaminergic drugs, particularly with NE re-uptake blockers. This condition may represent a dysfunction of the active wake (Figure 6) caused by NE/LC hypofunction. The condition is characterized by a prevalence of a motor-sensory areas and a decrease of working memory and inhibitory control. The deficit should not be considered a severe impairment insofar it is not associated with major alteration of limbic function. Right: Depression. The use of NE- (along with 5HT-) reuptake blockers is also in the mainstream treatment for depression. While depressed patients also display similar traits of ADHD subjects, like impaired working memory and low threshold for sensory activation, contrary to ADHD, depression is associated with long-term impairment of limbic function. According to our model, in depression, the normal physiological cycling between the 4 states illustrated in Figure 5 is turned into a single dysfunctional state. Refer to Figure 5 as legend for the representation of different brain areas. Captions as in Figure 6.
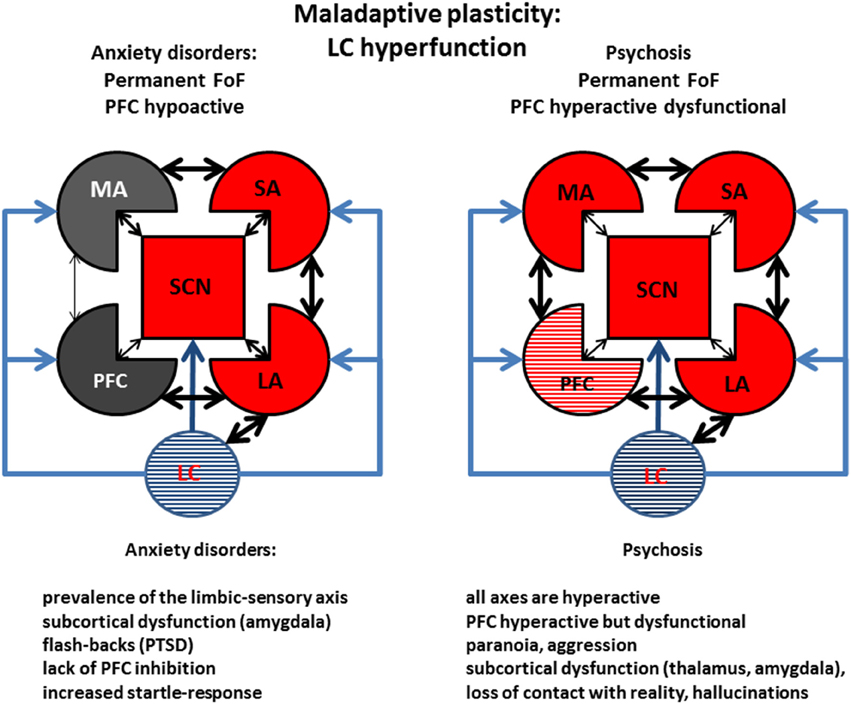
Figure 8. Maladaptive plasticity: Examples of LC hyperfunction. Left: Anxiety. Prolonged or intense stress may deplete organismic energy stores, possibly along with α1R overexpression, and βR β arrestin-induced internalization, leading to sensitization of the limbic areas (limbic cortices and amygdala) and of sensory areas. This condition would simulate a permanent reality-detached state of fight-or-flight. Right: Psychosis. Failure to eliminate a stress can turn an anxious condition into psychosis, by furthering the impairment of PFC function, possibly accompanied with aggression. Stress and stimulants may precipitate this condition by increasing monoaminergic—particularly dopaminergic and noradrenergic tone—in the PFC, where catecholamine transporter is responsible for the re-uptake of both molecules. Depression would differ from psychosis mainly in monoaminergic function (decreased in depression but increased in psychosis), causing an exaggerated motor response, but would share with it working memory impairment and sensory and limbic sensitization (compare with Figure 7, captions as in Figure 6).
While the causes and mechanisms of clinical depression involve factors other than the LC/NE system, a similar pharmacological argument—the efficacy of selective serotonin/norepinephrine reuptake inhibitors (SNRIs)—also indicates that a deficient noradrenergic system plays a critical role in the treatment of this affliction. In our hypothesis, depression—like ADHD—is also associated with a LC/NE and PFC deficit, but, compared to ADHD, is associated with opposite roles of limbic (hyperactive in depression) and motor (hypoactive in depression) areas (Figure 7, right).
As examples of conditions associated with long-term consequences (maladaptive plasticity) of LC hyper-function we selected anxiety disorder (Figure 8, left) and psychosis (Figure 8, right). Anxiety disorders are characterized by hyperactivation of the limbic-sensory axis, with a prevalence of a reality-detached negative mood. Different anxiety disorders may be associated with different degrees of motor activation, ranging from aggression (like in post-traumatic stress disorder), to freezing (like in a rodent response to a predator). Remarkably, anxiety and depression would only differ in terms of motor areas (in)activation, suggesting that further maladaptive plasticity may quickly convert an anxious state into a full-fledged depression, and that the same subject may oscillate between two conditions, which could even take place simultaneously. The large co-morbidity of anxiety and depression (van Tol et al., 2010) corroborates our hypothesis.
An example of even more severe mental condition associated with LC hyperfunction is psychosis (Figure 8, right). This condition is associated with hyperfunction of most cortical areas, promoted by a high monoaminergic tone, leading, in turn, to severe PFC functional impairment. Signs of generalized cortical hyper-function are pathognomonic symptoms of psychosis, like paranoia and hallucinations, as well as aggression. In this condition, prolonged and/or intense stress elicits a type of maladaptive plasticity that sensitizes limbic and sensory areas leading to loss of touch with reality, and—in the most dramatic cases—aggression and gross working memory impairment. Clinical support for a strong involvement of the LC/NE system—together with other monoaminergic systems—in psychoses, is the precipitation of psychotic episodes after intake of drugs including legal or illegal NE and other monoaminergic re-uptake blockers. Up-regulation of α1Rs may be a component of PFC impairment observed in the ventral-hippocampal lesion model of schizophrenia (Al-Khairi et al., 2009).
Depression and psychosis share the traits of working memory impairment, some level of detachment from reality, and hypersensitivity of limbic and sensory system, all of which can be triggered by prolonged or intense stress. Clinically, these two conditions may thus represent the result of a parallel process of stress-induced maladaptive plasticity landing on opposite poles of motor drive because of genetics or cultural factor. The similarities between depression and psychosis may explain the presence of both conditions (plus bipolar disease) associated with genes including DISC1 and neuregulins (Blackwood et al., 2007). This hypothesis is further supported by the finding of a reduced monoaminergic drive in a DISC1 animal model displaying depressive symptoms (Lipina et al., 2013).
Conclusions
Many unanswered questions remain about the role of the LC/NE system. While the presence of gap junctions within the LC has been suggested by anatomical (Rash et al., 2007) and functional (Ishimatsu and Williams, 1996) studies, to our knowledge, synchronous and proportional release of NE in different brain areas following LC activation has not yet been shown unambiguously. The question about simultaneous increase in NE concentration in different brain regions might be answered with precise—in time and space—measurement of monoamine levels, possibly with future developments of already existing electrochemical and microdialysis techniques.
Understanding the nature and extent of the interference between the noradrenergic system and other alertness- and attention-related modulator systems, notably, the serotoninergic, the histaminergic, and the cholinergic systems, and the possible specific role of each neurotransmitter in the global coordination of brain activity is also of critical importance. The presence of reciprocal presynaptic hetero-receptors between neurotransmitters pairs (including GABA and glutamate) may offer important and relatively unexplored mechanism of interaction between different modulatory systems. Hopefully, quantitative modeling will be able to pinpoint a precise correlation between global states induced by NE (and other modulators) and behavioral states.
Computational models reproducing experimental results (Gao and Holmes, 2007; Patel and Joshi, 2015), particularly on the roles of adrenoceptors in behavioral tasks (Chandler et al., 2014b; Chandler, 2015; Somkuwar et al., 2015) are starting to reach a remarkable level of sophistication, and will undoubtedly contribute to integrate the large amount of experimental results collected along many decades on the adrenergic effects on the modulation of intrinsic neuronal conductances and long- and short-term plasticity. Possibly the most important related issue concerns the specific mechanism through which distress elicits maladaptive plasticity, turning a number of physiologically connected, functional, brain areas into a series of dysfunctional circuits as seen in psychiatric disease.
An important and largely overlooked adrenergic mechanisms emerged in the last decade is the role of NE receptors in astrocyte and microglia modulation (O'Donnell et al., 2012; Pankratov and Lalo, 2015). Further, studies will be necessary to integrate the relationships among neuronal function, glial function, and noradrenergic activity.
Author Contributions
MA wrote the article, RC, EE, FG, RS, NS, MM, MT, JP, and HS contributed by designing part of the manuscript structure, with intellectual contributions prior to the manuscript layout, and by writing parts of the manuscript, and revising its full extent. All authors have read, discussed, and accepted the final version of the manuscript.
Conflict of Interest Statement
The authors declare that the research was conducted in the absence of any commercial or financial relationships that could be construed as a potential conflict of interest.
Acknowledgments
This work has been conducted in part with funds from CONACyT, CB-2013-01 221653 to MA.
References
Abraham, P. A., Xing, G., Zhang, L., Yu, E. Z., Post, R., Gamble, E. H., et al. (2008). beta1- and beta2-adrenoceptor induced synaptic facilitation in rat basolateral amygdala. Brain Res. 1209, 65–73. doi: 10.1016/j.brainres.2008.02.082
Agnati, L. F., Zoli, M., Strömberg, I., and Fuxe, K. (1995). Intercellular communication in the brain: wiring versus volume transmission. Neuroscience 69, 711–726. doi: 10.1016/0306-4522(95)00308-6
Al-Khairi, I., Baharnoori, M., Kamath, A., Bhardwaj, S. K., and Srivastava, L. K. (2009). Altered expression and alpha-1 adrenergic receptor mediated activity of protein kinase C in the prefrontal cortex of rats with neonatal ventral hippocampus lesions. Synapse 63, 1051–1059. doi: 10.1002/syn.20691
Alves, F. H. F., Crestani, C. C., Resstel, L. B. M., and Corrêa, F. M. A. (2014). Both α1- and α2-adrenoceptors in the insular cortex are involved in the cardiovascular responses to acute restraint stress in rats. PLoS ONE 9:e83900. doi: 10.1371/journal.pone.0083900
Amos, T., Stein, D. J., and Ipser, J. C. (2014). Pharmacological interventions for preventing post-traumatic stress disorder (PTSD). Cochrane Database Syst. Rev. 7:CD006239. doi: 10.1002/14651858.cd006239.pub2
Aono, Y., Taguchi, H., Saigusa, T., Uchida, T., Takada, K., Takiguchi, H., et al. (2015). Simultaneous activation of the α1A-, α1B- and α1D-adrenoceptor subtypes in the nucleus accumbens reduces accumbal dopamine efflux in freely moving rats. Behav. Pharmacol. 26, 73–80. doi: 10.1097/FBP.0000000000000113
Arnsten, A. F., and Li, B. M. (2005). Neurobiology of executive functions: catecholamine influences on prefrontal cortical functions. Biol. Psychiatry 57, 1377–1384. doi: 10.1016/j.biopsych.2004.08.019
Arnsten, A. F. T. (2011). catecholamine influences on dorsolateral prefrontal cortical networks. Biol. Psychiatry 69, e89–e99. doi: 10.1016/j.biopsych.2011.01.027
Arnsten, A. F. T., and Jin, L. E. (2012). Guanfacine for the treatment of cognitive disorders: a century of discoveries at Yale. Yale J. Biol. Med. 85, 45–58.
Arnsten, A. F. T., and Jin, L. E. (2014). Molecular influences on working memory circuits in dorsolateral prefrontal cortex. Prog. Mol. Biol. Transl. Sci. 122, 211–231. doi: 10.1016/B978-0-12-420170-5.00008-8
Arnsten, A. F. T., Wang, M. J., and Paspalas, C. D. (2012). Neuromodulation of thought: flexibilities and vulnerabilities in prefrontal cortical network synapses. Neuron 76, 223–239. doi: 10.1016/j.neuron.2012.08.038
Aston-Jones, G., and Cohen, J. D. (2005). An integrative theory of locus coeruleus-norepinephrine function: adaptive gain and optimal performance. Annu. Rev. Neurosci. 28, 403–450. doi: 10.1146/annurev.neuro.28.061604.135709
Aston-Jones, G., Rajkowski, J., Ivanova, S., Usher, M., and Cohen, J. (1998). Neuromodulation and cognitive performance: recent studies of noradrenergic locus ceruleus neurons in behaving monkeys. Adv. Pharmacol. 42, 755–759. doi: 10.1016/S1054-3589(08)60857-1
Aston-Jones, G., and Waterhouse, B. (2016). Locus Coeruleus: from global projection system to adaptive regulation of behavior. Brain Res. 1645, 75–78. doi: 10.1016/j.brainres.2016.03.001
Atzori, M., Garcia-Oscos, F., and Mendez, J. A. (2012). Role of IL-6 in the etiology of hyperexcitable neuropsychiatric conditions: experimental evidence and therapeutic implications. Future Med. Chem. 4, 2177–2192. doi: 10.4155/fmc.12.156
Bari, A., and Robbins, T. W. (2013). Noradrenergic versus dopaminergic modulation of impulsivity, attention and monitoring behaviour in rats performing the stop-signal task: possible relevance to ADHD. Psychopharmacol. 230, 89–111. doi: 10.1007/s00213-013-3141-6
Barnes, D. C., and Wilson, D. A. (2014). Slow-wave sleep-imposed replay modulates both strength and precision of memory. J. Neurosci. 34, 5134–5142. doi: 10.1523/JNEUROSCI.5274-13.2014
Barrot, M., Yalcin, I., Choucair-Jaafar, N., Benbouzid, M., and Freund-Mercier, M.-J. (2009). From antidepressant drugs to beta-mimetics: preclinical insights on potential new treatments for neuropathic pain. Recent Pat. CNS Drug Discov. 4, 182–189. doi: 10.2174/157488909789104794
Bencsics, A., Elenkov, I. J., and Vizi, E. S. (1995). alpha 2-, alpha 2A-, alpha 2B/2C-Adrenoceptor subtype antagonists prevent lipopolysaccharide-induced fever response in rabbits. Brain Res. 705, 302–306. doi: 10.1016/0006-8993(95)01154-4
Benes, F. M., Burke, R. E., Walsh, J., Berretta, S., Matzilevich, D., Minns, M., et al. (2004). Acute amygdalar activation induces an upregulation of multiple monoamine G protein coupled pathways in rat hippocampus. Mol. Psychiatry 9, 932–945. doi: 10.1038/sj.mp.4001524
Berridge, C. W., and Waterhouse, B. D. (2003). The locus coeruleus-noradrenergic system: modulation of behavioral state and state-dependent cognitive processes. Brain Res. Brain Res. Rev. 42, 33–84. doi: 10.1016/S0165-0173(03)00143-7
Blackwood, D. H. R., Pickard, B. J., Thomson, P. A., Evans, K. L., Porteous, D. J., and Muir, W. J. (2007). Are some genetic risk factors common to schizophrenia, bipolar disorder and depression? Evidence from DISC1, GRIK4 and NRG1. Neurotox. Res. 11, 73–83. doi: 10.1007/BF03033484
Bondi, C. O., Barrera, G., Lapiz, M. D. S., Bedard, T., Mahan, A., and Morilak, D. A. (2007). Noradrenergic facilitation of shock-probe defensive burying in lateral septum of rats, and modulation by chronic treatment with desipramine. Prog. Neuropsychopharmacol. Biol. Psychiatry 31, 482–495. doi: 10.1016/j.pnpbp.2006.11.015
Bouret, S., and Sara, S. J. (2005). Network reset: a simplified overarching theory of locus coeruleus noradrenaline function. Trends Neurosci. 28, 574–582. doi: 10.1016/j.tins.2005.09.002
Brennan, A. R., and Arnsten, A. F. (2008). Neuronal mechanisms underlying attention deficit hyperactivity disorder: the influence of arousal on prefrontal cortical function. Ann. N.Y. Acad. Sci. 1129, 236–245. doi: 10.1196/annals.1417.007
Brown, D. C., Co, M. S., Wolff, R. C., and Atzori, M. (2012). alpha-Adrenergic receptors in auditory cue detection: alpha(2) receptor blockade suppresses false alarm responding in the rat. Neuropharmacology 62, 2178–2183. doi: 10.1016/j.neuropharm.2011.12.024
Buckner, R. L., Andrews-Hanna, J. R., and Schacter, D. L. (2008). The brain's default network: anatomy, function, and relevance to disease. Ann. N.Y. Acad. Sci. 1124, 1–38. doi: 10.1196/annals.1440.011
Carey, M. R., and Regehr, W. G. (2009). Noradrenergic control of associative synaptic plasticity by selective modulation of instructive signals. Neuron 62, 112–122. doi: 10.1016/j.neuron.2009.02.022
Carr, D. B., Andrews, G. D., Glen, W. B., and Lavin, A. (2007). alpha2-Noradrenergic receptors activation enhances excitability and synaptic integration in rat prefrontal cortex pyramidal neurons via inhibition of HCN currents. J. Physiol. (Lond). 584, 437–450. doi: 10.1113/jphysiol.2007.141671
Carter, M. E., Brill, J., Bonnavion, P., Huguenard, J. R., Huerta, R., and de Lecea, L. (2012). Mechanism for Hypocretin-mediated sleep-to-wake transitions. Proc. Natl. Acad. Sci. U.S.A. 109, E2635–E2644. doi: 10.1073/pnas.1202526109
Cathala, L., Guyon, A., Eugene, D., and Paupardin-Tritsch, D. (2002). Alpha2-adrenoceptor activation increases a cationic conductance and spontaneous GABAergic synaptic activity in dopaminergic neurones of the rat substantia nigra. Neuroscience 115, 1059–1065. doi: 10.1016/S0306-4522(02)00542-0
Cathel, A. M., Reyes, B. A. S., Wang, Q., Palma, J., Mackie, K., Van Bockstaele, E. J., et al. (2014). Cannabinoid modulation of alpha2 adrenergic receptor function in rodent medial prefrontal cortex. Eur. J. Neurosci. 40, 3202–3214. doi: 10.1111/ejn.12690
Chandler, D. J. (2015). Evidence for a specialized role of the locus coeruleus noradrenergic system in cortical circuitries and behavioral operations. Brain Res. 1641, 197–206. doi: 10.1016/j.brainres.2015.11.022
Chandler, D. J., Gao, W.-J., and Waterhouse, B. D. (2014a). Heterogeneous organization of the locus coeruleus projections to prefrontal and motor cortices. Proc. Natl. Acad. Sci. U.S.A. 111, 6816–6821. doi: 10.1073/pnas.1320827111
Chandler, D. J., Waterhouse, B. D., and Gao, W.-J. (2014b). New perspectives on catecholaminergic regulation of executive circuits: evidence for independent modulation of prefrontal functions by midbrain dopaminergic and noradrenergic neurons. Front. Neural Circuits 8:53. doi: 10.3389/fncir.2014.00053
Chang, C., and Grace, A. A. (2013). Amygdala β-noradrenergic receptors modulate delayed downregulation of dopamine activity following restraint. J. Neurosci. 33, 1441–1450. doi: 10.1523/JNEUROSCI.2420-12.2013
Charney, D. S., Price, L. H., and Heninger, G. R. (1986). Desipramine-yohimbine combination treatment of refractory depression. Implications for the beta-adrenergic receptor hypothesis of antidepressant action. Arch. Gen. Psychiatry 43, 1155–1161. doi: 10.1001/archpsyc.1986.01800120041009
Chmielarz, P., Kuśmierczyk, J., Parlato, R., Schütz, G., Nalepa, I., and Kreiner, G. (2013). Inactivation of glucocorticoid receptor in noradrenergic system influences anxiety- and depressive-like behavior in mice. PLoS ONE 8:e72632. doi: 10.1371/journal.pone.0072632
Chong, W., Li, L. H., Lee, K., Lee, M. H., Park, J. B., and Ryu, P. D. (2004). Subtypes of alpha1- and alpha2-adrenoceptors mediating noradrenergic modulation of spontaneous inhibitory postsynaptic currents in the hypothalamic paraventricular nucleus. J. Neuroendocrinol. 16, 450–457. doi: 10.1111/j.1365-2826.2004.01180.x
Cilz, N. I., Kurada, L., Hu, B., and Lei, S. (2014). Dopaminergic modulation of GABAergic transmission in the entorhinal cortex: concerted roles of α1 adrenoreceptors, inward rectifier K+, and T-type Ca2+ channels. Cereb. Cortex 24, 3195–3208. doi: 10.1093/cercor/bht177
Clauw, D. J. (2014). Fibromyalgia: a clinical review. JAMA 311, 1547–1555. doi: 10.1001/jama.2014.3266
Coccurello, R., Bielawski, A., Zelek-Molik, A., Vetulani, J., Kowalska, M., D'Amato, F. R., et al. (2014). Brief maternal separation affects brain α1-adrenoceptors and apoptotic signaling in adult mice. Prog. Neuropsychopharmacol. Biol. Psychiatry 48, 161–169. doi: 10.1016/j.pnpbp.2013.10.004
Collette, K. M., Zhou, X. D., Amoth, H. M., Lyons, M. J., Papay, R. S., Sens, D. A., et al. (2014). Long-term α1B-adrenergic receptor activation shortens lifespan, while α1A-adrenergic receptor stimulation prolongs lifespan in association with decreased cancer incidence. Age 36, 9675. doi: 10.1007/s11357-014-9675-7
Constantinople, C. M., and Bruno, R. M. (2011). Effects and mechanisms of wakefulness on local cortical networks. Neuron 69, 1061–1068. doi: 10.1016/j.neuron.2011.02.040
Conversi, D., Cruciani, F., Accoto, A., and Cabib, S. (2014). Positive emotional arousal increases duration of memory traces: different role of dopamine D1 receptor and β-adrenoceptor activation. Pharmacol. Biochem. Behav. 122, 158–163. doi: 10.1016/j.pbb.2014.04.001
Cottingham, C., Ferryman, C. J., and Wang, Q. (2015). α2 Adrenergic receptor trafficking as a therapeutic target in antidepressant drug action. Prog. Mol. Biol. Transl. Sci. 132, 207–225. doi: 10.1016/bs.pmbts.2015.03.007
Cox, D. J., Racca, C., and LeBeau, F. E. N. (2008). Beta-adrenergic receptors are differentially expressed in distinct interneuron subtypes in the rat hippocampus. J. Comp. Neurol. 509, 551–565. doi: 10.1002/cne.21758
Dang, V., Medina, B., Das, D., Moghadam, S., Martin, K. J., Lin, B., et al. (2014). Formoterol, a long-acting β2 adrenergic agonist, improves cognitive function and promotes dendritic complexity in a mouse model of Down syndrome. Biol. Psychiatry 75, 179–188. doi: 10.1016/j.biopsych.2013.05.024
da Silva, E. S., Flores, R. A., Cella, E. C., Levone, B. R., Taschetto, A. P., Kochenborger, L., et al. (2014). Blockade of median raphe nucleus α1-adrenoceptor subtypes increases food intake in rats. Pharmacol. Biochem. Behav. 124, 350–355. doi: 10.1016/j.pbb.2014.06.010
Day, J. S., O'Neill, E., Cawley, C., Aretz, N. K., Kilroy, D., Gibney, S. M., et al. (2014). Noradrenaline acting on astrocytic β2-adrenoceptors induces neurite outgrowth in primary cortical neurons. Neuropharmacology 77, 234–248. doi: 10.1016/j.neuropharm.2013.09.027
De Kloet, E. R. (2004). Hormones and the stressed brain. Ann. N.Y. Acad. Sci. 1018, 1–15. doi: 10.1196/annals.1296.001
Deupree, J. D., Reed, A. L., and Bylund, D. B. (2007). Differential effects of the tricyclic antidepressant desipramine on the density of adrenergic receptors in juvenile and adult rats. J. Pharmacol. Exp. Ther. 321, 770–776. doi: 10.1124/jpet.106.118935
Devilbiss, D. M., and Waterhouse, B. D. (2000). Norepinephrine exhibits two distinct profiles of action on sensory cortical neuron responses to excitatory synaptic stimuli. Synapse 37, 273–282. doi: 10.1002/1098-2396(20000915)37:4<273::AID-SYN4>3.0.CO;2
DeWire, S. M., Ahn, S., Lefkowitz, R. J., and Shenoy, S. K. (2007). Beta-arrestins and cell signaling. Annu. Rev. Physiol. 69, 483–510. doi: 10.1146/annurev.physiol.69.022405.154749
Dienel, G. A., and Cruz, N. F. (2016). Aerobic glycolysis during brain activation: adrenergic regulation and influence of norepinephrine on astrocytic metabolism. J. Neurochem. 138, 14–52. doi: 10.1111/jnc.13630
Di Mauro, M., Li Volsi, G., and Licata, F. (2013). Noradrenergic control of neuronal firing in cerebellar nuclei: modulation of GABA responses. Cerebellum 12, 350–361. doi: 10.1007/s12311-012-0422-2
Ding, F., O'Donnell, J., Thrane, A. S., Zeppenfeld, D., Kang, H., Xie, L., et al. (2013). α1-Adrenergic receptors mediate coordinated Ca2+ signaling of cortical astrocytes in awake, behaving mice. Cell Calcium 54, 387–394. doi: 10.1016/j.ceca.2013.09.001
Dinh, L., Nguyen, T., Salgado, H., and Atzori, M. (2009). Norepinephrine Homogeneously inhibits alpha-amino-3-hydroxyl-5-methyl-4-isoxazole-propionate- (AMPAR-) mediated currents in all layers of the temporal cortex of the rat. Neurochem. Res. 34, 1896–1906. doi: 10.1007/s11064-009-9966-z
Doze, V. A., Papay, R. S., Goldenstein, B. L., Gupta, M. K., Collette, K. M., Nelson, B. W., et al. (2011). Long-term α1A-adrenergic receptor stimulation improves synaptic plasticity, cognitive function, mood, and longevity. Mol. Pharmacol. 80, 747–758. doi: 10.1124/mol.111.073734
Edeline, J. M. (2012). Beyond traditional approaches to understanding the functional role of neuromodulators in sensory cortices. Front. Behav. Neurosci. 6:45. doi: 10.3389/fnbeh.2012.00045
Egli, R. E., Kash, T. L., Choo, K., Savchenko, V., Matthews, R. T., Blakely, R. D., et al. (2005). Norepinephrine modulates glutamatergic transmission in the bed nucleus of the stria terminalis. Neuropsychopharmacology, 30, 657–668. doi: 10.1038/sj.npp.1300639
Fee, E., and Brown, T. (2002). Walter Bradford Cannon: pioneer physiologist of human emotions. Am. J. Public Health 92, 1594–1595. doi: 10.2105/AJPH.92.10.1594
Ferrero, J. J., Alvarez, A. M., Ramírez-Franco, J., Godino, M. C., Bartolomé-Martín, D., Aguado, C., et al. (2013). β-Adrenergic receptors activate exchange protein directly activated by cAMP (Epac), translocate Munc13-1, and enhance the Rab3A-RIM1α interaction to potentiate glutamate release at cerebrocortical nerve terminals. J. Biol. Chem. 288, 31370–31385. doi: 10.1074/jbc.M113.463877
Fitzgerald, P. J. (2013). Elevated Norepinephrine may be a unifying etiological factor in the abuse of a broad range of substances: alcohol, nicotine, marijuana, heroin, cocaine, and caffeine. Subst. Abuse 7, 171–183. doi: 10.4137/sart.s13019
Fitzgerald, P. J. (2014). Is elevated norepinephrine an etiological factor in some cases of schizophrenia? Psychiatry Res. 215, 497–504. doi: 10.1016/j.psychres.2014.01.011
Fitzgerald, P. J., Giustino, T. F., Seemann, J. R., and Maren, S. (2015). Noradrenergic blockade stabilizes prefrontal activity and enables fear extinction under stress. Proc. Natl. Acad. Sci. U.S.A. 112, E3729–E3737. doi: 10.1073/pnas.1500682112
Foster, D. J., and Wilson, M. A. (2006). Reverse replay of behavioural sequences in hippocampal place cells during the awake state. Nature 440, 680–683. doi: 10.1038/nature04587
Friedman, J. I., Stewart, D. G., and Gorman, J. M. (2004). Potential noradrenergic targets for cognitive enhancement in schizophrenia. CNS Spectr. 9, 350–355. doi: 10.1017/S1092852900009330
Fu, Q., and Xiang, Y. K. (2015). Trafficking of β-adrenergic receptors: Implications in intracellular receptor signaling. Prog. Mol. Biol. Transl. Sci. 132, 151–188. doi: 10.1016/bs.pmbts.2015.03.008
Fuxe, K., Dahlström, A. B., Jonsson, G., Marcellino, D., Guescini, M., Dam, M., et al. (2010). The discovery of central monoamine neurons gave volume transmission to the wired brain. Prog Neurobiol. 90, 82–100. doi: 10.1016/j.pneurobio.2009.10.012
Gainetdinov, R. R., Premont, R. T., Bohn, L. M., Lefkowitz, R. J., and Caron, M. G. (2004). Desensitization of G protein-coupled receptors and neuronal functions. Annu. Rev. Neurosci. 27, 107–144. doi: 10.1146/annurev.neuro.27.070203.144206
Gamo, N. J., and Arnsten, A. F. (2011). Molecular modulation of prefrontal cortex: rational development of treatments for psychiatric disorders. Behav. Neurosci. 125, 282–296. doi: 10.1037/a0023165
Gao, J., and Holmes, P. (2007). On the dynamics of electrically-coupled neurons with inhibitory synapses. J. Comput. Neurosci. 22, 39–61. doi: 10.1007/s10827-006-9676-3
George, S. A., Knox, D., Curtis, A. L., Aldridge, J. W., Valentino, R. J., and Liberzon, I. (2013). Altered locus coeruleus-norepinephrine function following single prolonged stress. Eur. J. Neurosci. 37, 901–909. doi: 10.1111/ejn.12095
Goddard, A. W., Ball, S. G., Martinez, J., Robinson, M. J., Yang, C. R., Russell, J. M., et al. (2010). Current perspectives of the roles of the central norepinephrine system in anxiety and depression. Depress. Anxiety 27, 339–350. doi: 10.1002/da.20642
Goertz, R. B., Wanat, M. J., Gomez, J. A., Brown, Z. J., Phillips, P. E. M., and Paladini, C. A. (2015). Cocaine increases dopaminergic neuron and motor activity via midbrain α1 adrenergic signaling. Neuropsychopharmacology 40, 1151–1162. doi: 10.1038/npp.2014.296
Goh, J. J., and Manahan-Vaughan, D. (2013). Hippocampal long-term depression in freely behaving mice requires the activation of beta-adrenergic receptors. Hippocampus 23, 1299–1308. doi: 10.1002/hipo.22168
Gold, P. W. (2015). The organization of the stress system and its dysregulation in depressive illness. Mol. Psychiatry 20, 32–47. doi: 10.1038/mp.2014.163
Gold, P. W., and Chrousos, G. P. (2002). Organization of the stress system and its dysregulation in melancholic and atypical depression: high vs low CRH/NE states. Mol. Psychiatry 7, 254–275. doi: 10.1038/sj.mp.4001032
Grigoryan, G., Ardi, Z., Albrecht, A., Richter-Levin, G., and Segal, M. (2015). Juvenile stress alters LTP in ventral hippocampal slices: involvement of noradrenergic mechanisms. Behav. Brain Res. 278, 559–562. doi: 10.1016/j.bbr.2014.09.047
Grigoryan, G., and Segal, M. (2013). Prenatal stress alters noradrenergic modulation of LTP in hippocampal slices. J. Neurophysiol. 110, 279–285. doi: 10.1152/jn.00834.2012
Grippo, A. J., and Johnson, A. K. (2009). Stress, depression and cardiovascular dysregulation: a review of neurobiological mechanisms and the integration of research from preclinical disease models. Stress 12, 1–21. doi: 10.1080/10253890802046281
Groch, S., Wilhelm, I., Diekelmann, S., Sayk, F., Gais, S., and Born, J. (2011). Contribution of norepinephrine to emotional memory consolidation during sleep. Psychoneuroendocrinology 36, 1342–1350. doi: 10.1016/j.psyneuen.2011.03.006
Grzanna, R. M. M. (1980). The locus coeruleus in the rat: an immunohistochemical delineation. Neuroscience 5, 21–40. doi: 10.1016/0306-4522(80)90068-8
Guijarro, A., Laviano, A., and Meguid, M. M. (2006). Hypothalamic integration of immune function and metabolism. Prog. Brain Res. 153, 367–405. doi: 10.1016/S0079-6123(06)53022-5
Gyoneva, S., and Traynelis, S. F. (2013). Norepinephrine modulates the motility of resting and activated microglia via different adrenergic receptors. J. Biol. Chem. 288, 15291–15302. doi: 10.1074/jbc.M113.458901
Haggerty, D. C., Glykos, V., Adams, N. E., and Lebeau, F. E. (2013). Bidirectional modulation of hippocampal gamma (20-80 Hz) frequency activity in vitro via alpha(alpha)- and beta(beta)-adrenergic receptors (AR). Neuroscience 253, 142–154. doi: 10.1016/j.neuroscience.2013.08.028
Hains, A. B., and Arnsten, A. F. (2008). Molecular mechanisms of stress-induced prefrontal cortical impairment: implications for mental illness. Learn. Mem. 15, 551–564. doi: 10.1101/lm.921708
Haj-Dahmane, S., and Shen, R.-Y. (2014). Chronic stress impairs α1-adrenoceptor-induced endocannabinoid-dependent synaptic plasticity in the dorsal raphe nucleus. J. Neurosci. 34, 14560–14570. doi: 10.1523/JNEUROSCI.1310-14.2014
Hansen, N., and Manahan-Vaughan, D. (2015). Locus coeruleus stimulation facilitates long-term depression in the dentate gyrus that requires activation of β-adrenergic receptors. Cereb. Cortex 25, 1889–1896. doi: 10.1093/cercor/bht429
Haroon, E., Raison, C. L., and Miller, A. H. (2012). Psychoneuroimmunology meets neuropsychopharmacology: translational implications of the impact of inflammation on behavior. Neuropsychopharmacology 37, 137–162. doi: 10.1038/npp.2011.205
Hauger, R. L., Risbrough, V., Oakley, R. H., Olivares-Reyes, J. A., and Dautzenberg, F. M. (2009). Role of CRF receptor signaling in stress vulnerability, anxiety, and depression. Ann. N.Y. Acad. Sci. 1179, 120–143. doi: 10.1111/j.1749-6632.2009.05011.x
Hecht, P. M., Will, M. J., Schachtman, T. R., Welby, L. M., and Beversdorf, D. Q. (2014). Beta-adrenergic antagonist effects on a novel cognitive flexibility task in rodents. Behav. Brain Res. 260, 148–154. doi: 10.1016/j.bbr.2013.11.041
Heisler, L. K., Cowley, M. A., Kishi, T., Tecott, L. H., Fan, W., Low, M. J., et al. (2003). Central serotonin and melanocortin pathways regulating energy homeostasis. Ann. N.Y. Acad. Sci. 994, 169–174. doi: 10.1111/j.1749-6632.2003.tb03177.x
Henny, P., Brischoux, F., Mainville, L., Stroh, T., and Jones, B. E. (2010). Immunohistochemical evidence for synaptic release of glutamate from orexin terminals in the locus coeruleus. Neuroscience 169, 1150–1157. doi: 10.1016/j.neuroscience.2010.06.003
Hirono, M., and Obata, K. (2006). Alpha-adrenoceptive dual modulation of inhibitory GABAergic inputs to Purkinje cells in the mouse cerebellum. J. Neurophysiol. 95, 700–708. doi: 10.1152/jn.00711.2005
Holmes, A., and Wellman, C. L. (2009). Stress-induced prefrontal reorganization and executive dysfunction in rodents. Neurosci. Biobehav. Rev. 33, 773–783. doi: 10.1016/j.neubiorev.2008.11.005
Hvoslef-Eide, M., Oomen, C. A., Fisher, B. M., Heath, C. J., Robbins, T. W., Saksida, L. M., et al. (2015). Facilitation of spatial working memory performance following intra-prefrontal cortical administration of the adrenergic alpha1 agonist phenylephrine. Psychopharmacology (Berl). 232, 4005–4016. doi: 10.1007/s00213-015-4038-3
Igata, S., Hayashi, T., Itoh, M., Akasu, T., Takano, M., and Ishimatsu, M. (2014). Persistent α1-adrenergic receptor function in the nucleus locus coeruleus causes hyperexcitability in AD/HD model rats. J. Neurophysiol. 111, 777–786. doi: 10.1152/jn.01103.2012
Inoue, W., Baimoukhametova, D. V., Füzesi, T., Wamsteeker Cusulin, J. I., Koblinger, K., Whelan, P. J., et al. (2013). Noradrenaline is a stress-associated metaplastic signal at GABA synapses. Nat. Neurosci. 16, 605–612. doi: 10.1038/nn.3373
Ishimatsu, M., and Williams, J. T. (1996). Synchronous activity in locus coeruleus results from dendritic interactions in pericoerulear regions. J. Neurosci. 16, 5196–5204.
Itoi, K., and Sugimoto, N. (2010). The brainstem noradrenergic systems in stress, anxiety and depression. J. Neuroendocrinol. 22, 355–361. doi: 10.1111/j.1365-2826.2010.01988.x
Ji, X. H., Ji, J. Z., Zhang, H., and Li, B. M. (2008). Stimulation of alpha2-adrenoceptors suppresses excitatory synaptic transmission in the medial prefrontal cortex of rat. Neuropsychopharmacol. Off. Publ. Am. Coll. Neuropsychopharmacol. 33, 2263–2271. doi: 10.1038/sj.npp.1301603
Jiang, L., Chen, S.-H., Chu, C.-H., Wang, S.-J., Oyarzabal, E., Wilson, B., et al. (2015). A novel role of microglial NADPH oxidase in mediating extra-synaptic function of norepinephrine in regulating brain immune homeostasis. Glia 63, 1057–1072. doi: 10.1002/glia.22801
Jiménez-Rivera, C. A., Figueroa, J., Vázquez-Torres, R., Vélez-Hernandez, M. E., Schwarz, D., Velásquez-Martinez, M. C., et al. (2012). Presynaptic inhibition of glutamate transmission by α2 receptors in the VTA. Eur. J. Neurosci. 35, 1406–1415. doi: 10.1111/j.1460-9568.2012.08029.x
Johnson, J. D., Zimomra, Z. R., and Stewart, L. T. (2013). Beta-adrenergic receptor activation primes microglia cytokine production. J. Neuroimmunol. 254, 161–164. doi: 10.1016/j.jneuroim.2012.08.007
Jones, B. E., and Yang, T. Z. (1985). The efferent projections from the reticular formation and the locus coeruleus studied by anterograde and retrograde axonal transport in the rat. J. Comp. Neurol. 242, 56–92. doi: 10.1002/cne.902420105
Kamath, J., Prpich, G., and Jillani, S. (2015). Sleep disturbances in patients with medical conditions. Psychiatr. Clin. North Am. 38, 825–841. doi: 10.1016/j.psc.2015.07.011
Kitchigina, V. F., Kutyreva, E. V., and Brazhnik, E. S. (2003). Modulation of theta rhythmicity in the medial septal neurons and the hippocampal electroencephalogram in the awake rabbit via actions at noradrenergic alpha2-receptors. Neuroscience 120, 509–521. doi: 10.1016/S0306-4522(03)00331-2
Knauber, J., and Müller, W. E. (2000). Decreased exploratory activity and impaired passive avoidance behaviour in mice deficient for the alpha(1b)-adrenoceptor. Eur. Neuropsychopharmacol. 10, 423–427. doi: 10.1016/S0924-977X(00)00100-0
Ko, A., Harada, M. Y., Barmparas, G., Thomsen, G. M., Alban, R. F., Bloom, M. B., et al. (2016). Early propranolol after traumatic brain injury is associated with lower mortality. J. Trauma Acute Care Surg. 80, 637–642. doi: 10.1097/TA.0000000000000959
Kovács, P., and Hernádi, I. (2003). Alpha2 antagonist yohimbine suppresses maintained firing of rat prefrontal neurons in vivo. Neuroreport 14, 833–836. doi: 10.1097/00001756-200305060-00011
Laing, M., and Bashir, Z. I. (2014). β-Adrenoceptors and synaptic plasticity in the perirhinal cortex. Neuroscience 273, 163–173. doi: 10.1016/j.neuroscience.2014.04.070
Laureys, G., Valentino, M., Demol, F., Zammit, C., Muscat, R., Cambron, M., et al. (2014). β2-adrenergic receptors protect axons during energetic stress but do not influence basal glio-axonal lactate shuttling in mouse white matter. Neuroscience 277, 367–374. doi: 10.1016/j.neuroscience.2014.07.022
Leão, R. M., and Von Gersdorff, H. (2002). Noradrenaline increases high-frequency firing at the calyx of Held synapse during development by inhibiting glutamate release. J. Neurophysiol. 87, 2297–2306.
Lee, A., Rosin, D. L., and Van Bockstaele, E. J. (1998). alpha2A-adrenergic receptors in the rat nucleus locus coeruleus: subcellular localization in catecholaminergic dendrites, astrocytes, and presynaptic axon terminals. Brain Res. 795, 157–169. doi: 10.1016/S0006-8993(98)00266-2
Lei, S., Deng, P. Y., Porter, J. E., and Shin, H. S. (2007). Adrenergic facilitation of GABAergic transmission in rat entorhinal cortex. J. Neurophysiol. 98, 2868–2877. doi: 10.1152/jn.00679.2007
Leo, G., Guescini, M., Genedani, S., Stocchi, V., Carone, C., Filaferro, M., et al. (2015). Acute isoproterenol induces anxiety-like behavior in rats and increases plasma content of extracellular vesicles. Physiol. Behav. 142, 79–84. doi: 10.1016/j.physbeh.2015.02.002
Lethbridge, R. L., Walling, S. G., and Harley, C. W. (2014). Modulation of the perforant path-evoked potential in dentate gyrus as a function of intrahippocampal β-adrenoceptor agonist concentration in urethane-anesthetized rat. Brain Behav. 4, 95–103. doi: 10.1002/brb3.199
Li, M., Yao, W., Li, S., and Xi, J. (2015). Norepinephrine induces the expression of interleukin-6 via β-adrenoreceptor-NAD(P)H oxidase system -NF-κB dependent signal pathway in U937 macrophages. Biochem. Biophys. Res. Commun. 460, 1029–1034. doi: 10.1016/j.bbrc.2015.02.172
Li, S., Jin, M., Zhang, D., Yang, T., Koeglsperger, T., Fu, H., et al. (2013). Environmental novelty activates β2-adrenergic signaling to prevent the impairment of hippocampal LTP by Aβ oligomers. Neuron 77, 929–941. doi: 10.1016/j.neuron.2012.12.040
Lipina, T. V., Fletcher, P. J., Lee, F. H., Wong, A. H. C., and Roder, J. C. (2013). Disrupted-in-schizophrenia-1 Gln31Leu polymorphism results in social anhedonia associated with monoaminergic imbalance and reduction of CREB and β-arrestin-1,2 in the nucleus accumbens in a mouse model of depression. Neuropsychopharmacology 38, 423–436. doi: 10.1038/npp.2012.197
Lippiello, P., Hoxha, E., Volpicelli, F., Lo Duca, G., Tempia, F., and Miniaci, M. C. (2015). Noradrenergic modulation of the parallel fiber-Purkinje cell synapse in mouse cerebellum. Neuropharmacology 89, 33–42. doi: 10.1016/j.neuropharm.2014.08.016
Liu, J.-F., Yang, C., Deng, J.-H., Yan, W., Wang, H.-M., Luo, Y.-X., et al. (2015). Role of hippocampal β-adrenergic and glucocorticoid receptors in the novelty-induced enhancement of fear extinction. J. Neurosci. 35, 8308–8321. doi: 10.1523/JNEUROSCI.0005-15.2015
Liu, W., Yuen, E. Y., Allen, P. B., Feng, J., Greengard, P., and Yan, Z. (2006). Adrenergic modulation of NMDA receptors in prefrontal cortex is differentially regulated by RGS proteins and spinophilin. Proc. Natl. Acad. Sci. U.S.A. 103, 18338–18343. doi: 10.1073/pnas.0604560103
Liu, Y., Liang, X., Ren, W.-W., and Li, B.-M. (2014). Expression of β1- and β2-adrenoceptors in different subtypes of interneurons in the medial prefrontal cortex of mice. Neuroscience 257, 149–157. doi: 10.1016/j.neuroscience.2013.10.078
Loebel, M., Grabowski, P., Heidecke, H., Bauer, S., Hanitsch, L. G., Wittke, K., et al. (2016). Antibodies to β adrenergic and muscarinic cholinergic receptors in patients with Chronic Fatigue Syndrome. Brain Behav. Immun. 52, 32–39. doi: 10.1016/j.bbi.2015.09.013
Luo, F., Guo, N.-N., Li, S.-H., Tang, H., Liu, Y., and Zhang, Y. (2014a). Reduction of glutamate release probability and the number of releasable vesicles are required for suppression of glutamatergic transmission by β1-adrenoceptors in the medial prefrontal cortex. Neuropharmacology 83, 89–98. doi: 10.1016/j.neuropharm.2014.03.020
Luo, F., Li, S.-H., Tang, H., Deng, W.-K., Zhang, Y., and Liu, Y. (2015a). Phenylephrine enhances glutamate release in the medial prefrontal cortex through interaction with N-type Ca2+ channels and release machinery. J. Neurochem. 132, 38–50. doi: 10.1111/jnc.12941
Luo, F., Tang, H., and Cheng, Z.-Y. (2015b). Stimulation of α1-adrenoceptors facilitates GABAergic transmission onto pyramidal neurons in the medial prefrontal cortex. Neuroscience 300, 63–74. doi: 10.1016/j.neuroscience.2015.04.070
Luo, F., Tang, H., Li, B., and Li, S. (2014b). Activation of α1-adrenoceptors enhances excitatory synaptic transmission via a pre- and postsynaptic protein kinase C-dependent mechanism in the medial prefrontal cortex of rats. Eur. J. Neurosci. 39, 1281–1293. doi: 10.1111/ejn.12495
Maity, S., Jarome, T. J., Blair, J., Lubin, F. D., and Nguyen, P. V. (2016). Noradrenaline goes nuclear: epigenetic modifications during long-lasting synaptic potentiation triggered by activation of β-adrenergic receptors. J. Physiol. 594, 863–881. doi: 10.1113/jp271432
Maity, S., Rah, S., Sonenberg, N., Gkogkas, C. G., and Nguyen, P. V. (2015). Norepinephrine triggers metaplasticity of LTP by increasing translation of specific mRNAs. Learn. Mem. 22, 499–508. doi: 10.1101/lm.039222.115
Manunta, Y., and Edeline, J. M. (1997). Effects of noradrenaline on frequency tuning of rat auditory cortex neurons. Eur. J. Neurosci. 9, 833–847. doi: 10.1111/j.1460-9568.1997.tb01433.x
Manunta, Y., and Edeline, J. M. (2004). Noradrenergic induction of selective plasticity in the frequency tuning of auditory cortex neurons. J. Neurophysiol. 92, 1445–1463. doi: 10.1152/jn.00079.2004
Martins, A. R. O., and Froemke, R. C. (2015). Coordinated forms of noradrenergic plasticity in the locus coeruleus and primary auditory cortex. Nat. Neurosci. 18, 1483–1492. doi: 10.1038/nn.4090
McElligott, Z. A., and Winder, D. G. (2008). Alpha1-adrenergic receptor-induced heterosynaptic long-term depression in the bed nucleus of the stria terminalis is disrupted in mouse models of affective disorders. Neuropsychopharmacology 33, 2313–2323. doi: 10.1038/sj.npp.1301635
McKinney, M., and Jacksonville, M. C. (2005). Brain cholinergic vulnerability: relevance to behavior and disease. Biochem. Pharmacol. 70, 1115–1124. doi: 10.1016/j.bcp.2005.05.019
Miller, A. H., Haroon, E., Raison, C. L., and Felger, J. C. (2013). Cytokine targets in the brain: impact on neurotransmitters and neurocircuits. Depress. Anxiety 30, 297–306. doi: 10.1002/da.22084
Mishima, K., Tanoue, A., Tsuda, M., Hasebe, N., Fukue, Y., Egashira, N., et al. (2004). Characteristics of behavioral abnormalities in alpha1d-adrenoceptors deficient mice. Behav. Brain Res. 152, 365–373. doi: 10.1016/j.bbr.2003.10.038
Nai, Q., Dong, H.-W., Hayar, A., Linster, C., and Ennis, M. (2009). Noradrenergic regulation of GABAergic inhibition of main olfactory bulb mitral cells varies as a function of concentration and receptor subtype. J. Neurophysiol. 101, 2472–2484. doi: 10.1152/jn.91187.2008
Nakamura, M., Suk, K., Lee, M.-G., and Jang, I.-S. (2013). α(2A) adrenoceptor-mediated presynaptic inhibition of GABAergic transmission in rat tuberomammillary nucleus neurons. J. Neurochem. 125, 832–842. doi: 10.1111/jnc.12259
Nalepa, I., Kreiner, G., Bielawski, A., Rafa-Zabłocka, K., and Roman, A. (2013). α1-Adrenergic receptor subtypes in the central nervous system: insights from genetically engineered mouse models. Pharmacol. Rep. 65, 1489–1497. doi: 10.1016/s1734-1140(13)71509-3
Nalepa, I., Kreiner, G., Kowalska, M., Sanak, M., Zelek-Molik, A., and Vetulani, J. (2002). Repeated imipramine and electroconvulsive shock increase alpha 1A-adrenoceptor mRNA level in rat prefrontal cortex. Eur. J. Pharmacol. 444, 151–159. doi: 10.1016/S0014-2999(02)01660-6
Nicolaides, N. C., Kyratzi, E., Lamprokostopoulou, A., Chrousos, G. P., and Charmandari, E. (2015). Stress, the stress system and the role of glucocorticoids. Neuroimmunomodulation 22, 6–19. doi: 10.1159/000362736
Nikiforuk, A. (2013). Quetiapine ameliorates stress-induced cognitive inflexibility in rats. Neuropharmacology 64, 357–364. doi: 10.1016/j.neuropharm.2012.06.042
O'Dell, T. J., Connor, S. A., Guglietta, R., and Nguyen, P. V. (2015). β-Adrenergic receptor signaling and modulation of long-term potentiation in the mammalian hippocampus. Learn. Mem. 22, 461–471. doi: 10.1101/lm.031088.113
O'Donnell, J., Zeppenfeld, D., McConnell, E., Pena, S., and Nedergaard, M. (2012). Norepinephrine: a neuromodulator that boosts the function of multiple cell types to optimize CNS performance. Neurochem. Res. 37, 2496–2512. doi: 10.1007/s11064-012-0818-x
Ordway, G. A., Schwartz, M. A., and Frazer, A. (2007). Brain Norepinephrine. Cambridge: Cambridge University Press.
Osaka, T. (2009). Heat loss responses and blockade of prostaglandin E2-induced thermogenesis elicited by alpha1-adrenergic activation in the rostromedial preoptic area. Neuroscience 162, 1420–1428. doi: 10.1016/j.neuroscience.2009.05.030
Pankratov, Y., and Lalo, U. (2015). Role for astroglial α1-adrenoreceptors in gliotransmission and control of synaptic plasticity in the neocortex. Front. Cell. Neurosci. 9:230. doi: 10.3389/fncel.2015.00230
Paschalis, A., Churchill, L., Marina, N., Kasymov, V., Gourine, A., and Ackland, G. (2009). beta1-Adrenoceptor distribution in the rat brain: an immunohistochemical study. Neurosci. Lett. 458, 84–88. doi: 10.1016/j.neulet.2009.04.023
Patel, M., and Joshi, B. (2015). Modeling the evolving oscillatory dynamics of the rat locus coeruleus through early infancy. Brain Res. 1618, 181–193. doi: 10.1016/j.brainres.2015.05.033
Peet, M., and Yates, R. A. (1981). Beta-blockers in the treatment of neurological and psychiatric disorders. J. Clin. Hosp. Pharm. 6, 155–171. doi: 10.1111/j.1365-2710.1981.tb00988.x
Phillips, C., Fahimi, A., Das, D., Mojabi, F. S., Ponnusamy, R., and Salehi, A. (2016). Noradrenergic system in down syndrome and Alzheimer's disease a target for therapy. Curr. Alzheimer Res. 13, 68–83. doi: 10.2174/1567205012666150921095924
Porterfield, V. M., Gabella, K. M., Simmons, M. A., and Johnson, J. D. (2012). Repeated stressor exposure regionally enhances beta-adrenergic receptor-mediated brain IL-1β production. Brain Behav. Immun. 26, 1249–1255. doi: 10.1016/j.bbi.2012.08.001
Rajbhandari, A. K., Baldo, B. A., and Bakshi, V. P. (2015). Predator stress-induced CRF release causes enduring sensitization of basolateral amygdala norepinephrine systems that promote PTSD-like startle abnormalities. J. Neurosci. 35, 14270–14285. doi: 10.1523/JNEUROSCI.5080-14.2015
Rajkowski, J., Kubiak, P., and Aston-Jones, G. (1994). Locus coeruleus activity in monkey: phasic and tonic changes are associated with altered vigilance. Brain Res. Bull. 35, 607–616. doi: 10.1016/0361-9230(94)90175-9
Ramakrishna, D., and Subhash, M. N. (2012). Effect of amitriptyline on adrenergic receptor number and second messenger function in rat brain. Pakistan J. Biol. Sci. 15, 871–876. doi: 10.3923/pjbs.2012.871.876
Ramos, B. P., and Arnsten, A. F. (2007). Adrenergic pharmacology and cognition: focus on the prefrontal cortex. Pharmacol. Ther. 113, 523–536. doi: 10.1016/j.pharmthera.2006.11.006
Rash, J. E., Olson, C. O., Davidson, K. G. V., Yasumura, T., Kamasawa, N., and Nagy, J. I. (2007). Identification of connexin36 in gap junctions between neurons in rodent locus coeruleus. Neuroscience 147, 938–956. doi: 10.1016/j.neuroscience.2007.04.061
Rauggi, R., Cassanelli, A., Raone, A., Tagliamonte, A., and Gambarana, C. (2005). Study of mirtazapine antidepressant effects in rats. Int. J. Neuropsychopharmacol. 8, 369–379. doi: 10.1017/S1461145705005146
Reiche, E. M. V., Nunes, S. O. V., and Morimoto, H. K. (2004). Stress, depression, the immune system, and cancer. Lancet Oncol. 5, 617–625. doi: 10.1016/S1470-2045(04)01597-9
Robbins, T. W., and Arnsten, A. F. T. (2009). The neuropsychopharmacology of fronto-executive function: monoaminergic modulation. Annu. Rev. Neurosci. 32, 267–287. doi: 10.1146/annurev.neuro.051508.135535
Robinson, A. M., Buttolph, T., Green, J. T., and Bucci, D. J. (2015). Physical exercise affects attentional orienting behavior through noradrenergic mechanisms. Behav. Neurosci. 129, 361–367. doi: 10.1037/bne0000054
Roszkowski, M., Manuella, F., von Ziegler, L., Durán-Pacheco, G., Moreau, J.-L., Mansuy, I. M., et al. (2016). Rapid stress-induced transcriptomic changes in the brain depend on beta-adrenergic signaling. Neuropharmacology 107, 329–338. doi: 10.1016/j.neuropharm.2016.03.046
Roychowdhury, S., Pena-Contreras, Z., Tam, J., Yadlapalli, A., Dinh, L., Nichols, J. A., et al. (2012). alpha(2)- and beta-adrenoceptors involvement in nortriptyline modulation of auditory sustained attention and impulsivity. Psychopharmacol. 222, 237–245. doi: 10.1007/s00213-012-2635-y
Roychowdhury, S., Zwierzchowski, A. N., Garcia-Oscos, F., Olguin, R. C., Delgado, R. S., and Atzori, M. (2014). Layer- and area-specificity of the adrenergic modulation of synaptic transmission in the rat neocortex. Neurochem. Res. 39, 2377–2384. doi: 10.1007/s11064-014-1440-x
Rozas, C., Carvallo, C., Contreras, D., Carreño, M., Ugarte, G., Delgado, R., et al. (2015). Methylphenidate amplifies long-term potentiation in rat hippocampus CA1 area involving the insertion of AMPA receptors by activation of β-adrenergic and D1/D5 receptors. Neuropharmacology 99, 15–27. doi: 10.1016/j.neuropharm.2015.07.003
Ryan, K. J., Griffin, É., Yssel, J. D., Ryan, K. M., McNamee, E. N., Harkin, A., et al. (2013). Stimulation of central β2-adrenoceptors suppresses NFκB activity in rat brain: a role for IκB. Neurochem. Int. 63, 368–378. doi: 10.1016/j.neuint.2013.07.006
Sakata, T., Yoshimatsu, H., and Kurokawa, M. (1997). Hypothalamic neuronal histamine: implications of its homeostatic control of energy metabolism. Nutrition 13, 403–411. doi: 10.1016/S0899-9007(97)91277-6
Salgado, H., Garcia-Oscos, F., Martinolich, L., Hall, S., Restom, R., Tseng, K. Y., et al. (2012a). Pre- and postsynaptic effects of norepinephrine on gamma-aminobutyric acid-mediated synaptic transmission in layer 2/3 of the rat auditory cortex. Synapse 66, 20–28. doi: 10.1002/syn.20979
Salgado, H., Garcia-Oscos, F., Patel, A., Martinolich, L., Nichols, J. A., Dinh, L., et al. (2011). Layer-specific noradrenergic modulation of inhibition in cortical layer II/III. Cereb. Cortex 21, 212–221. doi: 10.1093/cercor/bhq081
Salgado, H., Kohr, G., and Trevino, M. (2012b). Noradrenergic “tone” determines dichotomous control of cortical spike-timing-dependent plasticity. Sci. Rep. 2, 163–176. doi: 10.1038/srep00417
Salgado, H., Treviño, M., and Atzori, M. (2016). Layer- and area-specific actions of norepinephrine on cortical synaptic transmission. Brain Res. 1641, 163–176. doi: 10.1016/j.brainres.2016.01.033
Santulli, G., and Iaccarino, G. (2013). Pinpointing beta adrenergic receptor in ageing pathophysiology: victim or executioner? Evidence from crime scenes. Immun. Ageing, 10, 10. doi: 10.1186/1742-4933-10-10
Sapolsky, R. M. (2015). Stress and the brain: individual variability and the inverted-U. Nat. Neurosci. 18, 1344–1346. doi: 10.1038/nn.4109
Schmeichel, B. E., and Berridge, C. W. (2013). Wake-promoting actions of noradrenergic α1 - and β-receptors within the lateral hypothalamic area. Eur. J. Neurosci. 37, 891–900. doi: 10.1111/ejn.12084
Sekio, M., and Seki, K. (2015). Lipopolysaccharide-induced depressive-like behavior is associated with α1-adrenoceptor dependent downregulation of the membrane GluR1 subunit in the mouse medial prefrontal cortex and ventral tegmental area. Int. J. Neuropsychopharmacol. 18:pyu005. doi: 10.1093/ijnp/pyu005
Sessler, F. M., Liu, W., Kirifides, M. L., Mouradian, R. D., Lin, R. C., and Waterhouse, B. D. (1995). Noradrenergic enhancement of GABA-induced input resistance changes in layer V regular spiking pyramidal neurons of rat somatosensory cortex. Brain Res. 675, 171–182. doi: 10.1016/0006-8993(95)00060-4
Shakhawat, A. M. D., Gheidi, A., MacIntyre, I. T., Walsh, M. L., Harley, C. W., and Yuan, Q. (2015). Arc-expressing neuronal ensembles supporting pattern separation require adrenergic activity in anterior piriform cortex: an exploration of neural constraints on learning. J. Neurosci. 35, 14070–14075. doi: 10.1523/jneurosci.2690-15.2015
Shan, L., Bao, A.-M., and Swaab, D. F. (2015). The human histaminergic system in neuropsychiatric disorders. Trends Neurosci. 38, 167–177. doi: 10.1016/j.tins.2014.12.008
Sherpa, A. D., Xiao, F., Joseph, N., Aoki, C., and Hrabetova, S. (2016). Activation of β-adrenergic receptors in rat visual cortex expands astrocytic processes and reduces extracellular space volume. Synapse 70, 307–316. doi: 10.1002/syn.21908
Silvani, A., Calandra-Buonaura, G., Dampney, R. A. L., and Cortelli, P. (2016). Brain-heart interactions: physiology and clinical implications. Philos. Trans. A Math. Phys. Eng. Sci. 374:20150181. doi: 10.1098/rsta.2015.0181
Simpson, K. L., and Lin, R. C. (2007). “Neuroanatomical and chemical organization of the Locus cerouleus,” in Brain Norepinephrine, eds G. A. Ordway, M. A. Schwarts, and A. Frazer (New York, NY: Cambridge University Press), 9–52.
Siuda, E. R., McCall, J. G., Al-Hasani, R., Shin, G., Il Park, S., Schmidt, M. J., et al. (2015). Optodynamic simulation of β-adrenergic receptor signalling. Nat. Commun. 6, 8480. doi: 10.1038/ncomms9480
Smythies, J. (2005a). Section I. The cholinergic system. Int. Rev. Neurobiol. 64, 1–122. doi: 10.1016/S0074-7742(05)64001-9
Smythies, J. (2005b). Section V. Serotonin system. Int. Rev. Neurobiol. 64, 217–268. doi: 10.1016/S0074-7742(05)64005-6
Sofuoglu, M., and Sewell, R. A. (2009). Norepinephrine and stimulant addiction. Addict. Biol. 14, 119–129. doi: 10.1111/j.1369-1600.2008.00138.x
Somkuwar, S. S., Kantak, K. M., and Dwoskin, L. P. (2015). Effect of methylphenidate treatment during adolescence on norepinephrine transporter function in orbitofrontal cortex in a rat model of attention deficit hyperactivity disorder. J. Neurosci. Methods 252, 55–63. doi: 10.1016/j.jneumeth.2015.02.002
Song, D., Xu, J., Hertz, L., and Peng, L. (2015). Regulatory volume increase in astrocytes exposed to hypertonic medium requires β1 -adrenergic Na(+)/K(+) -ATPase stimulation and glycogenolysis. J. Neurosci. Res. 93, 130–139. doi: 10.1002/jnr.23469
Spreng, M., Cotecchia, S., and Schenk, F. (2001). A behavioral study of alpha-1b adrenergic receptor knockout mice: increased reaction to novelty and selectively reduced learning capacities. Neurobiol. Learn. Mem. 75, 214–229. doi: 10.1006/nlme.2000.3965
Steenbergen, L., Sellaro, R., de Rover, M., Hommel, B., and Colzato, L. S. (2015). No role of beta receptors in cognitive flexibility: evidence from a task-switching paradigm in a randomized controlled trial. Neuroscience 295, 237–242. doi: 10.1016/j.neuroscience.2015.03.049
Stemmelin, J., Cohen, C., Terranova, J.-P., Lopez-Grancha, M., Pichat, P., Bergis, O., et al. (2008). Stimulation of the beta3-Adrenoceptor as a novel treatment strategy for anxiety and depressive disorders. Neuropsychopharmacology 33, 574–587. doi: 10.1038/sj.npp.1301424
Sterley, T.-L., Howells, F. M., and Russell, V. A. (2016). Genetically determined differences in noradrenergic function: The spontaneously hypertensive rat model. Brain Res. 1641, 291–305. doi: 10.1016/j.brainres.2015.11.019
Stone, E. A., and Quartermain, D. (1999). Alpha-1-noradrenergic neurotransmission, corticosterone, and behavioral depression. Biol. Psychiatry 46, 1287–1300. doi: 10.1016/S0006-3223(99)00234-6
Szot, P., Lester, M., Laughlin, M. L., Palmiter, R. D., Liles, L. C., and Weinshenker, D. (2004). The anticonvulsant and proconvulsant effects of alpha2-adrenoreceptor agonists are mediated by distinct populations of alpha2A-adrenoreceptors. Neuroscience 126, 795–803. doi: 10.1016/j.neuroscience.2004.04.030
Tamburella, A., Micale, V., Leggio, G. M., and Drago, F. (2010). The beta3 adrenoceptor agonist, amibegron (SR58611A) counteracts stress-induced behavioral and neurochemical changes. Eur. Neuropsychopharmacol. 20, 704–713. doi: 10.1016/j.euroneuro.2010.04.006
Terakado, M. (2014). Adrenergic regulation of GABA release from presynaptic terminals in rat cerebral cortex. J. Oral Sci. 56, 49–57. doi: 10.2334/josnusd.56.49
Tohyama, M., Maeda, T., Hashimoto, J., Shrestha, G. R., and Tamura, O. (1974). Comparative anatomy of the locus coeruleus. I. Organization and ascending projections of the catecholamine containing neurons in the pontine region of the bird, Melopsittacus undulatus. J. Hirnforsch. 15, 319–330.
Ursino, M. G., Vasina, V., Raschi, E., Crema, F., and De Ponti, F. (2009). The beta3-adrenoceptor as a therapeutic target: current perspectives. Pharmacol. Res. 59, 221–234. doi: 10.1016/j.phrs.2009.01.002
Usher, M., Cohen, J. D., Servan-Schreiber, D., Rajkowski, J., and Aston-Jones, G. (1999). The role of locus coeruleus in the regulation of cognitive performance. Science 283, 549–554. doi: 10.1126/science.283.5401.549
van Tol, M.-J., van der Wee, N. J. A., van den Heuvel, O. A., Nielen, M. M. A., Demenescu, L. R., Aleman, A., et al. (2010). Regional brain volume in depression and anxiety disorders. Arch. Gen. Psychiatry 67, 1002–1011. doi: 10.1001/archgenpsychiatry.2010.121
Velásquez-Martínez, M. C., Vázquez-Torres, R., Rojas, L. V., Sanabria, P., and Jiménez-Rivera, C. A. (2015). Alpha-1 adrenoreceptors modulate GABA release onto ventral tegmental area dopamine neurons. Neuropharmacology 88, 110–121. doi: 10.1016/j.neuropharm.2014.09.002
Verheij, M. M. M., Saigusa, T., Koshikawa, N., and Cools, A. R. (2015). Accumbal α-adrenoceptors, but not β-adrenoceptors, regulate behaviour that is mediated by reserpine-sensitive storage vesicles. Behav. Pharmacol. 26, 81–90. doi: 10.1097/FBP.0000000000000098
Viemari, J.-C., Garcia, A. J., Doi, A., Elsen, G., and Ramirez, J.-M. (2013). β-Noradrenergic receptor activation specifically modulates the generation of sighs in vivo and in vitro. Front. Neural Circuits 7:179. doi: 10.3389/fncir.2013.00179
Villégier, A.-S., Drouin, C., Bizot, J.-C., Marien, M., Glowinski, J., Colpaërt, F., et al. (2003). Stimulation of postsynaptic alpha1b- and alpha2-adrenergic receptors amplifies dopamine-mediated locomotor activity in both rats and mice. Synapse 50, 277–284. doi: 10.1002/syn.10267
Volk, N., Paul, E. D., Haramati, S., Eitan, C., Fields, B. K. K., Zwang, R., et al. (2014). MicroRNA-19b associates with Ago2 in the amygdala following chronic stress and regulates the adrenergic receptor beta 1. J. Neurosci. 34, 15070–15082. doi: 10.1523/JNEUROSCI.0855-14.2014
Wada, H., Inagaki, N., Yamatodani, A., and Watanabe, T. (1991). Is the histaminergic neuron system a regulatory center for whole-brain activity? Trends Neurosci. 14, 415–418. doi: 10.1016/0166-2236(91)90034-R
Wahlbeck, K., Cheine, M. V., Gilbody, S., and Ahonen, J. (2000). Efficacy of beta-blocker supplementation for schizophrenia: a systematic review of randomized trials. Schizophr. Res. 41, 341–347. doi: 10.1016/S0920-9964(99)00069-9
Wanaka, A., Kiyama, H., Murakami, T., Matsumoto, M., Kamada, T., Malbon, C. C., et al. (1989). Immunocytochemical localization of beta-adrenergic receptors in the rat brain. Brain Res. 485, 125–140. doi: 10.1016/0006-8993(89)90674-4
Wang, D., Fu, Q., Zhou, Y., Xu, B., Shi, Q., Igwe, B., et al. (2013). β2 adrenergic receptor, protein kinase A (PKA) and c-Jun N-terminal kinase (JNK) signaling pathways mediate tau pathology in Alzheimer disease models. J. Biol. Chem. 288, 10298–10307. doi: 10.1074/jbc.M112.415141
Wang, M., Gamo, N. J., Yang, Y., Jin, L. E., Wang, X.-J., Laubach, M., et al. (2011). Neuronal basis of age-related working memory decline. Nature 476, 210–213. doi: 10.1038/nature10243
Wang, Y., Zhang, Q. J., Liu, J., Ali, U., Gui, Z. H., Hui, Y. P., et al. (2010). Noradrenergic lesion of the locus coeruleus increases the firing activity of the medial prefrontal cortex pyramidal neurons and the role of alpha2-adrenoceptors in normal and medial forebrain bundle lesioned rats. Brain Res. 1324, 64–74. doi: 10.1016/j.brainres.2010.02.009
Waselus, M., Valentino, R. J., and Van Bockstaele, E. J. (2011). Collateralized dorsal raphe nucleus projections: a mechanism for the integration of diverse functions during stress. J. Chem. Neuroanat. 41, 266–280. doi: 10.1016/j.jchemneu.2011.05.011
Waterhouse, B. D., Sessler, F. M., Liu, W., and Lin, C. S. (1991). Second messenger-mediated actions of norepinephrine on target neurons in central circuits: a new perspective on intracellular mechanisms and functional consequences. Prog. Brain Res. 88, 351–362. doi: 10.1016/s0079-6123(08)63822-4
Weinshenker, D., and Schroeder, J. P. (2007). There and back again: a tale of norepinephrine and drug addiction. Neuropsychopharmacology 32, 1433–1451. doi: 10.1038/sj.npp.1301263
Wenzel, J. M., Cotten, S. W., Dominguez, H. M., Lane, J. E., Shelton, K., Su, Z.-I., et al. (2014). Noradrenergic β-receptor antagonism within the central nucleus of the amygdala or bed nucleus of the stria terminalis attenuates the negative/anxiogenic effects of cocaine. J. Neurosci. 34, 3467–3474. doi: 10.1523/JNEUROSCI.3861-13.2014
Wilson, M. A., and McNaughton, B. L. (1994). Reactivation of hippocampal ensemble memories during sleep. Science 265, 676–679. doi: 10.1126/science.8036517
Wohleb, E. S., Hanke, M. L., Corona, A. W., Powell, N. D., Stiner, L. M., Bailey, M. T., et al. (2011). β-Adrenergic receptor antagonism prevents anxiety-like behavior and microglial reactivity induced by repeated social defeat. J. Neurosci. 31, 6277–6288. doi: 10.1523/JNEUROSCI.0450-11.2011
Yang, L. J., Liu, X., Liu, D. X., Jiang, H., Mao, X. Q., Wang, C., et al. (2012). Effects of different adrenergic blockades on the stress resistance of Wistar rats. Neurosci. Lett. 511, 95–100. doi: 10.1016/j.neulet.2012.01.046
Yuen, E. Y., Qin, L., Wei, J., Liu, W., Liu, A., and Yan, Z. (2014). Synergistic regulation of glutamatergic transmission by serotonin and norepinephrine reuptake inhibitors in prefrontal cortical neurons. J. Biol. Chem. 289, 25177–25185. doi: 10.1074/jbc.M114.567610
Zhang, L., Ouyang, M., Ganellin, C. R., and Thomas, S. A. (2013). The slow afterhyperpolarization: a target of β1-adrenergic signaling in hippocampus-dependent memory retrieval. J. Neurosci. 33, 5006–5016. doi: 10.1523/JNEUROSCI.3834-12.2013
Zhang, Z., Cordeiro Matos, S., Jego, S., Adamantidis, A., and Séguéla, P. (2013). Norepinephrine drives persistent activity in prefrontal cortex via synergistic α1 and α2 adrenoceptors. PLoS ONE 8:e66122. doi: 10.1371/journal.pone.0066122
Zhou, H.-C., Sun, Y.-Y., Cai, W., He, X.-T., Yi, F., Li, B.-M., et al. (2013). Activation of β2-adrenoceptor enhances synaptic potentiation and behavioral memory via cAMP-PKA signaling in the medial prefrontal cortex of rats. Learn. Mem. 20, 274–284. doi: 10.1101/lm.030411.113
Zhou, J., Luo, Y., Zhang, J.-T., Li, M.-X., Wang, C.-M., Guan, X.-L., et al. (2015). Propranolol decreases retention of fear memory by modulating the stability of surface glutamate receptor GluA1 subunits in the lateral amygdala. Br. J. Pharmacol. 172, 5068–5082. doi: 10.1111/bph.13272
Zhou, L., Williams, T., Lachey, J. L., Kishi, T., Cowley, M. A., and Heisler, L. K. (2005). Serotonergic pathways converge upon central melanocortin systems to regulate energy balance. Peptides 26, 1728–1732. doi: 10.1016/j.peptides.2004.12.028
Keywords: norepinephrine, adrenoceptors, stress, fight-or-flight response, ADHD, depression, psychosis, anxiety
Citation: Atzori M, Cuevas-Olguin R, Esquivel-Rendon E, Garcia-Oscos F, Salgado-Delgado RC, Saderi N, Miranda-Morales M, Treviño M, Pineda JC and Salgado H (2016) Locus Ceruleus Norepinephrine Release: A Central Regulator of CNS Spatio-Temporal Activation? Front. Synaptic Neurosci. 8:25. doi: 10.3389/fnsyn.2016.00025
Received: 12 June 2016; Accepted: 05 August 2016;
Published: 26 August 2016.
Edited by:
Dirk Feldmeyer, RWTH Aachen University, GermanyReviewed by:
Gonzalo Flores, Benemérita Universidad Autónoma de Puebla, MexicoMaria Concetta Miniaci, University of Naples Federico II, Italy
Copyright © 2016 Atzori, Cuevas-Olguin, Esquivel-Rendon, Garcia-Oscos, Salgado-Delgado, Saderi, Miranda-Morales, Treviño, Pineda and Salgado. This is an open-access article distributed under the terms of the Creative Commons Attribution License (CC BY). The use, distribution or reproduction in other forums is permitted, provided the original author(s) or licensor are credited and that the original publication in this journal is cited, in accordance with accepted academic practice. No use, distribution or reproduction is permitted which does not comply with these terms.
*Correspondence: Marco Atzori, bWFyY28uYXR6b3JpQHVhc2xwLm14; bWFyY29fYXR6b3JpQGhvdG1haWwuY29t
†These authors have contributed equally to this work.