- Unité de Neurosciences, Information et Complexité, Centre National de la Recherche Scientifique Gif sur Yvette, France
A computationally rich algorithm of synaptic plasticity has been proposed based on the experimental observation that the sign and amplitude of the change in synaptic weight is dictated by the temporal order and temporal contiguity between pre- and postsynaptic activities. For more than a decade, this spike-timing-dependent plasticity (STDP) has been studied mainly in brain slices of different brain structures and cultured neurons. Although not yet compelling, evidences for the STDP rule in the intact brain, including primary sensory cortices, have been provided lastly. From insects to mammals, the presentation of precisely timed sensory inputs drives synaptic and functional plasticity in the intact central nervous system, with similar timing requirements than the in vitro defined STDP rule. The convergent evolution of this plasticity rule in species belonging to so distant phylogenic groups points to the efficiency of STDP, as a mechanism for modifying synaptic weights, as the basis of activity-dependent development, learning and memory. In spite of the ubiquity of STDP phenomena, a number of significant variations of the rule are observed in different structures, neuronal types and even synapses on the same neuron, as well as between in vitro and in vivo conditions. In addition, the state of the neuronal network, its ongoing activity and the activation of ascending neuromodulatory systems in different behavioral conditions have dramatic consequences on the expression of spike-timing-dependent synaptic plasticity, and should be further explored.
Introduction
Modification of the efficacy of synaptic transmission, or synaptic plasticity, is widely considered as the basis of activity-dependent neuronal development and learning (Feldman and Brecht, 2005). A well-characterized form of synaptic plasticity is the potentiation and depression of synaptic transmission occurring at many neuronal structures including primary sensory cortices (see, e.g., Frégnac and Shulz, 1999; Foeller and Feldman, 2004). Experimental approaches to unveil changes in the strength of connections between two neurons have extensively developed since the 1970ies, based on the theoretical ground proposed by Hebb (1949). In Chapter 4 (The first stages of perception: growth of the assembly) of his book “The organization of behavior,” Hebb proposes that “repeated stimulation of specific receptors will lead slowly to the formation of an ‘assembly’ of association area cells which can act briefly as a closed system after stimulation has ceased.” The formation of a neuronal assembly was proposed to be implemented by a decrease of “synaptic resistances” induced by the persistence of reverberating activity that is sustained ongoing activity after transient inputs. Locally, at the level of a synapse, a period of maintained temporal correlation between pre- and postsynaptic activity would lead to an increase in the efficacy of excitatory synaptic transmission.
Although Hebb’s rule became a widely used algorithm in computational models of brain functioning, its straightforward application leads to instability of the system induced by the continuous growth of synaptic efficacies which in turn leads to a saturation of all the plastic elements of the network. This divergence of synaptic weights was solved by theoreticians by using various rules of normalization which require, in addition to Hebb’s rule, depression of the gain of other competing synapses (Stent, 1973; von der Malsburg, 1973; Sejnowski, 1977a,b). For instance, a model of self-organization was proposed by von der Malsburg (1973) to account for the development of orientation selectivity in the visual cortex. Based on the Hebbian principle, the model introduces a normalization rule where the sum of the synaptic weights of afferent contacts on one neuron remains constant with time. This implies that local increase in synaptic weight is obtained in detriment of all other contacts that were inactive at the same time. This rule bears similarities with the rule proposed by Stent (1973) who assumed a selective decrease in the efficacy of synaptic transmission of afferent fibers which were inactive at the time when the postsynaptic neuron was discharging under the influence of other inputs.
The algorithms of synaptic plasticity introduced by Sejnowski (1977a,b) to model plasticity in the cerebellum, and later by Bienenstock et al. (1982) for the primary visual cortex, overcome the problem of the synaptic saturation without introducing an ad hoc normalization rule. They are based on an input/output covariance algorithm where the change in synaptic efficacy is proportional to the covariation of pre- and postsynaptic activities. This covariation corresponds to the product of the differences of the instantaneous pre- and postsynaptic activities from their respective mean values (averaged over a certain period of preceding time). Covariance-based algorithms predict that the same synapse can both increase and decrease its synaptic efficacy, thereby allowing the connectivity state of the network to evolve into non-trivial states, i.e., non-diverging stable points that attract the dynamics of the system. Correlation-based algorithms of synaptic modification have been extensively studied experimentally in vivo in the developing visual cortex (Frégnac et al., 1988, 1992; Reiter and Stryker, 1988; Frégnac and Shulz, 1989; Bear et al., 1990; Debanne et al., 1998; McLean and Palmer, 1998), the adult visual cortex (Shulz and Frégnac, 1992) and the adult auditory cortex (Ahissar et al., 1992, 1998; Cruikshank and Weinberger, 1996).
Thus, most algorithms used to model synaptic plasticity in the developing or adult cortex include synaptic potentiation and depression rules. They can be mathematically described by a general equation where the modification of synaptic weight as a function of time is proportional to the product of a presynaptic and a postsynaptic term (review in Frégnac and Shulz, 1994, 1999). In these plasticity algorithms a precise temporal order between pre- and postsynaptic activation onsets is not required. Nonetheless, a temporal contiguity between the two events, that is a proximity in time of not more than several tens of milliseconds was required for synaptic potentiation in cortex both in vivo (Baranyi and Feher, 1981; Wigström and Gustafsson, 1985) and in vitro (Frégnac et al., 1994).
Temporal Contiguity and Order Matter: The STDP Rule
More recently, a new form of Hebbian plasticity has been described in which tight temporal contiguity and order between presynaptic and postsynaptic activities determine the amplitude and the sign of the synaptic change respectively. On theoretical grounds, this plasticity rule called spike-timing-dependent plasticity (STDP) has been proposed to be a major, computationally powerful, mechanism for induction of synaptic plasticity in vivo (Gerstner et al., 1996; Abbott and Nelson, 2000; Song et al., 2000; van Rossum et al., 2000) and a biologically plausible information storage mechanism in the brain. As we will see later, experimental evidence in vivo for this is still scarce.
STDP has been readily observed in vitro. The induction of synaptic potentiation and depression depends, at least in the quiescent in vitro network, on the relative millisecond-scale timing of presynaptic and postsynaptic action potentials (Debanne et al., 1994, 1997; Bell et al., 1997; Markram et al., 1997; Bi and Poo, 1998; Nishiyama et al., 2000; Kobayashi and Poo, 2004; Wang et al., 2005; Fino et al., 2009). In pyramidal cells of layers 2–3 and 5 of sensory cortices, when an excitatory postsynaptic potential (EPSP) generated by the presynaptic action potential precedes by up to a few tens of milliseconds the postsynaptic action potential, potentiation of the synapse is induced. Conversely, depression of the synapse is observed when the EPSP follows the postsynaptic action potential by short (0–20 ms) or long (0–100 ms) intervals, depending on the synapse being studied (Feldman, 2000; Sjöström et al., 2001; Froemke and Dan, 2002; Sjöström and Nelson, 2002). This in vitro demonstrated STDP has been specifically proposed to be important for experience-dependent plasticity at layer 4 to layer 2/3 synapses in vivo (Feldman and Brecht, 2005). An anti-Hebbian form of STDP with similar temporal requirements but inverse order has been described in cerebellum-like structures with comparable cell types (Bell et al., 1997; Tzounopoulos et al., 2004) and in some corticostriatal connections (Fino et al., 2005). In the electrosensory lobe of the electric fish, this anti-Hebbian STDP has been proposed to suppress the afferent sensory consequences of an associated motor act, facilitating the detection of unexpected stimuli (review in Bell, 1989; Bell et al., 1999).
STDP in the Intact Brain
Despite intensive studies in brain slices and cultured neurons showing that STDP is a robust phenomenon at many cortical synapses, much scarcer evidence is available about how STDP is induced by neuronal activity in the mammalian cortices in vivo (review in Dan and Poo, 2006; Caporale and Dan, 2008). Since the statistical properties of neuronal activity patterns differ between in vitro and in vivo recording conditions, it is crucial to determine if STDP exhibits similar induction requirements. An increasing number of studies are addressing this question, although only a few have directly observed STDP at the level of synaptic responses (see, e.g., Bell et al., 1997; Meliza and Dan, 2006; Cassenaer and Laurent, 2007; Jacob et al., 2007). One of the main difficulties in assessing STDP in vivo is that the induction protocols are not as uniform as in vitro, rendering the comparison between them hazardous.
The pioneering work of Levy and Steward (1983) defined for the first time the coactivity requirements for synaptic potentiation in the hippocampus of anesthetized rats. The associative induction of long-term potentiation did not require perfect synchronicity of convergent presynaptic elements but unexpectedly, Levy and Steward observed that the order of the potentiation trains of stimulation was crucial in defining the polarity, potentiation or depression, of the synaptic change. Based on this observation, Levy and Steward speculated that “a retrograde interaction between a process initiated within the main dendritic shaft and individual spines” was necessary and proposed a “retrograde electrical invasion of the spine structure” as an appealing possibility (see Stuart et al., 1997 for a review on action potential backpropagation into the dendrites).
STDP has been further shown in vivo at the single-cell level in the developing tectum of Xenopus tadpoles (Zhang et al., 1998). Evoked synaptic currents were recorded through whole-cell perforated patch electrodes while the contralateral retina was stimulated electrically at minimal stimulation intensity. By varying the time between the postsynaptic tectal action potential and the retinal input, Zhang and collaborators showed synaptic potentiation for inputs that repetitively arrived within 20 ms before the tectal spike and depression for inputs repetitively arriving within 20 ms after the tectal action potential. In the same preparation, visual inputs, instead of electrical stimulation, with particular time relationships with the postsynaptic action potential can induce long-term potentiation and depression compatible with STDP (Mu and Poo, 2006; see also Engert et al., 2002). The functional consequences of such changes in retinotectal connections have been studied by reverse correlation mapping at the level of the visual receptive field (Vislay-Meltzer et al., 2006). Positive or negative STDP protocols combining visual activation in ectopic areas of the visual field (i.e., outside the classical receptive field) and the postsynaptic current activation through the recording patch pipette were applied. These protocols were shown to sculpt receptive fields by enhancing or removing responses arising from the stimulation of conditioned sub-regions of the receptive field. (René et al. 2003) have shown similar changes in the receptive field structure of primary visual cortex in cat, induced by a combined visual and intracellular stimulation protocol. Interestingly, the changes in receptive field structure could be dynamically reversed, although not completely, by 10 min of spiking activity induced by white noise visual stimulation (Vislay-Meltzer et al., 2006). This is reminiscent of the finding by Zhou et al. (2003) showing the reversibility of activity-dependent synaptic changes by a short period of spontaneous activity (mainly bursting activity) and points to the strong lability of plastic changes in vivo compared to the in vitro conditions (see below). The quiescent state of the network in vitro allows the maintenance of an induced synaptic change since no spurious pairings occur. However, if randomized pairings at time intervals encompassing both LTP and LTD windows are imposed in vitro, robust LTD is observed (Feldman, 2000). This results since the temporal window (i.e., the integral of the learning curve) of LTD is longer than that of LTP (Feldman, 2000; Froemke and Dan, 2002). Consequently, the lability of the changes observed in vivo could result from higher levels of ongoing activity associated to an asymmetric STDP rule. The asymmetry of the learning rule in vivo depends however on the studied system (compare Meliza and Dan, 2006 and Cassenaer and Laurent, 2007). In the somatosensory cortex in vivo, the learning rule seems more symmetric than in vitro. However, one cannot exclude that a smaller asymmetry of the plasticity rule combined to a high level of ongoing activity could have the same overall reversal effect. Since the STDP window looks narrower in vivo compared to in vitro (compare Feldman, 2000 with Jacob et al., 2007), an alternative scenario would be that the temporal windows for LTD and LTP were dynamically adjusted by the ongoing activity. Because the level of activity is higher in vivo than in vitro, the system would compensate for the spurious pre-post pairings by decreasing and rendering more symmetric the temporal windows for LTD and LTP. This alternative needs however experimental validation.
In the in vivo visual cortex, the occurrence of STDP has been indirectly studied by pairing sensory and/or electrical stimulations at time intervals compatible with the STDP rule (Schuett et al., 2001; Yao and Dan, 2001; Fu et al., 2002; Yao et al., 2004). The sensory stimulations increase the firing probability of neurons within a defined window of time, and thus the pairing of two stimuli favors the imposed spike-timing interactions. In most studies using sensory–sensory associations (see also Dahmen et al., 2008 for a similar study in A1), the modifications of the neuronal response properties are rather small, particularly at the single-cell level, but the temporal specificity and the sign of the resulting response modifications are in agreement with the direction of response modifications expected from an STDP scenario and support the idea that STDP could mediate experience-dependent modulation of receptive fields in the visual cortex in vivo. The protocol used by Schuett et al. (2001) included more than 25,000 pairings between a visual stimulus presented at 7 Hz during 3 h and an associated intracortical electrical stimulation. Although the changes in the intensity of the voltage sensitive dye signal and the expansion of the cortical area representing the paired visual orientation last for up to 18 h after pairing, that is, much more than shown in any other study, the number of pairings is a hundred times larger than the average number of pairings of other studies and thus precludes a reasonable comparison.
STDP has been directly observed in vivo at the level of synaptic responses in the visual cortex (Meliza and Dan, 2006). Whole-cell recordings in the rat primary visual cortex were used to pair visually induced depolarization with spiking of the recorded neuron induced by current injection. Depending on the order of the visual input and the postsynaptic action potential, potentiation or depression was observed. Here, but also as a general observation of synaptic modification in vivo (see, e.g., Jacob et al., 2007), the amplitude of the modifications is smaller and more variable than those observed in cortical slices (Froemke and Dan, 2002).
In the intact brain neurons are submitted to a strong bombardment of input activity that affects the temporal control of presynaptic activity during pairing and in turn, affects STDP induction. Thus, the question of STDP incidence in intact sensory cortices in mammals has still to be substantiated. In the in vivo somatosensory cortex of the rat, whisker deprivation results in cortical map modifications, which are concomitant with changes in the relative timing of thalamic and cortical action potentials within the STDP range (Allen et al., 2003; Celikel et al., 2004). This indicates that STDP could be involved in response modifications at the cellular level during experience-driven network reorganizations. However, evidence for STDP in the in vivo somatosensory cortex remains scarce. Indirect evidence for this comes from a combined electrical stimulation of somatosensory afferents and transcranial magnetic stimulation (TMS) of the somatosensory cortex in humans (Wolters et al., 2005, see also Wolters et al., 2003 for a similar study on the motor cortex). Evoked potentials induced by the TMS were either enhanced or depressed as a function of the order of the paired associative stimulation.
In the primary somatosensory cortex of anesthetized adult rats, backward pairings of spontaneous postsynaptic action potentials with subthreshold afferent excitation elicited by whisker deflections lead to depression of responses to the paired whisker with no significant changes to the unpaired whisker (Jacob et al., 2007). The experimental protocol was based on mechanical deflections of the whiskers only, and no electrical stimulation of the afferent pathway was used during the pairing (see Figure 1A1). Since the action potentials of the recorded neuron were not artificially triggered but spontaneously fired by the recorded neuron, it was impossible to program a whisker stimulation that systematically preceded the action potential. Consequently, only the depression side of the STDP curve was studied. Although still in agreement with the STDP rule, the effect was observed only for time intervals shorter than −17 ms(see an example in Figure 1A2), indicating that the range of synaptic delays that drive synaptic depression is narrower in vivo (see also Yao and Dan, 2001; Fu et al., 2002; Cassenaer and Laurent, 2007; Dahmen et al., 2008 for a similar observation) than in vitro (Feldman, 2000). The shortening of the STDP window seems to depend on the developmental stage of the animal, since short windows (<20 ms) were observed in the adult (Yao and Dan, 2001; Fu et al., 2002; Dahmen et al., 2008 and the extracellular backward pairings in Jacob et al., 2007, see Figure 1A3) whereas longer STDP windows (35–50 ms) were observed in younger (intracellular pairings in Jacob et al., 2007, see Figure 1B3) or developing (Meliza and Dan, 2006) animals. In the backward pairing (Jacob et al., 2007), the trains of whisker deflections were temporally irregular because the pairing was based on the spontaneously emitted action potentials of the recorded neuron. To assess the impact of such irregularities during pairing, several established models of integration of STDP (Song and Abbott, 2001; Froemke and Dan, 2002) were used to fit the experimental data. However, no satisfactory fitting was obtained (Jacob et al., 2007). To explore the potentiation side of the STDP curve in vivo, whole-cell patch recordings were needed (see Figure 1B1). Using this technique, a timing-dependent depression of responses specific for the paired whisker was observed but spike-timing-dependent potentiation was more sporadically induced (Figures 1B2,B3). Thus, spike-timing-dependent depression can be effectively induced in vivo and is therefore a plausible plasticity mechanism in the somatosensory cortex although a refinement of specific plasticity models is still necessary to fully account for the observed response and synaptic changes.
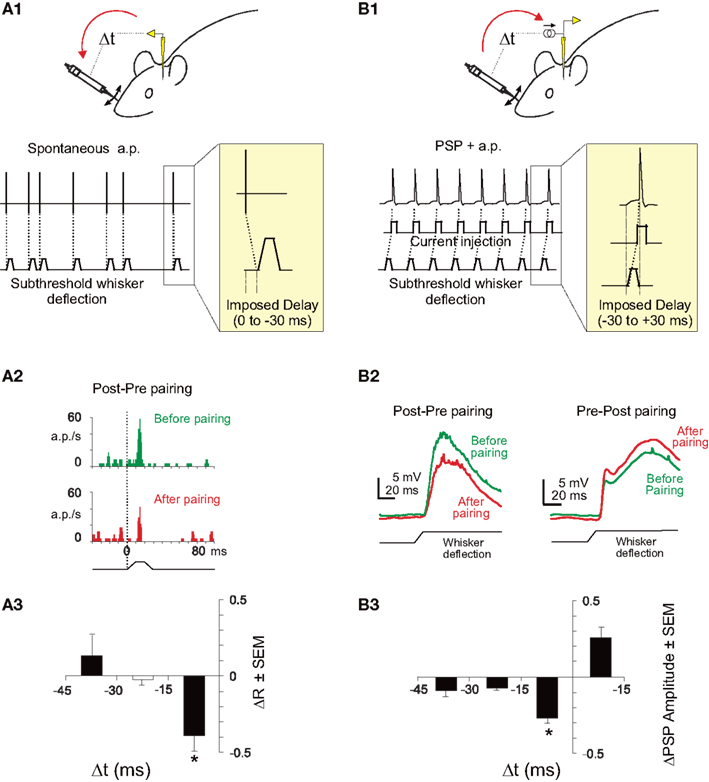
Figure 1. STDP in the somatosensory cortex of the rat. (A1) Experimental protocol for backward pairing. During control and test (not shown) whisker deflection of the principal and one adjacent whiskers were presented in a pseudorandom sequence at 0.5 Hz. The input waveform for each deflection was a 10 ms rostrocaudal movement followed by a 10 ms plateau and a ramp back to the rest position. During pairing, a spontaneously emitted action potential triggered a subthreshold deflection of one whisker with a fixed delay (0, 10, 20, or 30 ms). One pairing period contained 400 associations between an action potential and a whisker deflection. (A2) Significant depression (p < 0.05) of response of a single neuron in the barrel cortex after a backward pairing (red histogram, After pairing) compared to control (green histogram, Before pairing). (A3) Specific depression for short delays of pairing. The mean response modification for the paired whisker (ΔR = after − before/after + before) is plotted against the delay of the pairing. The delay has been corrected to take into account the latency of the cortical response. The depression is significant (t-test, *p < 0.05) only for pairings at a short-delay window (<17 ms). (B1) Experimental protocol for whole-cell induction of STDP in vivo. During control and test (not shown) whisker deflection of the principal and one adjacent whiskers were presented in alternation at 0.5 Hz. During pairing, whisker deflection was paired with current injection to elicit postsynaptic spikes at different delays (from −30 to +30 ms). (B2) Induction of spike-timing-dependent synaptic depression (left) or potentiation (right) in two representative neurons. Whisker deflection induced PSP (wPSP) were averaged over 50 trials during baseline (green line) and after pairing (red line). (B3) Learning rule for spike-timing-dependent synaptic depression in L2/3 in vivo. Mean pairing-induced changes in amplitude of the wPSP (ΔPSP = after − before/before) as a function of delay between postsynaptic spikes and wPSP onset. Adapted from Jacob et al. (2007).
Diversity of STDP Protocols Applied in the Intact Brain
As already mentioned, the different experimental protocols applied in vivo to induce STDP are rather heterogeneous in terms of the temporal frequency of the pairing and of the number of associations. In addition, while some studies combined peripheral sensory stimulation with juxtacellular or intracellular current injection to control the postsynaptic spiking discharge, others used combined sensory-sensory stimulation at inter-stimulus intervals compatible with the STDP rule. A comparative analysis is presented in Table 1 that shows a list of in vivo experiments with the corresponding characteristics of the pairings as well as the amplitude and duration of the induced effect. A peculiar correlation appears from such comparative study, which is the inverse link between the number of pairings (the Table is sorted from the highest to the lowest number of pairings) and the amplitude of the resulting modification in sensory responses [see column “Change (LTP or LTD),” expressed as percentage of baseline, in Table 1]. The inverse relation holds for response potentiations and depressions separately as well as for the cumulated effect (not shown in Table 1). The interpretation of this relationship is hazardous since there are noticeable experimental differences between the studies, including the age, the cortical area, the recording methods, the temporal frequency of pairings and more importantly, the induction protocols themselves (sensory versus electrical afferent activation). Many of the studies showing larger effects induced by a relatively small number of pairings include an intracellular control of the postsynaptic spiking activity whereas on the other hand many of the studies with a high number of pairings and relatively small plastic changes include sensory-sensory stimulation as a way of controlling the temporal correlation of pre and postsynaptic activities. Nonetheless, one plausible interpretation is that in vivo spontaneous activity generates spurious coincidences of both signs that dilute the effect of the pairing, and this effect accumulates with the number of pairings. Alternatively, homeostatic mechanisms with longer time scales than the STDP rule and saturation of synaptic modifications with several tens of associations (see Froemke et al., 2006) can regulate the expression of synaptic plasticity.
Rate Dependence of STDP
Developmental synaptic plasticity based on covariance rules in sensory cortices depends on the firing rate of presynaptic neurons. At high firing rates, the synapse is potentiated whereas at low firing rates, the synapse is depressed (see review in Bear, 2003; Malenka and Bear, 2004). Conversely, within the framework of the STDP rule, potentiation or depression can be obtained by changing the relative timing between pre- and postsynaptic action potentials with no need for changes in the firing rates. The pairing protocol applied in the somatosensory cortex (Jacob et al., 2007) controls the temporal correlation between pre and postsynaptic spikes without inducing significant modifications of the firing rate of the neuron. Consequently, the induction of the observed functional plasticity is rate-independent although the level of plasticity itself can be modulated by the temporal frequency of the ongoing activity during pairing (see below). This is similar to the study by Ahissar et al. (1992, 1998) where an increase of the functional connectivity between two neurons was induced by increasing the temporal correlation of their activity using a backward pairing similar to the one applied in our study.
The temporal frequency of the pairing was shown to influence STDP (Sjöström et al., 2001): at low frequencies, depression dominates, whereas potentiation is induced at high frequencies. In the somatosensory cortex experiments (Jacob et al., 2007), the depression induced by short-delay pairings depended on the firing frequency during pairing. It should be noted that the pairing frequency in this protocol was dictated by the spontaneous activity of the recorded neuron and was not arbitrarily chosen by the experimenter. The vulnerable nature of the activity-dependent synaptic modifications in vivo could result from the effect of the ongoing activity irrespective of the sensory driven activity. Under this scenario, there should be a dependence of the level of synaptic plasticity on the ongoing activity. Indeed, the induced depression of response was maximal for intermediate spontaneous firing rates, with an optimal firing rate at 2.5 Hz (see Figure 2). Below one action potential per second, less depression was observed than for the intermediate firing frequencies. This decrease of the level of depression for very low frequency pairings has not been observed in vitro, and may result from the fact that at very low frequency of discharge the overall time of the pairing period (400 pairings in Jacob et al., 2007) is too long compared to the duration of the effect produced by each individual pair of action potentials. This result suggests that there is an optimal level of ongoing activity for the induction of STDP. Then, it can be proposed that in vivo, cortical structures with intermediate (a few action potentials per second) or sparser activities are more prone to show STDP.
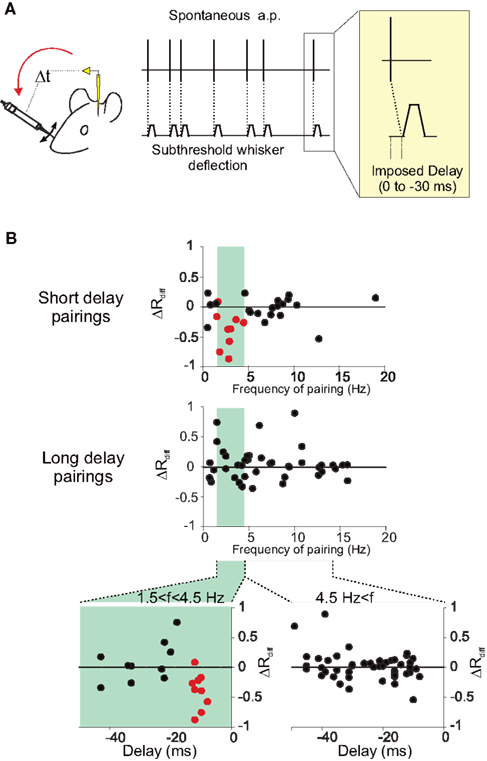
Figure 2. Influence of the temporal frequency of pairing on STDP. (A) Experiments were performed in the barrel cortex of adult anesthetized rats (see Jacob et al., 2007 for further details). During pairing, a spontaneously emitted action potential triggers a subthreshold deflection of a whisker with a fixed delay (0, 10, 20, or 30 ms). One pairing period contains 400 associations between an action potential and a stimulation of the whisker. (B) The differential change in response (ΔRdiff) defined as the difference between modifications of responses in the paired and the unpaired pathways, is plotted against the mean temporal frequency of the spontaneous action potential discharge during pairing, at short delays (upper plot, −17 < delay < −7 ms) and longer delays (middle plot, delay <−17 ms). A band-pass curve fitted to ΔRdiff for the short-delay pairings (not shown) gives a preferred frequency for the induction of response depression of 2.57 Hz. In the lower two scatter plots, relative changes in response (ΔRdiff) are plotted against the delay of the pairing computed as the imposed delay plus the latency between the stimulation and the arrival of the afferent volley to the cortex. The separation of the pairing population was based on the frequency during the pairing with frequencies between 1.5 and 4.5 Hz (left plot) leading to depression.
Impact of in vivo Activity Patterns on STDP Induction
STDP may be particularly useful in brain regions in which spike rates are low and information is conveyed in spike-timing information. The range of ongoing and evoked firing rate in awake animals differs in the different cortical areas. Extracellular recordings revealed firing rates between 10 and 25 Hz in the visual cortex (Kasamatsu and Adey, 1974; Livingstone and Hubel, 1981), less than 5 Hz in the auditory cortex (Edeline et al., 2001) and less than 1 Hz in the barrel subfield of the somatosensory cortex (Crochet and Petersen, 2006). Ongoing activity in the network affects postsynaptic membrane properties and can modulate the induction of plasticity and compromise the stability of the synaptic modifications. There are striking differences as well between sensory cortices in the ratio between phasic or tonic patterns of evoked firing.
Does STDP efficiency correlate with the sparseness of natural activity? In the retinotectal synapses of developing Xenopus, where STDP has been extensively demonstrated, the activity is sparser than in the cortex of mammals (the spontaneous firing rate is below 1 Hz, the evoked firing rate is between 1 and 2 Hz). In the Locust olfactory system, STDP has been induced in the synapses formed between the Kenyon cells of the mushroom body and cells located in the β-lobe (Cassenaer and Laurent, 2007). Here too, the activity of the Kenyon cells is extremely sparse: the average spontaneous firing rate is below 0.01 spikes per second and the activity evoked by odor presentation is still below 2 spikes per second (Perez-Orive et al., 2002; Jortner et al., 2007). Remarkably, long-term potentiation and depression can be induced in this system after only a few pairs of action potentials.
Different neuronal structures studied in awake animals show a range of activity patterns. Are the pairings used for inducing STDP likely to occur naturally? In other terms, is STDP plausible in a natural in vivo condition? In the hippocampus, a prominent activity pattern is that of theta oscillations between 4 and 12 Hz (Buzsáki and Draguhn, 2004). The phase and frequency of the theta rhythm is under the fine control of at least two independent generators acting together (Kocsis et al., 1999). Recent intracellular recordings in hippocampus of freely moving rats confirmed that theta rhythms are present at the synaptic level (Lee et al., 2006, 2009). Single-cell activity includes a few action potentials per cycle constrained to a particular phase. However, the phase of action potentials in the cycle changes as a function of the position and the direction of the animal (O’Keefe and Recce, 1993). This observation suggests that the timing of the spikes conveys information and constitutes a potential basis for physiological STDP to occur. Similarly to the observation in the somatosensory cortex, STDP-like pairings in the CA1 field of the in vivo hippocampus by stimulation of the Schaffer pathway and of the contralateral commissural pathway, induced potentiation at 5 Hz but not at 1 or 10 Hz (Dong et al., 2008). It is likely that the occurrence and amplitude of plasticity is tuned by the frequency of the theta rhythm and the phase of the spikes in the theta cycle leads to a selection of the synapses being potentiated or depressed.
Different rhythms are present at the microscopic and macroscopic level also in the cortex (Steriade, 2001; Crochet and Petersen, 2006; Poulet and Petersen, 2008) and they might affect the induction of plasticity. Non-alert states have been associated with large oscillations at low frequency (1–5 Hz), which reveal a synchronized activity and are reminiscent of the up and down states observed in anesthetized animals. Sensory cortical neurons are highly sensitive to EEG state. Changes in the amplitude of the sensory responses, size of receptive fields, and rate of spontaneous firing are observed in the alert animal compared to the non-alert animal in the visual (Wörgötter et al., 1998; Eyding et al., 2003; Stoelzel et al., 2009), the auditory (Edeline et al., 2001) and the somatosensory (Chapin and Lin, 1984; de Kock and Sakmann, 2009) cortices. Interestingly, the spontaneous firing rate of thalamocortical neurons is lower and at the same time the rate of burst discharges is higher during synchronized non-alert states compared to alert desynchronized states (Stoelzel et al., 2009). These patterns of activity characteristic of the non-alert brain should have an impact in STDP induction since for example, cortical STDP induced in vitro at low frequency (Sjöström et al., 2001; Sjöström and Nelson, 2002; Froemke et al., 2006) and/or by bursts of action potentials (Birtoli and Ulrich, 2004; Froemke et al., 2006) favors synaptic depression, even if one has to keep in mind that these observations were made in layers 2–3 and 5, that is, in non-thalamo-recipient cortical layers.
Attention related modulatory signals can change the sparseness of activity in the cortex (Vinje and Gallant, 2002), increasing the temporal precision of the network activity regime (Frégnac et al., 2006), and rendering the system more prone to STDP induction. Cortical release of noradrenaline for example, produces a reduction of spontaneous and evoked activity in the visual cortex (Ego-Stengel et al., 2002). Through this powerful inhibitory action, the noradrenergic system might provide a reset signal (Dayan and Yu, 2006), broadcasted to the whole cortical mantle, leading to an optimized level of activity for the induction of STDP. Other neuromodulators can dynamically regulate timing-based plasticity rules by modifying the biophysical properties of dendrites and the efficacy of spike back propagation (Tsubokawa and Ross, 1997; Sandler and Ross, 1999). Action potentials back propagating into the dendritic tree critically determine the induction of STDP (Engelmann et al., 2008; Sjöström et al., 2008), and its amplitude can be modulated by the network state (Waters and Helmchen, 2004) and dendritic depolarization (Sjöström and Häusser, 2006), both known to be modulated in turn by ascending neuromodulatory signals. A recent comparative study of corticostriatal plasticity in anesthetized and awake animals (Stoetzner et al., 2010) showed that the STDP plasticity rule depends critically on the behavioral state. Further in vivo experiments combining STDP induction protocols concomitant with the activation of neuromodulatory ascending systems (for slice and cell culture studies see Lin et al., 2003; Couey et al., 2007; Seol et al., 2007; Pawlak and Kerr, 2008; Zhang et al., 2009) are needed to explore how local rules of synaptic plasticity in general (Shulz et al., 2000, 2003; Ego-Stengel et al., 2001) and STDP in particular are regulated by global factors acting on several spatial (dendrites, neurons, network) and temporal (milliseconds to minutes) scales.
In conclusion, in vivo experimental evidence for STDP, although not yet compelling, has been recently provided in various species and neural structures. Significant variations of the rule (e.g., the temporal length of the STDP window) have been observed and some properties of the induced plasticity, like the duration of the effect, differ from those observed in in vitro preparations. Endogenous patterns of ongoing in vivo activity like oscillatory rhythms, burstiness and sparse neural activity might help to counteract the effect of spurious spike coincidences and reduce the vulnerability of plasticity in vivo. In addition, attention related neuromodulatory signals, through the regulation of activity patterns and/or the adaptation of the plasticity rule itself (i.e., metaplasticity, Abraham, 2008) might optimize the induction and expression of STDP in the intact brain.
Conflict of Interest Statement
The authors declare that the research was conducted in the absence of any commercial or financial relationships that could be construed as a potential conflict of interest.
Acknowledgments
We thank Yves Frégnac and Kirsty Grant for helpful comments on the manuscript. This work was supported by the European Union under the FP6 program (FP6-015879, FACETS) and the FET Open program (FP7-243914, Brain-i-Net).
References
Abbott, L. F., and Nelson, S. B. (2000). Synaptic plasticity: taming the beast. Nat. Neurosci. 3, 1178–1183.
Abraham, W. C. (2008). Metaplasticity: tuning synapses and networks for plasticity. Nat. Rev. Neurosci. 9, 387–399.
Ahissar, E., Abeles, M., Ahissar, M., Haidarliu, S., and Vaadia, E. (1998). Hebbian-like functional plasticity in the auditory cortex of the behaving monkey. Neuropharmacology 37, 633–655.
Ahissar, E., Vaadia, E., Ahissar, M., Bergman, H., Arieli, A., and Abeles, M. (1992). Dependence of cortical plasticity on correlated activity of single neurons and on behavioral context. Science 257, 1412–1415.
Allen, C. B., Celikel, T., and Feldman, D. E. (2003). Long-term depression induced by sensory deprivation during cortical map plasticity in vivo. Nat. Neurosci. 6, 291–299.
Baranyi, A., and Feher, O. (1981). Long-term facilitation of excitatory synaptic transmission in single motor cortical neurons produced by repetitive pairing of synaptic potentials and action potentials following intracellular stimulation. Neurosci. Lett. 23, 303–308.
Bear, M. F. (2003). Bidirectional synaptic plasticity: from theory to reality. Philos. Trans. R. Soc. Lond., B, Biol. Sci. 358, 649–655.
Bear, M. F., Kleinschmidt, A., Gu, Q. A., and Singer, W. (1990). Disruption of experience-dependent synaptic modifications in striate cortex by infusion of an NMDA receptor antagonist. J. Neurosci. 10, 909–925.
Bell, C. C. (1989). Sensory coding and corollary discharge effects in mormyrid electric fish. J. Exp. Biol. 146, 229–253.
Bell, C. C., Caputi, A., Grant, K., and Serrier, J. (1993). Storage of a sensory pattern by anti-Hebbian synaptic plasticity in an electric fish. Proc. Natl. Acad. Sci. U.S.A. 90, 4650–4654.
Bell, C. C., Han, V. Z., Sugawara, Y., and Grant, K. (1997). Synaptic plasticity in a cerebellum-like structure depends on temporal order. Nature 387, 278–281.
Bell, C. C., Han, V. Z., Sugawara, Y., and Grant, K. (1999). Synaptic plasticity in the mormyrid electrosensory lobe. J. Exp. Biol. 202, 1339–1347.
Bi, G. Q., and Poo, M. M. (1998). Synaptic modifications in cultured hippocampal neurons: dependence on spike timing, synaptic strength, and postsynaptic cell type. J. Neurosci. 18, 10464–10472.
Bienenstock, E., Cooper, L. N., and Munro, P. (1982). Theory for the development of neuron selectivity: orientation specificity and binocular interaction in visual cortex. J. Neurosci. 2, 32–48.
Birtoli, B., and Ulrich, D. (2004). Firing mode-dependent synaptic plasticity in rat neocortical pyramidal neurons. J. Neurosci. 24, 4935–4940.
Buzsáki, G., and Draguhn, A. (2004). Neuronal oscillations in cortical networks. Science 304, 1926–1929.
Caporale, N., and Dan, Y. (2008). Spike timing-dependent plasticity: a Hebbian learning rule. Annu. Rev. Neurosci. 31, 25–46.
Cassenaer, S., and Laurent, G. (2007). Hebbian STDP in mushroom bodies facilitates the synchronous flow of olfactory information in locusts. Nature 448, 709–713.
Celikel, T., Szostak, V. A., and Feldman, D. E. (2004). Modulation of spike timing by sensory deprivation during induction of cortical map plasticity. Nat. Neurosci. 7, 534–541.
Chapin, J. K., and Lin, C. S. (1984). Mapping the body representation in the SI cortex of anesthetized and awake rats. J. Comp. Neurol. 229, 199–213.
Couey, J. J., Meredith, R. M., Spijker, S., Poorthuis, R. B., Smit, A. B., Brussaard, A. B., and Mansvelder, H. D. (2007). Distributed network actions by nicotine increase the threshold for spike-timing-dependent plasticity in prefrontal cortex. Neuron 54, 73–87.
Crochet, S., and Petersen, C. C. (2006). Correlating whisker behavior with membrane potential in barrel cortex of awake mice. Nat. Neurosci. 9, 608–610.
Cruikshank, S. J., and Weinberger, N. M. (1996). Receptive field plasticity in adult auditory cortex induced by Hebbian covariance. J. Neurosci. 16, 861–875.
Dahmen, J. C., Hartley, D. E. H., and King, A. J. (2008). Stimulus-timing-dependent plasticity of cortical frequency representation. J. Neurosci. 28, 13629–13639.
Dan, Y., and Poo, M. M. (2006). Spike timing-dependent plasticity: from synapse to perception. Physiol. Rev. 86, 1033–1048.
Dayan, P., and Yu, A. J. (2006). Phasic norepinephrine: a neural interrupt signal for unexpected events. Network 17, 335–350.
de Kock, C. P., and Sakmann, B. (2009). Spiking in primary somatosensory cortex during natural whisking in awake head-restrained rats is cell-type specific. Proc. Natl. Acad. Sci. U.S.A. 106, 16446–16450.
Debanne, D., Gahwiler, B. H., and Thompson, S. M. (1994). Asynchronous presynaptic and postsynaptic activity induces associative long-term depression in area CA1 of the rat hippocampus in vitro. Proc. Natl. Acad. Sci. U.S.A. 91, 1148–1152.
Debanne, D., Gahwiler, B. H., and Thompson, S. M. (1997). Bidirectional associative plasticity of unitary CA3-CA1 EPSPs in the rat hippocampus in vitro. J. Neurophysiol. 77, 2851–2855.
Debanne, D., Shulz, D. E., and Frégnac, Y. (1998). Activity-dependent regulation of ‘on’ and ‘off’ responses in cat visual cortical receptive fields. J. Physiol. 15, 523–548.
Dong, Z., Han, H., Cao, J., Zhang, X., and Xu, L. (2008). Coincident activity of converging pathways enables simultaneous long-term potentiation and long-term depression in hippocampal CA1 network in vivo. PLoS ONE 3, e2848. doi: 10.1371/journal.pone.0002848.
Edeline, J. M., Dutrieux, G., Manunta, Y., and Hennevin, E. (2001). Diversity of receptive field changes in auditory cortex during natural sleep. Eur. J. Neurosci. 14, 1865–1880.
Ego-Stengel, V., Bringuier, V., and Shulz, D. E. (2002). Noradrenergic modulation of functional selectivity in the cat visual cortex: an in vivo extracellular and intracellular study. Neuroscience 111, 275–289.
Ego-Stengel, V., Shulz, D. E., Haidarliu, S., Sosnik, R., and Ahissar, E. (2001). Acethylcholine dependent induction and expression of functional plasticity in the barrel cortex of the adult rat. J. Neurophysiol. 86, 422–437.
Engelmann, J., van den Burg, E., Bacelo, J., de Ruijters, M., Kuwana, S., Sugawara, Y., and Grant, K. (2008). Dendritic backpropagation and synaptic plasticity in the mormyrid electrosensory lobe. J. Physiol. (Paris) 102, 233–245.
Engert, F., Tao, H. W., Zhang, L. I., and Poo, M. M. (2002). Moving visual stimuli rapidly induce direction sensitivity of developing tectal neurons. Nature 419, 470–475.
Eyding, D., Macklis, J. D., Neubacher, U., Funke, K., and Wörgötter, F. (2003). Selective elimination of corticogeniculate feedback abolishes the electroencephalogram dependence of primary visual cortical receptive fields and reduces their spatial specificity. J. Neurosci. 23, 7021–7033.
Feldman, D. E. (2000). Timing-based LTP and LTD at vertical inputs to layer II/II pyramidal cells in rat barrel cortex. Neuron 27, 45–56.
Feldman, D. E., and Brecht, M. (2005). Map plasticity in somatosensory cortex. Science 310, 810–815.
Fino, E., Glowinski, J., and Venance, L. (2005). Bidirectional activity-dependent plasticity at corticostriatal synapses. J. Neurosci. 25, 11279–11287.
Fino, E., Paille, V., Deniau, J. M., and Venance, L. (2009). Asymmetric spike-timing dependent plasticity of striatal nitric oxide-synthase interneurons. Neuroscience 160, 744–754.
Foeller, E., and Feldman, D. E. (2004). Synaptic basis for developmental plasticity in somatosensory cortex. Curr. Opin. Neurobiol. 14, 89–95.
Frégnac, Y., Blatow, M., Changeux, J., DeFelipe, J., Markram, H., Lansner, A., Maass, W., McCormick, D., Michel, C., Monyer, H., Szathmary, E., and Yuste, R. (2006). “Ups and downs in the genesis of cortical computation,” in Microcircuits: The Interface between Neurons and Global Brain Function Microcircuits: Dahlem Workshop Report, ed S. Grillner (Cambridge: The MIT Press), 397–437.
Frégnac, Y., Burke, J., Smith, D., and Friedlander, M. J. (1994). Temporal covariance of pre and postsynaptic activity regulates functional connectivity in the visual cortex. J. Neurophysiol. 71, 1403–1421.
Frégnac, Y., and Shulz, D. E. (1989). “Hebbian synapses in the visual cortex,” in Seeing Contour and Colour, ed. K. K. Kulikowski (Oxford: Pergamon Press), 711–718.
Frégnac, Y., and Shulz, D. E. (1994). “Models of synaptic plasticity and cellular analogs of learning in the developing and adult vertebrate visual cortex,” in Advances in Neural and Behavioral Development, eds V. Casagrande and P. Shinkman (New Jersey: Neural Ablex Publishers), 149–235.
Frégnac, Y., and Shulz, D. E. (1999). Activity-dependent regulation of receptive field properties of cat area 17 by supervised Hebbian learning. J. Neurobiol. 41, 69–82.
Frégnac, Y., Shulz, D. E., Thorpe, S., and Bienenstock, E. (1988). A cellular analogue of visual cortical plasticity. Nature 333, 367–370.
Frégnac, Y., Shulz, D. E., Thorpe, S., and Bienenstock, E. (1992). Cellular analogs of visual cortical epigenesis: I. Plasticity of orientation selectivity. J. Neurosci. 12, 1280–1300.
Froemke, R. C., and Dan, Y. (2002). Spike-timing-dependent synaptic modification induced by natural spike trains. Nature 416, 433–438.
Froemke, R. C., Tsay, I. A., Raad, M., Long, J. D., and Dan, Y. (2006). Contribution of individual spikes in burst-induced long-term synaptic modifications. J. Neurophysiol. 95, 1620–1629.
Fu, Y. X., Djupsund, K., Gao, H., Hayden, B., Shen, K., and Dan, Y. (2002). Temporal specificity in the cortical plasticity of visual space representation. Science 296, 1999–2003.
Gerstner, W., Kempter, R., van Hemmen, L., and Wagner, H. (1996). A neuronal learning rule for sub-millisecond temporal coding. Nature 383, 76–78.
Jacob, V., Brasier, D. J., Erchova, I., Feldman, D., and Shulz, D. E. (2007). Spike timing-dependent synaptic depression in the in vivo barrel cortex of the rat. J. Neurosci. 27, 1271–1284.
Jortner, R. A., Farivar, S. S., and Laurent, G. (2007). A simple connectivity scheme for sparse coding in an olfactory system. J. Neurosci. 27, 1659–1669.
Kasamatsu, T., and Adey, W. R. (1974). Excitability changes in various types of visual cortical units in freely behaving cats. Physiol. Behav. 13, 101–112.
Kobayashi, K., and Poo, M. M. (2004). Spike train timing-dependent associative modification of hippocampal CA3 recurrent synapses by mossy fibers. Neuron 41, 445–454.
Kocsis, B., Bragin, A., and Buzsaki, G. (1999). Interdependence of multiple theta generators in the hippocampus: a partial coherence analysis. J. Neurosci. 19, 6200–6212.
Lee, A. K., Epsztein, J., and Brecht, M. (2009). Head-anchored whole-cell recordings in freely moving rats. Nat. Protoc. 4, 385–392.
Lee, A. K., Manns, I. D., Sakmann, B., and Brecht, M. (2006). Whole-cell recordings in freely moving rats. Neuron 51, 399–407.
Levy, W. B., and Steward, O. (1983). Temporal contiguity requirements for longterm associative potentiation/depression in the hippocampus. Neuroscience 8, 791–797.
Lin, Y. W., Min, M. Y., Chiu, T. H., and Yang, H. W. (2003). Enhancement of associative long-term potentiation by activation of beta-adrenergic receptors at CA1 synapses in rat hippocampal slices. J. Neurosci. 23, 4173–4181.
Livingstone, M. S., and Hubel, D. H. (1981). Effects of sleep and arousal on the processing of visual information in the cat. Nature 291, 554–561.
Markram, H., Lubke, J., Frotscher, M., and Sakmann, B. (1997). Regulation of synaptic efficacy by coincidence of postsynaptic APs and EPSPs. Science 275, 213–215.
McLean, J., and Palmer, L. A. (1998). Plasticity of neuronal response properties in adult cat striate cortex. Vis. Neurosci. 15, 177–196.
Meliza, C. D., and Dan, Y. (2006). Receptive-field modification in rat visual cortex induced by paired visual stimulation and single-cell spiking. Neuron 49, 183–189.
Mu, Y., and Poo, M. M. (2006). Spike timing-dependent LTP/LTD mediates visual experience-dependent plasticity in a developing retinotectal system. Neuron 50, 115–125.
Nishiyama, M., Hong, K., Mikoshiba, K., Poo, M. M., and Kato, K. (2000). Calcium stores regulate the polarity and input specificity of synaptic modification. Nature 408, 584–588.
O’Keefe, J., and Recce, M. L. (1993). Phase relationship between hippocampal place units and the EEG theta rhythm. Hippocampus 3, 317–330.
Pawlak, V., and Kerr, J. N. (2008). Dopamine receptor activation is required for corticostriatal spike-timing-dependent plasticity. J. Neurosci. 28, 2435–2446.
Perez-Orive, J., Mazor, O., Turner, G. C., Cassenaer, S., Wilson, R. I., and Laurent, G. (2002). Oscillations and sparsening of odor representations in the mushroom body. Science 297, 359–365.
Poulet, J. F., and Petersen, C. C. (2008). Internal brain state regulates membrane potential synchrony in barrel cortex of behaving mice. Nature 454, 881–885.
Reiter, H. O., and Stryker, M. P. (1988). Neural plasticity without postsynaptic action potentials: less-active inputs become dominant when kitten visual cortical cells are pharmacologically inhibited. Proc. Natl. Acad. Sci. U.S.A. 85, 3623–3627.
René, A., Huguet, N., Pananceau, M., Grant, K., and Frégnac, Y. (2003). An in vivo generalisation of Hebbian plasticity rules in adult visual cortex to multiple pre-post synaptic activity correlations. Soc. Neurosci. Abstr. 29, 266.17.
Sandler, V. M., and Ross, W. N. (1999). Serotonin modulates spike backpropagation and associated [Ca2+]i changes in the apical dendrites of hippocampal CA1 pyramidal neurons. J. Neurophysiol. 81, 216–224.
Schuett, S., Bonhoeffer, T., and Hubener, M. (2001). Pairing-induced changes of orientation maps in cat visual cortex. Neuron 32, 325–337.
Sejnowski, T. J. (1977a). Storing covariance with non-linearly interacting neurons. J. Math. Biol. 4, 303–321.
Sejnowski, T. J. (1977b). Statistical constraints on synaptic plasticity. J. Theor. Biol. 69, 387–389.
Seol, G. H., Ziburkus, J., Huang, S., Song, L., Kim, I. T., Takamiya, K., Huganir, R. L., Lee, H. K., and Kirkwood, A. (2007). Neuromodulators control the polarity of spike-timing-dependent synaptic plasticity. Neuron 55, 919–929.
Shulz, D. E., Ego-Stengel, V., and Ahissar, E. (2003). Acetylcholine-dependent potentiation of temporal frequency representation in the barrel cortex does not depend on response magnitude during conditioning. J. Physiol. Paris 97, 431–439.
Shulz, D. E., and Frégnac, Y. (1992). Cellular analogs of visual cortical epigenesis: II. Plasticity of binocular integration. J. Neurosci. 12, 1301–1318.
Shulz, D. E., Sosnik, R., Ego, V., Haidarliu, S., and Ahissar, E. (2000). A neuronal analogue of state-dependent learning. Nature 403, 549–553.
Sjöström, P. J., and Häusser, M. (2006). A cooperative switch determines the sign of synaptic plasticity in distal dendrites of neocortical pyramidal neurons. Neuron 51, 227–238.
Sjöström, P. J., and Nelson, S. B. (2002). Spike timing, calcium signals and synaptic plasticity. Curr. Opin. Neurobiol. 12, 305–314.
Sjöström, P. J., Rancz, E. A., Roth, A., and Häusser, M. (2008). Dendritic excitability and synaptic plasticity. Physiol. Rev. 88, 769–840.
Sjöström, P. J., Turrigiano, G. G., and Nelson, S. B. (2001). Rate, timing, and cooperativity jointly determine cortical synaptic plasticity. Neuron 32, 1149–1164.
Song, S., and Abbott, L. F. (2001). Cortical development and remapping through spike timing-dependent plasticity. Neuron 32, 339–350.
Song, S., Miller, K. D., and Abbott, L. F. (2000). Competitive Hebbian learning through spike-timing-dependent synaptic plasticity. Nat. Neurosci. 3, 919–926.
Stent, G. (1973). A physiological mechanism for Hebb’s postulate of learning. Proc. Natl. Acad. Sci. U.S.A. 70, 997–1001.
Steriade, M. (2001). Impact of network activities on neuronal properties in corticothalamic systems. J. Neurophysiol. 86, 1–39.
Stoelzel, C. R., Bereshpolova, Y., and Swadlow, H. A. (2009). Stability of thalamocortical synaptic transmission across awake brain states. J. Neurosci. 29, 6851–6859.
Stoetzner, C. R., Pettibone, J. R., and Berke, J. D. (2010). State-dependent plasticity of the corticostriatal pathway. Neuroscience 165, 1013–1018.
Stuart, G., Spruston, N., Sakmann, B., and Hausser, M. (1997). Action potential initiation and backpropagation in neurons of the mammalian CNS. Trends Neurosci. 20, 125–131.
Tsubokawa, H., and Ross, W. N. (1997). Muscarinic modulation of spike backpropagation in the apical dendrites of hippocampal CA1 pyramidal neurons. J. Neurosci. 17, 5782–5791.
Tzounopoulos, T., Kim, Y., Oertel, D., and Trussell, L. O. (2004). Cell-specific, spike timing-dependent plasticities in the dorsal cochlear nucleus. Nat. Neurosci. 7, 719–725.
van Rossum, M. C., Bi, G. Q., and Turrigiano, G. G. (2000). Stable Hebbian learning from spike timing-dependent plasticity. J. Neurosci. 20, 8812–8821.
Vinje, W. E., and Gallant, J. L. (2002). Natural stimulation of the nonclassical receptive field increases information transmission efficiency in V1. J. Neurosci. 22, 2904–2915.
Vislay-Meltzer, R. L., Kampff, A. R., and Engert, F. (2006). Spatiotemporal specificity of neuronal activity directs the modification of receptive fields in the developing retinotectal system. Neuron 50, 101–114.
von der Malsburg, C. (1973). Self-organization of orientation sensitive cells in the striate cortex. Kybernetik 14, 85–100.
Wang, H. X., Gerkin, R. C., Nauen, D. W., and Bi, G. Q. (2005). Coactivation and timing dependent integration of synaptic potentiation and depression. Nat. Neurosci. 8, 187–193.
Waters, J., and Helmchen, F. (2004). Boosting of action potential backpropagation by neocortical network activity in vivo. J. Neurosci. 24, 11127–11136.
Wigström, H., and Gustafsson, B. (1985). On long-lasting potentiation in the hippocampus: a proposed mechanism for its dependence on coincident pre- and postsynaptic activity. Acta Physiol. Scand. 123, 519–522.
Wolters, A., Sandbrink, F., Schlottmann, A., Kunesch, E., Stefan, K., Cohen, L. G., Benecke, R., and Classen, J. (2003). A temporally asymmetric Hebbian rule governing plasticity in the human motor cortex. J. Neurophysiol. 89, 2339–4523.
Wolters, A., Schmidt, A., Schramm, A., Zeller, D., Naumann, M., Kunesch, E., Benecke, R., Reiners, K., and Classen, J. (2005). Timing-dependent plasticity in human primary somatosensory cortex. J. Physiol. 565, 1039–1052.
Wörgötter, F., Suder, K., Zhao, Y., Kerscher, N., Eysel, U. T., and Funke, K. (1998). State-dependent receptive-field restructuring in the visual cortex. Nature 396, 165–168.
Yao, H., and Dan, Y. (2001). Stimulus timing-dependent plasticity in cortical processing of orientation. Neuron 32, 315–323.
Yao, H., Shen, Y., and Dan, Y. (2004). Intracortical mechanism of stimulus-timing dependent plasticity in visual cortical orientation tuning. Proc. Natl. Acad. Sci. U.S.A. 101, 5081–5086.
Zhang, J. C., Lau, P. M., and Bi, G. Q. (2009). Gain in sensitivity and loss in temporal contrast of STDP by dopaminergic modulation at hippocampal synapses. Proc. Natl. Acad. Sci. U.S.A. 106, 13028–13033.
Zhang, L. I., Tao, H. W., Holt, C. E., Harris, W. A., and Poo, M. (1998). A critical window for cooperation and competition among developing retinotectal synapses. Nature 395, 37–44.
Keywords: Hebb, STDP, in vivo, ongoing activity, synaptic plasticity, learning
Citation: Shulz DE and Jacob V (2010) Spike-timing-dependent plasticity in the intact brain: counteracting spurious spike coincidences. Front. Syn. Neurosci. 2:137. doi: 10.3389/fnsyn.2010.00137
Received: 17 May 2010;
Paper pending published: 02 July 2010;
Accepted: 06 August 2010;
Published online: 24 August 2010
Edited by:
Henry Markram, Ecole Polytechnique Federale de Lausanne, SwitzerlandReviewed by:
Anita Luthi, UNIL-CHUV, SwitzerlandShigeru Kubota, Yamagata University, Japan
Copyright: © 2010 Shulz and Jacob. This is an open-access article subject to an exclusive license agreement between the authors and the Frontiers Research Foundation, which permits unrestricted use, distribution, and reproduction in any medium, provided the original authors and source are credited.
*Correspondence: Daniel E. Shulz, Unité de Neurosciences, Information et Complexité, UPR CNRS 3293, Centre National de la Recherche Scientifique, 1 Avenue de la Terrasse, 91198 Gif sur Yvette, France. e-mail:c2h1bHpAdW5pYy5jbnJzLWdpZi5mcg==