- Hydrometallurgy and Environment Laboratory, The Robert M. Buchan Department of Mining, Queen's University, Kingston, ON, Canada
The extensive neutralization required in acidic bio-oxidation, a conventional pretreatment for low-grade refractory matrices in the gold industry, constitutes one of the principal drawbacks due to the large volume of waste streams. Performing an oxidative pretreatment at circumneutral pH with an in-situ neutralization would avoid the production of undesirable waste, causing potential economic and environmental advantages. For the first time, this investigation evaluates a novel process involving a biological oxidative pretreatment for low-grade refractory ore using two biosafety level 1 neutrophilic microorganisms encompassing Thiobacillus thioparus and Starkeya novella at near-neutral pH. Optimal bacterial growth conditions were determined regarding the culture medium and initial energy source using UV-visible and manual cell counting (cells/mL). Thereafter, biological oxidation of different matrices, including first elemental sulfur and subsequently a refractory sulfidic ore, was evaluated in batch flask cultures and then scaled up into a bioreactor using optimal experimental conditions. Results revealed that culture media containing ca. 4.5 and ca. 0.9 g/L thiosulfate favored biological oxidation of the refractory sulfidic ore using T. Thioparus and S. Novella, respectively, which led to corresponding sulfide oxidation of 27 and 14% within 10 days, comparable to reported studies. The biological action was confirmed by C/S detector and SEM technique of pre- and post-pretreatment residues. Overall, this research is a step forward to advance the understanding of a biological pretreatment out of the highly acidic pH range, promoting the view of a net-zero target by potentially reducing the production of more significant waste streams compared to conventional operations.
Introduction
A huge amount of hazardous waste e.g., cyanide bearing tailings and residues, along with additional operational costs could arise from direct processing of low-grade refractory gold ores without an oxidative pre-treatment (Marsden and House, 2006; Adams, 2016). The processing of low-grade refractory gold ores is a growing challenge in the industry as the average grade of deposits is constantly decreasing over time, which might include matrices with increasingly complex mineralogy associated with carbonaceous materials (Marsden and House, 2006; Adams, 2016). Currently, one-third of the total global gold production is obtained from refractory deposits, which require an oxidative pre-treatment to extract gold economically (Marsden and House, 2006; Yang et al., 2013).
The principal pre-treatment methods to oxidize refractory gold ores include roasting, pressure oxidation, and bio-oxidation. Roasting and pressure oxidation are effective technologies but have high capital and operating cost associated with increased energy input and emission of greenhouse gases and can be unprofitable for refractory ores with extremely low grades (Azizitorghabeh et al., 2022; Darvanjooghi et al., 2022), Bio-oxidation has several advantages over traditional methods owing to its less energy requirements and environmental impact by decreasing gas emissions and can be suitable for low-grade matrices (Aswegen et al., 2007; Kaksonen et al., 2014b). Bio-oxidation is a biological oxidation technique involving acidophilic bacteria to enhance the extraction of valuable metals by accelerating the oxidation of the sulfide matrix in refractory ores, which in turn improves gold recovery during cyanide leaching. Biological oxidative technologies encompassing BIOX® and BacTech Bacox have been successfully executed in the gold industry (Darvanjooghi et al., 2022), rendering uneconomic gold reserves into valuable materials which would otherwise be considered unprofitable resources owing to their low grade and generation of more production-related waste.
Despite the efficacy of bio-oxidation employing acidophilic bacteria, pH control endures as one of the main drawbacks as a consequence of significantly acidic pH conditions and extensive neutralization of effluents afterwards. In standard bio-oxidation plants, pH control accounts for ca. 30% of the total operational cost comprising sulfuric acid, lime, and limestone (Aswegen et al., 2007; Lee et al., 2021). Prior to cyanide leaching at alkaline pH, neutralizing reagents, e.g., slaked lime and limestone, are applied to generate effluents with near-neutral pH and avoid acid mine drainage (AMD) (Darvanjooghi et al., 2022). Moreover, highly acid pH operations could have more risk of leaving residual acid in cyanide leaching, which could lead to cyanide release as HCN (Lee et al., 2021). Hence, conducting an oxidative pretreatment out of the extreme acidic pH range would prevent the generation of undesirable waste streams and diminish the concentration of reagents required in the neutralization stage, bringing potential economic and environmental advantages.
A bio-oxidation pretreatment performed out of the extreme acidic pH zone must contemplate neutrophilic sulfur-oxidizing bacteria instead of acidophilic microorganisms. Neutrophilic bacteria include microorganisms that can thrive at pH > 4 but have a more favorable growth at a circumneutral pH between pH 6.0 and 8.0 (Kelly et al., 2015; Lee et al., 2021). Most neutrophiles are commonly found in soils, wastewater, and marine freshwater and have an important role in the natural sulfur cycle on earth (McNeice et al., 2022). Neutrophilic bacteria also have significant importance in the waste remediation industry, including desulfurizing both liquid and gas waste streams.
Most of the strains investigated includes but are not limited to Citrobacter, Halothiobacillus, Nocardioides, Parapedobacter, Rhodobacter, Rhodopseudomonas, Starkeya, Thiobacillus, Thermithiobacillus, and Thiomonas. Recent research has emphasized the efficacy of neutrophilic microorganisms in oxidizing reduced sulfur species, especially in gaseous waste streams such as hydrogen sulfide (H2S) which is preferentially oxidized to sulfate in batch experiments (Lee et al., 2021). The effectiveness of the desulfurization of H2S with neutrophilic bacteria has led to its industrial implementation in H2S odor control in industrial waste encompassing BIOREM technologies Inc. and ALKEN ENZ-ODOR® 8 Alken-Murray Corporation (Alken-Murray, 2006; Biorem Technology Inc, 2015). Nonetheless, implementing neutrophilic bacteria in mining has been scarcely explored, and require further research.
Until now, most research has comprised bio-oxidation with acidophilic bacteria at low pH, which is based on the oxidation of reduced sulfur species as well as ferric iron through contact and non-contact biological mechanisms (Azizitorghabeh et al., 2022). The overall bio-oxidation reaction of pyrite using acidophilic bacteria is displayed in Equation 1, where Fe3+ is regenerated throughout the reaction by iron-oxidizing bacteria, which is unlikely to take place at circumneutral pH due to Fe insolubility at pH > 4 (Percak-Dennett et al., 2017). Scarce research toward performing a bio-oxidation operation with neutrophilic bacteria applied to low-grade refractory sulfidic ores has been reported. Microbial oxidation of pyrite at circumneutral pH has been suggested to occur through a polythionate intermediate model and/or sorbed Fe redox cycling model (Moses et al., 1987; Moses and Herman, 1991; Schippers, 2004). The overall reaction for microbially assisted reaction in natural environments is shown in Equation 2. which assumed a rapid formation of iron (III) hydroxide (Fe(OH)3) due to iron insolubility.
Few research groups have reported the dissolution of solid sulfide matrices with neutrophilic bacteria, primarily using a solid-to-liquid ratio < 5 g/L. Vázquez-Rodríguez et al. (2015) evaluated T. thioparus in the dissolution of mercury sulfide (HgS), and Percak-Dennett et al. (2017) assessed indigenous microorganisms including Rhizobiales and Ralstonia species in synthetic pyrite (FeS2) oxidation. Nonetheless, to our knowledge, there is no direct investigation into the oxidative capacities of neutrophilic bacteria on the bio-oxidation of low-grade refractory sulfidic ores at initial circumneutral pH and high pulp densities.
This paper assesses a new technological opportunity evaluating the oxidative capacity of two neutrophilic bacteria with refractory sulfidic ore. First, the effect of medium composition on bacterial growth was evaluated by changing the individual concentration of certain ingredients in the culture medium to find optimal growth conditions for bio-oxidation tests. Second, elemental sulfur was selected as a baseline test to confirm the oxidative abilities of both microorganisms on solid matrices. Third, bio-oxidation tests were performed using a low refractory sulfidic ore by modifying experimental conditions and later scaling up in a 1 L jacketed bioreactor. Lastly, a comparative study with similar investigations was provided highlighting the output of this research.
Materials and methods
Sample preparation
Elemental sulfur (S°) with a purity of 99.5% (Alfa Aesar) and particle size < 44 μm was initially used in bio-oxidation tests. Subsequently, a low-grade refractory sulfidic ore from a Canadian deposit was employed in further bio-oxidation experiments. The ore was acquired in a coarse form and later crushed and pulverized into fine particles. Manual rifflers were used to obtain representative samples. The particle size of 80% passing (P80) of the sample prior to bio-oxidation was measured with laser particle size analyzer (Malvern Mastersizer 3000).
Growth of neutrophilic bacteria
Thiobacillus Thioparus (DSMZ 505) and Starkeya Novella (DSMZ 506) were acquired in freeze-dried form from The German Collection of Microorganism and Cell Cultures (DSMZ). Both neutrophilic microorganisms are classified as biosafety level 1 (BSL1) sulfur-oxidizing bacteria. T. thioparus was grown in batch cultures of Thiobacillus medium DSMZ 36 (TM) which is composed of biological grade reagents including 0.1 g/L (NH4)2SO4, 4 g/L K2HPO4, 4 g/L KH2PO4, 0.1 g/L MgSO4·7H2O, 0.1 g/L CaCl2, 0.02 g/L FeCl3·6H2O, 0.02 g/L MnSO4·H2O, 10 g/L Na2S2O3·5H2O (Sigma-Aldrich and Fisher scientific) unless specified otherwise. Thiosulfate was used as primary energy sources for its metabolism and the phosphate salts in the medium conditioned the initial pH at 6.6 (Kelly et al., 2015). On the other hand, S. novella was also cultured in a modified Thiobacillus medium DSMZ 36 (TM1–6) supplemented with glucose (1.5 g/L, Fisher scientific) and yeast extract (0.3 and 5 g/L, Sigma-Aldrich) to favor its mixotrophic growth (Table 1), which considered the utilization of organic and inorganic substances (Leefeldt and Matin, 1980; Purnomo et al., 2019). Furthermore, S. novella was also grown in S. novella medium DSMZ 69 (SM) containing 10.6 g/L Na2HPO4·12H2O, 1.5 g/L KH2PO4, 0.3 g/L NH4Cl, 0.3 g/L yeast extract, 2 mL/L phenol red solution (0.1% w/v), 0.1 g/L MgSO4·7H2O, 5 g/L Na2S2O3·5H2O (Sigma-Aldrich and Fisher scientific), 5 mL/L trace element solution (MD-TMS, Cedarlane) as well as Trypticase soy broth medium DSMZ 535 (BM) comprised of 30 g/L trypticase soy broth (Fisher scientific).
Cultivation of these microbes was carried out in a rotatory shaking incubator (Biobase; BJPX-2102C) at 30°C and mixing speed of 150 RPM using 5% v/v inoculum and 500 mL Erlenmeyer flasks with 200 mL of working volume, unless stated otherwise. Before the experiments, all glassware was autoclaved at 121°C for 20 min and solutions were filtered with 0.2 μm Thermo Scientific Nalgene Rapid-Flow filter to maintain aseptic conditions. Optimal bacterial growth conditions for subsequent bio-oxidation tests were studied by monitoring the pH, oxidation-reduction potential (ORP), dissolved oxygen (DO), optical density at 600 nm (OD600nm), bacterial density (cells/mL) and thiosulfate consumption (mg/L). Both microorganisms were subjected to an adaptation process to the sulfidic ore for 3 months following the method of Astudillo and Acevedo (2008) and were used in the experiments. Nevertheless, the adaptation was fruitless over the considered adaptation time compared to the original strains. All experiments were carried out in duplicates throughout this investigation.
Bio-oxidation experiments
In the first instance, elemental sulfur (S°) was used in bio-oxidation tests to evaluate the potential of both microorganisms to oxidize solid matrices. A concentration of 5,000 mg/L S° was initially employed as a sulfur material in bio-oxidation tests using a two-step methodology unless stated otherwise. A two-step bio-oxidation technique involves tests at which bacterial inoculum (5% w/v) of an existing culture is originally incubated in a fresh medium and allows to propagate using the medium components, e.g., thiosulfate, as an initial energy source to achieve an optimal growth. After the exponential phase of the bacterial growth (2–3 days) was accomplished, the sulfur material to be oxidized was introduced into the reactor, commencing the two-step reaction. Tests were conducted in a rotatory shaking incubator at 30°C and 150 RPM using 500 mL Erlenmeyer flasks with a working volume of 200 mL for a total experimental time of 10 days. pH, ORP, DO, and bacterial density were monitored throughout the tests. Control tests were aseptically carried out under identical testing conditions using the same bacterial growth medium with no microorganisms added.
Subsequently, the oxidative capacities of these neutrophilic microorganisms were tested with a refractory sulfidic ore following a similar experimental procedure used for S°, as specified in Table 2. Thus, once the exponential phase of the bacterial growth was successfully reached, a sulfidic ores sample at a solid to liquid ratio of 10–100 g/L was added into the reactor. Bio-oxidation tests with sulfidic ores were mainly performed in 500 mL Erlenmeyer flasks at the ideal conditions for bacterial growth mentioned in the previous section in a temperature-controlled incubator for 10 days of reaction time. Thymol (1% m/v) wash was used to ensure there was no other culture already present in the sulfidic ore before starting the tests (de Carvalho et al., 2019). 2 mL aliquots were withdrawn to monitor bacterial growth, pH, ORP and DO. Following tests in 500 mL reactors, bio-oxidation reactions with the refractory sulfidic ore were scaled up in a 1 L jacketed reactor (Chemglass) equipped with overhead mixing at 150 RPM as shown in Figure 1. The temperature was adjusted to 30°C, and the air was sparged at 0.1 L/min to maintain DO concentration throughout the test. A volume of 950 mL of fresh culture medium was added to the reactor, followed by 5% w/v inoculum of active bacterial culture, which was grown 2–3 days before starting the test with the ore. After 10 days of contact time, the reactor test was ended, and a liquid aliquot was taken for further analysis. The pulp was pressure-filtered, separating solid residue from the liquid phase and the solid residue was washed with deionized water and filtered one more time.
Analytical techniques
The minerology of the sulfidic ore was determined by X-ray diffraction analysis (XRD; Philips, Netherlands) and the morphology of solid surfaces was characterized with scanning electron microscopy (SEM; Bruker AXS Microanalysis GmbH, Germany). The concentration of elements in the sulfidic ores was determined with coupled plasma-optical emission spectrometry (ICP-OES; PerkinElmer ELAN 9000/NexION).
Cell growth was monitored with ultraviolet–visible spectroscopy (UV-VIS; Thermo Scientific GENESYS 10S) contrasted against aseptic culture medium at 600 nm and manual cell counting employing a Neubauer counting chamber and a Rebel microscope (ECHO) at 40–100 × magnification following the method of Abcam (2022) throughout the tests as shown in Supplementary Figure S1. Liquid samples were filtrated with 0.45 μm sterile syringe filter before further analysis. pH and oxidation-reduction potential (ORP) were monitored utilizing a saturated Ag/AgCl electrode on a multichannel pH/ORP probe (VWR; Symphony H30PCO). Dissolved oxygen (DO) was obtained using an optical DO sensor connected to a multimeter (METTLER TOLEDO, Fisher Scientific). Thiosulfate concentration was determined with iodometric titration using the method of Arima et al. (2004) and sulfate concentration was measured with ion chromatography (IC; Methrohm 930 Compact IC Flex).
After S° bio-oxidation, S° was washed with DI water and dried in a vacuum-sealed oven below 40°C (Liu et al., 2013). The oxidation rate in S° bio-oxidation was estimated by considering the concentration of initial elemental sulfur and the concentration of elemental sulfur in the final residue. Hence, sulfur oxidation was therefore calculated as shown in Equation 3:
where represent the mass (g) of S° initially and _residue stands for mass (g) S°-S in the residue.
Moreover, sulfide oxidation rate in sulfidic ore was determined using carbon/sulfur analyzer (Eltra CS 200) (Ofori-Sarpong et al., 2011; Kaksonen et al., 2014a). After finishing the tests, the oxidized sample was separated and dried in a vacuum-sealed oven below 40°C. The treated sample was washed with toluene in a ratio of 1 g: 50 mL at 60°C for 60 min to remove any S° formed during bio-oxidation and dried under identical conditions (Marzoughi et al., 2022). Subsequently, the oxidized sample was washed with 3M hydrochloric acid (HCl) at 65°C for 90 min to remove sulfate () (Ofori-Sarpong et al., 2011). The washed residues were dried and further analyzed with carbon/sulfur analyzer to determine the sulfide oxidation rate. The oxidation rate was calculated based on the total sulfide concentration in the residue as displayed in Equation 4 (Kaksonen et al., 2014a):
wherein msulfide − S_ore stands for the mass (g) of sulfide-S in the original ore and msulfide − S_residue represent mass (g) sulfide-S in the residue after washing with toluene and HCl.
Results and discussion
Sample characterization
The composition of the sample is presented in Table 3. The low-grade refractory sulfidic ore sample contained 5.7% w/w total sulfur and 1.7 ppm Au with a minor content of heavy metals. Moreover, Figure 2A displays the XRD pattern of the refractory sulfidic ores used in bio-oxidation. The main phases in the ore were quartz, muscovite, greigite, spangolite, and pyrite. Further, Figure 2B shows the particle size of 80% passing (P80) which was determined to be 55 μm.
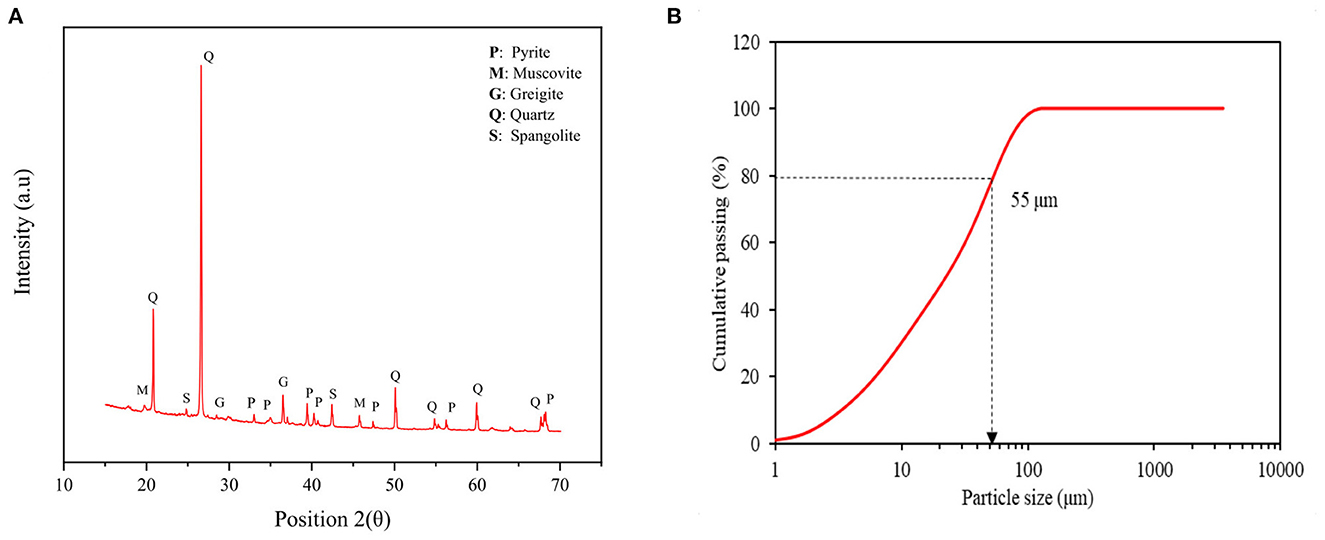
Figure 2. (A) XRD pattern and (B) particle size distribution of low-grade refractory sulfidic ore for bio-oxidation.
Effect of medium composition on bacterial growth
The bacterial growth of both neutrophilic bacteria was evaluated in response to changes in the concentration of certain ingredients in the culture media. Figures 3, 4 show the changes in bacterial growth as well as the fluctuations in pH, ORP (mv), and measured thiosulfate concentration (mg/L) over time for T. thioparus and S. novella, respectively.
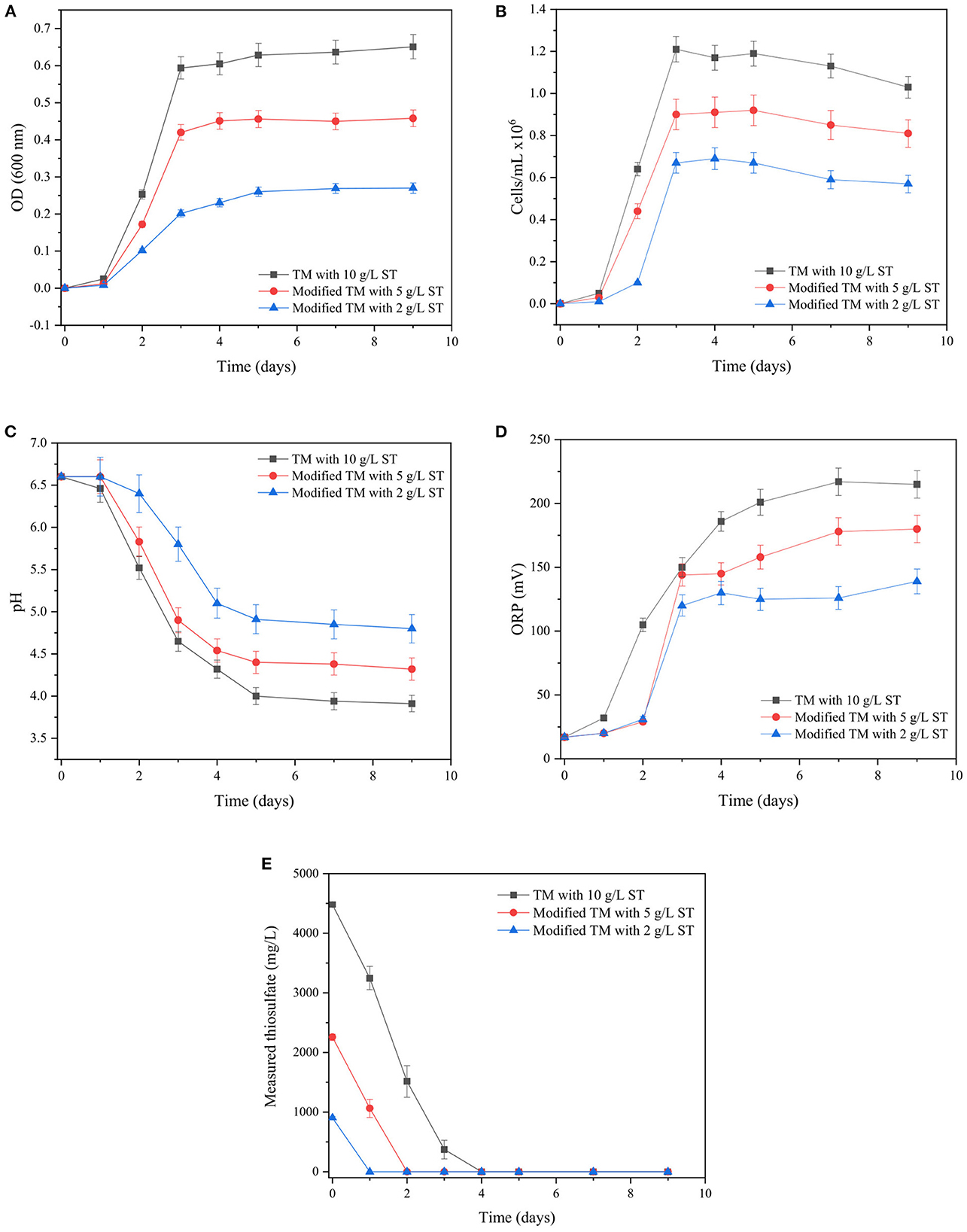
Figure 3. Changes in (A) OD600, (B) bacterial density (cells/mL), (C) pH, (D) ORP (mV), (E) measured thiosulfate (mg/L) over time with T. thioparus cultured in TM.
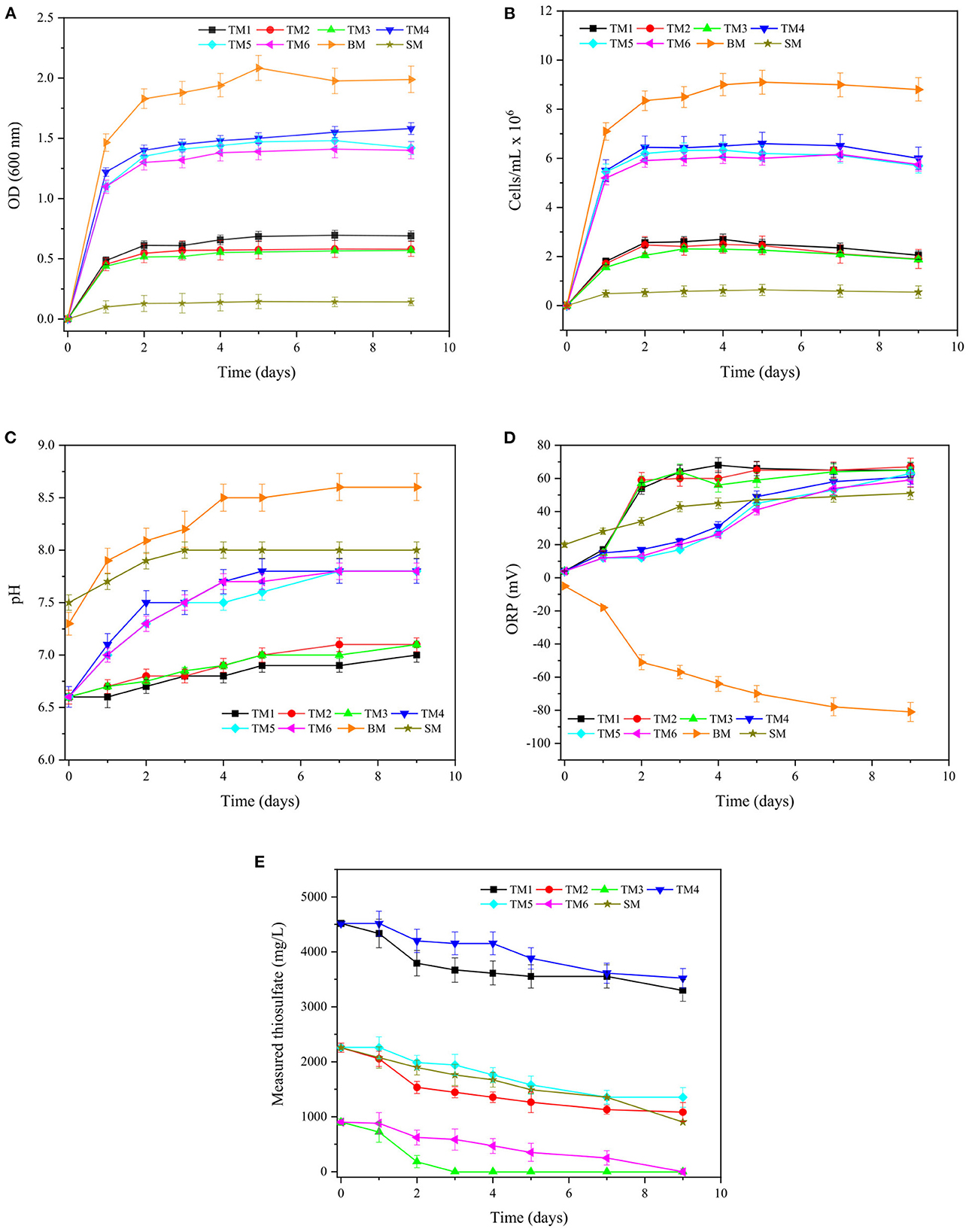
Figure 4. Changes in (A) OD600, (B) bacterial density (cells/mL), (C) pH, (D) ORP (mV), (E) measured thiosulfate (mg/L) over time for S. novella in culture media TM1–6, BM, and SM.
In the case of T. thioparus (Figure 3), a significant impact was produced by the change in the initial concentration of sodium thiosulfate in the culture medium, influencing the main parameters measured during its growth. From Figures 3A, B, the exponential phase of the bacterial growth was achieved after 3 days in all the experimental conditions, abruptly increasing optical density and bacterial density, correspondently. Optical density reflects the bacterial growth based on the turbidity of the bacterial solution (Widdel, 2010), which is normally determined at a wavelength of 600 nm in the case of the selected bacteria (Toth et al., 2015; Peighami et al., 2022). Thus, an increase in OD600 indicates that the bacterial population increases over time which is reflected in more turbid solutions compared to the original solution at day 0 in Figure 3. An increase in sodium thiosulfate concentration from 2 to 10 g/L led to a substantial rise of 2–3 folds in OD600 and bacterial density as thiosulfate is utilized as the principal energy source for the bacteria, favoring its metabolism at higher concentration in the range 2–10 g/L.
The more significant growth using 10 g/L sodium thiosulfate could be attributed to its metabolic characteristic since T. thioparus is an obligately chemolithotrophic bacteria that obtains its energy mainly from the oxidation of reduced sulfur compounds such as thiosulfate presented in the culture medium (Kelly et al., 2015). Reduced sulfur species are used as electron donors and oxygen is utilized as an electron acceptor to generate an electron flow in the cell membranes required to satisfy the bacterial metabolism through phosphorylation reactions (Kelly et al., 2015; Lee et al., 2021). Therefore, an increase in the concentration of sodium thiosulfate in the range 2–10 g/L seems to have helped to enhance the metabolism of T. thioparus, which resulted in an augment in OD600 > 0.6 with a bacterial density of 1.2 × 106 cells/mL.
A concentration higher than 10 g/L sodium thiosulfate was also employed to enhance the bacterial growth of T. thioparus. Nevertheless, a concentration of 20 g/L sodium thiosulfate (Supplementary Figure S2) had a negative impact, which seems to be related to a possible salinity stress generating a opposite effect on bacterial growth. Higher salinity in the medium affects the water balance of bacterial membranes due to its osmotic properties, which depends on the class of bacteria and environmental conditions (Boziaris et al., 2007; Kumar et al., 2021). Keller (1969) noted that the growth of T. thioparus ATCC 8158 was entirely inhibited at 0.3 M NaCl due to low tolerance to high saline environments.
Furthermore, Figures 3C, D show that a higher concentration of sodium thiosulfate (2–10 g/L) caused a more abrupt decrease in the solution pH and an increase in ORP during the evaluated period. A drop in pH was observed from initial pH 6.6 to pH 4.0–5.0 when the exponential phase of the growth was reached. ORP increased during the exponential phase and then remained in the range 130–230 mV depending on the initial sodium thiosulfate provided.
The decrease in pH and increase in ORP could be caused by the metabolism of thiosulfate during the bacterial growth and the release of protons into the medium by T. thioparus (Kelly, 2006; Tang et al., 2009). Thiosulfate was undetectable at the end of the bacterial growth in all the culture media as shown in Figure 3E. Control tests kept a near-neutral pH and ORP < 50 mV with insignificant changes in thiosulfate concentration compared with the test (data not shown), demonstrating that the T. thioparus was responsible for the changes observed during the evaluated interval. A concentration of 10 g/L sodium thiosulfate was preferred during the evaluation of its oxidative capacities of solid matrices due to a more robust growth was obtained under these conditions.
Figure 4 displays the bacterial growth of S. novella cultured in modified TM (Table 1), BM, and SM. S. novella is a facultative chemolithotrophic sulfur-oxidizing bacterium that can thrive using both organic and inorganic reagents to obtain energy required for its metabolism (Perez and Matin, 1980; Kelly and Wood, 2000). Media TM1–6 and SM favored a mixotrophic growth using organic and inorganic compounds, while medium BM supported a heterotrophic growth based on the utilization of only organic substrates.
The exponential phase of the bacterial growth was reached after 1–2 days of incubation in all the culture media as shown in Figure 4A, which resulted in an abrupt increase in OD in all media and thus more turbid solutions compared to the respective original culture medium at day 0 owing to a more significant number of microorganisms. In media TM1–6, the bacterial growth was increased almost 3 folds as the concentration of yeast extract was augmented from 0.3 to 5 g/L. Organic reagents such yeast extract, are used by S. novella as a source of carbon required for its growth (Kelly et al., 2000). In particular, yeast extract is a nourishing nutrient with a high diversity of proteins, amino acids, and minerals (Pasupuleti and Demain, 2010). Thus, adding a higher concentration of yeast extract in the culture medium could stimulate bacterial growth notably in terms of OD600 and bacterial density as shown in Figures 4A, B, respectively.
However, even though the bacterial growth of S. novella resulted in higher OD in culture media with 5 g/L yeast extract (TM4–6), the utilization of thiosulfate was slower compared to cultures in TM1–3 as illustrated in Figure 4E. The ability of S. novella to oxidize reduced sulfur species could be affected by an excess of yeast extract causing an inhibition of substrate utilization, as the microorganism might prefer other substrates for its metabolism such as organic reagents instead of thiosulfate (Leefeldt and Matin, 1980). When organic reagents were not provided in TM, the growth of S. novella was completely inhibited (data not shown) due to the requirement of organic reagents (Leefeldt and Matin, 1980; Kelly and Wood, 2000). Contrasting T. thioparus, the variation in sodium thiosulfate concentration in the range of 2–10 g/L in the modified TM1–6 was not an influential parameter for S. novella, producing a slight change in OD600 and bacterial density (Figures 4A, B).
Medium SM was also evaluated under similar conditions; nonetheless, the bacterial growth in OD600 and bacterial density was 4–6 times lower than TM1–6, which is reflected in the bacterial density (cells/mL). The difference between TM1–6 and SM seems to have been attributed to the addition of another exogenous organic matter in TM1–6, e.g., glucose, which has been noted to support bacterial metabolism and cell growth of S. novella (Rittenberg, 1969; Leefeldt and Matin, 1980; Kelly and Wood, 2000). The bacterial growth was also investigated in a pure organic medium (BM) containing trypticase soy broth, which is a substrate mainly composed of tryptone, a rich source of amino acid (Pasupuleti and Demain, 2010). This culture medium produced a noticeably more significant growth than TM1–6 and SM, which resulted in an OD600 > 2. However, the behavior of the bacteria under heterotrophic conditions could be different to mixotrophic growth due to dissimilar enzyme production as demonstrated by Aleem (1965) and Leefeldt and Matin (1980).
As shown in Figures 4C, D, pH and ORP generally increased during exponential phase and then stayed in the range 7.0–8.5 and 20–60 mV in most of the cases. Culture media containing yeast extract and tryptone showed an increase in pH due to the buffer capacity of these reagents, which seems to be associated with the decomposition of organic components and formation of alkaline products generated during bacterial growth (Losen et al., 2004). The increase in ORP in most of the culture media could be attributed to the metabolism of thiosulfate, as explained in the previous section. Additionally, it is worth mentioning that S. novella consumed up to 1,000 mg/L thiosulfate in all the experimental conditions, which was ca. 4 times lower than T. thioparus, causing a minor change in pH and ORP. Therefore, T. thioparus might have a higher potential to oxidize solid matrices than S. novella. Besides, the absence of thiosulfate in BM composition (not shown in Figure 4E) could have influenced the decreasing trend in ORP, reaching ca −80 mV at the end of the incubation.
Although all the culture media were tested for sulfide oxidation, TM3 was preferred in future experimentation with solid matrices since S. novella metabolized most of the initial thiosulfate presented in the solution after the exponential compared to the other cases, which could lead to increase the possibilities of oxidizing the sulfidic ore.
Bio-oxidation tests with elemental sulfur
A preliminary assessment was performed with elemental sulfur (S°) to explore the oxidative capacities of T. thioparus and S. novella on solid matrices. Although there is no extensive research on S° oxidation with these specific microorganisms, neutrophilic bacteria such as T. thioparus and S. novella have been commonly encountered in soils, showing a more notorious activity in soils containing S° due to their bacterial metabolism (Deluca et al., 1989; Chapman, 1990; Germida and Janzen, 1993; Pourbabaee et al., 2020).
Bio-oxidation tests with S° were used as a baseline for subsequent bio-oxidation tests with sulfidic ores. Two different approaches were implemented to confirm the ability of both microorganisms to oxidize S°. On the one hand, the bacteria were first grown with thiosulfate as an initial energy source until the exponential phase was achieved, and subsequently, 5,000 mg/L S° was added into the reactor. On the other hand, the bacteria were directly grown with S° (5,000 mg/L) as sole energy source material with no addition of thiosulfate in the medium. Figure 5 shows a comparison between both strategies along with the control tests for T. thioparus and S. novella.
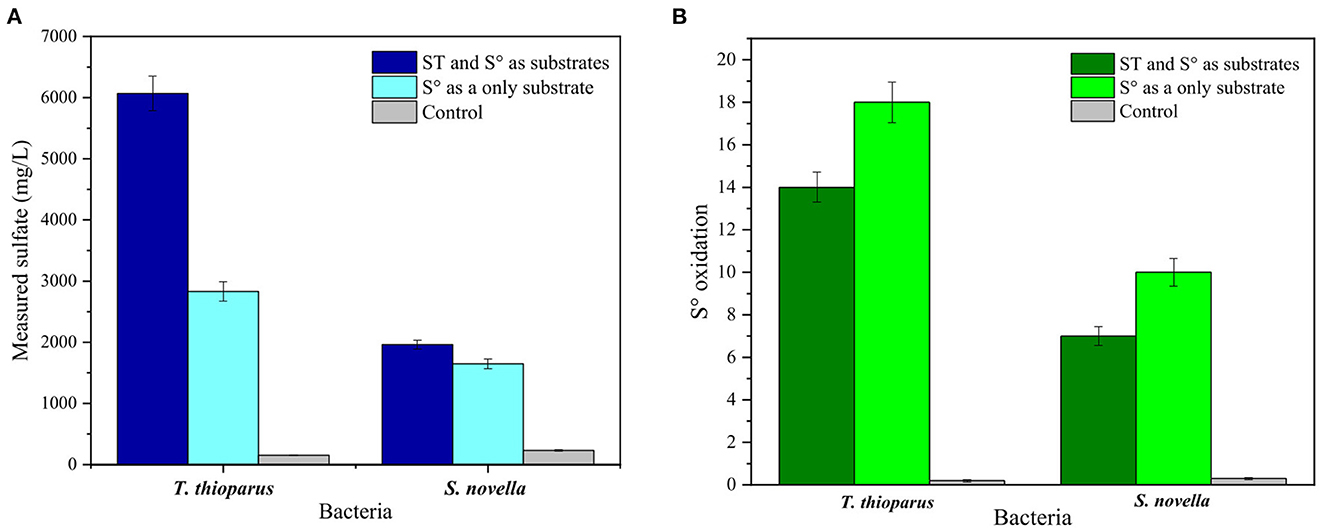
Figure 5. Bio-oxidation tests with 5,000 mg/L elemental sulfur (S°). (A) Measured sulfate, (B) S° oxidation rate. T. thioparus cultured in TM with 10 g/L sodium thiosulfate (ST) and S. novella cultured in TM3 with 2 g/L ST.
Thiobacillus thioparus showed more significant potential than S. novella in oxidizing S° using both approaches. When S° was used as the only substrate, 2,830 mg/L sulfate was measured in the presence of T. thioparus, which was almost 2 folds more sulfate compared to S. novella under similar conditions. A total S° oxidation rate of ca. 18% was oxidized by T. thioparus after 10 days of reaction time, while 10% of total S° were oxidized by S. novella (Figure 5B). During the tests with S° as the only energy source, T. thioparus increased solution acidity to pH 4, while S. novella maintained a near-neutral pH (data not shown). Chapman (1990) found that the addition of S° increased T. thioparus bacterial density, reaching 105 cells/g soil and more acid pH solution, and Lindemann et al. (1991) showed similar results with the same microorganism. In another study, Boretska and Bellenberg (2013) pointed out that S° stimulated T. thioparus to produce different extracellular polymeric substances (EPS), improving bacterial attachment in solid substrates. On the other hand, Pourbabaee et al. (2020) reported a closely related strain to S. novella that produced ca. 12–15% S oxidation with 0.3-unit pH variation over 2 weeks, which agreed with the results shown in Figure 5B.
By using thiosulfate as an initial substrate for bacterial growth before the addition of S°, T. thioparus formed ca. 6,000 ppm (Figure 5A). However, the oxidation rate of S° was 14%, which was slightly lower than the previous approach. The decrease in oxidation could be produced by the presence of thiosulfate, which also served as a source of energy for the bacterium (Kelly et al., 2015). S. novella cultured with 2 g/L sodium thiosulfate in TM3 showed that ca. 7% of the initial S° was oxidized in the medium. Blank tests showed a negligible formation of sulfate in the range 150–250 mg/L, which could be caused by the presence of traces of sulfate salts in the culture medium and minor chemical oxidation. The oxidation of S° produced by abiotic factors has been noticed to be limited in soils compared to biological reactions (Germida and Janzen, 1993), which agreed with the results displayed in Figure 5.
From Figure 5, it is clear that both microorganisms are able to oxidize solid matrices, achieving a maximum of 18% oxidation with T. thioparus over 10 days. Although there was no complete oxidation of S°, the sulfur content of the refractory gold ore used in this study is 5.7%, which is lower than the amount of S° utilized in this section. Thus, this opens an option to achieve higher oxidation when working with the refractory sulfidic ore compared to S°.
The surface morphology of S° before and after bio-oxidation was evaluated with SEM images (Figure 6). The surface of the S° before bio-oxidation was characterized by a smooth texture, and the S° surface obtained after sterile control showed subtle surface alterations compared to the as-received S° sample (Figures 6A, B). Liu et al. (2013) also confirmed that the S° surface in control tests remained almost unchanged and that the roughness of the S° surface was produced by bacterial action.
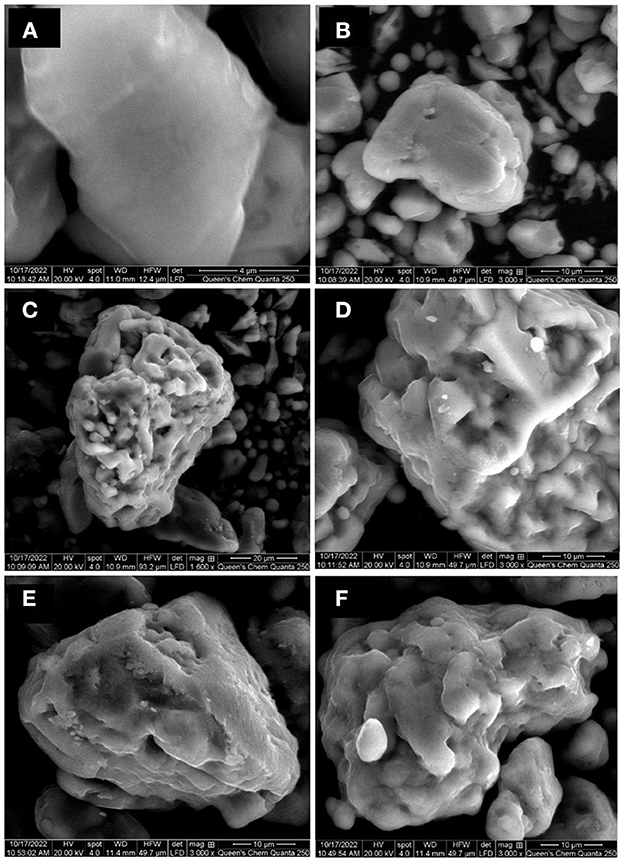
Figure 6. SEM images of elemental sulfur as received (A), sterile control test T. thioparus (B), after bio-oxidation using T. thioparus (C, D), and after bio-oxidation using S. novella (E, F).
Figures 6C–F show that the S° surface after contact with T. thioparus and S. novella became rougher compared to as received S° (Figure 6A). It was observed that T. thioparus led to more severe corrosion than S. novella, with more alteration and pits. The changes on S° surface were similar to the alteration produced by common acidophilic microorganisms (Briand et al., 1999). The bacterial action was generally irregular on the S° surface, causing non-uniform distortions (Espejo and Romero, 1987), which was less noticeable in the bio-oxidation test with S. novella due to the lower oxidation rate.
The more remarkable bacterial alteration produced by T. thioparus seems to be related to the higher sulfate production compared to S. novella. Briand et al. (1999) also noted more corroded surfaces led to a higher sulfate generation due to S° utilization in average 10–14 mmol/day. However, the sulfur consumption rate with neutrophilic bacteria was observed to be lower, which was reflected in the less corroded surfaces compared to acidophilic bacteria such a T. thiooxidans and A. ferroxidans.
Bio-oxidation tests with refractory sulfidic ore
Effect of culture medium on bio-oxidation tests
After confirming that the microorganisms were capable of oxidizing S°, the bio-oxidation of refractory sulfidic ore with both neutrophilic bacteria was further explored using selected culture media. Figure 7 displays 10-day bio-oxidation tests of refractory sulfidic ores. Different concentrations of sodium thiosulfate in the culture media were tested to promote the biological oxidation of sulfide in the sulfidic ore (Mubarok et al., 2017).
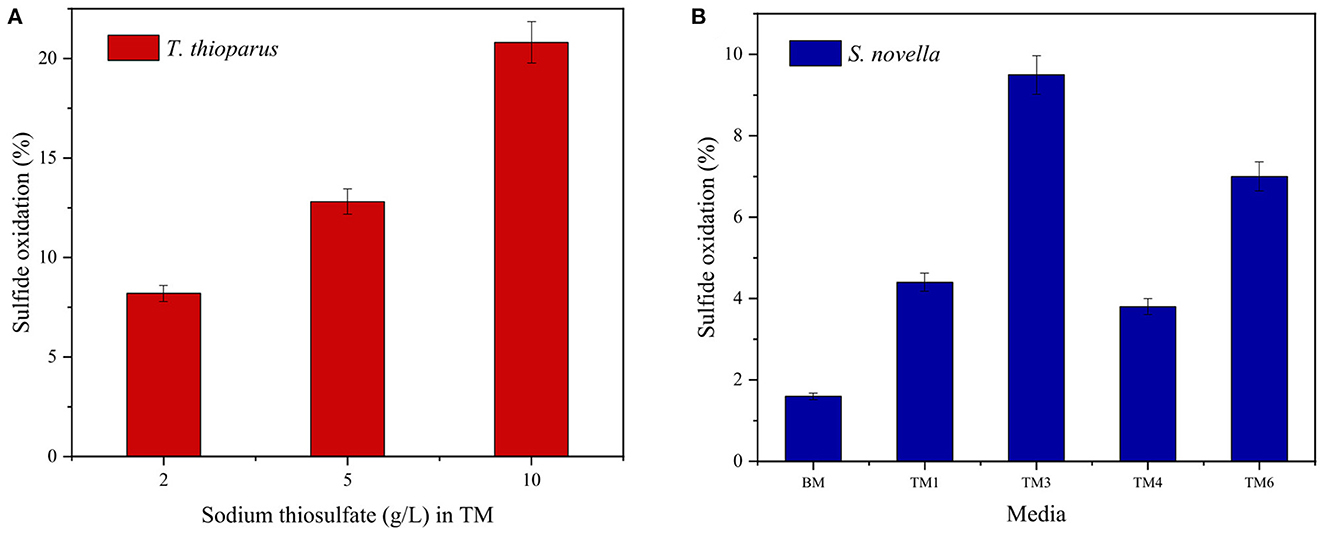
Figure 7. Effect of culture medium on 10-day bio-oxidation tests with (A) T. thioparus, and (B) S. novella using S/L = 10, 30°C and 150 RPM.
From Figure 7, T. thioparus displayed a higher potential in bio-oxidation of sulfidic ores than S. novella showing the same trend observed with S° with ca. 50% higher oxidation rate. T. thioparus increased the oxidation rate from 8.2 to 20.8% when the initial concentration of sodium thiosulfate in TM augmented from 2 to 10 g/L. Contrary to Mubarok et al. (2017), a higher sodium thiosulfate concentration in the culture medium led to a higher oxidation rate. The initial bacterial growth of T. thioparus mainly depended on the initial concentration of sodium thiosulfate, which was almost consumed during the exponential phase growth. Therefore, a concentration of 10 g/L resulted in a bacterial density of 1.2 × 106 cells/mL, which was 2 times higher compared to TM with 2 g/L sodium thiosulfate. Sajjad et al. (2019) pointed out that a greater bacterial density might result in a higher bio-oxidation rate, which agreed with the results found in Figure 7A. Control tests in TM with varied concentration sodium thiosulfate resulted in negligible oxidation < 1% (data not shown).
Contrary to T. thioparus, 2 g/L sodium thiosulfate favored the oxidation of the sulfidic ore with S. novella (Figure 7B), which resulted in 9.5 (TM3) and 7.9% (TM6) as suggested by Mubarok et al. (2017). BM, a nutrient-rich medium composed of trypticase soy broth, had a negligible sulfide oxidation rate despite the fact that this medium generated the highest OD and bacterial density compared to other culture media. An excess of nutrients in the culture medium could inhibit substrate utilization affecting the oxidative capacities of the bacteria and enzyme production. In a medium with an excess of nutrients, it might be possible that microorganisms have enough resources in terms of thiosulfate and organic reagents to thrive in the medium, decreasing the probability of oxidizing the ore. (Leefeldt and Matin, 1980; Mubarok et al., 2017). All the control tests also showed insignificant sulfur oxidation compared to tests with S. novella (data not shown).
Effect of modification of experimental parameters on bio-oxidation tests
Experimental parameters such as the solid to liquid ratio (g/L), bio-oxidation approach, mixing speed (RPM), and time of reaction (days) were further investigated, and the results are summarized in Figure 8. By augmenting the solid-to-liquid ratio from 10 to 100, sulfide oxidation using T. thioparus decreased from 20.8 to 3.8% over 10 days. S. novella showed a similar trend, decreasing oxidation rate from 9.5 to 1.6%, as shown in Figure 8A. The effect of higher solid-to-liquid ratios in bacterial performance has been addressed to possible poor mass transfer and heavier environmental stress due to higher concentrations of solids and heavy metals presented in the tested samples, affecting the bacterial performance and resulting in lower oxidation (Azizitorghabeh et al., 2022).
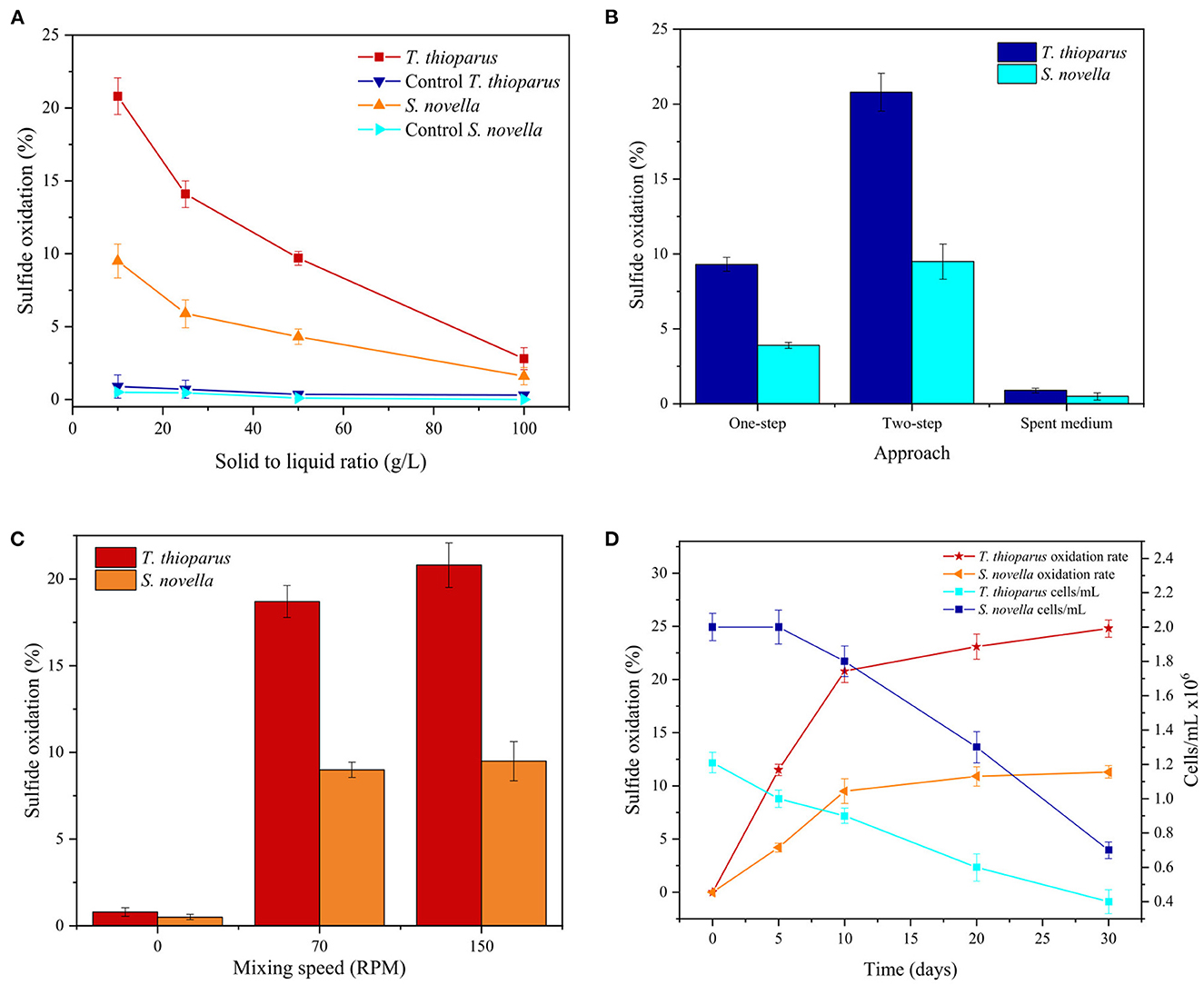
Figure 8. Effect of (A) solid to liquid ratio, (B) bio-oxidation approach, (C) mixing speed (RPM), and (D) extended experimental time on bio-oxidation tests with T. thioparus in TM and S. novella in TM3 at 30°C.
Furthermore, different approaches were explored for bio-oxidation including one-step, two-step and spent medium. Two-step bio-oxidation was used in most of the tests carried out in this investigation. However, there are other specific approaches to conducting tests with microorganisms, encompassing one-step and spent medium techniques (Faraji et al., 2018). Figure 8B compares each method using both bacteria. It was noted that one-step tests produced approximately half the sulfide oxidation compared to two-step tests. The decrease in oxidation rate could be caused by the lower concentration of microorganisms at the beginning of one-step test contracting with two-step test with an initial population >106 cells/ mL (Sajjad et al., 2019). In the spent medium technique, tests were carried out only with cell-free metabolites generated during the bacterial growth, which might include extracellular polymeric substances (EPS) of both bacteria (Gehrke et al., 1998). This approach was also more ineffective than the two-step methodology as sulfide oxidation was similar to the control tests, implying that microorganisms form part of the oxidation mechanism as reported in standard bio-oxidation using acidophilic bacteria (Azizitorghabeh et al., 2022).
Bacterial attachment to the sulfidic ore surface is essential in the bio-oxidation mechanism with microorganisms. A proper bacterial attachment influences biofilm formation, which is a protective barrier for cells during bio-oxidation (Boretska and Bellenberg, 2013). Thus, it is likely that bacterial attachment also has a predominant role in the case of neutrophilic bacteria. Bio-oxidation tests using < 150 RPM were proposed to improve the adherence of cells to the refractory sulfidic ore, and the results are displayed in Figure 8C. Nevertheless, the results revealed that a decrease in mixing speed was an ineffective parameter in boosting biological oxidation with T. thioparus and S. novella. The reduction of mixing speed could lead to bacterial sedimentation into the bottom of the reactor affecting the circulation of microorganisms and proper aeration of the culture medium, thus preventing an adequate mixture with the medium and substrates (Bates et al., 2016).
Moreover, experimental time is also an essential parameter in increasing sulfide oxidation biologically (Sajjad et al., 2019). Figure 8D shows the effect of time in bio-oxidation and bacterial population in 30 days. The results revealed that sulfide oxidation increased markedly within the initial 10 days, reaching 20.8 and 9.5% sulfide oxidation for T. thioparus and S. novella, respectively. Subsequently, sulfide oxidation rate was stalled resulting ca. 24.8 and 11.3% sulfide oxidation correspondingly within 30 days reaction. It was also noted that the bacterial density of both microorganisms decreased >50% with 30 days which might also affected the oxidation of sulfide (Sajjad et al., 2019). The deceleration in sulfide oxidation rate could also be caused by a depletion of nutrients and environmental conditions e.g., decrease in pH during more extended periods influencing bacterial population (Li et al., 2016; Bulaev, 2020) as well as precipitation of iron phases on the surface of the sulfidic ore over time (Ofori-Sarpong et al., 2011).
Optimization and scale-up of reactions
Following bio-oxidation conducted in shake flasks, reactions were scaled up in a 1 L bioreactor, and Table 4 compares both type of experiments using both microorganisms. By using the bioreactor, sulfide oxidation with T. thioparus showed an increase from 20.8 to 27.2% with 10 days reaction time. Moreover, S. novella followed a similar tendency by augmenting sulfide oxidation from 9.5 to 14%, as displayed in Table 4. The bacterial density of both cultures also displayed an increase in the population of ca. 20% as well as more fluctuation in pH and ORP compared to shake flask tests (Table 4). Control tests in the bioreactor resulted in minor oxidation with negligible changes in measured parameters compared to shake flask control tests, confirming that the enhancement observed was biologically caused. The improvement in bacterial growth and sulfide oxidation could be attributed to the agitation mode in the bioreactor, which may have enhanced the fluid dynamics of the system favoring the heat and mass transfer in the culture as well as oxygen and nutrient supply availability due to better homogenization of the culture solution (Büchs, 2001; Eppendorf, 2018). Although the bioreactor promoted greater oxidation, acidophilic bacteria can still provide more effective oxidation. However, some strategies to increase oxidation could optimize a future process with neutrophilic bacteria, including replenishing the culture medium, simulating a continuous operation (Do et al., 2022), and incorporating a reactor equipped with multiple online sensors for real-time process control, encompassing nutrients and pH over time (Eppendorf, 2018).
Regarding the bacterial action, the oxidation mechanism of reduced sulfur species varies depending on the physiological characteristics of each bacterium, which can oxidize various reduced sulfur compounds with varied kinetics. Generally, sulfur-oxidizing bacteria obtain energy from the oxidation of reduced sulfur compounds, using oxygen as electron donor, which form a gradient of electrons in the cell membrane that meditates phosphorylation reactions to generate energy as adenosine triphosphate (ATP) (Lee et al., 2021). The bacterial oxidation reactions are generally meditated by the catalytic action of extracellular enzymes secreted by the bacterium.
Regarding T. thioparus, the oxidation of reduced sulfur compounds proceeds through a chain of enzymatically mediated reactions, which might include the production of intermediate species such as sulfite and elemental sulfur with sulfate as final product. The oxidation with T. thioparus could involve the action of enzymes including but not limited to thiosulfate reductase, APS (adenosine phosphosulfate) reductase, AMP (adenosine monophosphate), ADP (adenosine diphosphate) sulfurylase, ATP (adenosine triphosphate) sulfurylase, and adenylate kinase (Skarzynski and Sczczepkowski, 1959; Chung et al., 1996; Kelly, 2006). Thus, sulfide oxidation could include an enzymatically meditated pathway that could consist of forming intermediate species, which can result subsequently in sulfate formation (Schippers et al., 1996, 2000) The oxidative mechanism of S. novella has been less investigated than T. thioparus. Still, it has been pointed out that it could have a mechanism encompassing soluble enzymes, similar to T. thioparus, and/or through multienzymes pathways oxidizing reduced sulfur species to sulfate (Yamanaka, 1996; Kappler et al., 2001).
The surface morphology of the sulfidic ore pre- and post-oxidative treatment was analyzed with SEM images (Figure 9). The surface of the as-received ore sample showed a smooth surface with no pits and cracks, as shown in Figure 9A. The surface of the sulfidic ore after the control test also displayed slight alterations, showing a similar morphology to the as-received sample. After the bio-oxidation pretreatment with both neutrophilic microorganisms, the surface of the sulfidic ore adopted a rougher appearance (Figures 9C–F). In the same way as S° SEM analysis in Figure 6, T. thioparus also led to greater biotransformation of the ore surface than S. novella, displaying a rougher surface and a more significant number of pits, which agreed with the higher oxidation rate obtained with T. thioparus. The pits identified after bio-oxidation with both strains have also been observed in bio-oxidation using acidophilic microorganisms (Azizitorghabeh et al., 2022). Nevertheless, the number of pits and degree of biotransformation was minor compared to the microorganisms commonly used in bio-oxidation at acidic pH (Li et al., 2016).
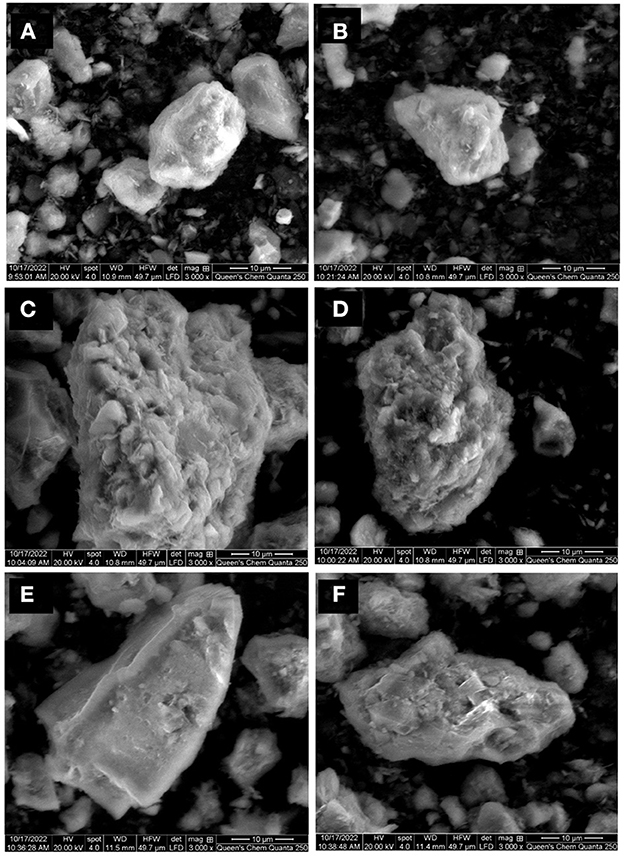
Figure 9. SEM images of refractory sulfidic ore as received (A), sterile control test T. thioparus (B), after bio-oxidation using T. thioparus (C, D), and after bio-oxidation using S. novella (E, F).
Literature comparison
Recent investigations comprising neutrophilic microorganisms are summarized in Table 5. Vázquez-Rodríguez et al. (2015) explored mercury (Hg) emission from sediment through the dissolution of mercury sulfide (HgS) employing T. thioparus. The study revealed that T.thioparus was able to use HgS as the sole energy source in replacement of thiosulfate and the sulfate generation reached ca. 0.2 mM, which was 9–1,000 times greater than naturally occurring conditions. In another study, Percak-Dennett et al. (2017) evaluated pyrite oxidation employing indigenous bacteria isolated from pyrite-containing sediments identified as Rhizobiales species and Ralstonia species and obtained overall 20% sulfide oxidation after 30 days. More recently, Purnomo et al. (2019) implemented a 5-day bio-oxidation pretreatment using C. youngae to improve gold recovery from gold tailings containing 0.53% w/w total S. The outcome of the study was an enhancement in gold recovery by 6.1%. Compared with the recent studies, sulfide oxidation rates were similar in most investigations. However, higher solid-to-liquid ratios were chosen in this study, which could lead to higher sulfide oxidation rate considering lower solid-to-liquid ratios used in other investigations. In addition, the reaction time was 3 to 7 times lower than most other studies, showing higher potential for T. thioparus and S. novella under similar experimental conditions. Even though this study demonstrated that both bacteria are able to bio-transform S in different matrices, T. thioparus would be a better option to continue this investigation owing to its better performance compared to S. novella. There is still a more evident need for further research in the case of S. novella to improve its catalytic action to oxidize sulfur matrices.
Conclusion
This study showed the potential of T. thioparus and S. novella in oxidizing solid matrices. The bacterium T. thioparus displayed better oxidation in a culture medium with 4.48 g/L thiosulfate with an OD600(max) = 0.6. Contrarily, S. novella showed a better performance with a lower thiosulfate concentration (0.9 g/L) due to its metabolic characteristics, requiring organic reagents (1.5 g/L glucose and 0.3 g/L yeast extract) with a OD600(max) = 0.5. In bio-oxidation tests with pure S°, the microorganisms could use S° as a sole energy source, replacing the requirement of thiosulfate for its growth. The oxidation of S° with T. thioparus and S. novella reached 18% and 10% within 10 days. Subsequently, bio-oxidation using the low-grade refractory sulfidic ores containing 5.7% S in shake flasks showed 20.8 and 9.5% sulfide oxidation for T. thioparus and S. novella, respectively, within 10 days. The bio-oxidation results indicated that bacteria oxidize S° more easily than S in the refractory ore. An extended experimental time of 30 days led to a ca. 25% sulfide oxidation with T. thioparus and 11.3% sulfide oxidation with S. novella. The implementation of a bioreactor positively increased sulfide oxidation with both microorganisms, reaching 27.2 and 14% for T thioparus and S. novella, respectively. SEM analysis revealed that the bacterial action led to rougher surfaces and pits in the ore. The comparative study showed that T. thioparus and S. novella performed better than other bacteria using higher pulp densities and shortened experimental time. We demonstrated the potential of both microorganisms to oxidize refractory sulfidic ores, which can inspire future investigation toward an effective bio-oxidation process at higher pH with a net-zero target in the mining industry.
Data availability statement
The raw data supporting the conclusions of this article will be made available by the authors, without undue reservation.
Author contributions
GH: investigation, data curation, resources, and writing—original draft. HM: conceptualization, supervision, and writing—review and editing. AG: conceptualization, project administration, supervision, and writing—review and editing. All authors contributed to the article and approved the submitted version.
Acknowledgments
The authors are thankful to MITACS and COREM for providing the financial support to prepare this article through MITACS Accelerate Fund IT13524.
Conflict of interest
The authors declare that the research was conducted in the absence of any commercial or financial relationships that could be construed as a potential conflict of interest.
Publisher's note
All claims expressed in this article are solely those of the authors and do not necessarily represent those of their affiliated organizations, or those of the publisher, the editors and the reviewers. Any product that may be evaluated in this article, or claim that may be made by its manufacturer, is not guaranteed or endorsed by the publisher.
Supplementary material
The Supplementary Material for this article can be found online at: https://www.frontiersin.org/articles/10.3389/fsrma.2023.1102488/full#supplementary-material
References
Abcam (2022). Counting Cells Using a Hemocytometer. Waltham: Abcam. Available online at: https://www.abcam.com/protocols/counting-cellsusing-a-haemocytometer (accessed October 3, 2022).
Adams, M. D. (2016). Gold Ore Processing Project Development and Operations, 2nd Edn. Singapore: Elsevier.
Aleem, M. I. H. (1965). Thiosulfate oxidation and electron transport in T. novellus. J. Bacteriol. 90, 95–101. doi: 10.1128/jb.90.1.95-101.1965
Alken-Murray (2006). Hydrogen Sulfide Odor Control and General Industrial Waste Treatment. Virgina: Alken-Murray. Available online at: https://www.alken-murray.com/EZ8pib.htm (accessed October 3, 2022).
Arima, H., Fujita, T., Yen, W. T. (2004). Using nickel as a catalyst in ammonium thiosulfate leaching for gold extraction. Mater. Trans. 45, 516–526. doi: 10.2320/matertrans.45.516
Astudillo, C., Acevedo, F. (2008). Adaptation of Sulfolobus metallicus to high pulp densities in the biooxidation of a flotation gold concentrate. Hydrometallurgy. 92, 11–15. doi: 10.1016/j.hydromet.2008.02.003
Aswegen, P. C., Niekerk, J., Waldemar, O., Olivier, W. (2007). “The BIOXTM process for the treatment of refractory,” in Biomining, eds D. E. Rawlings, and D. B. Johnson (Berlin: Springer), 1–33. doi: 10.1007/978-3-540-34911-2_1
Azizitorghabeh, A., Mahandra, H., Ramsay, J., Ghahreman, A. (2022). A sustainable approach for gold recovery from refractory source using novel BIOX-TC system. J. Ind. Eng. Chem. 115, 209–218. doi: 10.1016/j.jiec.2022.08.002
Bates, M. K., Phillips, D. S., O'Bryan, J. (2016). The Effect of Shaker Agitation Rate and Orbit on Growth of Cultured Bacteria. Frederick: Thermo Fisher Scientific ANSHORBGRT. Available online at: https://shop.haslab.ch/H179/DWN/d02594_pdf (accessed October 3, 2022).
Biorem Technology Inc (2015). Biofiltration for Odour Control. Puslinch: Biorem Technology Inc. Available online at: https://www.crd.bc.ca/docs/defaultsource/Wastewater-Planning-2014/TOP/biorem.pdf?sfvrsn=68ea5eca_0 (accessed October 3, 2022).
Boretska, M., Bellenberg, S. (2013). Change of extracellular polymeric substances composition of T. thioparus in presence of sulfur and steel. J. Microb. Biochem. Technol. 05, 68–73. doi: 10.4172/1948-5948.10000102
Boziaris, I. S., Skandamis, P. N., Anastasiadi, M., Nychas, G. J. E. (2007). Effect of NaCl and KCl on fate and growth/no growth interfaces of Listeria monocytogenes Scott A at different pH and nisin concentrations. J. Appl. Microbiol. 102, 796–805. doi: 10.1111/j.1365-2672.2006.03117.x
Briand, L. E., Bonetto, R. D., Ladaga, J. L., Donati, E. (1999). Bulk and surface characterization of crystalline and plastic sulphur oxidized by two Thiobacillus species. Process Biochem. 34, 249–256. doi: 10.1016/S0032-9592(98)00091-0
Büchs, J. (2001). Introduction to advantages and problems of shaken cultures. Biochem. Eng. J. 7, 91–98. doi: 10.1016/S1369-703X(00)00106-6
Bulaev, A. G. (2020). Effect of organic carbon source on pyrite biooxidation by moderately thermophilic acidophilic microorganisms. Microbiology. 89, 301–308. doi: 10.1134/S0026261720030145
Chapman, S. J. (1990). Thiobacillus populations in some agricultural soils. Soil Biol. Biochem. 22, 479–482. doi: 10.1016/0038-0717(90)90181-X
Chung, Y., Huang, C., Tseng, C. P. (1996). Operation optimization of T. thioparus CH11 biofilter for hydrogen sulfide removal. J. Biotechnol. 52, 31–38. doi: 10.1016/S0168-1656(96)01622-7
Darvanjooghi, M. H. K., Magdouli, S., Brar, S. K., Abdollahi, H., Zolfaghari, M. (2022). Bio-oxidation of gold from refractory sulfide ores: a journey ahead. Geomicrobiol. J. 39, 399–415. doi: 10.1080/01490451.2021.1977431
de Carvalho, L. C., da Silva, S. R., Giardini, R. M. N., de Souza, L. F. C., Leão, V. A. (2019). Bio-oxidation of refractory gold ores containing stibnite and gudmundite. Environ. Technol. Innov. 15, 100390. doi: 10.1016/j.eti.2019.100390
Deluca, T. H., Skogley, E. O., Engel, R. E. (1989). Band-applied elemental sulfur to enhance the phytoavailability of phosphorus in alkaline calcareous soils. Biol. Fertil. Soils. 7, 346–350. doi: 10.1007/BF00257831
Do, M. P., Jegan Roy, J., Cao, B., Srinivasan, M. (2022). Green closed-loop cathode regeneration from spent NMC-based lithium-ion batteries through bioleaching. ACS Sustain. Chem. Eng. 10, 2634–2644. doi: 10.1021/acssuschemeng.1c06885
Eppendorf (2018). It's Not Just About Size: Talking About Shake Flasks and Bioreactors. Hamburg: Eppendorf. Available online at: https://handling-solutions.eppendorf.com/cell-handling/bioprocess/processes-and-applications/detailview/news/its-not-just-about-size-talking-about-shake-flasks-and-bioreactors/ (accessed October 3, 2022).
Espejo, R. T., Romero, P. (1987). Growth of Thiobacillus ferrooxidans on elemental sulfur. Appl. Environ. Microbiol. 53, 1907–1912. doi: 10.1128/aem.53.8.1907-1912.1987
Faraji, F., Golmohammadzadeh, R., Rashchi, F., Alimardani, N. (2018). Fungal bioleaching of WPCBs using Aspergillus niger: observation, optimization and kinetics. J. Environ. Manage. 217, 775–787. doi: 10.1016/j.jenvman.2018.04.043
Gehrke, T., Telegdi, J., Thierry, D., Sand, W. (1998). Importance of extracellular polymeric substances from Thiobacillus ferrooxidans for bioleaching. Appl. Environ. Microbiol. 64, 2743–2747. doi: 10.1128/AEM.64.7.2743-2747.1998
Germida, J. J., Janzen, H. H. (1993). Factors affecting the oxidation of elemental sulfur in soil. Fertil. Res. 35, 101–114. doi: 10.1007/BF00750224
Kaksonen, A. H., Mudunuru, B. M., Hackl, R. (2014b). The role of microorganisms in gold processing and recovery: a review. Hydrometallurgy 142, 7083. doi: 10.1016/j.hydromet.2013.11.008
Kaksonen, A. H., Perrot, F., Morris, C., Rea, S., Benvie, B., Austin, P., et al. (2014a). Evaluation of submerged bio-oxidation concept for refractory gold ores. Hydrometallurgy 141, 117–125. doi: 10.1016/j.hydromet.2013.10.012
Kappler, U., Friedrich, C. G., Trüper, H. G., Dahl, C. (2001). Evidence for two pathways of thiosulfate oxidation in S. novella (formerly T. novellus). Arch. Microbiol. 175, 102–111. doi: 10.1007/s002030000241
Keller, P. (1969). The effect of some salts on T. thioparus. Can. J. Microbiol. 15, 314–318. doi: 10.1139/m69-058
Kelly, D. P. (2006). “Microbial inorganic sulfur oxidation: the APS pathway,” in Biochemistry and Physiology of Anaerobic Bacteria, eds L. G. Ljungdahl, M. W. Adams, L. L. Barton, J. G. Ferry, and M. K. Johnson (New York, NY: Springer), 205–219. doi: 10.1007/0-387-22731-8_15
Kelly, D. P., McDonald, I. R., Wood, A. P. (2000). Proposal for the reclassification of T. novellus as S. novella gen. nov., comb. nov., in the α-subclass of the proteobacteria. Int. J. Syst. Evol. Microbiol. 50, 1797–1802. doi: 10.1099/00207713-50-5-1797
Kelly, D. P., Wood, A. P. (2000). Reclassification of some species of Thiobacillus to the newly designated genera Acidithiobacillus gen. nov., Halothiobacillus gen. nov. and Thermithiobacillus gen. nov. Int. J. Syst. Evol. Microbiol. 50, 511–516. doi: 10.1099/00207713-50-2-511
Kelly, D. P., Wood, A. P., Stackebrandt, E. (2015). Thiobacillus. Bergey's Man. Syst. Archaea Bact. 14, 1–10. doi: 10.1002/9781118960608.gbm00969
Kumar, A., Singh, S., Mukherjee, A., Rastogi, R. P., Verma, J. P. (2021). Salt-tolerant plant growth-promoting Bacillus pumilus strain JPVS11 to enhance plant growth attributes of rice and improve soil health under salinity stress. Microbiol. Res. 242, 126616. doi: 10.1016/j.micres.2020.126616
Lee, J., Mahandra, H., Hein, G. A., Ramsay, J., Ghahreman, A. (2021). Toward sustainable solution for biooxidation of waste and refractory materials using neutrophilic and alkaliphilic microorganisms: a review. ACS Appl. Biol. Mater. 4, 2274–2292. doi: 10.1021/acsabm.0c01582
Leefeldt, R. H., Matin, A. (1980). Growth and physiology of T. novellus under nutrient-limited mixotrophic conditions. J. Bacteriol. 142, 645–650. doi: 10.1128/jb.142.2.645-650.1980
Li, J., Lu, J., Lu, X., Tu, B., Ouyang, B., Han, X., et al. (2016). Sulfur transformation in microbially mediated pyrite oxidation by Acidithiobacillus ferrooxidans: insights from X-ray photoelectron spectroscopy-based quantitative depth profiling. Geomicrobiol. J. 33, 118–134. doi: 10.1080/01490451.2015.1041182
Lindemann, W. C., Aburto, J. J., Haffner, W. M., Bono, A. A. (1991). Effect of sulfur source on sulfur oxidation. Soil Sci. Soc. Am. J. 55, 85–90. doi: 10.2136/sssaj1991.03615995005500010015x
Liu, H. C., Xia, J. L., Nie, Z. Y., Peng, A. A., Ma, C. Y., Zheng, L., et al. (2013). Comparative study of sulfur utilization and speciation transformation of two elemental sulfur species by thermoacidophilic Archaea Acidianus manzaensis YN-25. Process Biochem. 48, 1855–1860. doi: 10.1016/j.procbio.2013.09.005
Losen, M., Frölich, B., Pohl, M., Büchs, J. (2004). Effect of oxygen limitation and medium composition on Escherichia coli fermentation in shake-flask cultures. Biotechnol. Prog. 20, 1062–1068. doi: 10.1021/bp034282t
Marzoughi, O., Li, L., Pickles, C., Ghahreman, A. (2022). Regeneration and sulfur recovery of Lanxess Lewatit AF 5 catalyst from the acidic Albion leaching process using toluene and tetrachloroethylene as organic solvents. J. Ind. Eng. Chem. 107, 291–301. doi: 10.1016/j.jiec.2021.11.055
McNeice, J., Mahandra, H., Ghahreman, A. (2022). Biogenesis of thiosulfate in microorganisms and its applications for sustainable metal extraction. Rev. Environ. Sci. Biotechnol. 14, 1–23. doi: 10.1007/s11157-022-09630-3
Moses, C. O., Herman, J. S. (1991). Pyrite oxidation at circumneutral pH. Geochim. Cosmochim. Acta. 55, 471–472, doi: 10.1016/0016-7037(91)90005-P
Moses, C. O., Kirk Nordstrom, D., Herman, J. S., Mills, A. L. (1987). Aqueous pyrite oxidation by dissolved oxygen and by ferric iron. Geochim. Cosmochim. Acta. 51, 1561–1571. doi: 10.1016/0016-7037(87)90337-1
Mubarok, M. Z., Winarko, R., Chaerun, S. K., Rizki, I. N., Ichlas, Z. T. (2017). Improving gold recovery from refractory gold ores through biooxidation using iron-sulfur-oxidizing/sulfur-oxidizing mixotrophic bacteria. Hydrometallurgy 168, 69–75. doi: 10.1016/j.hydromet.2016.10.018
Ofori-Sarpong, G., Osseo-Asare, K., Tien, M. (2011). Fungal pretreatment of sulfides in refractory gold ores. Miner. Eng. 24, 499–504. doi: 10.1016/j.mineng.2011.02.020
Pasupuleti, V., Demain, A. (2010). Protein Hydrolysates in Biotechnology, 1st Edn. New York, NY: Springer. doi: 10.1007/978-1-4020-6674-0
Peighami, R., Rasekh, B., Motamedian, E., Yazdian, F., Khodaverdi, H. (2022). Investigating the ability of sulfur oxidizing strains in biodesulfurisation of sulfide-containing streams, screening the most capable strain and determining the optimum condition for elemental sulfur recovery from sulfide using response surface method. Fuel 309, 121985. doi: 10.1016/j.fuel.2021.121985
Percak-Dennett, E., He, S., Converse, B., Konishi, H., Xu, H., Corcoran, A., et al. (2017). Microbial acceleration of aerobic pyrite oxidation at circumneutral pH. Geobiology. 15, 690–703. doi: 10.1111/gbi.12241
Perez, R. C., Matin, A. (1980). Growth of T. novellus on mixed substrates (Mixotrophic growth). J. Bacteriol. 142, 633–638. doi: 10.1128/jb.142.2.633-638.1980
Pourbabaee, A. A., Koohbori Dinekaboodi, S., Seyed Hosseini, H. M., Alikhani, H. A., Emami, S. (2020). Potential application of selected sulfur-oxidizing bacteria and different sources of sulfur in plant growth promotion under different moisture condition. Commun. Soil Sci. Plant Anal. 51, 735–745. doi: 10.1080/00103624.2020.1729377
Purnomo, I., Chaerun, S. K., Mubarok, M. Z. (2019). “Biooxidation pretreatment of low grade refractory gold tailings using a sulfur-oxidizing mixotrophic bacterium,” in IOP Conference Series: Materials Science and Engineering (Bristol: IOP Publishing), 1–9. doi: 10.1088/1757-899X/478/1/012020
Rittenberg, S. C. (1969). The roles of exogenous organic matter in the physiology of chemolithotrophic bacteria. Adv. Microb. Physiol. 3, 159–196. doi: 10.1016/S0065-2911(08)60368-9
Sajjad, W., Zheng, G., Din, G., Ma, X., Rafiq, M., Xu, W. (2019). Metals extraction from sulfide ores with microorganisms: the bioleaching technology and recent developments. Trans. Indian Inst. Met. 72, 559–579. doi: 10.1007/s12666-018-1516-4
Schippers, A. (2004). Biogeochemistry of metal sulfide oxidation in mining environments, sediments, and soils. Geol. Soc. Am. 379, 49–62. doi: 10.1130/0-8137-2379-5.49
Schippers, A., Jozsa, P. G., Sand, W. (1996). Sulfur chemistry in bacterial leaching of pyrite. Appl. Environ. Microbiol. 62, 3424–3431. doi: 10.1128/aem.62.9.3424-3431.1996
Schippers, A., Jozsa, P. G., Sand, W., Kovacs, Z. M., Jelea, M. (2000). Microbiological pyrite oxidation in a mine tailings heap and its relevance to the death of vegetation. Geomicrobiol. J. 17, 151–162. doi: 10.1080/01490450050023827
Skarzynski, B., Sczczepkowski, T. W. (1959). Oxidation of thiosulfate by T. thioparus. Nature 183, 1413–1414. doi: 10.1038/1831413a0
Tang, K., Baskaran, V., Nemati, M. (2009). Bacteria of the sulphur cycle: an overview of microbiology, biokinetics and their role in petroleum and mining industries. Biochem. Eng. J. 44, 73–94. doi: 10.1016/j.bej.2008.12.011
Toth, G., Nemestóthy, N., Bélafi-Bakó, K., Vozik, D., Bakonyi, P. (2015). Degradation of hydrogen sulfide by immobilized T. thioparus in continuous biotrickling reactor fed with synthetic gas mixture. Int. Biodeterior. Biodegrad. 105, 185–191. doi: 10.1016/j.ibiod.2015.09.006
Vázquez-Rodríguez, A., Hansel, C. M., Zhang, T., Lamborg, C., Santelli, C. M., Webb, S., et al. (2015). Microbial- and thiosulfate-mediated dissolution of mercury sulfide minerals and transformation to gaseous mercury. Front. Microbiol. 6, 596. doi: 10.3389/fmicb.2015.00596
Widdel, F. (2010). Theory and measurement of bacterial growth. Di Dalam Grundpraktikum Mikrobiologie 4, 1–11.
Yamanaka, T. (1996). Mechanisms of oxidation of inorganic electron donors in autotrophic bacteria. Plant Cell Physiol. 37, 569–574. doi: 10.1093/oxfordjournals.pcp.a028982
Keywords: bio-oxidation, low-grade refractory ores, neutrophiles, neutral oxidation, sulfur removal, biohydrometallurgy
Citation: Hein G, Mahandra H and Ghahreman A (2023) A developing novel alternative bio-oxidation approach to treat low-grade refractory sulfide ores at circumneutral pH. Front. Sustain. Resour. Manag. 2:1102488. doi: 10.3389/fsrma.2023.1102488
Received: 18 November 2022; Accepted: 04 January 2023;
Published: 23 January 2023.
Edited by:
Rasoul Nekouei, University of New South Wales, AustraliaCopyright © 2023 Hein, Mahandra and Ghahreman. This is an open-access article distributed under the terms of the Creative Commons Attribution License (CC BY). The use, distribution or reproduction in other forums is permitted, provided the original author(s) and the copyright owner(s) are credited and that the original publication in this journal is cited, in accordance with accepted academic practice. No use, distribution or reproduction is permitted which does not comply with these terms.
*Correspondence: Guillermo Hein, MTZnYWEzQHF1ZWVuc3UuY2E=; Harshit Mahandra,
aG05MEBxdWVlbnN1LmNh