- 1Department of Engineering, University of Palermo, Palermo, Italy
- 2Department of Mechanical Engineering, University of Massachusetts, Lowell, MA, United States
Introduction
Today, more than half of the world's population resides in urban areas, marking a significant change from the predominantly rural life of past centuries. Urbanization is most pronounced in better-developed regions, where more than 80% of inhabitants live in urban areas, while in many less-developed countries, the majority of the population still lives in rural areas (Ritchie et al., 2024). Projections indicate that by 2050, 68% of the global population will live in urban areas, adding about 2.5 billion people to urban centers, with nearly 90% of this growth concentrated in Asia and Africa. India, China and Nigeria are expected to account for 35% of this increase, highlighting the critical need for sustainable urban planning and infrastructure development to manage this unprecedented growth (United Nations, Department of Economic and Social Affairs, Population Division, 2019).
This rapid urbanization compounds the challenges posed by climate change. The atmospheric concentration of carbon dioxide continues to rise, with a global annual increase of 0.8% between 2018 and 2022. Even with a hypothetical 1% annual reduction, carbon neutrality targets remain unattainable within the next century (Draghi, 2024). This highlights the urgent need for intensified decarbonization efforts. Climate change consequences include prolonged heatwaves, more often and more severe hurricanes and winter storms, water scarcity (Zachariah et al., 2024), floods (WMO, 2024), wildfire (Swain et al., 2025), ocean acidification, and rising sea levels (Gwynn et al., 2024).
Mitigating these challenges calls for novel adaptation policies, technological innovation, and international collaboration. Energy use, particularly electricity generation, is a major greenhouse gas contributor (Li et al., 2024). In 2023, global electricity demand reached 30,000 TWh, driven by economic growth, population increases, and electrification (Ember, 2024). Furthermore, projections suggest that this demand will rise to 45,000 TWh by 2030 and 60,000 TWh by 2050 (IEA, 2023a). Some theoretical studies suggest that meeting this demand sustainably will require tripling renewable energy capacity by 2030 and exceeding 80% renewable penetration by 2050 (Hassan et al., 2024).
High-density urban areas are significant contributors to global energy consumption and carbon emissions, accounting for 30% of global energy use and 37% of energy-related CO2 emissions in 2023, approximately 9.5 gigatons of CO2 per year (IEA, 2023b, 2024). Additionally, the Urban Heat Island (UHI) effect—characterized by elevated temperatures in dense urban areas compared to surrounding rural regions—exacerbates energy demands for cooling and heating, worsens air quality, and reduces thermal comfort (Oke, 1982; Mohajerani et al., 2017). To address these challenges, innovative solutions are essential. Among the most promising is the integration of solar thermal technologies into public infrastructure. These systems offer a dual benefit: they mitigate the UHI effect while reducing carbon emissions and enhancing energy efficiency. By harnessing the untapped potential of urban surfaces such as roads, pavements, and rooftops, solar thermal technologies align with broader sustainability goals and provide a scalable solution to the pressing needs of rapidly growing urban environments. This Specialty Grand Challenge article explores the role of the integration of solar thermal technologies into public infrastructure as a strategic approach to address these challenges. It also determines the research agenda in this field, with each of the sections discussing several major research areas of interest including key trends and associated challenges. By leveraging urban surfaces such as rooftops, pavements, and roads, solar thermal systems can significantly contribute to improving local thermal comfort, controlling buildings and infrastructure surface temperatures, as well as reducing carbon emissions through improving overall building and community scale energy efficiencies.
Solar system integration in high-density urban areas and associated challenges
Promoting widespread renewable energy adoption in high-density urban areas requires integrating solar energy with numerous aspects of architectural and urban design (Peng et al., 2020). However, this is a complex task due to various architectural and functional challenges. For example, rooftops, often utilized for photovoltaic systems, face limitations such as building complexity, interference from the space heating and/or air conditioning systems, as well as variable daily and annual shading (Ren et al., 2022; Hassan et al., 2023). These factors can significantly impact the system's energy/thermal performance. Finally, it needs to be remembered that urban areas usually face serious space constraints that hinder the deployment of large-scale renewable energy systems.
To overcome the above barriers, it is critical to explore innovative technologies that utilize public spaces without compromising their primary functionality. Leveraging public infrastructure, such as streets, parking lots, and pavements, offers an innovative line of solutions. In developed countries, streets alone can account for about 20% of a city's land area, while parks, open spaces, and rooftops present further opportunities for solar energy integration (EU Urban Mobility Observatory, 2021). Paved surfaces across Europe and the United States are predominantly composed of concrete and asphalt, which can reach elevated temperatures during the summer months (Santamouris, 2013). Research highlights that pavements account for nearly 29% of the urban landscape in the United States. Additional studies cited in Akbari and Rose (2001) and Gray and Finster (2000) for various cities in the United States reveal that paved surfaces—such as roads, sidewalks, and parking areas—constitute between 29% and 39% of the urban area. Strategically repurposing these surfaces for solar energy systems unlocks substantial renewable energy generation potential, while preserving the primary functionality of these spaces.
It is also worth mentioning, that, it has been already demonstrated in numerous geographic locations that municipal water reservoirs offer valuable passive solar solutions, such as thermal energy storage and temperature control in urban settings (Gross, 2017; Broadbent et al., 2018). These reservoirs can absorb and store excess thermal energy captured from solar systems during peak radiation periods. Stored heat can then be utilized for district heating or converted into electricity using special heat exchange systems (Sarbu and Sebarchievici, 2018). Additionally, water reservoirs moderate local temperatures by absorbing heat during the day and releasing it at night, helping to mitigate the Urban Heat Island (UHI) effect (Van Hove et al., 2015). On a community scale, this dual functionality reduces energy demands for air conditioning, lowering greenhouse gas emissions while enhancing local thermal comfort.
In the light of the above, the premise of integrating solar technologies into public infrastructure, such as roadways and pavements, seems to be particularly promising. These often-underutilized areas/surfaces can be transformed into multifunctional solar energy assets. For example, roadways equipped with solar thermal collectors can capture and store heat during the day, contributing to district heating systems and reducing dependence on fossil fuels. This approach also mitigates the UHI effect, improving thermal comfort in densely populated areas. By optimizing urban spaces for dual purposes, such as energy generation and their original use, cities can enhance sustainability, address space constraints, and accelerate their transition to a low-carbon future. These strategies align with broader urban planning goals, demonstrating the feasibility of renewable energy adoption even in the most challenging contexts.
It needs to be also considered that economic, regulatory, and social factors further complicate the adoption/incorporation of solar technologies in urban environments. High upfront costs, aesthetic concerns, and competing urban priorities often deter investment and public acceptance. Integrating solar technologies with existing infrastructure and ensuring compliance with local regulations is especially challenging in retrofitting projects, often requiring costly modifications. Addressing these challenges necessitates equally innovative architectural/urban designs, novel solar energy integration strategies, and often, creative code compliance approaches.
Technology innovation for solar thermal exploitation and potential research and development (R&D) trends in urban areas
This section outlines several promising areas of solar thermal technologies to be used in the urban environment. Specifically, it discusses them as potential future components of a broad research agenda in this field, with each of the subsections introducing a major research area of interest, including key trends and summarizing research challenges. Pavements and road surfaces may play a critical role in absorbing and storing solar radiation, which amplifies local temperature increases, contributing to the urban heat island (UHI) effect (Carnielo and Zinzi, 2013). Addressing these capabilities requires an implementation of sustainable urban design strategies, such as for example cool pavements utilizing innovative surfaces designed to reduce temperatures and enhance thermal comfort. Cool pavements can be classified as reflective, evaporative, and heat-harvesting systems (Qin, 2015).
Reflective pavements
Reflective pavements utilize materials with high albedo (high reflectivity of solar radiation from a surface) to reflect solar radiation, thereby reducing surface temperatures. For example, studies have shown that increasing surface reflectivity can lower peak temperatures by 2–4°C in urban areas, significantly improving local thermal comfort (Donthu et al., 2024). Reflective coatings developed using high visible and near-infrared reflectance have demonstrated temperature reduction of up to 12°C under field conditions (Santamouris, 2013; Kousis and Pisello, 2023). Large-scale implementations, such as in Athens, Greece, achieved surface cooling effects of up to 11°C and near-surface air temperature reductions of up to 1.5°C (Kyriakodis and Santamouris, 2018). Similarly, researches by the Lawrence Berkeley National Laboratory (LBNL) Heat Island Group (Brown, 2018; Mohegh et al., 2018; Taha et al., 2018) demonstrate that increasing neighborhood-scale albedo through cool roofs and reflective pavements can effectively lower air temperatures. Their studies indicate that enhancing surface albedo across urban landscapes contributes to a measurable decrease in daytime air temperatures, especially in heat-prone regions such as Los Angeles, offering simple, low-cost, scalable solutions to combat urban heat islands. Robinson et al. (2024) emphasize the potential of cool pavement technologies, demonstrating that increasing neighborhood-scale albedo by factor of 0.1 can achieve average air temperature reductions of 0.75–1.1 K, with notable localized cooling benefits. For pavements, their findings indicate that increasing albedo from 0.15 to 0.25 significantly enhances cooling effects, highlighting their importance in promoting sustainable urban development (Robinson et al., 2024).
Evaporative pavements
Evaporative pavements, on the other hand, incorporate water-retentive materials that cool the surrounding air through latent heat transfer. Research indicates that permeable pavements can lower surface temperatures by 10–25°C during wet conditions compared to impermeable surfaces (Li et al., 2013). Such systems have proven effective in Tokyo, where water-retentive pavements reduced temperatures by 13–19°C (Takahashi and Yabuta, 2009). However, their performance is highly dependent on local climatic conditions and water availability, which may limit their performance in arid regions.
Heat harvesting pavement systems
Heat-harvesting systems, referred to as Urban Area Thermal Collectors (UATCs), are designed to capture solar energy absorbed by asphalt and reuse it for practical thermal applications, such as heating, snow melting, and energy storage (Bobes-Jesus et al., 2013; Pei et al., 2021). These systems integrate embedded heat exchangers with road or pavement structures, where absorbed solar energy is transferred to a working fluid. UATCs have demonstrated seasonal energy storage efficiency of 17% and surface temperature reductions of up to 7°C in experimental conditions (Zhou et al., 2015). Similarly, advanced simulations by Ma et al. (2022) showed that pavement-integrated solar thermal (PIST) systems could reduce asphalt temperatures up to 10.6°C and achieve an energy efficiency of 37.3%, further supporting their role in mitigating urban heat island effects (Ma et al., 2022). It is worth considering that UATCs are relatively inexpensive and simple to install, which renders them an excellent sustainable strategy for infrastructure retrofit/resurfacing works. They can be embedded beneath various urban surfaces, such as roads, pavements, and parking lots, eliminating the need for additional land and making them particularly suitable for densely populated areas.
Integration strategies with thermal storage
In colder climates, UATCs are particularly effective in preventing road freezing, significantly improving road safety during winter. For instance, a UATC system in South Korea not only reduced surface temperatures by 10–12°, during summer months, but also in winter, it lowered snow melting energy demands by 35% (Hyun et al., 2024). Similar technologies can be integrated with Borehole Thermal Energy Storage (BTES) systems, allowing surplus heat collected during summer to be stored underground, and utilized during winter months. This integration enhances system efficiency and reliability (Yang et al., 2021; Mohebi and Roshandel, 2023; Simpson et al., 2024). A study demonstrated that in central Sweden climate, hydronic heating pavement combined with a BTES system harvested 352.1 kWh/m2 annually, significantly exceeding the 81.2 kWh/m2 required for anti-icing operations. Additionally, these systems reduced the number of slippery hours on roads by 65% during winter and lowered summer road surface temperatures by 5.1°C (Mirzanamadi et al., 2018).
UATCs and thermal storage can be also integrated with neighboring buildings. In the Netherlands, a pilot project, which combined UATCs with BTES, demonstrated a 25% reduction in heating energy costs in integrated adjacent buildings, underscoring the economic and environmental benefits of UATC applications (Pei et al., 2021). Finally, by integrating UATCs with advanced underground or urban-scale thermal storage, surplus heat can be effectively collected and utilized across seasons. This seasonal energy management strategy balances energy supply and demand, reducing peak loads and supporting better integration of buildings and communities with the electric power grid. Through their ability to harness existing urban surfaces while preserving their primary functionality, UATCs provide a scalable, sustainable solution to urban energy challenges.
Cool pavements and urban area thermal collectors: example of simple, practical, and affordable urban solar applications
Cool pavements and UATCs are cost-effective and practical solutions for improving local thermal comfort and enhancing urban sustainability. By utilizing existing urban surfaces such as roads, pavements, and parking lots, these systems absorb and utilize solar energy to reduce reliance on fossil fuels and, during summer, mitigate local ambient temperatures. Their integration into public infrastructure optimizes energy generation without involving additional grounds, or structures. Key advantages of UATCs include their adaptability, simplicity, and low implementation cost. Unlike conventional solar panels, which often require significant structural modifications, UATCs can be incorporated into existing surfaces with minimal construction disruption. Considering that this infrastructure must be periodically maintained/resurfaced, this approach can be a cost-effective solution. Asphalt and concrete, which inherently absorb and store heat, form the foundation for these systems, reducing the need for significant additional investments. Furthermore, combining these systems with seasonal thermal storage enhances energy efficiency, allowing the collection of surplus heat during the summer, to be used in winter.
For example, studies have demonstrated that the long-term cost-effectiveness of integrating UATCs with geothermal or hydronic systems and seasonal energy storage may yield high coefficients of performance (COP) of 4–5, compared to conventional systems with a COP of 1.0 (Jaiswal et al., 2023). Furthermore, their ability to reduce surface temperatures not only mitigates the UHI effect but also helps prolong pavement lifespan, reducing maintenance costs and conserving natural resources (Wang et al., 2021; Seifeddine et al., 2023). In prototype installation by the University of Palermo (UNIPA) (see Figures 1, 2) an absorbent surface in a parking lot was combined with seasonal geothermal storage to power high-efficiency heat pumps for winter heating. This approach reduced university campus heating costs and mitigated UHI effects without compromising the primary functionality of urban surfaces (Buscemi et al., 2023). By leveraging the potential of existing infrastructure, these systems demonstrate how innovation can transform ordinary urban infrastructure into multifunctional assets. The adaptability, scalability, and low cost of UATCs make them an essential tool for addressing urban energy challenges, contributing to climate resilience and long-term sustainability.
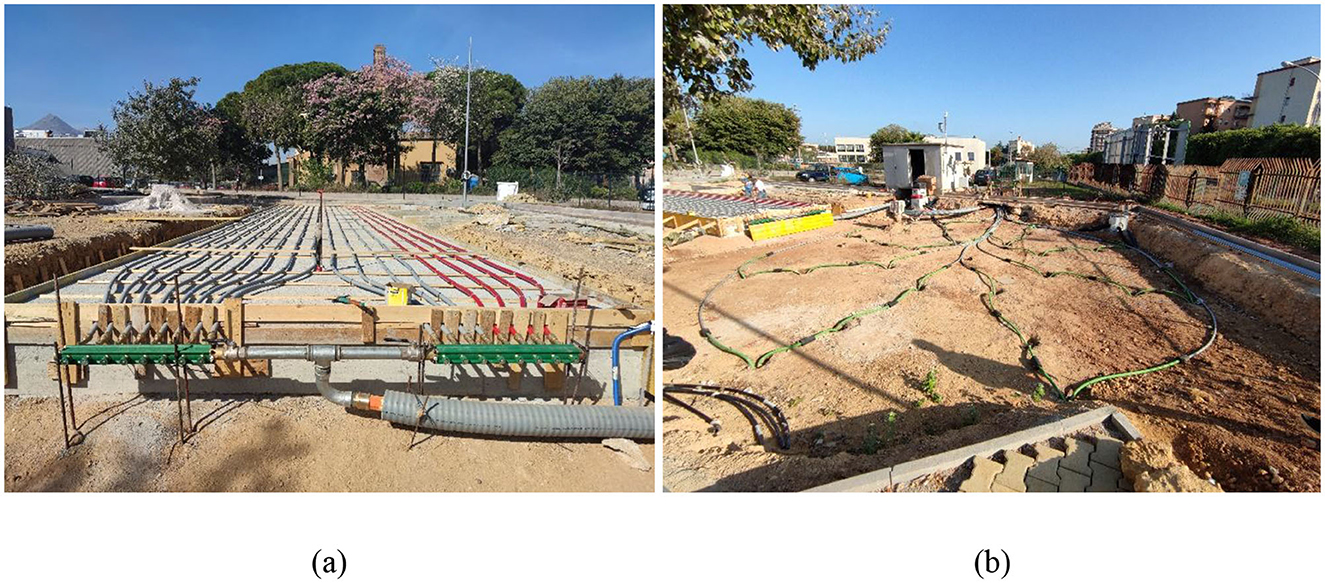
Figure 1. Urban area thermal collector (a) and borehole thermal energy storage system (b) under construction at the University of Palermo campus.
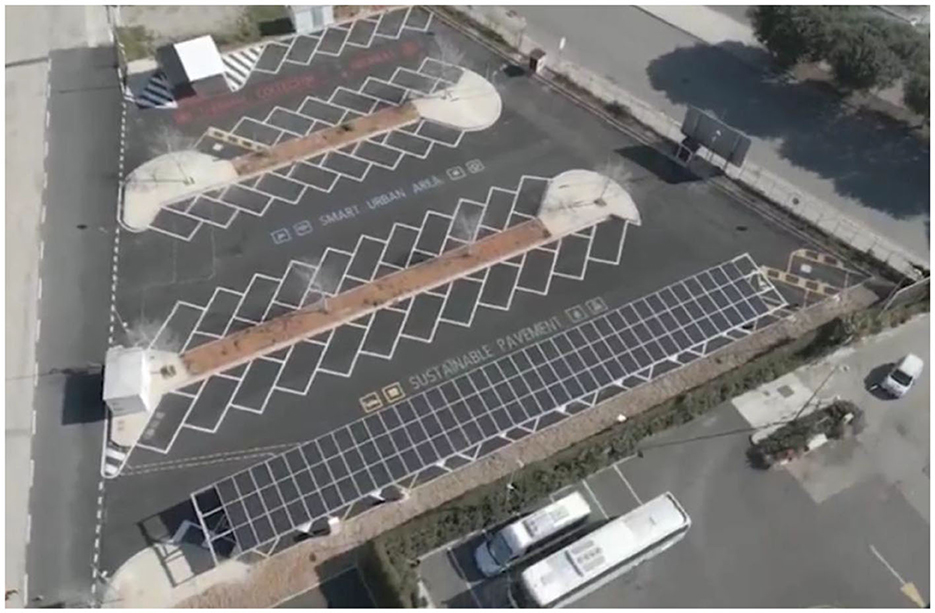
Figure 2. Aerial view of the parking place over the road thermal collector—University of Palermo campus.
The role of a numerical modeling in advancing UATC systems: current trends and future research directions
Numerical modeling is a cornerstone for the development, integration, and optimization of Urban Area Thermal Collector (UATC) systems. Beyond its role in evaluating system performance, numerical modeling serves as a crucial driver for defining future research directions in this field. This section outlines the major areas of interest in UATC modeling, identifies current challenges, and highlights key opportunities for advancing research and technological innovation. By framing this discussion within an agenda of research priorities, the objective is to illustrate a roadmap for further developments in UATC numerical modeling.
Thermal performance optimization models
Numerical models have been employed to optimize the thermal performance of UATC systems, particularly in urban pavements and roadways. Computational Fluid Dynamics (CFD) simulations allow the influence of variables such as pipe spacing, fluid flow rates, and material properties on energy efficiency to be evaluated. For example, simplified models designed for pavement solar collectors have shown thermal efficiencies reaching 49% under optimized configurations (Johnsson and Adl-Zarrabi, 2020). Coupled modeling approaches integrating street canyon dynamics with solar system performance have revealed reductions in surface temperature by up to 10°C and near-surface air temperature decreases of up to 4.6°C (Xu et al., 2021). These findings underscore the crucial role of numerical simulations in refining system configurations, ensuring optimal performance before large-scale implementations.
Cooling strategies and urban heat island mitigation
Advances in cool pavement technologies have benefited from modeling approaches that support the integration of innovative materials, such as phase-change components, which improve energy performance and durability (Anupam et al., 2021). Observations in Los Angeles show that increasing albedo by 0.1 reduces daytime air temperatures by up to 2.8°C and nighttime temperatures by 0.9°C (Taha et al., 2018). Similarly, a 0.1 increase in vegetation canopy cover can lower nighttime air temperatures by up to 1.5°C (Mohegh et al., 2018).
In addition to direct cooling, hydronic systems integrated with UATC technologies have demonstrated significant potential for reducing surface temperatures and optimizing energy harvesting processes. Simplified models, like those designed for pavement solar collectors, allow for efficient evaluation of energy transfer processes, making them particularly valuable during early design stages (Ghalandari et al., 2021). For example, Ghalandari et al. developed a simplified model to evaluate the thermal performance of pavement solar collectors, highlighting key parameters like pipe spacing and material properties (Ghalandari et al., 2021). Zhou et al. validated the cooling benefits of such systems, showing reductions of up to 7°C in pavement surface temperatures and a thermal storage efficiency of 17% (Zhou et al., 2015). Field studies and Finite Element Method (FEM) simulations have further validated these findings. In Sweden, the HyRoSim model was employed to assess a 70 m2 pavement solar collector, demonstrating precise energy output predictions and system reliability (Johnsson and Adl-Zarrabi, 2020). Similarly, two-dimensional transient models have been developed and validated to simulate pavement-integrated solar thermal (PIST) systems. These simulations revealed asphalt temperature reductions of up to 10.57°C and energy efficiencies exceeding 37% under optimal conditions (Ma et al., 2022). The Lawrence Berkeley National Laboratory (LBNL) further investigated the impact of reflective surfaces such as cool roofs and pavements. Modeling revealed potential temperature drops of up to 6.56°C under extreme conditions, with mean daily air temperature reductions of 1.84°C observed in Central Los Angeles when roof albedo was increased by 0.1, compared to 0.25°C in the San Fernando Valley (Mohegh et al., 2018).
Numerical analysis plays also a pivotal role in assessing UATC systems' effectiveness in reducing urban heat. Reflective and evaporative cooling pavements have been modeled to quantify their impact on urban microclimates. For example, Nasir et al. (2021) demonstrated that Road Pavement Solar Collectors (RPSCs) embedded in urban canyons can achieve surface temperature reductions of up to 8°C and ambient air temperature drops of approximately 0.8°C under optimal configurations. Krayenhoff et al. provided a systematic review emphasizing that albedo modifications and green infrastructure interventions can yield cooling effects ranging from 0.2°C to 0.6°C for every 0.1 increase in neighborhood albedo, highlighting the role of reflective materials in mitigating urban heat (Krayenhoff et al., 2021). CFD and ENVI-met simulations have highlighted the potential of water-retentive pavements in reducing surface temperatures by leveraging evaporation as a cooling mechanism. For instance, the REALCOOL project demonstrated that incorporating shading, ventilation, and water mist features alongside evaporative cooling pavements can reduce air temperatures by up to 0.6°C and Physiological Equivalent Temperature (PET) by up to 1.9°C in compact urban environments (Jacobs et al., 2020). Moreover, evaporative pavements studied in Wuhan revealed that water-cooling intensity varies significantly with urban form, suggesting that larger impervious surface areas in proximity to water-retentive pavements enhance cooling effects (Lu et al., 2024). These findings underscore the importance of urban form considerations in maximizing the effectiveness of evaporative cooling strategies.
Integration with renewable energy storage
Numerical methods have been widely used in the study of Borehole Thermal Energy Storage (BTES) and Road Thermal Collector (RTC) systems. FEM-based simulations have been applied to optimize the arrangement of borehole heat exchangers (BHEs), assess thermal conductivity variations in different soil layers, and predict temperature profiles in operational systems (Yang et al., 2021; Mohebi and Roshandel, 2023). Similarly, studies in Antwerp and Adelaide have utilized TRNSYS and COMSOL Multiphysics to simulate the long-term viability of these systems, demonstrating substantial heating cost reductions and improved system efficiencies (Ghalandari et al., 2022; Motamedi et al., 2022).
In a Mediterranean context, a validated numerical model combining FEM and TRNSYS was developed to analyze the thermal performance of an integrated UATC-BTES system (Buscemi et al., 2023). Experimental data from thermal sensors embedded in the pavement stratigraphy and BHEs were used to calibrate the model, ensuring high accuracy in replicating real-world conditions. Simulations revealed that the seasonal storage efficiency of the BTES could reach up to 80%, with fluid temperatures of approximately 45°C achieved during peak summer months. This integration allowed for a significant reduction in borehole length compared to conventional geothermal systems (Buscemi et al., 2023).
Numerical modeling is a key element in the wider effort to integrate solar thermal solutions into urban environments. Refining simulation capabilities and validating results through real-world applications can accelerate the deployment of UATC systems, contributing to the broader climate-resilient urban development agenda. By addressing these challenges, future research on numerical modeling will play a key role in optimizing UATC systems for sustainable urban environments. These methodologies ensure that UATC systems are not only efficient and scalable but also adaptable to the evolving demands of sustainable urban development.
Future research directions in numerical modeling for UATCs
Despite significant advancements, there are still several key research areas that require further exploration to enhance the effectiveness of numerical modeling for UATC systems:
• Hybrid modeling approaches: Integrating machine learning techniques with CFD/FEM methods could reduce computational time while improving predictive accuracy. Hybrid models could enable real-time optimization of UATC systems in dynamic urban conditions.
• Dynamic urban climate interactions: Current models often simplify urban microclimatic effect. Advancing multi-layered, high-resolution urban models that incorporate wind patterns, radiation exchange, and anthropogenic heat sources will improve accuracy.
• Multiscale modeling strategies: Developing a more comprehensive framework that links microscale thermal interactions (pavements, roads) with macroscale energy systems (district heating, smart grids) will facilitate integrated urban energy planning.
• Experimental validation of large-scale deployments: Despite promising numerical results, large-scale pilot projects remain limited. Expanding real-world experimental validation of UATC systems will enhance model reliability and support their adoption in urban policies.
• Economic and policy integration: Integrating cost-benefit analyses and regulatory frameworks into UATC models will facilitate adoption by urban planners and policy makers.
By addressing these challenges, future research in numerical modeling will play a pivotal role in optimizing UATC systems for sustainable urban environments. These methodologies ensure that UATC systems are not only efficient and scalable but also adaptable to the evolving demands of climate resilience and urban sustainability.
Conclusions
High-density urban areas are significant contributors to global energy consumption and carbon emissions. However, these areas also belong to the most promising ways in the potential integration of solar thermal technologies into public infrastructure. The urban area-integrated solar systems offer unique benefits, including the reduction of carbon emissions, while enhancing energy efficiency and mitigating the UHI effect. By harnessing the untapped potential of urban surfaces such as roads, pavements, and rooftops, solar thermal technologies align with broader sustainability goals and provide a scalable solution to the pressing needs of rapidly growing urban environments.
This Specialty Grand Challenge article outlines the transformative potential of Urban Area Thermal Collector (UATC) systems and in addressing contemporary urban energy and climate changes. The findings presented here provide a foundation for interdisciplinary collaborations, large-scale pilot studies, and policy-driven innovation. Future efforts should aim at bridging the gap between numerical simulations and real-world applications, ensuring that urban energy solutions are not only technically feasible but also economically and socially viable.
The most effective approaches are associated with the integration of reflective (cool) surfaces and/or evaporative pavement systems, applied alongside UATCs. They can amplify cooling effects, with reflective surfaces and by utilizing evaporative cooling energy, which yields, not only the reduction of surface temperatures but also, lower the ambient air temperatures during the cooling season.
Further improvements in thermal performance can be achieved by the integration of UATCs with thermal energy storage. For instance, systems incorporating UATCs and Borehole Thermal Energy Storage (BTES) demonstrate seasonal energy storage efficiencies of up to 17% while reducing road surface temperatures by 7°C (East-Central China, Sicilia). In the case of evaporative pavements, surface temperatures can be lowered by as much as 25°C (Zhou et al., 2015). In colder climates, in addition to reducing summer surface temperatures (10–12°C in South Korean climate), the UATCs technology has also shown effectiveness in preventing road freezing, and by cutting snow-melting energy demands by 35%, or reducing the number of slippery hours on roads by 65% (in Sweden climate). Numerous studies performed with a wide variety of advanced numerical models (both, commercially available tools, and custom programs) further underscore these systems' potential, revealing high energy storage efficiencies and localized surface cooling effects.
Beyond thermal benefits, UATCs contribute to decarbonization by repurposing existing infrastructure. A Dutch pilot project integrating UATCs with BTES achieved a 25% reduction in heating costs for adjacent buildings, demonstrating their economic feasibility.
While significant advancements have been made, several challenges remain. The large-scale deployment of UATC technologies requires further investigation into long-term durability, maintenance requirements, and lifecycle costs. Additionally, further research should explore innovative materials, adaptive control strategies, and hybrid energy integration approaches to maximize efficiency. Addressing these challenges is crucial for improving the feasibility and economic viability of UATC systems.
Further research should prioritize:
• Optimization of thermophysical properties of materials to enhance system efficiency across diverse climatic conditions.
• Development of advanced control algorithms to adapt UATC systems dynamically to changing urban energy demands.
• Comprehensive economic analyses to balance installation and operational costs with long-term energy savings.
• Integration of UATC technologies into urban energy grids to maximize synergies with district heating and cooling networks.
By focusing on these areas, cities can accelerate their transition toward low-carbon, climate-resilient futures. International collaboration and continued innovation will be essential to redefining urban energy infrastructures and ensuring that UATC systems contribute meaningfully to global sustainability goals. Establishing a dedicated research agenda for UATCs will drive further advancements, bridging the gap between technological potential and large-scale implementation.
Author contributions
SG: Data curation, Formal analysis, Investigation, Writing – original draft. VL: Conceptualization, Funding acquisition, Methodology, Supervision, Writing – original draft, Writing – review & editing. JK: Conceptualization, Writing – original draft, Writing – review & editing.
Funding
The author(s) declare that financial support was received for the research and/or publication of this article. This work was financially supported by: Project SmartEP (CUP: G58I18000770007) financed within the call PO-FESR Sicilia “Sostegno all'avanzamento tecnologico delle imprese attraverso il finanziamento di linee pilota e azioni di validazione precoce dei prodotti e di dimostrazione su larga scala” and Center for Energy Innovation, University of Massachusetts, Lowell, 2024/25 Seed Funding.
Conflict of interest
The authors declare that the research was conducted in the absence of any commercial or financial relationships that could be construed as a potential conflict of interest.
The author(s) declared that they were an editorial board member of Frontiers, at the time of submission. This had no impact on the peer review process and the final decision.
Publisher's note
All claims expressed in this article are solely those of the authors and do not necessarily represent those of their affiliated organizations, or those of the publisher, the editors and the reviewers. Any product that may be evaluated in this article, or claim that may be made by its manufacturer, is not guaranteed or endorsed by the publisher.
References
Akbari, H., and Rose, L. S. (2001). Characterizing the Fabric of the Urban Environment: A Case Study of Metropolitan Chicago, Illinois. Berkeley, CA: Lawrence Berkeley Natl. Lab. Rep.
Anupam, B. R., Sahoo, U. C., Chandrappa, A. K., and Rath, P. (2021). Emerging technologies in cool pavements: a review. Constr. Build. Mater. 299:123892. doi: 10.1016/j.conbuildmat.2021.123892
Bobes-Jesus, V., Pascual-Muñoz, P., Castro-Fresno, D., and Rodriguez-Hernandez, J. (2013). Asphalt solar collectors: a literature review. Appl. Energy 102, 962–970. doi: 10.1016/j.apenergy.2012.08.050
Broadbent, A. M., Coutts, A. M., Tapper, N. J., Demuzere, M., and Beringer, J. (2018). The microscale cooling effects of water sensitive urban design and irrigation in a suburban environment. Theor. Appl. Climatol. 134, 1–23. doi: 10.1007/s00704-017-2241-3
Brown, E. G. (2018). Modeling and Observations to Detect Neighborhood-Scale Heat Islands and Inform Effective Countermeasures in Los Angeles. California, CA: California Energy Commission (CEC).
Buscemi, A., Beccali, M., Guarino, S., and Lo Brano, V. (2023). Coupling a road solar thermal collector and borehole thermal energy storage for building heating: first experimental and numerical results. Energy Convers. Manag. 291:117279. doi: 10.1016/j.enconman.2023.117279
Carnielo, E., and Zinzi, M. (2013). Optical and thermal characterisation of cool asphalts to mitigate urban temperatures and building cooling demand. Build. Environ. 60, 56–65. doi: 10.1016/j.buildenv.2012.11.004
Donthu, E. V. S. K. K., Long, Y. P., Wan, M. P., Zhou, M., and Ng, B. F. (2024). Dynamics of cool surface performance on urban microclimate: a full-scale experimental study in Singapore. Sustain. Cities Soc. 102:105218. doi: 10.1016/j.scs.2024.105218
Draghi, M. (2024). The Future of European Competitiveness Part A: A Competitiveness Strategy for Europe. Belgium: European Commission. Retrieved from: https://coilink.org/20.500.12592/9kbtlhp (accessed March 28, 2025).
Ember (2024). Yearly Electricity Data. Available online at: https://ember-climate.org/data-catalogue/yearly-electricity-data/ (accessed January 16, 2025).
EU Urban Mobility Observatory (2021) Identifying the Amount of Urban Space Occupied by Roads. European Commission Directorate-General for Mobility and Transport. Retrieved from: https://urban-mobility-observatory.transport.ec.europa.eu/news-events/news/identifying-amount-urban-space-occupied-roads-2021-06-21_en (accessed 21 June, 2021).
Ghalandari, T., Baetens, R., Verhaert, I., Nasir, D., Van den bergh, W., and Vuye, C. (2022). Thermal performance of a controllable pavement solar collector prototype with configuration flexibility. Appl. Energy 313:118908. doi: 10.1016/j.apenergy.2022.118908
Ghalandari, T., Ceulemans, D., Hasheminejad, N., Guldentops, G., Van den bergh, W., Verhaert, I., et al. (2021). A simplified model to assess the thermal performance of pavement solar collectors. Appl. Therm. Eng. 197:117400. doi: 10.1016/j.applthermaleng.2021.117400
Gray, K. A., and Finster, M. E. (2000). The Urban Heat Island, Photochemical Smog, and Chicago: Local Features of the Problem and Solution. Evanston, IL: Civil and Environmental Engineering Department. Northwest. Univ. Evanston.
Gross, G. (2017). Some Effects of Water Bodies on the Environment – Numerical Experiments. Available online at: https://api.semanticscholar.org/CorpusID:40757826 (accessed January 24, 2025).
Gwynn, J. P., Hatje, V., Casacuberta, N., Sarin, M., and Osvath, I. (2024). The effect of climate change on sources of radionuclides to the marine environment. Commun. Earth & Environ. 5:135. doi: 10.1038/s43247-024-01241-w
Hassan, Q., Algburi, S., Sameen, A. Z., Salman, H. M., and Jaszczur, M. (2023). A review of hybrid renewable energy systems: solar and wind-powered solutions: challenges, opportunities, and policy implications. Results Eng. 20:101621. doi: 10.1016/j.rineng.2023.101621
Hassan, Q., Nassar, A. K., Al-Jiboory, A. K., Viktor, P., Telba, A. A., Awwad, E. M., et al. (2024). Mapping Europe renewable energy landscape: insights into solar, wind, hydro, and green hydrogen production. Technol. Soc. 77:102535. doi: 10.1016/j.techsoc.2024.102535
Hyun, S. W., Kim, S., Jeong, H., Ko, H. S., and Shin, D. H. (2024). Development of snow removal system using embedded pipes inside road with solar thermal energy collector and packed bed latent heat thermal energy storage. J. Energy Storage 83:110737. doi: 10.1016/j.est.2024.110737
IEA (2023a). World Energy Outlook 2023. Paris. Available online at: https://www.iea.org/reports/world-energy-outlook-2023 (accessed January 16, 2025).
IEA (2023b). Tracking Clean Energy Progress 2023. Paris. Available online at: https://www.iea.org/reports/tracking-clean-energy-progress-2023 (accessed January 7, 2025).
IEA (2024). World Energy Outlook 2024. Paris. Available online at: https://www.iea.org/reports/world-energy-outlook-2024/ (accessed January 7, 2025).
Jacobs, C., Klok, L., Bruse, M., Cortesão, J., Lenzholzer, S., and Kluck, J. (2020). Are urban water bodies really cooling? Urban Clim. 32:100607. doi: 10.1016/j.uclim.2020.100607
Jaiswal, P., Anupam, B. R., Chandrappa, A. K., and Sahoo, U. C. (2023). Harvesting heat energy using geothermal and hydronic pavements for sustainable cities: a comprehensive review of an emerging idea. Sustain. Cities Soc. 93:104539. doi: 10.1016/j.scs.2023.104539
Johnsson, J., and Adl-Zarrabi, B. (2020). A numerical and experimental study of a pavement solar collector for the northern hemisphere. Appl. Energy 260:114286. doi: 10.1016/j.apenergy.2019.114286
Kousis, I., and Pisello, A. L. (2023). Evaluating the performance of cool pavements for urban heat island mitigation under realistic conditions: a systematic review and meta-analysis. Urban Clim. 49:101470. doi: 10.1016/j.uclim.2023.101470
Krayenhoff, E. S., Broadbent, A. M., Zhao, L., Georgescu, M., Middel, A., Voogt, J. A., et al. (2021). Cooling hot cities: a systematic and critical review of the numerical modelling literature. Environ. Res. Lett. 16:53007. doi: 10.1088/1748-9326/abdcf1
Kyriakodis, G.-E., and Santamouris, M. (2018). Using reflective pavements to mitigate urban heat island in warm climates - results from a large scale urban mitigation project. Urban Clim. 24, 326–339. doi: 10.1016/j.uclim.2017.02.002
Li, H., Harvey, J. T., Holland, T. J., and Kayhanian, M. (2013). The use of reflective and permeable pavements as a potential practice for heat island mitigation and stormwater management. Environ. Res. Lett. 8:015023. doi: 10.1088/1748-9326/8/1/015023
Li, Y., Yang, X., Du, E., Liu, Y., Zhang, S., Yang, C., et al. (2024). A review on carbon emission accounting approaches for the electricity power industry. Appl. Energy 359:122681. doi: 10.1016/j.apenergy.2024.122681
Lu, Y., Hu, Y., He, T., Yue, W., Shan, Z., and Chen, Y. (2024). How does urban form explain water cooling effect heterogeneity: a case study in Wuhan, China. Build. Environ. 265:111973. doi: 10.1016/j.buildenv.2024.111973
Ma, T., Li, S., Gu, W., Weng, S., Peng, J., and Xiao, G. (2022). Solar energy harvesting pavements on the road: comparative study and performance assessment. Sustain. Cities Soc. 81:103868. doi: 10.1016/j.scs.2022.103868
Mirzanamadi, R., Hagentoft, C.-E., and Johansson, P. (2018). Numerical investigation of harvesting solar energy and anti-icing road surfaces using a hydronic heating pavement and borehole thermal energy storage. Energies 11:3443. doi: 10.3390/en11123443
Mohajerani, A., Bakaric, J., and Jeffrey-Bailey, T. (2017). The urban heat island effect, its causes, and mitigation, with reference to the thermal properties of asphalt concrete. J. Environ. Manage. 197, 522–538. doi: 10.1016/j.jenvman.2017.03.095
Mohebi, P., and Roshandel, R. (2023). Optimal design and operation of solar energy system with heat storage for agricultural greenhouse heating. Energy Convers. Manag. X 18:100353. doi: 10.1016/j.ecmx.2023.100353
Mohegh, A., Levinson, R., Taha, H., Gilbert, H., Zhang, J., Li, Y., et al. (2018). Observational evidence of neighborhood scale reductions in air temperature associated with increases in roof albedo. Climate 6:98. doi: 10.3390/cli6040098
Motamedi, Y., Makasis, N., Gu, X., Narsilio, G. A., Arulrajah, A., and Horpibulsuk, S. (2022). Numerical investigation of geothermal pavements: design optimisation & boundary conditions. Transp. Geotech. 37:100843. doi: 10.1016/j.trgeo.2022.100843
Nasir, D. S. N. M., Pantua, C. A. J., Zhou, B., Vital, B., Calautit, J., and Hughes, B. (2021). Numerical analysis of an urban road pavement solar collector (U-RPSC) for heat island mitigation: Impact on the urban environment. Renew. Energy 164, 618–641. doi: 10.1016/j.renene.2020.07.107
Oke, T. R. (1982). The energetic basis of the urban heat island. Q. J. R. Meteorol. Soc. 108, 1–24. doi: 10.1002/qj.49710845502
Pei, J., Guo, F., Zhang, J., Zhou, B., Bi, Y., and Li, R. (2021). Review and analysis of energy harvesting technologies in roadway transportation. J. Clean. Prod. 288:125338. doi: 10.1016/j.jclepro.2020.125338
Peng, J., Yan, J., Zhai, Z., Markides, C. N., Lee, E. S., Eicker, U., et al. (2020). Solar energy integration in buildings. Appl. Energy 264:114740. doi: 10.1016/j.apenergy.2020.114740
Qin, Y. (2015). A review on the development of cool pavements to mitigate urban heat island effect. Renew. Sustain. Energy Rev. 52, 445–459. doi: 10.1016/j.rser.2015.07.177
Ren, H., Xu, C., Ma, Z., and Sun, Y. (2022). A novel 3D-geographic information system and deep learning integrated approach for high-accuracy building rooftop solar energy potential characterization of high-density cities. Appl. Energy 306:117985. doi: 10.1016/j.apenergy.2021.117985
Robinson, A., Fernandes, S., Hong, T., and Lee, S. H. (2024). Potential Urban Heat Island Countermeasures and Building Energy Efficiency Improvements in Los Angeles County. California, CA: Lawrence Berkeley National Laboratory. Retrieved from: https://escholarship.org/uc/item/5692c9gw
Santamouris, M. (2013). Using cool pavements as a mitigation strategy to fight urban heat island - a review of the actual developments. Renew. Sustain. Energy Rev. 26, 224–240. doi: 10.1016/j.rser.2013.05.047
Sarbu, I., and Sebarchievici, C. (2018). A Comprehensive Review of Thermal Energy Storage. Sustainability 10:191. doi: 10.3390/su10010191
Seifeddine, K., Amziane, S., Toussaint, E., and Ouldboukhitine, S.-E. (2023). Review on thermal behavior of cool pavements. Urban Clim. 51:101667. doi: 10.1016/j.uclim.2023.101667
Simpson, J. G., Long, N., and Zhu, G. (2024). Decarbonized district energy systems: past review and future projections. Energy Convers. Manag. X 24 :100726. doi: 10.1016/j.ecmx.2024.100726
Swain, D. L., Prein, A. F., Abatzoglou, J. T., Albano, C. M., Brunner, M., Diffenbaugh, N. S., et al. (2025). Hydroclimate volatility on a warming Earth. Nat. Rev. Earth & Environ. 6, 35–50. doi: 10.1038/s43017-024-00624-z
Taha, H., Levinson, R., Mohegh, A., Gilbert, H., Ban-Weiss, G., and Chen, S. (2018). Air-temperature response to neighborhood-scale variations in albedo and canopy cover in the real world: fine-resolution meteorological modeling and mobile temperature observations in the Los Angeles climate archipelago. Climate 6:53. doi: 10.3390/cli6020053
Takahashi, K., and Yabuta, K. (2009). “Road temperature mitigation effect of “roadv cool,” a water-retentive material using blast furnace slag,” in JFE Tech. Rep. 58–62. Available online at: https://www.scopus.com/inward/record.uri?eid=2-s2.0-69249139556&partnerID=40&md5=125a6d3d1ba7ce95672860546a65404b (accessed January 20, 2025).
United Nations, Department of Economic and Social Affairs, Population Division. (2019). World Urbanization Prospects 2018: Highlights (ST/ESA/SER.A/421). New York, NY: United Nations, Department of Economic and Social Affairs, Population Division.
Van Hove, L. W. A., Jacobs, C. M. J., Heusinkveld, B. G., Elbers, J. A., Van Driel, B. L., and Holtslag, A. A. M. (2015). Temporal and spatial variability of urban heat island and thermal comfort within the Rotterdam agglomeration. Build. Environ. 83, 91–103. doi: 10.1016/j.buildenv.2014.08.029
Wang, C., Wang, Z.-H., Kaloush, K. E., and Shacat, J. (2021). Cool pavements for urban heat island mitigation: a synthetic review. Renew. Sustain. Energy Rev. 146:111171. doi: 10.1016/j.rser.2021.111171
WMO (2024). Devastating Rainfall Hits Spain in Yet Another Flood-Related Disaster. Available online at: https://wmo.int/media/news/devastating-rainfall-hits-spain-yet-another-flood-related-disaster (accessed January 16, 2025).
Xu, W., Jimenez-Bescos, C., Pantua, C. A. J., Calautit, J., and Wu, Y. (2021). A coupled modelling method for the evaluation of the impact of pavement solar collector on urban air temperature and thermal collection. Futur. Cities Environ. 7, 1–16. doi: 10.5334/fce.109
Yang, T., Liu, W., Kramer, G. J., and Sun, Q. (2021). Seasonal thermal energy storage: a techno-economic literature review. Renew. Sustain. Energy Rev. 139:110732. doi: 10.1016/j.rser.2021.110732
Zachariah, M., Fioravanti, G., Acosta Navarro, J., Kimutai, J., Dosio, A., Pasotti, L., et al. (2024). Climate Change Key Driver of Extreme Drought in Water Scarce Sicily and Sardinia. WWA: World Weather Attribution. Retrieved from: https://coilink.org/20.500.12592/1yrjrxf (accessed March 28, 2025).
Keywords: high-density urban area, urban heat island effect, solar thermal collectors, cool pavements, thermal energy storage
Citation: Guarino S, Lo Brano V and Kosny J (2025) Understanding the transformative potential of solar thermal technology for urban sustainability. Front. Sustain. Cities 7:1583316. doi: 10.3389/frsc.2025.1583316
Received: 25 February 2025; Accepted: 24 March 2025;
Published: 08 April 2025.
Edited and reviewed by: James Evans, The University of Manchester, United Kingdom
Copyright © 2025 Guarino, Lo Brano and Kosny. This is an open-access article distributed under the terms of the Creative Commons Attribution License (CC BY). The use, distribution or reproduction in other forums is permitted, provided the original author(s) and the copyright owner(s) are credited and that the original publication in this journal is cited, in accordance with accepted academic practice. No use, distribution or reproduction is permitted which does not comply with these terms.
*Correspondence: Valerio Lo Brano, dmFsZXJpby5sb2JyYW5vQHVuaXBhLml0