- 1School of Population Health, Faculty of Medical and Health Sciences, The University of Auckland, Auckland, New Zealand
- 2School of Environment, Faculty of Science, The University of Auckland, Auckland, New Zealand
- 3Department of Civil and Environmental Engineering, Faculty of Engineering, The University of Auckland, Auckland, New Zealand
As air pollution varies significantly in both space and time, commuter exposure may differ considerably depending on the route taken between home to work. This is especially the case for active mode commuters who often have a wider range of route choices available to them compared with those traveling by private motor vehicle or by public transport. The aim of this study was to investigate the effect of route choice on air pollution exposure among active commuters, and to estimate, based on modeling, the health benefits able to be achieved from air pollution exposure reductions, modeled across a population, through route optimization. We searched for studies that used portable personal air pollution monitoring equipment during active mode commuting, and reported measurements of air quality on at least two routes, either as a journey to work or to school. The World Health Organization (WHO) model AirQ+ was then exploited to estimate the premature deaths attributable to air pollution according to route choice. Ten publications were identified that met the inclusion criteria. Ultrafine particle counts (UFP), black carbon (BC), and carbon monoxide (CO) were the most commonly measured pollutants in the studies identified. The exposures associated with “high exposure” and “low exposure” routes (categorized based on differences in traffic counts on the roads along the commute route or walking on opposite sides of the road with different levels of traffic traveling in each direction) were found to vary on average by 30 ± 8%, 42 ± 35%, and 55 ± 17% for BC, CO and UFP, respectively. On the basis of modeling, and on the estimated exposures to BC, up to 36 out of 10,000 deaths could be prevented by choosing a low exposure route compared with a high exposure route during active commuting. The results of this study may be useful for both individuals in their commute planning, and also for urban transport planners as impetus for investing in infrastructure to support healthy active mode commuting.
Introduction
Over the past half a century, urbanization and the reliance on passive transport (especially private cars) have led to low levels of physical activity across the world (World Health Organization, 2020c), which is a major risk factor for mortality (World Health Organization, 2010). More than 1.4 billion people of the world's population are deemed to not be sufficiently active to be healthy (Thornton, 2018), with insufficient physical activity contributing to a higher risk of cardiovascular disease, Type 2 diabetes, dementia, and some cancers (Guthold et al., 2018).
The promotion of active transportation (AT) is one of the strategies that has been used by governments to help address the issue of inactivity (Goodin, 2015). While AT has traditionally been considered to be walking, running and cycling, this category includes any type of transport powered by human energy (Litman, 2018). AT can, to a large extent, provide sufficient physical activity to remain healthy (Shoham et al., 2015) and, if rolled out at scale, worldwide, it could potentially prevent 3.2 million deaths due to inactivity (Guthold et al., 2018). AT is also associated with environmental improvements, and has been shown to also have social and cultural benefits (Hong, 2018). For instance, parents who choose active transportation influence their children to also be active, and employees using active transport have been shown to help in encouraging co-workers to change their choice of mode of transport to ones that are more active (Hong, 2018).
Active commuting is also a more environmentally sustainable mode of transport than the use of private vehicles as it requires little in the way of fossil fuels or cost (Banister, 2009; Page and Nilsson, 2017; Hong, 2018). Private cars also contribute more to soil and water pollution through the production of vehicle spray, and the dry deposition of particles from vehicles and roads dispersed into roads' verges and surface water (Schipper et al., 2007; Maibach et al., 2008; Hong, 2018).
Despite the important advantages of AT, there are some associated risks, with the fear of traffic injury being a common reason given by many for their preference for motorized transport over walking or cycling (Sonkin et al., 2006). While this perception is not unfounded [the risk of hospital admission due to traffic related crashes is higher for walkers and cyclists than for drivers (Mindell et al., 2012)], the risk is none-the-less low in comparison with many activities such as do-it-yourself repairs (DIY) at home, as well as common recreational activities (Chieng et al., 2017).
The risk that is often not taken into account in active transportation is the health impact caused by exposure to air pollution (Bigazzi and Figliozzi, 2014; Dirks et al., 2018). Active travelers tend to spend more time than passive commuters traveling the same distance, and experience higher minute ventilation, potentially increasing the net dose of air pollution received by the body and consequently, for the same origin/destination pair and the same route, active commuters may experience a higher dose of pollution compared with passive commuters (Bigazzi and Figliozzi, 2014; Cepeda et al., 2017; de Nazelle et al., 2017; Targino et al., 2018).
One approach that has been used to reduce active commuters' exposure and promote active commuting is through changes in the urban design of cities, such as increasing the separation between commuters and motorized road traffic. For example, in one study, it was found that a separation of 100 meters decreased ultrafine particle counts (or UFPs which are particles smaller than 100 nanometers in diameter), black carbon (BC) and carbon monoxide (CO) concentrations relative to the roadside by 60 to 80 percent (Zhu et al., 2002). Providing such a separated path has also been found to result in the public perception of active transport as safe from the point of view of road traffic injury (Hull and O'Holleran, 2014). Thus, creating the appropriate transport infrastructure for active commuters can encourage people to shift from private cars to active commuting (Caulfield et al., 2012). Another strategy can be to limit road traffic, particularly HCVs (heavy commercial vehicles including buses and trucks) in specific areas, and to redesignate these as active commuter areas, as shown by Mueller et al. (2020).
In the absence of any information regarding air pollution exposure and route choice, active commuters tend to choose routes that involve the least number of directional changes or the shortest distance (Shatu et al., 2019). Given that the shortest route is not always the one associated with the lowest air pollution dose (Armeni and Chorianopoulos, 2013), the provision of information regarding air pollution and traffic could help commuters shift toward lower exposure routes (Bunds et al., 2019). The information could be provided using either traditional environmental monitoring equipment or low-cost sensors with community involvement provided at a grass-roots level (Mahajan et al., 2020). As such, governments may exploit citizen science (a process in which people with potentially minimal relevant experience become involved in data collection) to provide fine-grained information using mobile apps and low-cost sensors (Mahajan et al., 2020). In such a case, academic scientists are often responsible for implementing and interpreting the results (Eitzel et al., 2017). In this case, this would be in relation to identifying the least polluted routes, and estimates of health gains, for example, that could be achieved by using such routes.
Exposure assessment at the individual level is a key tool for assessing health impacts and developing suitable exposure-reduction policies. Traditionally, estimates have been made based on fixed-site monitoring, supplemented by exposure modeling, albeit with a high degree of uncertainty due to the high spatial and temporal variability of air pollution levels (Dias and Tchepel, 2018). A more reliable and accurate way of estimating personal exposure is through the use of personal portable air pollution monitoring devices in combination with a Global Position System (GPS) to track location (Dias and Tchepel, 2018). Consequently, a large and growing body of literature has explored individual's exposure using such devices (Strak et al., 2010; Jarjour et al., 2013; Dirks et al., 2016, 2018; Good et al., 2016; Pattinson et al., 2017; Hofman et al., 2018; Jereb et al., 2018; Brand et al., 2019; Luengo-Oroz and Reis, 2019). While such studies provide, at the individual level, reliable exposure information, they do not generally link directly to health outcomes. In particular, there is a lack of research into the health benefits that could be achieved though route optimization.
In recent years, there has been a growing interest in the use of health impact assessments (HIA) to estimate the potential benefits to health associated with various interventions (Mueller et al., 2015). Many models have been developed for such a purpose, including those published by the European Study of Cohorts for Air Pollution Effects (ESCAPE) project and the American Cancer Society (ACS) (Malmqvist et al., 2018). The World Health Organization (WHO) model AirQ+ (World Health Organization, 2020b) is designed to allow estimation of both the long- and short-term health impacts of exposure to different pollutants based on a concentration-response function extracted from epidemiological studies. In light of the many health effects attributed to combustion-related BC, including all-cause and cardiopulmonary mortality (Janssen et al., 2012; Kirrane et al., 2019), this study links evidence from the literature about the reduction in exposure to BC able to be achieved through route optimization, and uses an HIA approach to estimate the associated predicted human health benefits.
This paper begins with a systematic review of measurement studies that have used portable air quality monitoring equipment to investigate differences in air pollution exposure experienced by active mode commuters' exposure, taking different routes for the same origin-destination pair. The extent of health benefit that may be able to be achieved through route optimization is then estimated using AirQ+ software.
Materials and Methods
Systematic Review
The systematic review was performed based on the PRISMA guidelines for the reporting of systematic reviews (Moher et al., 2009). The search was conducted in October 2019 using the following databases: MEDLINE, Web of Science, and Scopus. The following keywords were used in the search:
(“route choice” or (route AND optimi*) OR “short* path”) AND (“air pollut*” OR “air quality” OR emission* OR “traffic pollut*” OR (pollut* AND exposure)) AND (walk* OR bicycl* OR cyclist OR cycling OR bik* OR pedestrian OR “active commut*” OR “active transport*”)
The search was restricted to publications written in the English language and that included an abstract. The eligible papers also had to meet all of the following criteria:
1. Air pollution data were measured directly (not modeled) using portable air pollution monitors.
2. Air pollution exposure was measured for at least two routes for the same origin and destination pair.
3. Quantitative results of dose/exposure were reported for the time when the routes were traversed.
4. The percentage reduction achieved by taking the lowest exposure route compared to the highest exposure route was reported or able to be calculated based on the figures provided.
The study data collected included air pollution exposure, sample size, location, time and date of sampling, and target group (children or adults). The literature search, study selection, data extraction, and synthesis were performed between February and March 2020. The primary outcome measure was the percentage difference in exposure between the highest and lowest exposure route. The mean exposures for the high and low exposure routes were extracted. If the study involved more than two routes, the route with the highest exposure was selected and compared with the route with the lowest exposure.
Estimate of Health Effects
Based on the results of the systematic review, the long-term health benefits, able to be achieved by taking the low exposure route rather than the high exposure route, were estimated. This was based on the predicted health effects of exposure to BC for a commuter who travels the same route every working day for a year. All-cause mortality was chosen as it is an important public health benchmark, providing more robust results than cause-specific mortality and morbidity (Rojas-Rueda et al., 2016). The proportion of deaths attributable to BC was also able to be provided by AirQ+ on the basis of limited data input requirements.
To estimate the annual mean exposure to air pollution, a series of assumptions were made. Firstly, it was assumed that a person spends 1 h per day [which is the average time of daily travel to work for many countries (Silveira Neto et al., 2014; Perry, 2016)] over 236 days of a year in the transport microenvironment (assuming 25 days of holidays and 104 weekend days), and that a person is exposed to background levels of BC at other times. The mean exposures for the person commuting the high exposure route and the low exposure route were calculated for each of the reviewed studies. By dividing the difference by 24, the daily difference (for a weekday) of exposure was calculated. This number was then multiplied by the fraction of working days over a year to get the yearly reduction in the long-term exposure to BC for a commuter who consistently follows the low-exposure route compared with the high exposure route. The background concentration was calculate by assuming (arbitrarily) the ratio of the background to the average concentration along the low exposure route to be 1, 0.5, 0.33, 0.25, and 0.20. On this basis, the annual exposures for both the high and low exposure routes were calculated for each of the studies identified in the systematic review. The final output is the annual mean predicted BC exposure for a given route.
To estimate the long-term health effects of exposure to BC, AirQ+ was used (World Health Organization, 2020b). This model employs concentration-response functions derived from a systematic review of epidemiological studies until 2013 (World Health Organization, 2020b). In contrast with other models, such as ACS and ESCAPE, each of which was developed for a specific region and for a limited number of pollutants, AirQ+ includes BC (which is not included in the other models) and is considered to be applicable across a range of countries, including those in Western Europe and in North America (World Health Organization, 2020b).
To quantify the attributable proportion of all-cause mortality using AirQ+, the model requires a cut-off value of concentration (we considered this to be zero) and air quality data, including long-term exposure to BC (above the cut-off). The attributable proportion is given by Ansari and Ehrampoush (2019) as
where RR(c) is the relative risk for the health endpoint in a specific category of exposure (c), and p(c) is the proportion of the population in the category of exposure (c). For calculating the RR, the default values provided by AirQ+ were used, and a linear-log method was exploited to estimate the relative risks associated with different exposures. In this study, the differences in the estimated attributable proportion of deaths for high and low exposure routes have been considered to be the contribution to mortality reduction of the least polluted commuting route compared with the most polluted route.
Results
This section is divided in three subsections. The first part explains the results of the search. Then, a description of the extent of reduction in exposure to pollutants found in the searched studies is provided. Finally, a health impact assessment approach, used to estimate the health benefit able to be achieved through route optimization for air pollution exposure, is presented.
Search Result
In total, 333 studies were identified with 25, 72, and 233 from Medline, Scopus, and the Web of Science, respectively, and another three studies identified from other sources. Of the 333 studies, 24 were duplicates and were thus removed. Additionally, MR screened the titles manually and deleted 31 more studies because of duplication. After screening the title and abstract, 18 papers were considered to meet our inclusion criteria. The full texts were then analyzed to assess for eligibility. Eight papers were considered to be eligible according to our criteria. Two more studies were included through cross-references, leaving a total of 10 studies for the systematic review (Figure 1).
Table 1 shows the main characteristics of the included studies, such as the pollutant of interest, the mode of travel, the country and the city in which data collection took place, the population density of the city, the study group, the mean and standard deviation of the exposure for each route, the number and time of day of the trips, and the mean percentage difference associated with the low exposure route compared with the high exposure route.
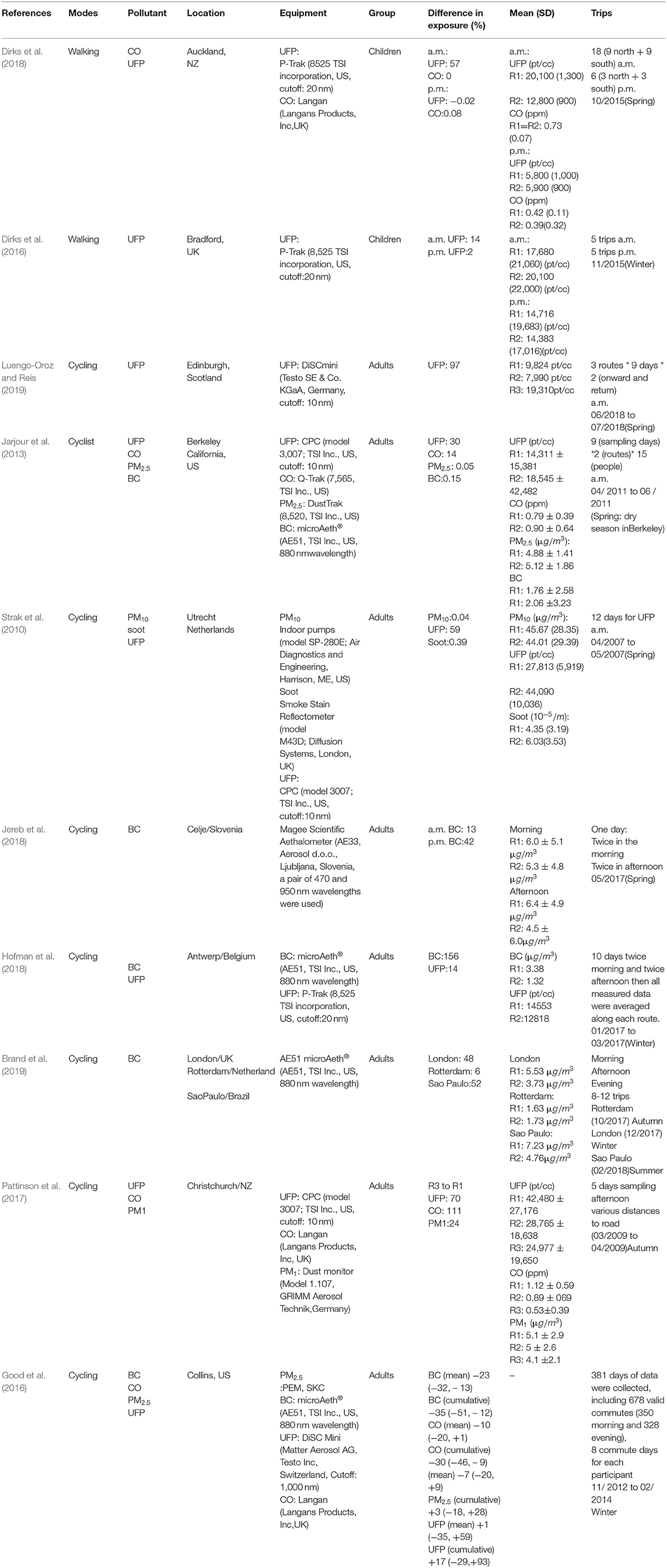
Table 1. Main characteristics of the studies included in the systematic review (Int = interval, Pop den = Population density, cutoff: the threshold for the size of particles).
Exposure Reduction
The most commonly measured pollutants were UFP (seven studies), BC (five studies), and CO (four studies). However, other pollutants, including PM2.5 and PM1, (particulate matter of <2.5 and 1 micrometers in diameter) were also measured. The mean ratios of high-to-low exposure were found to be 1.30 ± 0.08, 1.55 ± 0.16 and 1.42 ± 0.035 for BC, UFP and CO, respectively. In each of the studies, two routes were compared with respect to the active commuters' exposure to air pollution. One of the routes was through high traffic roads (or on the high traffic side of the road), and the other route was either away from traffic or on the low traffic side of the road. The percentage reduction in exposure between routes varied depending on the type of pollutant, the time of day, data collection location and the device used.
Figure 2 shows concentration differences in UFP ranging from 7,990 to 44,090 pt/cc, depending on the device used and the study setting. By reviewing the dates of the data collection for the different studies measuring UFP exposure, it was found that older studies tended to result in higher mean exposures compared with the more recent studies. For example, the highest mean UFP exposure was observed in the study by Strak et al. (2010) in which data collection took place in April 2007, while the second highest exposure to UFP was measured in Christchurch by Pattinson et al. (2017) based on data collected in 2009. However, in the other studies, the UFP concentration in the high exposure route was found to be close to 20,000 pt/cc or less.
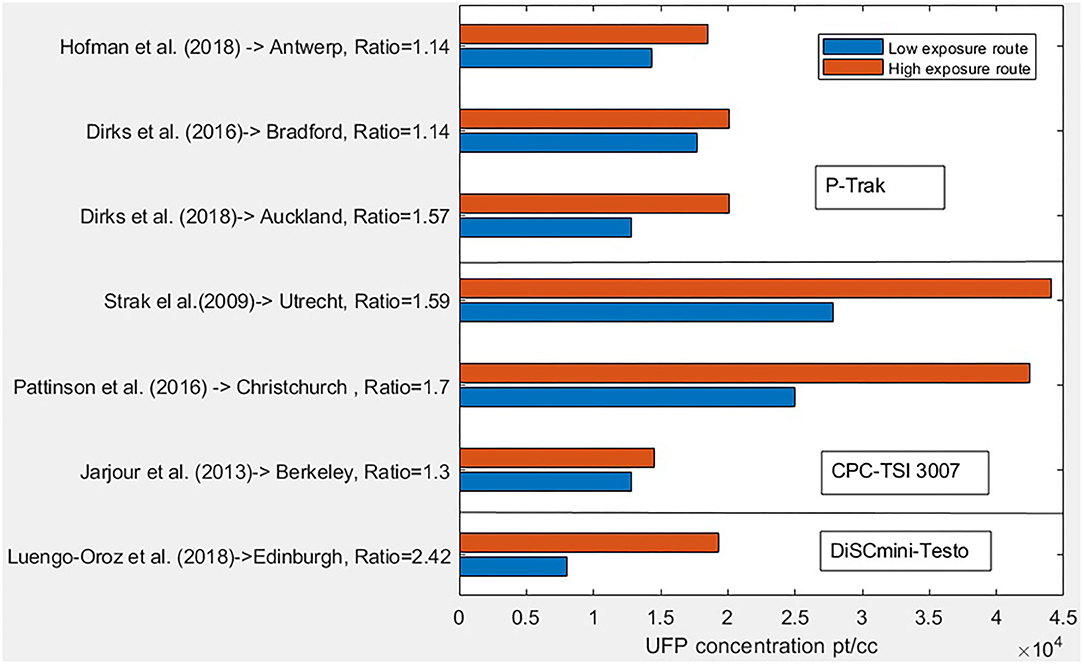
Figure 2. Comparison of the concentration of UFP in the studies. High exposure is in orange color and the low exposure in blue. The ratio is the mean of high exposure divided to the low exposure route.
BC was measured in four of the studies. The study by Brand et al. (2019) measured BC at three different cities (Figure 3). In this study, the mean reduction in exposure between routes was found to be 1.21 (SD = 0.97) μg/m3. The highest values of BC were observed by Brand et al. in Sao Paulo and the lowest in Rotterdam (Brand et al., 2019). Furthermore, the highest reduction in exposure to BC achieved by choosing the low exposure route compared to the highest route was found in the study conducted in Sao Paulo (Brand et al., 2019).
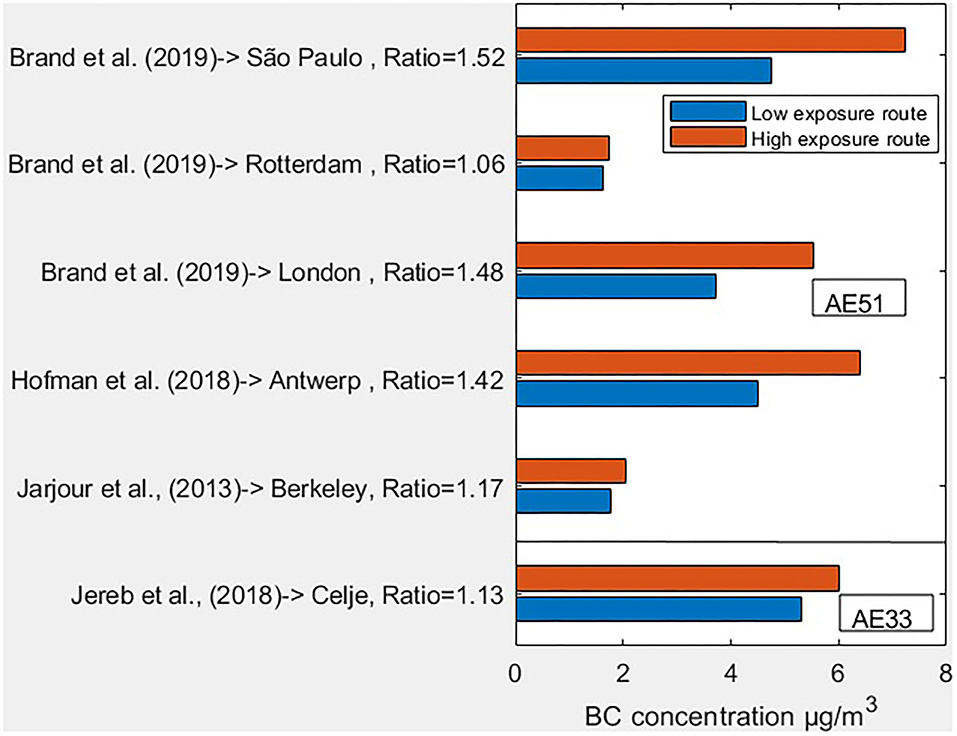
Figure 3. Comparison of low and high exposure routes in different cities. The blue bar shows the mean of the low exposure route. The high exposure route is illustrated in orange color. Brand et al. (2019) measured BC exposure for the cyclist in three routes in three different cities. We used the mean of three low exposure and three high exposure routes for each city.
Four studies measured CO. One of these reported only the percentage difference in the mean exposure (−10%, CI=-20 ± 1), which was found to be not significant. The other three studies provided the numerical means for each route (Figure 4). The mean reduction was found to be 0.23 (SD = 0.31) ppm. The highest ratio of reduction was found in a study by Pattinson et al. (2017) based on measurements made in 2009. This suggests that higher levels of concentration tend to be recorded in older studies carried at a time when the vehicle fleet technology was less advanced.
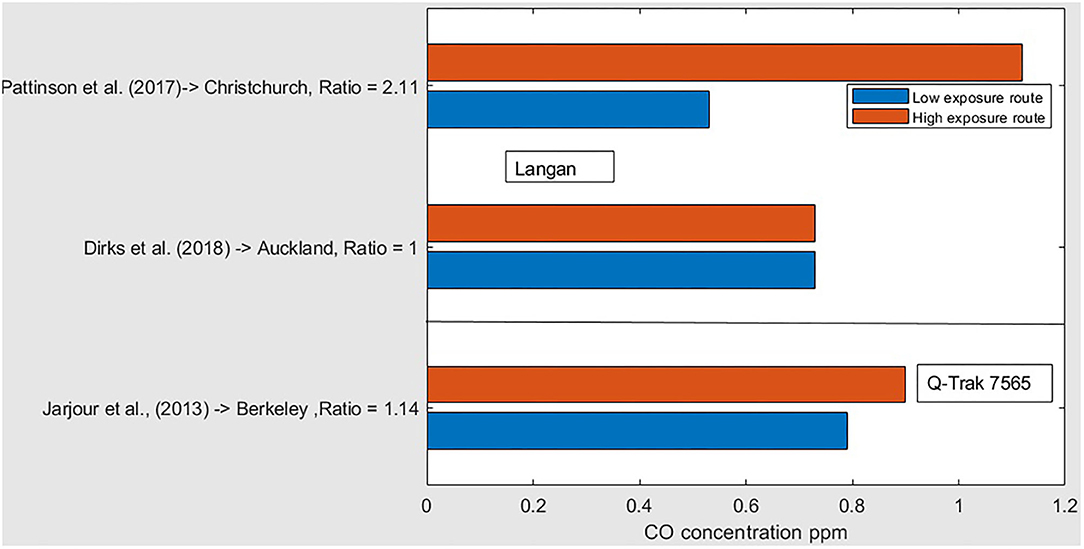
Figure 4. Comparison of concentration of CO in low and high exposure route in the studies. High exposure is in orange color and the low exposure in blue. The ratio is the mean of high exposure divided to the low exposure route.
In some studies, other pollutants were measured, including soot, PM2.5, PM10, and PM1. In two studies, PM2.5 was considered (Jarjour et al., 2013; Good et al., 2016). In both studies, the reduction in mean exposure between routes was found to be not significant. Strak et al. reported 8.10 μg/m3 and 1.68 ×10−5/m reductions in cyclist's exposure to PM10 and soot, respectively. One study considered PM1 (Pattinson et al., 2017) and observed a decrease of 1 μg/m3.
In these studies, various instruments (with different settings) were used for the measurement of pollutants. For example, three different devices [the CPC TSI 3007 (Strak et al., 2010; Jarjour et al., 2013; Pattinson et al., 2017), P-Trak (Dirks et al., 2016, 2018; Hofman et al., 2018), DiscMini (Luengo-Oroz and Reis, 2019), and MiniDiSC (Good et al., 2016) were used to measure UFP numbers for particle sizes in the range of 0.01 to 1 μm, 0.02 to 1 μm (Matson et al., 2004), 0.01 to 0.3 μm (Testo SE and Co, 2019), 0.01 to 0.3 μm (NANEOS, 2020)]. All of the studies presented in this review used an AE51 for measuring black carbon (Jarjour et al., 2013; Hofman et al., 2018; Brand et al., 2019), except for one (Jereb et al., 2018) which used an AE33. The aethalometer AE51 measures only BC at a wavelength of 880 nm, and is used to detect black carbon. An AE33 can be operated using various different wavelengths. However, in the study by Jereb et al. (2018), a pair of wavelengths, 470 and 950 nm, was used to measure traffic-related BC. In some studies, soot and BC were considered to be the same, although there are fundamental differences between them (Long et al., 2013). Optical methods were used to measure BC but for the quantification of EC, Strak et al. (2010) post-processed PM10 filters using a Smoke Stain Reflectometer (model M43D; Diffusion Systems, London, UK) and transformed the results into absorbance. For evaluating CO, two instruments were used: a Langan (Good et al., 2016; Dirks et al., 2018) and a Qtrak (Jarjour et al., 2013).
Of the ten studies included in the review, five compared either morning and afternoon or evening exposures (Dirks et al., 2016, 2018; Hofman et al., 2018; Jereb et al., 2018; Brand et al., 2019). Of the five studies, three considered UFP, one considered CO and three considered BC. For the studies that considered UFP, the ratios of the morning-to-afternoon concentrations were found to be 2.80, 1.30, and 1.65 for Auckland, New Zealand (Dirks et al., 2018), Bradford, UK (Dirks et al., 2016) and Antwerp, Belgium (Hofman et al., 2018), respectively. The one study (Auckland) that measured CO (as well as UFP), reported a ratio of morning to afternoon mean concentration of 1.04 (Dirks et al., 2018). Mixed results were found for BC. It was shown that in Sao Paulo, Brazil, Rotterdam, The Netherlands, and Antwerp, Belgium, the mean morning exposures to BC were 49, 13, and 33% higher than in the evening, respectively. The morning-to-afternoon black carbon levels measured in Celje, Slovenia were found to be 0.94 and 1.12 on the main and alternative routes, respectively. Brand et al. (2019) also observed lower concentrations in the morning relative to the evening, with a ratio of 0.93. Therefore, although the differences in exposure between morning and afternoon or evening varied, most studies found average exposures to be higher during the morning rush hour than at other times of the day.
Long-Term Health Outcomes
Using AirQ+, the Estimated Attributable Proportion of the all-cause mortality– fraction of mortality attributed to exposure to black carbon for both the high and low exposure routes were calculated based on the average commuting exposures, along with the differences between the two routes. Four studies provided cyclists' exposure to BC in six different cities. Based on the data presented in the studies reviewed, the mean exposures and background concentrations were calculated based on the measurements made for the different cities, and for when the ratios of the background concentration to the low exposure route are 1, 2, 3, 4, and 5, as presented in Table 2. Given that the background concentrations are not the same around the world [some cities (including Auckland) tend to have cleaner air, while many cities have much worse conditions], the five different background concentrations for each city were calculated by dividing the low exposure route to 1, 2, 3, 4, and 5 to account for the variability in background concentration. The annual exposure was computed by choosing low and high exposure routes and considering various background exposures (Table 3). The results of the model output from AirQ+ are presented in Table 4. Note that the differences in the attributable proportion of mortality is affected significantly by route choice, though the levels of background concentration also have an influence, with the relative difference being smaller in conditions of high levels of background. On average across cities, the difference in attributed proportions was found to be 0.17%, indicating that, on average, 17 of 10,000 deaths (of the people biking 1 h per day to and from their work) could be prevented by taking a lower exposure commuting route. In Sao Paulo, where the highest difference in exposure between routes was recorded, on the basis of this methodology, up to 36 in 10,000 deaths may be able to be prevented (Brand et al., 2019).
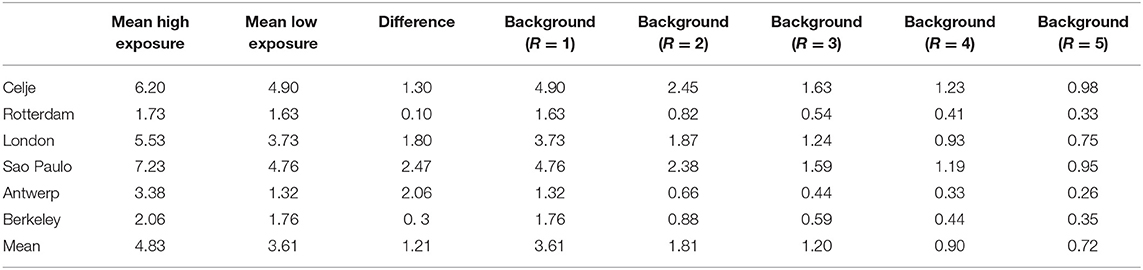
Table 2. The reduction in exposure to pollutants by taking an alternative route in cycling (R = ratio of mean low exposure route to background, the unit for all numbers is μg/ m3).
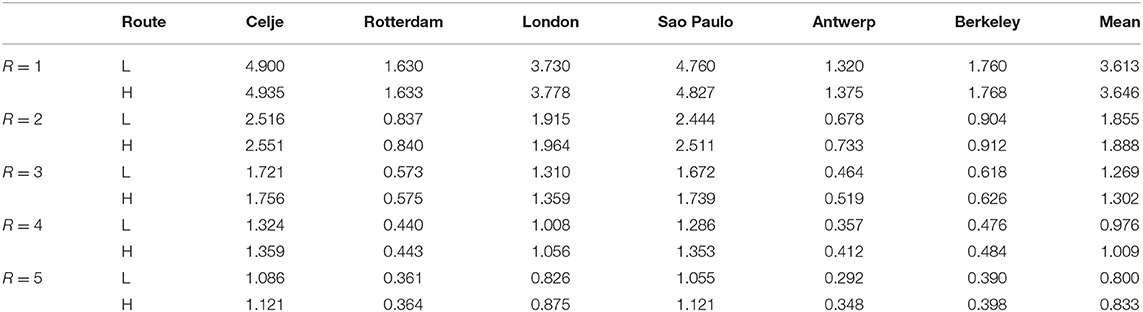
Table 3. Mean annual BC exposure by taking each route (L = low exposure, H = high exposure route, the unit for all numbers is μg/h.m3, R = ratio of mean low exposure route to background).
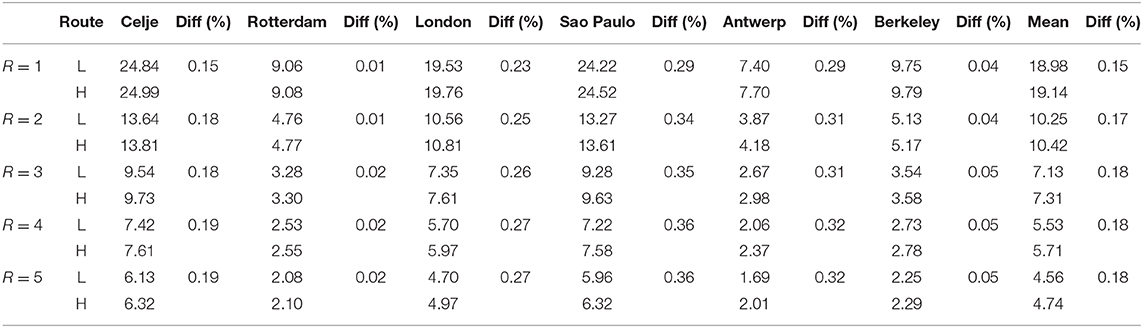
Table 4. Estimated attributable proportion by taking the routes based on the measured black carbon (Diff = difference in attributable proportion, L = low exposure, H = high exposure route).
Discussion
This systematic review compiled papers investigating the extent to which reductions in air pollution exposure could be achieved by the selection of lower exposure alternative routes by active mode commuters. The review showed that, generally, using alternative routes significantly decreases active commuters' exposure to air pollution, with average reductions of 55, 42, and 30 percent for UFP, BC, and CO, respectively. The extent of the reductions varied depending on the nature of the alternative routes considered, with more significant reductions observed for those commuting through green spaces, for example, where the route involved significant separation from the road.
The measured exposure depends on many factors which can vary from one pollutant to another. These include the instruments used for capturing pollutant concentrations, the time of day, the season and the year of data collection, the fleet composition, and the population density. Furthermore, factors such as passing construction sites, intersections and heavy duty vehicles were shown to lead to spikes in exposure to UFP whilst active traveling (Dirks et al., 2018; Luengo-Oroz and Reis, 2019). Significant reductions in exposure can thus be achieved by avoiding these sources by, perhaps temporary, changes in routes.
While CO is mostly associated with emission exhaust from petrol cars (Kingham et al., 2013), diesel vehicles such as trucks and buses are responsible for high rates of emissions of UFP (Luengo-Oroz and Reis, 2019) and BC (Longley et al., 2019); Heavy-duty vehicles, generally powered using diesel fuel, emit higher amounts of BC (Kumar et al., 2018) and UFP (Knibbs et al., 2011) per vehicle than petrol cars. Note that the ratio of the emission factors between heavy-duty vehicles and light-duty vehicles has been estimated to be about four for BC (de Miranda et al., 2019) and up to 40 for UFP (Xiang et al., 2019). High traffic roads tend to have larger numbers of heavy-duty vehicles than roads with relatively light traffic (i.e., low numbers of vehicles of all kinds) (Jarjour et al., 2013).
The estimation of the long-term effect of exposure to black carbon in relation to route choice showed that, based on the average exposure of all studies, 17 of 10,000 deaths would be able to be prevented if active mode commuters took the associated low exposure route rather than the high exposure route for their journey to work. Based on the highest difference between the low and high exposure routes, which was recorded in Sao Paulo, it is estimated that up to 36 in 10,000 deaths are able to be prevented on the basis of the route. By comparing the impact of various levels of background concentration, it was found that when the background concentration was higher, the effect of route choice was smaller. However, if other pollutants and also short-term peaks in exposure are considered (which are commonly observed in active traveling), the number of preventable deaths could be significantly more.
It should be taken into account that active commuters are at high risk of air pollution exposure; they may inhale higher amounts of air pollution compared with other commuters, due to their close proximity to the source of air pollution, their often longer travel times and their increased minute ventilation (Bigazzi and Figliozzi, 2014; Cepeda et al., 2017; Targino et al., 2018). Additionally, exposure to spikes in pollution (a high concentration of air pollution for a short period of time) during walking or cycling may also result in adverse health effects (Michaels and Kleinman, 2000; Dons et al., 2019). However, it has been reported that as long as the average air pollution exposure throughout the commute remains below 1.1 and 2 times the background level for a cyclist and walker, respectively, on average, the benefits of active travel can be considered to outweigh the health risk of air pollution exposure (Johan de Hartog et al., 2010). However, exposure to traffic-related pollution may negate the benefits of active travel when commuting along high-trafficked routes, particularly for people with pre-existing conditions such as COPD and ischemic heart disease (Sinharay et al., 2018).
There are some limitations associated with this review that need to be recognized. Although the search terms used were comprehensive, the review was limited to articles written in English; non-English studies were excluded. The lack of Asian and African studies is acknowledged. A small number of cities in this study were analyzed. All of the cities except one (Sao Paulo) were from developed countries, indicating a lack of studies in least developed and developing countries. However, had a larger number of studies from cities from developing, least developed as well as developed countries been included in the study, a larger range of differences in exposure between low and high exposure routes may have been found. In this study, for each of the publications included in the review, only two routes were investigated: a high exposure route and a low exposure route. However, many active commuting routes include a mix of low and high exposure sections along the route, sometimes with choice available, and sometimes not. Thus, is may not always be possible to choose a low exposure route for the whole of the journey. In the present study, only the air pollution exposure along the routes was considered, with other factors influencing an active commuter's route choice, including the presence of trees and the route topography, ignored.
The Health Impact Assessment aspect of this study included a number of assumptions. For example, we assumed that the commuter spends 1 h per weekday traveling. Additionally, the estimates of health impacts are based only on what is known about the health effects associated with one pollutant (BC). Had other pollutants been considered, the results might have been different. However, BC is considered to be one of the pollutants most strongly associated with adverse health outcomes (Magalhaes et al., 2018). Additionally, only the long-term health impacts were considered; the short-term effects induced by exposure to spikes in air pollution, should also be taken into account in evaluating the risks associated with high exposure routes compared with low exposure routes.
It is also noted that there are limitations associated with the use of AirQ+ for estimating the health risks. For example, the estimate of relative risks rely on studies taken place in situations of high pollution (World Health Organization, 2020a). Also, the model equations are based on studies carried in Western Europe and North America (World Health Organization, 2020a), with little input provided from other continents with cities also affected by traffic-related air pollution. Therefore, model predictions may not be reliable for these regions. The model also assumes that ambient concentrations are reliable indicators of population exposure (World Health Organization, 2020a). Thus, invariably, there is a risk of, introducing bias in the estimation as a result (Evangelopoulos et al., 2020). Furthermore, the equations used for estimating the health risks are based on concentration-response functions in which many assumptions have been made. This includes issue of transferability, the generalizability of health impacts from one population to another, and the use of individual pollutants derived from single-pollutant statistical models rather than from a mixture of pollutants (World Health Organization, 2020a), which may or may not be realistic, depending on the specific situation.
This research has identified many questions that are also worthy of further investigation. In particular, future research is needed to examine more closely the links between the nature of vehicle fleets and traffic congestion, and their impact on air pollution exposure. Additionally, there is limited information on the health effects associated with exposure to short-term spikes in air pollution. Thus, there is abundant room for further progress to be made in terms of understanding the health consequences of exposure to the intermittent spikes in concentration typical of traffic pollution concentration traces.
Furthermore, there remains a lack of research regarding children and elderly people, especially given these groups are more vulnerable to the adverse effects of air pollution (Friedrich, 2018). Children's organs are not fully developed, hence their respiratory and immune systems are less efficient than those of adults (Zielinska and Hamulka, 2019). Moreover, epidemiological studies suggest that excess mortality due to air pollution is greater among older age groups (especially 75+) (Simoni et al., 2015).
The review also suggests that there is a lack of studies focused specifically on walking. Walkers are more susceptible to traffic-related air pollution due to the long travel time as walking generally takes longer to travel the same distance compared to other modes of transport, and because of walkers' close proximity to traffic. Walkers are also at risk of exposure to short-term spikes since there are no physical barriers between the source of traffic pollution and pedestrians (Dirks et al., 2016). Therefore, more research is required to determine the benefits of choosing alternative routes with respect to exposure amongst children and the walking mode of transport.
It is also acknowledged that in many cities, particularly in developing or least developed countries, the selection of low exposure routes and the associated avoidance of exposure to air pollution might simply not be feasible. In the short term, an economic alternative could be to encourage active travelers to wear effective masks, such as N95, that can result in significant reductions in exposure to airborne particles (Kyung et al., 2020). This could be particularly effective for susceptible groups of the population and people using various open vehicles (e.g., motor scooters, open delivery vehicles, etc.) who are exposed to large amounts of traffic pollution due to their mode choice and the amount of time spent in the transport microenvironment. It is noted that wearing a mask should be considered to be a short-term solution to limit exposure to air pollution exposure in anticipation of more effective solutions, including the widespread introduction of clean vehicle technology.
Conclusions
The main goal of the current study was to review studies investigating the role of route choice on the air pollution exposure of active mode commuters. The results clearly indicate that significant reductions can be obtained by choosing low traffic routes away from traffic congestion. Moreover, identifying the cleanest path from the point of view of air pollution is important in active commuters' route planning, as even a modest reduction in daily air pollution exposure can lead to a significant reduction in exposure to a population as a whole, or for an individual when considering their cumulative exposure over a period of years. People, especially those who walk/cycle the same route every day, would benefit from information about air pollution for the purpose of route planning.
Governments play a crucial role in helping to reduce active commuters' exposure to air pollution by the provision of transport infrastructure that is conducive to improved localized air quality. Although the most important factor in active commuters' route choice is often the distance involved or the number of directional changes required, route choice is a complex function of other parameters, considered in varying amounts, including safety aspects and route aesthetics. Planners should consider identifying and targeting paths of low exposure, in addition to active commuters' influencing factors regarding preferred routes when considering further infrastructure development, thus encouraging more journeys along such routes. Furthermore, active commuters themselves may also have an important role to play, with opportunities for community participation via the use of mobile apps to help in finding the least polluted routes and in raising awareness regarding air pollution (Mahajan et al., 2020). This would help to increase the levels of participation in active transport and makes the city more liveable (Koh and Wong, 2013) while promoting active commuters' health.
Data Availability Statement
The original contributions presented in the study are included in the article/supplementary materials, further inquiries can be directed to the corresponding author/s.
Author Contributions
Data extraction was conducted by MR. It was subsequently sent to KD for cross-checking and consultation. MR screened all of the papers and carried out the health impact assessment. The extracted data were crosschecked by KD who monitored all levels of the screening. AW suggested adding a health impact assessment. KD supervised the project. All authors discussed the results and contributed to the final manuscript by providing critical feedback.
Conflict of Interest
The authors declare that the research was conducted in the absence of any commercial or financial relationships that could be construed as a potential conflict of interest.
References
Ansari, M., and Ehrampoush, M. H. (2019). Meteorological correlates and AirQ(+) health risk assessment of ambient fine particulate matter in Tehran, Iran. Environ. Res. 170, 141–150. doi: 10.1016/j.envres.2018.11.046
Armeni, I., and Chorianopoulos, K. (2013). “Pedestrian navigation and shortest path: preference versus distance,” in The 9th International Conference on Intelligent Environments, eds J. A. Botía and D. Charitos, 647–652. doi: 10.3233/978-1-61499-286-8-647
Bigazzi, A. Y., and Figliozzi, M. A. (2014). Review of urban bicyclists' intake and uptake of traffic-related air pollution. Transp. Rev. 34, 221–245. doi: 10.1080/01441647.2014.897772
Brand, V. S., Kumar, P., Damascena, A. S., Pritchard, J. P., Geurs, K. T., and Andrade, M. D. F. (2019). Impact of route choice and period of the day on cyclists' exposure to black carbon in London, Rotterdam and São Paulo. J. Transp. Geogr. 76, 153–165. doi: 10.1016/j.jtrangeo.2019.03.007
Bunds, K. S., Casper, J. M., Hipp, J. A., and Koenigstorfer, J. (2019). Recreational walking decisions in urban away-from-home environments: the relevance of air quality, noise, traffic, and the natural environment. Transp. Res. F Traffic Psychol. Behav. 65, 363–375. doi: 10.1016/j.trf.2019.08.006
Caulfield, B., Brick, E., and McCarthy, O. T. (2012). Determining bicycle infrastructure preferences – a case study of Dublin. Transp. Res. D Transp. Environ. 17, 413–417. doi: 10.1016/j.trd.2012.04.001
Cepeda, M., Schoufour, J., Freak-Poli, R., Koolhaas, C. M., Dhana, K., Bramer, W. M., et al. (2017). Levels of ambient air pollution according to mode of transport: a systematic review. Lancet Public Health 2, e23–e34. doi: 10.1016/S2468-2667(16)30021-4
Chieng, M., Lai, H., and Woodward, A. (2017). How dangerous is cycling in New Zealand? J. Transp. Health 6, 23–28. doi: 10.1016/j.jth.2017.02.008
de Miranda, R. M., Perez-Martinez, P. J., de Fatima Andrade, M., and Ribeiro, F. N. D. (2019). Relationship between black carbon (BC) and heavy traffic in São Paulo, Brazil. Transp. Res. D Transp. Environ. 68, 84–98. doi: 10.1016/j.trd.2017.09.002
de Nazelle, A., Bode, O., and Orjuela, J. P. (2017). Comparison of air pollution exposures in active vs. passive travel modes in European cities: a quantitative review. Environ. Int. 99, 151–160. doi: 10.1016/j.envint.2016.12.023
Dias, D., and Tchepel, O. (2018). Spatial and temporal dynamics in air pollution exposure assessment. Int. J. Environ. Res. Public Health 15:558. doi: 10.3390/ijerph15030558
Dirks, K. N., Salmond, J. A., and Talbot, N. (2018). Air pollution exposure in walking school bus routes: a New Zealand case study. Int. J. Environ. Res. Public Health 15:2802. doi: 10.3390/ijerph15122802
Dirks, K. N., Wang, J. Y. T., Khan, A., and Rushton, C. (2016). Air pollution exposure in relation to the commute to school: a Bradford UK case study. Int. J. Environ. Res. Public Health 13:1064. doi: 10.3390/ijerph13111064
Dons, E., Laeremans, M., Orjuela, J. P., Avila-Palencia, I., de Nazelle, A., Nieuwenhuijsen, M., et al. (2019). Transport most likely to cause air pollution peak exposures in everyday life: evidence from over 2000 days of personal monitoring. Atmos. Environ. 213, 424–432. doi: 10.1016/j.atmosenv.2019.06.035
Eitzel, M. V., Cappadonna, J. L., Santos-Lang, C., Duerr, R. E., Virapongse, A., West, S. E., et al. (2017). Citizen science terminology matters: exploring key terms. Citizen Sci. Theory Practice 2, 1–20. doi: 10.5334/cstp.96
Evangelopoulos, D., Katsouyanni, K., Keogh, R. H., Samoli, E., Schwartz, J., Barratt, B., et al. (2020). PM2.5 and NO2 exposure errors using proxy measures, including derived personal exposure from outdoor sources: a systematic review and meta-analysis. Environ. Int. 137:105500. doi: 10.1016/j.envint.2020.105500
Friedrich, M. J. (2018). Global impact of air pollution on children's health. JAMA 320, 2412–2412. doi: 10.1001/jama.2018.19559
Good, N., Molter, A., Ackerson, C., Bachand, A., Carpenter, T., Clark, M. L., et al. (2016). The Fort collins commuter study: impact of route type and transport mode on personal exposure to multiple air pollutants. J. Expo. Sci. Environ. Epidemiol. 26, 397–404. doi: 10.1038/jes.2015.68
Goodin, H. (2015). Promoting Physical Activity at the Local Government Level. Wellington: Agencies for Nutrition Action.
Guthold, R., Stevens, G. A., Riley, L. M., and Bull, F. C. (2018). Worldwide trends in insufficient physical activity from 2001 to 2016: a pooled analysis of 358 population-based surveys with 1·9 million participants. Lancet Glob. Health 6, e1077–e1086. doi: 10.1016/S2214-109X(18)30357-7
Hofman, J., Samson, R., Joosen, S., Blust, R., and Lenaerts, S. (2018). Cyclist exposure to black carbon, ultrafine particles and heavy metals: an experimental study along two commuting routes near Antwerp, Belgium. Environ. Res. 164, 530–538. doi: 10.1016/j.envres.2018.03.004
Hong, A. (2018). “2 - environmental benefits of active transportation,” in Children's Active Transportation, ed R. Larouche (Elsevier).
Hull, A., and O'Holleran, C. (2014). Bicycle infrastructure: can good design encourage cycling? Urban Plann. Transport Res. 2, 369–406. doi: 10.1080/21650020.2014.955210
Janssen, N. A., Gerlofs-Nijland, M. E., Lanki, T., OSalonen, R., Cassee, F., Hoek, G., et al. (2012). Health Effects of Black Carbon. Geneva: World Health Organization.
Jarjour, S., Jerrett, M., Westerdahl, D., De Nazelle, A., Hanning, C., Daly, L., et al. (2013). Cyclist route choice, traffic-related air pollution, and lung function: a scripted exposure study. Environ. Health 12:14. doi: 10.1186/1476-069X-12-14
Jereb, B., Batkovič, T., Herman, L., Šipek, G., Kovše, Š., Gregorič, A., et al. (2018). Exposure to black carbon during bicycle commuting–alternative route selection. Atmosphere 9:21. doi: 10.3390/atmos9010021
Johan de Hartog, J., Boogaard, H., Nijland, H., and Hoek, G. (2010). Do the health benefits of cycling outweigh the risks? Environ. Health Perspect. 118, 1109–1116. doi: 10.1289/ehp.0901747
Kingham, S., Longley, I., Salmond, J., Pattinson, W., and Shrestha, K. (2013). Variations in exposure to traffic pollution while travelling by different modes in a low density, less congested city. Environ. Pollut. 181, 211–218. doi: 10.1016/j.envpol.2013.06.030
Kirrane, E. F., Luben, T. J., Benson, A., Owens, E. O., Sacks, J. D., Dutton, S. J., et al. (2019). A systematic review of cardiovascular responses associated with ambient black carbon and fine particulate matter. Environ. Int. 127, 305–316. doi: 10.1016/j.envint.2019.02.027
Knibbs, L. D., Cole-Hunter, T., and Morawska, L. (2011). A review of commuter exposure to ultrafine particles and its health effects. Atmos. Environ. 45, 2611–2622. doi: 10.1016/j.atmosenv.2011.02.065
Koh, P. P., and Wong, Y. D. (2013). Influence of infrastructural compatibility factors on walking and cycling route choices. J. Environ. Psychol. 36, 202–213. doi: 10.1016/j.jenvp.2013.08.001
Kumar, P., Patton, A. P., Durant, J. L., and Frey, H. C. (2018). A review of factors impacting exposure to PM2.5, ultrafine particles and black carbon in Asian transport microenvironments. Atmos. Environ. 187, 301–316. doi: 10.1016/j.atmosenv.2018.05.046
Kyung, S. Y., Kim, Y., Hwang, H., Park, J.-W., and Jeong, S. H. (2020). Risks of N95 face mask use in subjects with COPD. Respir. Care 65, 658–664. doi: 10.4187/respcare.06713
Litman, T. (2018). Evaluating Active Transport Benefits and Costs. Victoria, BC: Victoria Transport Policy Institute.
Long, C. M., Nascarella, M. A., and Valberg, P. A. (2013). Carbon black vs. black carbon and other airborne materials containing elemental carbon: physical and chemical distinctions. Environ. Pollut. 181, 271–286. doi: 10.1016/j.envpol.2013.06.009
Longley, I., Tunno, B., Somervell, E., Edwards, S., Olivares, G., Gray, S., et al. (2019). Assessment of spatial variability across multiple pollutants in Auckland, New Zealand. Int. J. Environ. Res. Public Health 16:1567. doi: 10.3390/ijerph16091567
Luengo-Oroz, J., and Reis, S. (2019). Assessment of cyclists' exposure to ultrafine particles along alternative commuting routes in Edinburgh. Atmos. Pollut. Res. 10, 1148–1158. doi: 10.1016/j.apr.2019.01.020
Magalhaes, S., Baumgartner, J., and Weichenthal, S. (2018). Impacts of exposure to black carbon, elemental carbon, and ultrafine particles from indoor and outdoor sources on blood pressure in adults: a review of epidemiological evidence. Environ. Res. 161, 345–353. doi: 10.1016/j.envres.2017.11.030
Mahajan, S., Kumar, P., Pinto, J. A., Riccetti, A., Schaaf, K., Camprodon, G., et al. (2020). A citizen science approach for enhancing public understanding of air pollution. Sust. Cities Soc. 52:101800. doi: 10.1016/j.scs.2019.101800
Maibach, M., Schreyer, C., Sutter, D., Van Essen, H., Boon, B., Smokers, R., et al. (2008). Handbook on Estimation of External Cost in the Transport Sector: Produced Within the Study Internalisation Measures and Policies for All external Cost of Transport (IMPACT). Delft: The European Commission DG TREN.
Malmqvist, E., Oudin, A., Pascal, M., and Medina, S. (2018). Choices behind numbers: a review of the major air pollution health impact assessments in Europe. Curr. Environ. Health Rep. 5, 34–43. doi: 10.1007/s40572-018-0175-2
Matson, U., Ekberg, L. E., and Afshari, A. (2004). Measurement of ultrafine particles: a comparison of two handheld condensation particle counters. Aerosol Sci. Technol. 38, 487–495. doi: 10.1080/02786820490462200
Michaels, R. A., and Kleinman, M. T. (2000). Incidence and apparent health significance of brief airborne particle excursions. Aerosol Sci. Technol. 32, 93–105. doi: 10.1080/027868200303803
Mindell, J. S., Leslie, D., and Wardlaw, M. (2012). Exposure-based, ‘Like-for-Like’ assessment of road safety by travel mode using routine health data. PLoS ONE 7:e50606. doi: 10.1371/journal.pone.0050606
Moher, D., Liberati, A., Tetzlaff, J., Altman, D. G., and Group, P. (2009). Preferred reporting items for systematic reviews and meta-analyses: the PRISMA statement. PLoS Med. 6:e1000097. doi: 10.1371/journal.pmed.1000097
Mueller, N., Rojas-Rueda, D., Cole-Hunter, T., de Nazelle, A., Dons, E., Gerike, R., et al. (2015). Health impact assessment of active transportation: a systematic review. Prev. Med. 76, 103–114. doi: 10.1016/j.ypmed.2015.04.010
Mueller, N., Rojas-Rueda, D., Khreis, H., Cirach, M., Andres, D., Ballester, J., et al. (2020). Changing the urban design of cities for health: the superblock model. Environ. Int. 134:105132. doi: 10.1016/j.envint.2019.105132
NANEOS (2020). Personal Aerosol Monitor. Available online at: http://fierz.ch/minidisc/pdf/miniDiSC.pdf (accessed March 20, 2020).
Page, N. C., and Nilsson, V. O. (2017). Active commuting: workplace health promotion for improved employee well-being and organizational behavior. Front. Psychol. 7:1994. doi: 10.3389/fpsyg.2016.01994
Pattinson, W., Kingham, S., Longley, I., and Salmond, J. (2017). Potential pollution exposure reductions from small-distance bicycle lane separations. J. Transp. Health 4, 40–52. doi: 10.1016/j.jth.2016.10.002
Perry, M. J. (2016). To Bring Attention to the 23% “Gender Commute Time Gap” I Introduce the New “Equal Commute Day” on April 14. Available online at: https://www.aei.org/carpe-diem/to-bring-attention-to-the-23-gender-commute-time-gap-i-introduce-the-new-equal-commute-day-on-april-14/ (accessed August 06, 2020).
Rojas-Rueda, D., de Nazelle, A., Andersen, Z. J., Braun-Fahrlander, C., Bruha, J., Bruhova-Foltynova, H., et al. (2016). Health impacts of active transportation in Europe. PLoS ONE 11:e0149990. doi: 10.1371/journal.pone.0149990
Schipper, P. N. M., Comans, R. N. J., Dijkstra, J. J., and Vergouwen, L. (2007). Runoff and windblown vehicle spray from road surfaces, risks and measures for soil and water. Water Sci. Tech. 55, 87–96. doi: 10.2166/wst.2007.076
Shatu, F., Yigitcanlar, T., and Bunker, J. (2019). Shortest path distance vs. least directional change: empirical testing of space syntax and geographic theories concerning pedestrian route choice behaviour. J. Transp. Geogr. 74, 37–52. doi: 10.1016/j.jtrangeo.2018.11.005
Shoham, D. A., Dugas, L. R., Bovet, P., Forrester, T. E., Lambert, E. V., Plange-Rhule, J., et al. (2015). Association of car ownership and physical activity across the spectrum of human development: modeling the Epidemiologic Transition Study (METS). BMC Public Health 15, 173–173. doi: 10.1186/s12889-015-1435-9
Silveira Neto, R., Duarte, G., and Páez, A. (2014). Gender and commuting time in São Paulo Metropolitan region. Urban Stud. 52, 298–313. doi: 10.1177/0042098014528392
Simoni, M., Baldacci, S., Maio, S., Cerrai, S., Sarno, G., and Viegi, G. (2015). Adverse effects of outdoor pollution in the elderly. J. Thorac. Dis. 7, 34–45. doi: 10.3978/j.issn.2072-1439.2014.12.10
Sinharay, R., Gong, J., Barratt, B., Ohman-Strickland, P., Ernst, S., Kelly, F. J., et al. (2018). Respiratory and cardiovascular responses to walking down a traffic-polluted road compared with walking in a traffic-free area in participants aged 60 years and older with chronic lung or heart disease and age-matched healthy controls: a randomised, crossover study. Lancet 391, 339–349. doi: 10.1016/S0140-6736(17)32643-0
Sonkin, B., Edwards, P., Roberts, I., and Green, J. (2006). Walking, cycling and transport safety: an analysis of child road deaths. J. R. Soc. Med. 99, 402–405. doi: 10.1177/014107680609900817
Strak, M., Boogaard, H., Meliefste, K., Oldenwening, M., Zuurbier, M., Brunekreef, B., et al. (2010). Respiratory health effects of ultrafine and fine particle exposure in cyclists. Occup. Environ. Med. 67, 118–124. doi: 10.1136/oem.2009.046847
Targino, A. C., Rodrigues, M. V. C., Krecl, P., Cipoli, Y. A., and Ribeiro, J. P. M. (2018). Commuter exposure to black carbon particles on diesel buses, on bicycles and on foot: a case study in a Brazilian city. Environ. Sci. Pollut. Res. In.t 25, 1132–1146. doi: 10.1007/s11356-017-0517-x
Testo SE and Co. (2019). Testo DiSCmini - Handheld Measuring Instrument for Nanoparticle Counting. Available online at: https://www.testo.com/en-US/testo-discmini/p/133 (accessed October 30, 2019).
Thornton, J. (2018). A quarter of people are not being active enough to stay healthy. BMJ 362:k3796. doi: 10.1136/bmj.k3796
World Health Organization (2010). “Physical activity for health” in Global Recommendations on Physical Activity for Health (Geneva: World Health Organization).
World Health Organization (2020a). AirQ+: Key Features. Available online at: https://www.euro.who.int/__data/assets/pdf_file/0007/309994/AirQ1-0-Key-features.pdf (accessed December 10, 2020).
World Health Organization (2020b). AirQ+: Software Tool for Health Risk Assessment of Air Pollution. Available online at: http://www.euro.who.int/en/health-topics/environment-and-health/air-quality/activities/airq-software-tool-for-health-risk-assessment-of-air-pollution (accessed February 25, 2020).
World Health Organization (2020c). Physical Inactivity: A Global Public Health Problem. Available online at: https://www.who.int/dietphysicalactivity/factsheet_inactivity/en/ (accessed May 08, 2020).
Xiang, S., Yu, Y. T., Hu, Z., and Noll, K. E. (2019). Characterization of dispersion and ultrafine-particle emission factors based on near-roadway monitoring part ii: heavy duty vehicles. Aerosol Air Q. Res. 19, 2421–2431. doi: 10.4209/aaqr.2019.08.0386
Zhu, Y., Hinds, W. C., Kim, S., Shen, S., and Sioutas, C. (2002). Study of ultrafine particles near a major highway with heavy-duty diesel traffic. Atmos. Environ. 36, 4323–4335. doi: 10.1016/S1352-2310(02)00354-0
Keywords: air pollution, route choice, health outcome, active commuters, systematic review
Citation: Rafiepourgatabi M, Woodward A, Salmond JA and Dirks KN (2021) The Impact of Route Choice on Active Commuters' Exposure to Air Pollution: A Systematic Review. Front. Sustain. Cities 2:565733. doi: 10.3389/frsc.2020.565733
Received: 26 May 2020; Accepted: 16 December 2020;
Published: 28 January 2021.
Edited by:
Thomas Krafft, Maastricht University, NetherlandsReviewed by:
Pim Martens, Maastricht University, NetherlandsMark Rosenberg, Queen's University, Canada
Copyright © 2021 Rafiepourgatabi, Woodward, Salmond and Dirks. This is an open-access article distributed under the terms of the Creative Commons Attribution License (CC BY). The use, distribution or reproduction in other forums is permitted, provided the original author(s) and the copyright owner(s) are credited and that the original publication in this journal is cited, in accordance with accepted academic practice. No use, distribution or reproduction is permitted which does not comply with these terms.
*Correspondence: Mehrdad Rafiepourgatabi, m.rafiepour@auckland.ac.nz