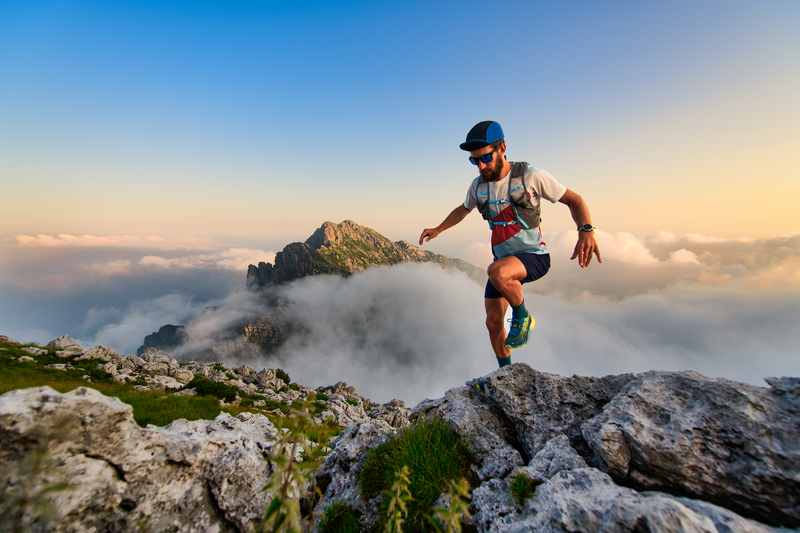
94% of researchers rate our articles as excellent or good
Learn more about the work of our research integrity team to safeguard the quality of each article we publish.
Find out more
REVIEW article
Front. Sustain. , 12 February 2025
Sec. Resilience
Volume 6 - 2025 | https://doi.org/10.3389/frsus.2025.1453170
This article is part of the Research Topic Climate Change and Sustainable Food Security: Prospects and Challenges of Feeding 9 Billion People View all articles
Crop wild relatives (CWRs) - wild plant taxa genetically closely related to domesticated plants - are considered an alternative pathway to solving global food insecurity in a changing climate. However, their potential contribution is undermined by fundamental knowledge gaps in taxa diversity, distributions, taxonomic affiliations, conservation strategies, and valuable traits. To address these gaps, we reviewed the literature on the progress made between 2000 and 2021 in support of in situ conservation and use of CWRs under the changing climate in five thematic areas focusing on the genus Vigna: (1) species diversity, global distribution, conservation status, gene pools, and importance of the genus; (2) CWR-in situ conservation-protected area debate; (3) cultivation and domestication of CWR populations; (4) adaptive response to drought stress; and (5) adaptive response to Striga stress. We report that 104 Vigna CWR species in five subgenera, Ceratotropis, Haydonia, Lasiosporon, Plectotropis, and Vigna, are distributed mostly in Africa and Asia. Nine species are domesticated while six are threatened. Vigna gene pools remain poorly understood. Many Vigna CWRs provide various ecosystem services for human and environmental health. Attention is increasing towards in situ conservation of CWRs, within and outside protected areas, and complemented by ex situ conservation approaches. Several Vigna CWR taxa exhibit good agronomic traits for potential cultivation and neo-domestication. Many taxa have demonstrated tolerance to drought stress and race-specific Striga resistance. We conclude that if effectively conserved and used, Vigna CWRs can contribute to sustainable and climate-resilient food systems, either as wild edible plants, new plants for neo-domestication or novel sources of genetic material for use in pre-breeding programmes to improve the resilience, quantity and quality of Vigna domesticates under the changing climate. This study could stimulate further research and policy change for effective CWR conservation and use for sustainable food security in a changing climate.
With the changing climate, providing sustainable global food security for the growing human population while halting biodiversity loss is one of the greatest challenges facing humanity in the 21st century (Godfray, 2014; Mehrabi et al., 2018; Myers et al., 2017; Springmann et al., 2018; Tilman et al., 2011). Climate change is predicted to drastically reduce the yield of major crops, such as wheat (6.0%), rice (3.2%), maize (7.4%) and soybean (3.1%) by the end of the century (Zhao et al., 2017). Yet, global food supplies should increase by over 62% to feed nearly 10 billion people by 2050 (Godfray et al., 2010; Tilman et al., 2011; van Dijk et al., 2021). Given that no single pathway can solve this challenge (Ruben et al., 2021; Springmann et al., 2018), global strategies for food systems transformation to adapt to climate change have been proposed. These include transformation to sustainable food production and climate-friendly healthy diets (Ruben et al., 2021; Steiner et al., 2020; Willett et al., 2019), reducing food inequalities (HLPE, 2023), and halving food loss and waste (Geyik et al., 2022). However, region-specific adaptation strategies that can transition smallholder farmers to become more resilient to climate change, especially in food and nutrition insecurity hotspots such as sub-Saharan Africa (SSA), are attracting more attention (Cohn et al., 2017; Ghanem, 2022; Mutyasira, 2023; Sulaiman et al., 2023; van Zonneveld et al., 2023; Zhao et al., 2017).
Here, we report on the literature review of research on the progress made in support of the effective conservation and use of crop wild relatives (CWRs)—wild plant species that are genetically closely related to domesticated species (Maxted et al., 2006)—which are widely considered a strategic option for transitioning (Dempewolf et al., 2014; Ghanem, 2022; Pironon et al., 2019; Satori et al., 2021; Sulaiman et al., 2023). For instance, Pironon et al. (2019) have shown that CWRs can provide resilience to agricultural production in SSA where many of the 29 major crops are likely to fail in the new climatic envelopes. Consequently, interest in CWRs has been growing worldwide since the mid-1990s (Figure 1).
Figure 1. Trend of publications for crop wild relatives since 1974 (based on the raw number of hits in PubMed alone) (accessed June 2023). One article was published in 1974, 13 in 1994, 51 in 2004, 210 in 2014, 572 in 2022 and 352 by June 2023. The increase beginning from the mid-1990s coincides with the Convention on Biological Diversity (CDB) coming into force in 1993. The sharp rise immediately after 2010, and immediately thereafter, coincides with The Second Report on the State of the World’s Plant Genetic Resources for Food and Agriculture (FAO, 2010) and FAO’s Second Global Plan of Action for Plant Genetic Resources for Food and Agriculture (FAO, 2011).
Three reasons may explain this trend. First, it is believed that CWRs can help increase the food quantity either as donors of novel genes for pre-breeding programmes to improve crop yield, as direct food resources or as new crops through cultivation and neo-domestication (Dempewolf et al., 2014; Maxted et al., 2010; Singh et al., 2019; Sulaiman et al., 2023; Tomooka et al., 2014; Zhang et al., 2018). According to Smýkal et al. (2018), it is risky to have global food security dependent on <20 domesticated species, with rice, wheat and potato providing nearly 95% of the world’s caloric intake. Second, most CWR taxa are nutrient-dense (Castañeda-Álvarez et al., 2016; Harouna et al., 2018); therefore, they could improve the food quality and ameliorate the malnutrition burden with a relatively lower carbon footprint (Ferrari et al., 2022; Heusala et al., 2020). This comes against a background of increasing global malnutrition levels (Willett et al., 2019), coupled with growing concerns over animal-based proteins for their hidden environmental and human health costs (Ferrari et al., 2022; Godfray et al., 2018), which are shifting global diets towards plant-based nutritious foods (Aimutis, 2022; Willett et al., 2019). Lastly, it is widely believed that the breadth of diversity inherent in CWRs could help in developing a climate-resilient food production system (Bohra et al., 2021; Castañeda-Álvarez et al., 2016; Dempewolf et al., 2014; McCouch et al., 2013; Zhang et al., 2017). This is considering the increasing vulnerability of the current domesticated varieties to climate-induced abiotic and biotic stresses (Kapazoglou et al., 2023; Pironon et al., 2019; Zhao et al., 2017), and model predictions of increased frequency and severity of these stresses, e.g., drought (Dai, 2013; Trenberth et al., 2014) and parasitic weeds (David et al., 2022; Ronald et al., 2017), respectively.
Moreover, the actual and potential uses of many CWR taxa in improving economically important crops have been extensively documented (Coyne et al., 2020; Dempewolf et al., 2017; Hajjar and Hodgkin, 2007; Mammadov et al., 2018; Muñoz et al., 2017; Tirnaz et al., 2022), with substantial contribution to the global economy estimated at US$120–186 billion annually (Brozynska et al., 2015; Dulloo et al., 2021; PricewaterhouseCoopers, 2013; Tyack et al., 2020). According to Brozynska et al. (2015), about 30% of modern crop production since 1945, worth hundreds of billions of US dollars worldwide, is attributable to the use of CWRs in plant breeding programmes. Further, CWRs have high visibility in the global political and business agenda (e.g., CBD, 2005, 2010, 2022; FAO, 2009, 2011). Taken together, this provides an urgency for effective conservation and sustainable use of CWRs to secure vital ecosystem services in a warming world (Jaradat, 2015; Tyack et al., 2020), potentially contributing to the attainment of several UN Sustainable Development Goals (SDGs) (e.g., Goal 1—End poverty; Goal 2—Zero hunger; Goal 10—Reduced inequalities; Goal 13—Resilience and adaptation to climate change) (United Nations, 2015).
Unfortunately, CWRs are threatened in their natural environments by various factors including land-use change and anthropogenic-induced climate change (Ford-Lloyd et al., 2011; Goettsch et al., 2021; Jarvis et al., 2008; Khoury et al., 2020a; Magos Brehm et al., 2022). Further, they are rarely a primary focus of conservation strategies, especially in situ conservation (Hunter et al., 2011; Petitpierre et al., 2023). Furthermore, they remain largely under-conserved, with in situ conservation still very incipient whereas ex situ is a bit more advanced though still inadequate, and are also underutilised in breeding programmes (Castañeda-Álvarez et al., 2016; Ford-Lloyd et al., 2011; Maxted and Magos Brehm, 2023). This is attributed to various fundamental knowledge gaps, including limited information regarding their conservation and beneficial traits (Khoury et al., 2020b; Maxted and Magos Brehm, 2023; van Zonneveld et al., 2020). These factors have motivated the urgency for effective conservation and use enhancement of CWRs over the past decades.
This study reviews strides made between 2000 and 2021 in support of the conservation of CWRs and the promotion of their use in light of climate change, focussing on the genus Vigna Savi. It is premised on the conceptual framework that considers Vigna CWRs as a natural resource based in the wild with the potential to contribute to sustainable global food and nutrition security. We perceive that two overarching pillars are associated with Vigna CWRs, conservation on the one hand, and use on the other. Conservation ensures the availability for the present and the future, while use is crucial for their recognition and valuation in conservation efforts (Catarino et al., 2021; Dempewolf et al., 2017; Engels and Thormann, 2020; Khoury et al., 2020b). To inform decision-making for effective conservation and use in this two-way interaction, fundamental knowledge of Vigna CWR taxa such as spatio-temporal distribution, habitat characteristics (floristic composition), local community knowledge associated with the taxa, and valuable traits for adaptation to abiotic (e.g., drought) and biotic (e.g., Striga) stress factors is needed (Figure 2).
Figure 2. Conceptual framework for effective in situ conservation and use of Vigna crop wild relatives.
Several review studies supporting the conservation and use of Vigna CWR plant genetic resources (PGR) have been undertaken (e.g., Bisht and Singh, 2013; Harouna et al., 2018; Maxted et al., 2004; Tomooka et al., 2002). However, these studies have not addressed the climate change dimesion, one of the major threats to biodiversity and food security in the century. The current study aims to provide an understanding of the efforts undertaken to support conservation work and use enhancement of Vigna CWRs under the changing climate. We proceed by first, providing an update on the species diversity, global distribution, conservation status, gene pools, and importance of the genus. Next, we tackle the CWR-in situ conservation-protected area debate with a particular emphasis on those of the genus Vigna. Then, we provide efforts made towards the cultivation and domestication of populations of Vigna CWR taxa. Next, we explore the adaptive response to drought stress of Vigna CWR taxa. Lastly, we review the adaptive response to Striga stress of Vigna CWR taxa. We have used the term “use” to refer to the direct use of CWRs by local communities or utilisation by plant breeders following Engels and Thormann (2020).
We have borrowed elements of a systematic review study in our approach (Haddaway et al., 2015), although we do not intend to provide a typical systematic review. For theme one (Species richness, native distribution), we used three online species databases (1) Plants of the World Online (POWO),1 (2) International Legume Database and Information System (ILDIS),2 and (3) African Plant Database (APD).3 We used the POWO as the principal database following Catarino et al. (2021). The ILDIS is a specialised database, while the APD has an African regional focus, one of the native distribution areas for the genus Vigna Savi.
For the remaining four themes, we followed and modified the approach used by Powell et al. (2015). We used two online databases, Google Scholar and PubMed, to obtain our literature (original research papers, books, book chapters, conference proceedings, manuals and reports) published in English between 2000 and 2021. We used the search terms “Vigna crop wild relatives,” “Vigna wild relatives,” “wild Vigna,” and “Vigna.” From the top 200 hits in each database, we selected the most popular papers addressing our themes and excluded those that dealt exclusively with domesticated taxa. We also applied snowballing (Wohlin, 2014), tracking the references and citations of the selected publications covering the same period (2000–2021).
Further, we tracked the work of experts on the genus Vigna. We did this based on the following two considerations: (i) the genus Vigna is a complex taxon with many species (Maxted et al., 2004; Pratap et al., 2018; van Zonneveld et al., 2020), whose information may be fragmented in the literature and therefore difficult to capture details of all the taxa (Powell et al., 2015); (ii) Vigna is a neglected and underutilised taxon (Harouna et al., 2018); as such, research effort on many such taxa is, to a large extent, done at the mercy of a few dedicated scholars (Assogbadjo et al., 2021). As such, we used expert relations and knowledge to track publications in the areas of our interest that could not be picked by our initial search approach (Powell et al., 2015). Experts in the genus Vigna are mostly based at the University of Birmingham (UK), the World Vegetable Centre in Taiwan, the International Institute for Tropical Agriculture (IITA) in Nigeria, the National Bureau of Plant Genetic Resources (NBPGR) in India, the Plant Genetic Resources Conservation Unit (PGRCU) at the University of Georgia USDA-ARS, USA, the Genetic Research Centre - National Agricultural and Food Research (NARO) in Japan, Institut de l’Environnement et de Recherches Agricoles (INERA) in Burkina Faso, and University of Virginia (USA). Eleven publications addressing four thematic areas were finally selected and retained for this review (Table 1).
The genus Vigna (Savi, 1824) belongs to the tribe Phaseoleae of the family Fabaceae. It is a warm pantropical and subtropical taxon comprising a diversity of wild and cultivated species. The species richness of the genus varies with authors. For instance, Lewis et al. (2005) report <80 species; Delgado-Salinas et al. (2011) suggest >100 species; >200 species are suggested by Pratap et al. (2018); >88 species (van Zonneveld et al., 2020); 104 species by Somta et al. (2019); and 105 species by Catarino et al. (2021). Five subgenera of Vigna have been recognised, namely, Ceratotropis, Haydonia, Lasiosporon, Plectotropis, and Vigna (Delgado-Salinas et al., 2011; Takahashi et al., 2016; Verma et al., 2022). Nine species have been domesticated from three subgenera (Takahashi and Tomooka, 2020; Verma et al., 2022). These are adzuki bean [Vigna angularis (Wild) Ohwi and Ohashi], black gram or urd bean [Vigna mungo (L.) Hepper], creole bean (Vigna reflexo-pilosa Hayata), moth bean [Vigna aconitifolia (Jacq.) Marechal], mung bean [Vigna radiata (L.) Wilczek], and rice bean [Vigna umbellata (Thunb.) Ohwi and Ohashi] from subgenus Ceratotropis (Asian Vigna); one species, zombie pea [Vigna vexillata (L.) A. Rich] from subgenus Plectotropis; and two species, Bambara ground nut [Vigna subterranea (L.) Verdc.] and cowpea [Vigna unguiculata (L.) Walpers] from Vigna (African Vigna). A recent semi-domesticated species from Asia, Vigna stipulacea Kuntze, has also been reported in previous studies (Harouna et al., 2018; Takahashi et al., 2016).
Variations in the reported species richness of the genus Vigna may be due to the different authors’ treatment of infraspecies. Numerous morphotypes and high rates of intra-hybridization among the taxa have been reported (Maxted et al., 2004; Umdale et al., 2023) and several taxonomic complexes in the genus remain unpacked (Gore et al., 2019; van Zonneveld et al., 2020). As such, new information is still emerging leading to the movement of taxa within or between Vigna and closely related taxonomic groups like Phaseolus (Delgado-Salinas et al., 2011; Takahashi et al., 2018). Unpacking the correct taxonomic identifications in the genus would provide an important knowledge base to inform priority-setting for effective conservation and use enhancement of Vigna CWR germplasm (Engels and Thormann, 2020; Nair et al., 2023).
Therefore, to contribute to the body of knowledge on the species richness in the genus and their native distribution, we collated information from three online species databases, POWO, APD, and ILDIS. We retrieved 106 Vigna species from the POWO. We removed eight species: Vigna ambacensis Welw. ex Baker, a synonym of Vigna heterophylla A. Rich. based on the APD and ILDIS; Vigna fischeri Harms considered a synonym of Vigna luteola (Jacq.) Benth based on the APD and ILDIS; Vigna hainiana Babu, Gopin. & S. K. Sharma is a synonym of Vigna subramaniana (Babu ex Raizada) Raizada (Takahashi et al., 2018); Vigna jaegeri Harms is a synonym of Vigna luteola (Jacq.) Benth based on the APD and ILDIS; Vigna lobatifolia Baker following Catarino et al. (2021); Vigna nuda N.E.Br. is a synonym of Vigna antunesii Harms based on the APD and ILDIS; Vigna pubigera Baker is considered a synonym of Vigna heterophylla A. Rich. following the APD and ILDIS; Vigna wittei Baker f. is regarded as a synonym of Vigna radicans Welw. ex Baker following the APD and ILDIS.
We added six species to the list: Vigna desmodioides R. Wilczek based on the APD, ILDIS and Nduche et al. (2021); Vigna diffusa (Scott Elliott) A. Delgado & Verdc. (Delgado-Salinas et al., 2011, 2022); Vigna nakashimae (Ohwi) Ohwi & H. Ohashi and Vigna riukiuensis (Ohwi) Ohwi & H. Ohashi (Delgado-Salinas et al., 2011; Ogiso-Tanaka et al., 2023); Vigna schottii (Bentham) A. Delgado & Verdc (Delgado-Salinas et al., 2011, 2022); and Vigna trinervia (Heyne ex Wight & Arn.) Tateishi & Maxted (Delgado-Salinas et al., 2011; Pootakham et al., 2022; Takahashi et al., 2018). We therefore report 104 species in the genus Vigna belonging to five subgenera, namely, Ceratotropis, Haydonia, Lasiosporon, Plectotropis, and Vigna, their biology (longevity, habit, size, pubescence), and native distribution in Africa, America, Asia, and Australasia (Supplementary Table 1). Selected Vigna CWR species with wide African distribution are presented (Figure 3).
Figure 3. (A–D) Selected Vigna Crop wild relatives with wide distribution in Africa (A, Vigna reticulata; B, Vigna luteola; C, Vigna vexillata; D, Mature, dry pods and seeds of V. vexillata).
Most Vigna taxa display dual habits, annual or perennial, with perennials usually possessing rootstocks. The presence of rootstocks could be an adaptation to either arid conditions or seasonally burnt areas (Maxted et al., 2004). Further, most Vigna CWR taxa have various degrees of pubescence on their tissues like stems, leaves, and pods, which could be an adaptation to varying biotic and abiotic stress factors (Boukar et al., 2020; Maxted et al., 2004).
Understanding the threat status of a species is crucial in the conservation of PGR, as it may inform conservation decision-making about where to channel conservation efforts. However, unlike other wild plant species, threat status assessment of CWR using the IUCN Red List Categories and Criteria4 is generally recent. For instance, at the time of writing this article, only the African Vigna CWRs (Maxted et al., 2004), European CWRs (Kell et al., 2011), CWRs of the brinjal eggplant (Solanum melongena) (Syfert et al., 2016), CWRs of North Africa (Lala et al., 2018), coffee CWRs (Davis et al., 2019), CWRs of the United States of America (Khoury et al., 2020a) and the Mesoamerican CWRs (Goettsch et al., 2021) were elaborately reported. Consequently, only about 16–35% of all CWR taxa are assessed as threatened to date (Maxted and Magos Brehm, 2023). For the genus Vigna, 41 of 104 species (∼39%) have been assessed (Supplementary Table 1). Of these, six are threatened and all are African Vigna. One of them, Vigna dolomitica R. Wilczek is Critically Endangered (CR) (McFarlane and Maxted, 2019b), while the other five are Endangered (EN): Vigna bosseri Du Puy & Labat (McFarlane and Maxted, 2019a), Vigna desmodioides R. Wilczek (Maxted and McFarlane, 2019), Vigna keraudrenii Du Puy & Labat (Rhodes, 2016a), Vigna laurentii De Wild. (McFarlane and Maxted, 2019c) and Vigna monantha Thulin (Rhodes, 2016b).
Considering that many other species remain unassessed and most of those assessed are Data Deficient (DD) (Supplementary Table 1), many more Vigna CWR species might be threatened than is currently reported. Specific threats may not be known for most wild Vigna species (Maxted et al., 2004). However, based on the assessed Vigna CWR taxa, habitat loss and/or fragmentation (largely due to agricultural expansion, urbanisation, overgrazing, mining, and fires) is the major threat to Vigna CWR taxa (Maxted and McFarlane, 2019; McFarlane and Maxted, 2019a, 2019b; Rhodes, 2016a, 2016b). Climate change has also been reported (Jarvis et al., 2008; Manda et al., 2022; Vincent et al., 2019). In addition, overharvesting has been reported for some Vigna CWR taxa (Catarino et al., 2021). Therefore, increasing efforts ought to be made to evaluate the conservation status of the remaining described Vigna CWR taxa.
Harlan and De (1971) proposed the gene pool concept to provide a classification system for domesticated plant taxa and their wild relatives. The development of a gene pool concept marked an important step towards effective conservation and sustainable use of PGR, given the apparent inconsistencies among taxonomists regarding species limits (Maxted et al., 2006). In this case, the gene pool concept provides an informal classification of the species limits for a given target taxon, thus providing a tool for decision-making in the conservation and sustainable use of PGR (Engels and Thormann, 2020). The system uses the ease of crossing, such that CWR taxa can be classified into three categories, primary, secondary and tertiary gene pools. The primary gene pool (GP-1) within which GP-1A are the cultivated forms and GP-1B are the wild or weedy forms of the domesticated species (Maxted et al., 2006). The primary gene pool corresponds to the traditional biological species concept and is characterized by the ease of crossing, normal gene segregation and simple gene transfer. The secondary gene pool (GP-2) includes all biological species and coenospecies (less closely related species) from which gene transfer to the domesticated plant is possible but difficult using conventional breeding techniques. The GP-2 is characterised by the sterility of hybrids, poor or no pairing of chromosomes, and difficulties in obtaining mature hybrids or recovering desired traits in advanced generations (Maxted et al., 2006). The tertiary gene pool (GP-3) includes the taxa from which gene transfer to the domesticated plant is virtually impossible. The GP-3 is characterised by anomalous hybrids which may become lethal or completely sterile. This requires radical techniques such as embryo culture or genetic engineering to obtain normal hybrids (Maxted et al., 2006).
Based on the gene pool concept, van Zonneveld et al. (2020) have combined phylogenetics and crossing compatibility information to allocate Vigna CWR taxa in four gene pools, two under subgenus Vigna, and one each under subgenus Plectotropis and subgenus Ceratotropis (Figure 4). Evidently, Vigna gene pools remain poorly understood, largely due to limited information on their crossability with crops. Therefore, more information is required to unpack most Vigna species complexes.
Figure 4. Vigna gene pools (adapted and reprinted with permission from van Zonneveld et al., 2020).
While the gene pool concept best illustrates what constitutes a CWR, it has also been criticised for its shortcomings (Maxted et al., 2006). According to Maxted et al. (2006), the reliance of the concept on the relative ease of hybridization limits its application in conservation science, considering that many CWR taxa remain poorly studied. As such, Maxted et al. (2006) have suggested the use of a taxonomic group (TG) as an alternative. The authors have described five categories in this respect: Taxonomic Group (TG-1), with TG-1A and TG-1B representing the domesticated plant taxon (e.g., V. vexillata var. vexillata) and the same species as the domesticated plant (e.g., V. vexillata var. angustifolia), respectively; TG-2 being the same series or section as the domesticated plant species (e.g., V. kirkii); TG-3 being the same subgenus as the domesticated species (e.g., V. longissima); TG-4 being the same genus (e.g., Vigna spp.); and TG-5 being the same tribe but different genus to the domesticated species (e.g., Phaseolus spp.) (Maxted et al., 2006).
The potential importance of the genus Vigna for global food and nutrition security is well documented (e.g., Harouna et al., 2018). Its importance is owing to many factors. Many of its species are distributed in a wide range of harsh environments including salty, arid and semi-arid, and waterlogged areas (Maxted et al., 2004; Takahashi et al., 2016; Tomooka et al., 2014; van Zonneveld et al., 2020). This may offer diverse novel genes that have made them adapt to various stresses which could be used to improve domesticated Vigna. Moreover, even the domesticated Vigna such as cowpea and Bambara ground nut are comparably more versatile and adaptable to many environmental shocks such as drought stress than soybean and ground nut (Fatokun et al., 2012). Additionally, most Vigna taxa are densely nutritious, with various macro- and micro-nutrients reported in the literature (Boukar et al., 2011; Dakora, 2013; Tripathi et al., 2021). For instance, in addition to containing starch (13.21 ± 0.03%), Tripathi et al. (2021) report high levels of protein in the tubers of V. vexillata (9.93 ± 0.13%) in India which is seven and nine times greater than the content in potato (1.33 ± 0.21%) and cassava (1.03 ± 0.10%), respectively, thus making it one of the potentially protein-calorie crops. The agronomic and nutrition-dense traits in wild Vigna species may be used for developing more productive, nutritious, and climate-resilient Vigna crop varieties. Besides, abiotic stress-tolerant species could also be used as rootstocks for Vigna domesticates (Maxted et al., 2004; Tejada-Alvarado et al., 2023).
Further, most Vigna CWR taxa have versatile uses as food (root and tubers, pulses and vegetables) and feed (forage) (Catarino et al., 2021; Maxted et al., 2004; Tomooka et al., 2011). This makes the genus a potential suitable taxon for meeting the diverse social and cultural needs, facilitating high acceptability in the food system (Azam-Ali, 2021; Enriquez and Archila-Godinez, 2022). The ability of most Vigna taxa to fix soil nitrogen owing to their symbiosis with rhizobia (Foyer et al., 2016), makes them attractive candidates for soil improvement and low-input agricultural systems. To this effect, they can also contribute to reduced global greenhouse gas emissions associated with synthetic nitrogen fertiliser production, and nitrous oxide emission from the soil (Jensen et al., 2012). It also provides an opportunity for the cultivated Vigna taxa to be used in cereal-legume intercropping and crop rotation, thereby improving the production of other crops. Some wild Vigna are also resistant to legume pests. For instance, V. vexillata is resistant to bruchids (Callosobruchus spp.), which are serious storage-insect pests for legumes (Amkul et al., 2019). Further, the genus has potential use in pest smothering as trap crops. For example, V. radiata is used for managing Apolygus lucorum in cotton (Lu et al., 2009) and V. unguiculata as a companion crop to manage Striga spp. in maize and sorghum (Khan et al., 2007).
Furthermore, some Vigna species such as mung bean (V. radiata) and Bambara ground nut (V. subterranea) are promising alternatives for egg-protein due to their high albumin and globulin content (Yang et al., 2022). Moreover, the genus is known for its antioxidative properties which have several health benefits (Jayathilake et al., 2018; Ramatsetse et al., 2023). For example, antidiabetic effects in V. comosa (Lucrèce et al., 2021) and V. nakashimae (Kim et al., 2018), and anti-obese effects in V. aconitifolia (Bhadkaria et al., 2022). Further exploration of these properties would make the genus an invaluable source of functional foods for managing non-communicable chronic ailments such as diabetes and obesity, which have become global public health concerns (Willett et al., 2019).
Put together, this demonstrates that the genus Vigna is an important taxon with high potential to contribute not only to global food and nutrition security but also human and environmental health. Given the global shift towards alternative protein sources that have low carbon footprints (Willett et al., 2019), plant-based proteins, and legumes in particular, are placed high on the global agenda in this regard (Foyer et al., 2016; Jensen et al., 2012). Legume protein is of global interest owing to its low cost, high nutritional value and high bioavailability (Feng et al., 2023). Therefore, demand for nutrient-dense legumes like Vigna taxa is anticipated to increase in the near future, underscoring the need for their effective conservation and exploration for their valuable traits.
The CBD (2005) defines ex situ conservation as “the conservation of components of biological diversity outside their natural habitats.” Engels et al. (2008) have described several ex situ conservation techniques including seed bank, field bank, in vitro storage, cryopreservation, pollen storage, and DNA storage. Reports (e.g., Engels and Ebert, 2021a, 2021b; Khoury et al., 2022) indicate that genetic erosion in CWRs, largely stemming from the “Green Revolution,” was realised in the late 1960s as a global threat to food security. Since then, their conservation has traditionally been by ex situ approaches through a global network of gene banks (national and international) maintained by plant breeders mostly working at the Consultative Group on International Agricultural Research (CGIAR) centres under the coordination of the Food and Agricultural Organisation (FAO). The centres and their associated gene banks were established for the conservation of PGR in the 1920s (Engels and Ebert, 2021a). Presently, most gene banks holding CWRs receive financial and technical support from FAO. A historical perspective and chronology of events regarding ex situ conservation of CWRs is well documented (Engels and Ebert, 2021a), including the system’s strengths and weaknesses (Engels and Ebert, 2021b).
Ex situ conservation of PGR has been criticised for many limitations, chief among them, is the freezing of the evolutionary process of germplasm (Bellon et al., 2017; Kasso and Balakrishnan, 2013; Maxted et al., 2008a). It has been argued that freezing the evolutionary process of germplasm may make it less adaptable to the unique future climate envelopes (Bellon et al., 2017; Moray et al., 2014). Besides, uncertainty still lingers over the long-term seed viability in ex situ collections (Solberg et al., 2020) and the effects of regeneration activities on the morphological and phenological traits of wild populations under ex situ conservation (Solberg et al., 2017). Nonetheless, ex situ conservation has several advantages. Several thousands of CWR taxa can be stored in a relatively small space under ex situ conservation. It also ensures germplasm is protected from natural disasters in the wild, and is therefore an insurance policy against extinction (Li and Pritchard, 2009) (but c.f. Herbold and Engels, 2023). Besides, it promotes access to, and availability of, germplasm for use by both breeders and local communities (Engels and Thormann, 2020). Additionally, ex situ conservation can become a source of material for in situ population enhancement (Engels et al., 2008).
According to the CBD (2005), in situ conservation is the conservation of ecosystems and natural habitats and the maintenance and recovery of viable populations of species in their natural surroundings and, in the case of domesticated or cultivated species, in the surroundings where they have developed their distinctive properties. In situ CWR conservation can be done in field margins, national parks, and biosphere reserves, or natural environments outside these establishments. Although the use of CWRs for crop improvement began as early as 1940s, in situ conservation of CWRs was only suggested in the 1970s due to accelerating threats of species extinctions (Meilleur and Hodgkin, 2004). However, compared with the relatively more developed ex situ conservation, the potential development of CWR in situ conservation has been negatively impacted by policy management conflicts between agriculture and the environment (Maxted and Magos Brehm, 2023). As such, it was only until the early 90s after the involvement of naturalists and conservationists in CWR conservation that the focus shifted more towards in situ conservation.
It is widely agreed that in situ conservation of CWRs is a dynamic approach, as it provides for a continued evolutionary process with abiotic and biotic factors, making the germplasm best suited for meeting future unique environments imposed by climate change (Bellon et al., 2017; Maxted et al., 2012; Maxted and Kell, 2009). Existing protected areas (PAs) have been suggested for systematic in situ conservation of CWRs (Maxted et al., 2012, 2016; Maxted and Kell, 2009; Stolton et al., 2006). Several factors support this reasoning (1) that PAs already have some minimum protection and, therefore, less management effort and resources would be required; (2) that it would be almost impractical to establish PAs solely for CWRs since PAs are usually established based on charismatic species or specific habitats (Maxted et al., 2016; Maxted et al., 2008b); and (3) that the creation of new PAs may have huge cost implications while also exacerbating the existing human-conservation conflicts (Jensen and Pliscoff, 2023; Maxted et al., 2016). Therefore, genetic reserves have been proposed for effective CWR conservation in PAs (Maxted et al., 2008b). According to Maxted et al. (2008a), genetic reserves are meant to avoid passive conservation of CWRs in PAs and ensure that viable populations are conserved, managed, and monitored. Guidelines for establishing genetic reserves (Dulloo et al., 2008; Iriondo et al., 2021) and management plans (FAO, 2018; Maxted et al., 2008b; Iriondo et al., 2021) have since been developed.
To promote and facilitate the use of PGR through these networks for the benefit of all society, Maxted and Magos Brehm (2023) have proposed establishing a governance structure at national, regional, and global levels, somewhat mirroring the ex situ system. We contend that linking genetic reserves to community seed banks would provide extra strength to CWR conservation at the local level in this regard. Given the large volumes of CWR taxa to be conserved (Engels and Thormann, 2020; Maxted et al., 2010), against limited conservation resources along with socio-cultural and political complexes (Walls, 2018), countries are encouraged to prioritise target CWR taxa for sound conservation planning. Guidelines to facilitate prioritisation are available (Kell et al., 2017; Magos Brehm et al., 2008; Maxted et al., 2007), and various tools to facilitate conservation planning have been developed (Magos Brehm et al., 2019). Further, descriptors to provide standards for CWR germplasm documentation that would facilitate information sharing have been developed (Alercia et al., 2021).
Remarkable efforts to support CWR in situ conservation of priority taxa have been reported. For example, the genetic reserve networks in the European Union (Álvarez Muñiz et al., 2020; Rubio Teso et al., 2020), SADC region (Álvarez Muñiz et al., 2020; Dulloo et al., 2021; Magos Brehm et al., 2022), and West Africa (Nduche et al., 2022). For Vigna CWRs, key priority conservation countries in West Africa include Benin, Cote d’Ivoire, and Nigeria (Nduche et al., 2022). On the other hand, 13 priority taxa including V. keraudrenii, V. schlechteri Harms, and V. unguiculata subsp. burundiensis Pasquet have been identified for in situ conservation action in the SADC regional network (Allen et al., 2019). The highest priority areas are in Angola, Botswana, Malawi, Namibia, Tanzania, and South Africa (Magos Brehm et al., 2022). Some formal in situ conservation actions have been initiated, for example, in the Nyika National Park in Malawi (Dulloo et al., 2021).
However, the effectiveness of in situ conservation of CWRs has been questioned in light of climate change effects. For instance, Jarvis et al. (2008) have predicted that 16–22% of CWRs, including 51 Vigna CWRs risk extinction globally by the year 2055 and most species may lose over 50% of their climatically suitable habitats. In southern Africa, 64% of the 113 regional priority CWR taxa, including Vigna taxa, are predicted to be negatively impacted by climate change (Magos Brehm et al., 2022). Growing concerns have thus been raised about whether PAs would effectively offer protection to CWRs under the changing climate. This is particularly so because PAs are static entities while species are shifting, and many existing PAs were established before climate change came into the picture (Heywood, 2019; Thomas and Gillingham, 2015). Indeed, PAs are becoming climatically unsuitable habitats for CWR taxa as seen in Ethiopia (Davis et al., 2019), Mexico (Lira et al., 2009), Netherlands (Aguirre-Gutiérrez et al., 2017), and Sri Lanka (Ratnayake et al., 2021).
To investigate the potential location of a global network of genetic reserves under the changing climate, Vincent et al. (2019) used 1,261 CWR taxa, including those of the genus Vigna, implemented in the MaxEnt algorithm under the Representative Concentration Pathway 4.5 (RCP 4.5). One hundred-fifty sites have been identified that could potentially conserve nearly 65.7% of CWR species. However, considering that severe climate change impacts are forecast in many areas where Vigna taxa are currently distributed, such as Africa and Asia (IPCC, 2021), the use of RCP 4.5 may not reflect the climate change dynamics in these regions. Therefore, to meaningfully contribute to the global network of genetic reserves using the PA network, it would be important to assess the impacts of climate change on the effectiveness of the existing PA network at national levels where implementation would take place. This is also particularly pertinent given that using PA alone may not be the only best option to conserve CWRs under the changing climate (Goettsch et al., 2021; Iriondo et al., 2021; Manda et al., 2022). For example, Manda et al. (2022) have shown that only 10% of the existing PA network in Benin may potentially cover climatically suitable habitats of the studied 15 Vigna CWR taxa, and about half of the studied taxa may lose 5–40% of their suitable habitats by 2055.
The use of PAs has also been questioned if it could effectively conserve CWR taxa, considering that they were largely established for charismatic or flagship species (Maxted et al., 2008b). To address this concern, Moray et al. (2014) explored the possibility of conserving 13 prioritised African Vigna CWR species in Africa’s PA network, and concluded that the African Vigna CWR taxa could potentially be conserved in the existing PAs. However, the use of PAs as advanced by Moray et al. (2014) without taking into consideration the effects of climate change as argued herein above would render ineffective in situ conservation of Vigna taxa.
Limited information regarding the biology, threat status, ecology and taxonomy of many CWR taxa is another factor undermining successful in situ conservation of CWRs (Engels and Thormann, 2020; Khoury et al., 2020b). These are important preconditions to achieve effective in situ conservation, as they are crucial for designing management strategies for taxa and monitoring to ensure efficacy of conservation interventions. Monitoring of CWR populations, according to Engels and Thormann (2020), may include assessing the population genetic structure, demographic trajectory and habitat quality such as the floristic composition of target taxa with reference to previously established baseline information. Here, the use of indirect measures such as change in herbaceous vegetation relative to other vegetation types to reflect changes in habitat quality may be used to provide populations trends for small, data-poor and herbaceous species such as Vigna CWRs (Iriondo et al., 2008).
Considering that a substantial amount of CWR taxa are found outside the existing formal PAs (e.g., Al-Atawneh et al., 2007; Fagandini Ruiz et al., 2021; Jarvis et al., 2015; Maxted et al., 2004; Salako et al., 2014), and that PAs alone may not actively conserve CWR taxa in a changing climate, calls have been growing for extra-PA site based in situ conservation of CWR taxa. Consequently, variants of these conservation approaches have been suggested. For instance, trans situ (Riordan and Nabhan, 2019) and “other effective area-based conservation measures” (OECMs) (IUCN, 2019). Apart from ensuring the conservation of a wider CWR diversity, extra-PA site based in situ conservation approaches are likely to promote the active participation of local communities in CWR conservation, which is also crucial for local support, sustainability, and ownership for in situ conservation initiatives (Maxted and Magos Brehm, 2023). They may also allow for the continuation of long-standing indigenous peoples’ or local community practices that have shaped the diversity of some CWR taxa we see today. Some examples include the fire-stick farming by the Aborigines in Australia that has maintained V. lanceolata (Castelli and Mikić, 2019) and the foraging behavior of the Hadza hunter-gatherers in Tanzania whose food security is also dependent on tuberous plants like V. frutescens (Safari et al., 2021). To address the inadequacies of in situ conservation focusing on the use of PAs alone and ex situ conservation, Iriondo et al. (2021) have proposed an integration of multiple in situ and ex situ conservation approaches taking into account OECMs.
Local communities and their indigenous knowledge systems, including local ecological knowledge (LEK), are considered crucial for the success of in situ conservation of wild resources such as CWRs. The CDB (Article 8j), for instance, places particular emphasis on this aspect (CBD, 2005). Although few ethnobotanical studies explicitly report on CWRs (e.g., Pawera et al., 2017; Shigaeva et al., 2019), numerous studies show the critical role played by LEK in biodiversity conservation, including threatened and data-poor taxa (e.g., Castagnino et al., 2023; Kanagavel et al., 2020; Leduc et al., 2021). The significant role of local communities in biodiversity conservation also stems from the recognition of their vital role played in shaping species diversity. For example, domestication and selection of wild species were initiated by cultures of various local communities (e.g., Diamond, 2002; Ross-Ibarra et al., 2007), and studies (e.g., Casas et al., 2007) have shown how local communities are changing the evolutionary process of wild species, including CWRs. As such, it is undisputable that excluding local communities in natural resources management would render ineffective in siu conservation efforts (Manda et al., 2023; Maxted et al., 2008a; Padulosi et al., 2011).
Specifically for the conservation of CWRs, local communities would be key in the location and management of genetic reserves. Their knowledge associated with targeted CWR taxa would be valuable in CWR conservation and use. This is especially true considering that most CWR taxa are exploited by local communities for multiple uses. Indeed, owing to the long-time interaction with nature, local communities know the distribution of particular kinds of resources in the wild, and are usually aware of species population dynamics (Kanagavel et al., 2020; Tomasini and Theilade, 2019). Besides, most OECMs popularised for global CWR conservation (Iriondo et al., 2021) are in the custody of local communities.
Moreover, given that populations of a significant number of CWR taxa are cultivated, weedy or found in hedges and margins of crop fields (e.g., Catarino et al., 2021; Jarvis et al., 2015; Maxted et al., 2004), local communities would also be key in the conservation of such CWR taxa. To this end, trade-offs in form of monetary incentives for local communities or land owners to conserve CWRs have been calculated (Drucker et al., 2023; Wainwright et al., 2019). For instance, Drucker et al. (2023) have shown that farmers in Malawi are willing to conserve about 22 CWR species across 17 related crops for an estimated MWK 20,000 (≈US$ 25) per annum. While these incentives may help motivate local communities actively participate in CWR conservation, practicalities of such an approach are yet to be known. Its long-term sustainability is also questionable, especially in low-income countries (Leimona et al., 2015). Instead, consideration should probably be on non-monetary incentives. For example, ensuring that local communities see the need for sustaining important ecosystem services they obtain from CWRs could be explored as an option in this respect, as demonstrated in agroforestry systems (Leakey et al., 2022), and wildlife management (Allendorf and Yang, 2013; Manda et al., 2023).
With the current crops increasingly being challenged by various climate-induced abiotic and biotic stresses, there is an urgency for a sustainable and resilient agricultural production system to increase the quantity and quality of the food basket. One option is the cultivation and/or domestication of populations of some CWR taxa. Moreover, most populations of Vigna CWR taxa are already under cultivation or incipient management. The promotion of their cultivation and domestication can, therefore, provide a profile of new crops that could suit novel climate envelopes (Zhang et al., 2018). Indeed, domestication of new crops will promote agrobiodiversity and could be a solution to many challenges associated with intensive and homogenous agriculture (Østerberg et al., 2017). It could also contribute to market diversification (Watteyn et al., 2023), and culture-based economies, as is the case with wild rice (Abdelghany et al., 2021). According to von Wettberg et al. (2020), domestication of populations of CWR taxa may not be difficult, since their template is already available. However, for meaningful cultivation and domestication, elite taxa with preferred agronomic traits and cultivation protocols are needed (Abdelghany et al., 2021; Watteyn et al., 2023). To contribute to this field of development, agro-morphological studies have been conducted in the genus Vigna (e.g., Bisht et al., 2005; Harouna et al., 2020; Ogunkanmi et al., 2019; Popoola et al., 2015; Pratap et al., 2018).
Bisht et al. (2005) assessed the morphological diversity of 206 accessions of 14 wild Vigna taxa from India. The study has demonstrated a higher within-species variation in the wild Vigna including V. trilobata. This is especially related to flower, pod, and seed characteristics, and resistance to abiotic and biotic stresses (Bisht et al., 2005). In Nigeria, Popoola et al. (2015) used 31 morphological characters (quantitative and qualitative, vegetative and reproductive traits) to evaluate the intra-and inter-specific morphological variabilities among 20 accessions of six Vigna species comprising wild and cultivated species. The authors have reported higher genetic diversity among wild species including V. luteola, V. oblongifolia, V. racemosa, and V. vexillata, particularly related to reproductive traits such as days to 50% flowering, days to 50% ripe pod, the number of pods per peduncle and 100-seed weight. Harnessing the utilization of this diversity for food and nutrition, fodder for ruminant animals, and as cover crops for rotational farming has been recommended (Popoola et al., 2015).
Pratap et al. (2018) sought to identify wild Vigna with promising genotypes for multiple agronomic and adaptive traits based on morphological descriptors in India. They used 44 accessions including wild and domesticated belonging to 12 species. As expected, the accessions have demonstrated variations at the species level. Accessions such as wild V. radiata (IC 251424 & IC 251425) and V. trilobata (IC 331436) have been identified for multiple traits such as the number of seeds/pod, seed quality and early maturation. Likewise, three accessions of V. trilobata (IC 331545, IC 349701 and JAP/10–7), one wild V. radiata (IC 251427), and wild V. mungo (IC 251335) have also been identified for earliness. The identified accessions could have potential use in improving the Asian Vigna, especially mungbean and urdbean.
Similarly, Ogunkanmi et al. (2019) carried out a morphological evaluation of 390 accessions comprising 130 wild cowpea and 260 cultivated accessions in Nigeria using 27 cowpea descriptors including pigmentation and pubescence. The study has revealed about 29% presence of pigmentation on stems, 21% presence of stripes on pods, and 20% presence of pubescence in wild Vigna compared with 12, 1.53, and 0% in the cultivated taxa, respectively. Accessions V. trilobata (TVNu 953), V. grandiflora (TVNu 539) and V. vexillata have particularly been selected for their dense pubescence which may offer resistance to insect pests (Ogunkanmi et al., 2019).
A higher pubescence density on plant parts, such as leaves and stems as found in wild relatives by Ogunkanmi et al. (2019), is probably associated with higher water-use efficiency, which is an adaptative trait for arid conditions (Boukar et al., 2020; Konrad et al., 2015; Maxted et al., 2004). It may also serve as a barrier to predators, parasites and pathogens (Boukar et al., 2020). For instance, high pubescence on pods of V. vexillata reduced damage from pod borer, Maruca vitrata Fab and pod sucking bug, Clavigralla tomentosicollis Stål (Boukar et al., 2020). Whereas, a high density of leaf hairs reduced infestation of the tarnished plant bug, Lygus lineolaris (Palisot de Beauvois) in cotton (Wood et al., 2017), and different types of hairs were found to confer resistance to cold, drought, and insects in tomato (Solanum lycopersicum) (Zhang et al., 2020). On the other hand, colouration of seeds, which usually correlates with pod colour (Chamoli et al., 2021), is associated with higher nutritional and health compounds (Francavilla and Joye, 2020), and resistance to abiotic and biotic stresses (Al-Khayri et al., 2023; Kopecká et al., 2023). Seed coat colour may also relate to seed nutrient composition, and seed vigour and germination qualities (Atis et al., 2011; Mandizvo and Odindo, 2019). For instance, Mandizvo and Odindo (2019) noted good crop stand establishment and a higher mineral element in dark-coloured compared with light-coloured seeds of Bambara ground nut.
In Tanzania, an agro-morphological characteristic exploration of 160 accessions of wild Vigna (Harouna et al., 2018) has shown that V. racemosa, V. reticulata, and V. vexillata have important agro-morphological traits comparable with domesticated Vigna legumes such as cowpea that have attracted farmers’ preferences. The involvement of farmers in selecting preferable traits as done by Harouna et al. (2018) is particularly crucial for adoption of PGR.
Collectively, the findings from these five case studies provide a stepping stone for active cultivation and/or domestication of wild Vigna populations, given that a lack of agronomic information has partly slowed down the cultivation of native wild plants including CWRs (George et al., 2023). They also demonstrate the existence of high genetic diversity in Vigna CWR taxa that could further be explored for potential utilization in Vigna breeding programmes for improved quantity and quality, and resistance to abiotic and biotic stresses. Some materials chosen for drought tolerance could also become potential rootstocks for the drought-susceptible Vigna domesticates (Maxted et al., 2004; Tejada-Alvarado et al., 2023). Tirnaz et al. (2022), on the other hand, advise that care ought to be taken when incorporating CWRs into traditional farming systems since this may lead to unfavourable consequences, such as the emergence of new pests as evidenced when a maize wild relative (Zea diploperennis Iltis, Doebley & R. Guzmán) was introduced into the Mexican and Argentinian farms.
Considering that conventional domestication is a long process, and that it is usually challenging to have crops with resistance to abiotic and biotic stress that simultaneously have higher nutritional quality, de novo domestication – a gene editing technology - has been suggested as an alternative route (Gasparini et al., 2021). It is believed that the approach can accelerate CWR domestication to create new crops and circumvent some of the challenges encountered with conventional domestication, such as loss of genetic diversity due to domestication drag (Fernie and Yan, 2019; Gasparini et al., 2021). Recent work on Vigna stipulacea Kuntze (Takahashi et al., 2019) provides evidence for potential use of this technology in the genus Vigna. The technology has also been successfully applied in Solanum pimpinellifolium L., a wild relative of tomato (Zsögön et al., 2017). While this technology might accelerate the creation of new crops for the future, Bartlett et al. (2023) posit that its success will, in part, require collaboration across traditional boundaries, suggesting participatory breeding with local communities in the targeted areas for acceptability of new crops.
Drought relates to soil moisture deficit, impacting crop ecology and behaviour (Beebe et al., 2013; Trenberth et al., 2014). It can be described as early, intermittent, and terminal drought, with the later having the most detrimental consequences for agricultural production (Farooq et al., 2017; Labastida et al., 2023). Drought is the most important abiotic stress factor limiting global agricultural productivity, especially in arid- and semi-arid areas (Basu et al., 2016; Nadeem et al., 2019). Many studies spanning different plant taxa, such as cereals (e.g., Sallam et al., 2019), tubers and vegetables (Flores-Saavedra et al., 2023), and legumes (Farooq et al., 2017; Nadeem et al., 2019; Ullah and Farooq, 2021), have shown the disastrous effects of drought stress. The effects include reduced germination rate, stunted plant growth, a reduction in water and nutrient uptake, damage to the photosynthetic apparatus, reduction in flowering, and decrease in the seed number, seed weight, and seed composition, ultimately resulting in reduced quantity and quality of yield for the cultivated species (Farooq et al., 2017; Nadeem et al., 2019; Ullah and Farooq, 2021). Drought stress can also increase the vulnerability of plants to several biotic stresses, such as phytophagous insects (Showler, 2013) and pathogens (van Munster, 2020). Climate change is poised to exacerbate these effects, given the predictions for increased frequency, severity and duration of drought (Dai, 2013; Trenberth et al., 2014), with resultant major implications for global food security, economy and trade (Guadarrama-Escobar et al., 2024; Rao et al., 2013).
Drought effects on plants may vary depending on three main factors: plant growth stage (Farooq et al., 2017); intensity, rate, and duration of the stress (Dong et al., 2019), and (3) plant genotype (Farooq et al., 2017; Ullah and Farooq, 2021). Although all plant developmental stages can be affected by drought stress, the reproductive stage (including flowering and grain filling stages) is the most critical stage for grain-producing species like Vigna (Farooq et al., 2017; Labastida et al., 2023; Nadeem et al., 2019; Oguz et al., 2022; Senapati et al., 2019). At this stage, drought stress can cause irreversible impairment to embryo mitotic processes, thereby affecting flowering, grain set and grain development, seed and pod number, and other yield attributes, ultimately, negatively impacting the quality and quantity of the final yield (Beshir et al., 2016; Fang et al., 2010; Farooq et al., 2017; Nadeem et al., 2019; Oguz et al., 2022). Others suggest that drought stress imposed at the germination stage can also impose a critical limitation to plant growth and development, which may lead to reduction in crop stand establishment (Carvalho et al., 2019; Ullah and Farooq, 2021). Collectively, this underscores the importance of testing genotypes at different plant growth stages and with different stress intensities and durations. Indeed, some taxa that may be drought tolerant at the vegetative stage may be sensitive at the flowering stage or vice versa (Sallam et al., 2019). Key to screening for drought stress is also the identification of traits that could clearly discriminate tolerant and susceptible genotypes (Sallam et al., 2019).
In response to drought stress conditions, plants have evolved several drought resistance strategies at the biochemical, morphological, and physiological levels. Drought resistance is a broad term applied to plant species with adaptive traits that enable them to escape, avoid or tolerate drought stress (Basu et al., 2016; Beebe et al., 2013; Seleiman et al., 2021). Drought escape is the ability of a plant species to modulate its life cycle according to soil water availability, and is characterised by earliness and developmental plasticity (Basu et al., 2016; Beebe et al., 2013). Drought avoidance is the ability of a plant to maintain a relatively higher tissue water content despite reduced water content in the soil (Basu et al., 2016). It may be achieved through adaptable traits involving either minimization of water loss, i.e., effective water use under drought stress (water savers), such as reduced evapotranspiration under drought stress (Agbicodo et al., 2009; Basu et al., 2016) or optimization of water uptake under drought stress (water spenders), such as deep rooting (Basu et al., 2016). To avoid drought stress, many legume species, including Vigna taxa, may go into “hibernation,” and recuperate when the stress is over, growing new organs in the process (Agbicodo et al., 2009; Nadeem et al., 2019). Some also tend to have delayed leaf senescence, a phenomenon called stay-green (Agbicodo et al., 2009; Muchero et al., 2013). Such drought avoidance mechanisms have compelled others (e.g., Fang and Xiong, 2015; Tadele, 2019) to treat recovery as a different drought resistance strategy. Lastly, drought tolerance is the plant’s ability to endure low tissue water content (Basu et al., 2016). It may be achieved through various adaptable traits, such as maintenance of cell turgor, e.g., by osmotic adjustment and increase in cell elasticity, and desiccation tolerance by increasing protoplasmic resistance (Basu et al., 2016; Beebe et al., 2013). Although the three drought stress strategies may seem distinct, plant adaptation to drought stress reflects a balance among escape, avoidance, and tolerance while maintaining adequate productivity (Agbicodo et al., 2009; Nadeem et al., 2019). Indeed, as with most plants, Vigna taxa are known to switch from one strategy and/or mechanism to another (Agbicodo et al., 2009), underlining the importance of varying the timing of drought stress in exploring drought resistance traits.
Several cultural and management practices are used to alleviate the adverse effects of drought stress. These include early planting, increasing plant density, crop rotation, the use of drought-resistant varieties, and soil and water conservation practices, such as mulching (Ogundeji and Okolie, 2022; Seleiman et al., 2021). Increasing effort has also been made on the application of exogenous regulators (phytohormones), chemicals as in seed priming, synthetic hormones and compounds, and soil microbes such as rhizobacteria (Khatun et al., 2021; Seleiman et al., 2021). Despite these measures, drought stress remains one of the major production constraints for Vigna varieties and landraces (e.g., Nkomo et al., 2021; van Zonneveld et al., 2020). While agrochemicals may be effective, but they are costly for resource-constrained farmers in drought-prone areas. Concern is also growing over their increased use for their hidden environmental and human health costs (Devi et al., 2022). Therefore, sustainable solutions are required to meet the global food demand in a changing climate, and the development of host-plant resistance to drought stress is considered an alternative (e.g., Porch et al., 2013; Zaki and Radwan, 2022). Host-plant resistance is considered cost-effective and easy to adopt by resource-poor smallholder farmers, disproportionately affected by climate-induced drought stress factors (Vermeulen et al., 2012). This provides a basis for the urgency in the exploration for germplasm that could yield under drought stress for use by farmers and plant breeders, and CWRs are regarded as a valuable resource in this regard.
Unfortunately, rarely have studies been conducted to explore drought resistance traits in Vigna CWRs. Chief among the reasons is the belief amongst breeders that Vigna landraces might be harbouring drought resistance traits which have not been fully explored (Takahashi and Tomooka, 2020). Limited expertise in this field (Agbicodo et al., 2009), low investment in the Vigna crop and linkage drag of undesired traits associated with CWRs (Dempewolf et al., 2017; van Zonneveld et al., 2020), the complexity of drought resistance (Gorim and Vandenberg, 2017; Nadeem et al., 2019), and practical challenges related to the long growth cycles and seed traits (Cortés et al., 2013) have all been implicated in the slow progress of exploring sources of resistance in wild relatives. Consequently, to our knowledge, only one notable study (Iseki et al., 2018) was reported in the literature to evaluate the adaptive response to drought stress of Vigna CWR taxa at the time of this review.
Iseki et al. (2018) used 69 accessions (54 wild and 15 domesticated) of Vigna to screen for drought tolerance during the vegetative stage. Plants were planted in polyvinyl chloride (PVC) pipes and pots representing non-terminal and terminal drought stress, respectively. The evaluation traits were relative shoot weight (RSW) and number of days to wilting (DTW) for the pipe and pot experiments, respectively. Nineteen wild accessions have been identified as target drought tolerant accessions, with their tolerance related to higher plant water status attributable to water saving abilities of the species. The accessions include Vigna sp. N11135 (ID28), V. trilobata (ID47), V. vexillata var. ovata (ID55), V. radiata var. sublobata (ID27), V. vexillata var. lobatifolia (ID59), and V. unguiculata subsp. dekindtiana (ID64) (Iseki et al., 2018). According to Iseki et al. (2018), the high-water status traits demonstrated by these accessions may be related to their origin of dry, sandy habitats, a suggestion corroborated by van Zonneveld et al. (2020). Based on the high-water status observed by Iseki et al. (2018), the taxa could be described as having a drought avoidance strategy. Indeed, like many other herbaceous species (Kooyers, 2015), many Vigna species appear to employ escape or avoidance strategies for drought resistance (Agbicodo et al., 2009; van Zonneveld et al., 2020).
Interestingly, accessions that were found tolerant in the non-terminal drought experiment were different from those in the terminal drought evaluation, suggesting the taxa employ different mechanisms for adaptation to each of the non-terminal and terminal drought conditions (Iseki et al., 2018). Use of multiple drought-resistant strategies or switching from one strategy to another is not uncommon among plants (Agbicodo et al., 2009; Gorim and Vandenberg, 2017; Kooyers, 2015). For example, Gorim and Vandenberg (2017) observed the use of more than one strategy within the same genotype of wild lentils in Canada.
Iseki et al. (2018) further attempted to determine mechanisms for adaptation in the tested accessions. They have thus classified the tested accessions into three groups (1) those with low RSW and long DTW, (2) high RSW and short DTW, and (3) high RSW and long DTW. Accessions with low RSW and long DTW include mostly domesticated species such as V. unguiculata (ID62), V. aconitifolia (ID39) and V. subterranea (ID69). The authors have regarded these as drought susceptible based on the low RSW, but have suggested the plants might be adapted to areas experiencing frequently severe drought stress based on their long DTW and ability to rapidly close their stomata (Iseki et al., 2018). Early stomata closure and long DTW are some of the important drought avoidance traits in Vigna species (Agbicodo et al., 2009; Muchero et al., 2013).
Accessions with high RSW and short DTW include Vigna sp. N11135 (ID28), V. minima (ID19) and V. radiata var. sublobata (ID27). The high RSW helps in water uptake under mild drought stress. However, the short DTW implies the accessions are “water spenders” and cannot survive prolonged severe drought stress. Nonetheless, these traits according to Iseki et al. (2018) might be advantageous in areas with intermittent mild drought stress. Lastly, accessions with high RSW and long DTW include V. trilobata (ID47), V. vexillata var. ovata (ID55), V. vexillata var. lobatifolia (ID59) and wild V. aconitifolia (ID40-42). Although Iseki et al. (2018) have not identified tolerant mechanisms for these accessions, tuberous rooting and high pubescence, for instance, may explain the observed high RSW and long DTW in V. vexillata accessions, respectively (Maxted et al., 2004).
Iseki et al. (2018) have unravelled important adaptive traits for drought stress and have provided a stepping stone for drought tolerance studies in wild Vigna. However, it would be interesting to see how the identified drought-stress tolerant accessions may respond to drought stress in the reproductive stage, since some of these may be sensitive at the flowering stage which is mostly a yield-deciding stage (Farooq et al., 2017; Saha et al., 2022). Moreover, concern is growing over the underutilization of germplasm identified for drought tolerance alone, with less attention on the potential yield gain under drought stress (Nature Editorial, 2024), suggesting adaptive response experiments on drought stress with a focus on yield gain.
Given the rarity of studies on the adaptive response to drought stress of Vigna CWR taxa, we have selected some agro-morphological studies (Table 2) that could help facilitate pre-breeding for drought tolerance in Vigna. Despite the shortcomings of agronomic studies, such as slowness, agro-morphological traits are still the primary focus of plant breeders and researchers (Rahimi et al., 2016; Rao et al., 2013; Reyes et al., 2022; Rubio Teso et al., 2022), such that hallmark drought stress explorations have often used realistic soil conditions and different watering regimes (Rao et al., 2013). According to Rahimi et al. (2016), plant breeders use morphological characters such as the plant height, pod number, length and width of leaf, days to flowering, days to maturity and seed yield as selection criteria for drought stress tolerance. These traits have been widely used in screening for drought tolerance in legumes including Vigna.
Table 2. Selected studies for potential use in screening for adaptive response to drought stress in Vigna CWRs.
Striga species (family Orobanchaceae), commonly called witchweeds, are among the estimated 4,500 flowering plants (representing 1% of angiosperms) that parasitize other plants (Těšitel, 2016). Weeds are responsible for about 34% reduction in crop yield globally (Scott and Freckleton, 2022), and over US$100 billion annual loss globally (Swanton et al., 2015). Striga spp. alone affect over 40 countries annually, especially in Africa and Asia, with 25 countries in SSA (Dafaallah, 2019), where they infest over 100 million ha of land, threatening food security and livelihoods for over 300 million resource-constrained farmers (Jamil et al., 2021; Makaza et al., 2023), and causing huge yield losses valued between US$ 7 to 10 billion annually (Jamil et al., 2021). Yield losses usually range between 20 and 80% (Spallek et al., 2013), but total crop failure has also been reported in susceptible cultivars (Atera and Itoh, 2011; Sawadogo et al., 2021a; Teka, 2014), often resulting in crop field abandonment (Atera and Itoh, 2011; Vissoh et al., 2004). The severity of Striga may be influenced by soil nitrogen availability, host genotype, Striga species and biotype, and infection time and level (Alonge et al., 2005a; Cissoko et al., 2011; Hayatu et al., 2016; Mandumbu et al., 2019). For instance, Alonge et al. (2005a) report serious Striga damage in late-planted compared with early-planted susceptible cowpea varieties. In contrast, varying Striga infestation levels produced differential responses in cowpea varieties (Hayatu et al., 2016).
Striga spp. are root, obligate hemi-parasitic plants. Their seed germination relies on exogenous signals from host-plant roots, called strigolactones (Zwanenburg et al., 2016). Their survival depends on forming vascular connections with their host by haustoria, specialised organs through which nutrients and water are withdrawn from hosts (Yoshida et al., 2016; Zwanenburg et al., 2016). The extraction of nutrients and water causes biochemical and physiological changes in the host plant often manifesting through reduced photosynthesis, increased root and shoot ratio, stunting, chlorosis, wilting, premature defoliation, reduced dry biomass, and reduced grain yield (Atera and Itoh, 2011; Leandre et al., 2018; Parker, 2012; Spallek et al., 2013), and reduced nutrient content (Alonge et al., 2005b). Striga also induces an increased dry weight partitioning to the host root system at the expense of the shoot system which further manifests through an increased root and shoot ratio (Kroschel, 2001). Striga spp. can also be a source of various pathogens to their host plants (Gogoi et al., 2022), which could further worsen the health of host plants. Low-input farming systems, coupled with poor soils, continuous monocropping, and limited crop rotation, have been widely implicated in the Striga spread and infestation (Ejeta, 2007; Mandumbu et al., 2019; Parker, 2014; Scott and Freckleton, 2022). The Striga scourge mostly hits resource-constrained smallholder farmers in developing countries as they usually lack knowledge or resources for effective Striga control or management (Mandumbu et al., 2019; Mwangangi et al., 2021; Scholes and Press, 2008; Tippe et al., 2017).
For long, four main components of Striga resistance, namely, escape, avoidance, tolerance, and necrosis have been reported in the literature (Rubiales, 2003; Rubiales et al., 2006). Given that there is usually a delay of four to seven weeks between the time when the host plant germinates and when the Striga attaches depending on the plant species (Mandumbu et al., 2019), host plants may escape Striga attack through reduced phenology, such as earliness, as demonstrated in sorghum (Kansaye et al., 2022) and in maize (Oswald and Ransom, 2004; Fernández-Aparicio et al., 2012). Avoidance may be achieved by developing root architecture that avoids soil layers in which Striga seeds are more common or by reducing the root biomass (Rubiales et al., 2006), as observed in a maize inbred line derived from wild maize (Zea diploperennis) (Amusan et al., 2008).
Tolerance, where a plant limits damage caused by the parasite, may be achieved by factors that influence source-sink relationships, such as osmotic pressure, e.g., low production of germination stimulants, as has been reported in sorghum and legumes like wild chickpea (Cicer spp.) (Rubiales, 2003; Rubiales et al., 2006). In contrast, necrosis (darkening or reddening colouration and localised cell death) is a characteristic condition where a host plant develops a dead protective layer at the point of attack acting as a mechanical barrier to haustorial penetration (Rubiales et al., 2006), arresting growth of the parasite, with resultant premature death of the parasite due to lack of nutrients. Blockage to the haustorial penetration due to incompatibility may also occur in the cortex or at the endodermis (Jhu et al., 2023). However, it is now becoming clear that necrosis is just one component of hypersensitive response (HR) mechanisms due to incompatibility (Jhu et al., 2023). It is one of the common defence mechanisms against Striga in Vigna taxa (Huang et al., 2012; Li and Timko, 2009; Omoigui et al., 2017), likewise in Striga-resistant wild sorghum (Mbuvi et al., 2017). Further, Striga resistance may now be categorised into: (1) pre-attachment resistance, involving strategies or mechanisms employed before haustorial attachment, such as low germination stimulant production; and (2) post-attachment resistance, involving strategies employed to arrest haustorial attachment to the host plant (Figure 5). In this respect, escape and avoidance are components of pre-attachment resistance, while tolerance is a component of post-attachment resistance.
Figure 5. Host resistance responses during different stages of the Striga life cycle. (A) Pre-attachment resistance response during Striga seed germination. Host plants growing in nutrient-poor soil release strigolactones, promoting beneficial arbuscular mycorrhizal fungus symbiosis. Striga seeds perceive these host strigolactones as germination stimulants. However, mutations in genes responsible for strigolactone biosynthesis or alterations in their composition significantly reduce Striga seed germination rates. For example, mutations in the LOW GERMINATION STIMULANT 1 (LGS1) gene in resistant sorghum plants alter the composition of strigolactones in root exudates, reducing their stimulatory effect on Striga germination. (B) Pre-attachment resistance response during haustorium initiation. Germinated Striga seedlings grow towards host roots and perceive haustorium induction factors (HIFs) for haustorium initiation. Resistant host plants produce toxic compounds in root exudates that inhibit the development of parasitic plant seedlings. Some resistant host plants produce lower levels of HIFs, reducing Striga haustorium formation. (C) Post-attachment resistance response during haustorium attachment. Following the detection of pathogen-associated molecular patterns (PAMPs) or damage-associated molecular patterns (DAMPs) from Striga, plants initiate pattern-triggered immunity (PTI) to obstruct haustorium attachment. However, parasitic plant effectors can suppress PTI to facilitate parasitism. Consequently, effector-triggered immunity (ETI) overcomes this suppression and triggers hypersensitive responses (HRs) to discourage parasite penetration. (D) Post-attachment resistance response during haustorium vascular connection. Plants fortify cell walls to create physical barriers that hinder the establishment of vascular connections. Cell wall enhancement-based resistance responses probably occur downstream of PTI and ETI. Examples of these barriers include accumulating substances such as lignin or callose in the cortex, impeding the progress of parasites. Moreover, the endodermis serves as a barrier by inducing lignin accumulation, effectively preventing parasitic plant penetration and vascular connection (Adapted and reprinted with permission from Jhu et al., 2023).
Between 28 and 30 Striga species have been characterised (Ohlson and Timko, 2020; Spallek et al., 2013). Of these, three species, namely, the purple witchweed [Striga hermonthica (Del.) Benth.], Asiatic witchweed [Striga asiatica (L.) Kuntze], and cowpea witchweed [Striga gesnerioides (Willd.) Vatke.] are the most economically important (Ohlson and Timko, 2020; Parker, 2012). The primary hosts for S. hermonthica and S. asiatica are cereals in the Poaceae family such as sorghum, maize, and rice. In contrast, S. gesnerioides infests broad-leaved plant species, with the main hosts being cowpeas and wild legumes, particularly in West Africa, South and South East Asia (Parker, 2014). Non-leguminous genera, such as Ipomea, Jaquemontia, Merremia, Euphorbia, and Nicotiana, are also potential hosts (Ohlson and Timko, 2020). Striga gesnerioides, the subject of this paper, is the most variable and widely distributed witchweeds that infests cowpea in Africa (Botanga and Timko, 2006; Parker, 2012). Six races (biotypes) of S. gesnerioides, SG1, SG2, SG3, SG4, SG5, and SG6, have recently been described and their geographical distribution documented (Ohlson and Timko, 2020).
The control of Striga infestation is rather challenging, largely owing to its complex life cycle. The life cycle is intricately linked to the host plant such that effective control methods selective enough to kill the Striga without damaging the host plant are difficult to apply (Fernández-Aparicio et al., 2020). Striga control is also a challenge due to its inherent life history straits. These include high fecundity, dispersal efficiency, persistent seed bank, and rapid responses to changes in agricultural practices, which allow Striga to quickly adapt to new hosts and increase aggressiveness against new resistant cultivars (Fernández-Aparicio et al., 2020; Runo and Kuria, 2018). For instance, a single Striga plant can produce 50,000–500,000 seeds annually, and owing to their small size (∼0.2 mm in length), they are easily dispersed by wind and water and can persist in the soil for over 10 years (Runo and Kuria, 2018). Nevertheless, several strategies and technologies are used by farmers and many more have been proposed to mitigate the impacts of Striga infestation. These include the use of resistant varieties; chemical control, such as use of suicidal germination and herbicides; cultural and agronomic methods, such as hand weeding, intercropping practices, legume-cereal crop rotation, deep planting, early planting; and biological control, such as the use of microbiomes (Emeghebe et al., 2004; Haruna et al., 2018; Jamil et al., 2021; Masteling et al., 2019; Parker, 2021; Samejima and Sugimoto, 2018; Van Delft et al., 2000).
However, even with these notable control measures, Striga continues to spread (Ejeta, 2007; Ohlson and Timko, 2020; Parker, 2021). Several factors have been implicated in this state of affairs. Low adoption of most technologies, largely owing to limited access to the technologies by resource-constrained farmers (Parker, 2021). The global campaign against chemical-based technologies (e.g., herbicides) due to their negative impacts on the environment and human health is slowing down their continued use (Devi et al., 2022). Further, although Striga-resistant Vigna varieties may be accessible to some farmers, the resistance in most varieties continues to break down with new races or different virulence (Omoigui et al., 2017; Samejima and Sugimoto, 2018). On the other hand, despite the increasing attention to the upcoming technologies, their feasibility for use has not been demonstrated and their success and durability have not been proven (Parker, 2021). The upcoming technologies include suicidal germination (Kountche et al., 2019), bioherbicides (Lado et al., 2018; Sawadogo et al., 2022), and the “toothpick” technique (Nzioki et al., 2016). Lastly, most available and upcoming technologies are high-input management strategies, such that they may be unsustainable for low-input farming systems where the Striga burden is mostly experienced at present (Rubiales, 2023).
Given these limitations and considering that climate change is expected to exacerbate the dispersal and distribution of Striga (David et al., 2022; Ronald et al., 2017), host-plant defence against Striga is regarded as one of the most desirable strategies, as it is easy-to-adopt, eco-friendly, cost-effective, and more sustainable for many resource-constrained farmers (Makaza et al., 2023; Mandumbu et al., 2019; Rubiales et al., 2018). Unfortunately, sources of plant-based resistance for Striga are presently limited in Vigna germplasm. According to Ohlson and Timko (2020), the multi-race resistance gene from the Botswana cowpea landrace B301 (Li and Timko, 2009) is the only best characterised and widely used, even if it appears to succumb to the SG4 from Zakpota in Benin (Huang et al., 2012; Jhu and Sinha, 2022; Su et al., 2020).
Taken together, this underlines the imperativeness for continued identification of new sources of resistance to S. gesnerioides for use in plant breeding and integrated pest management by Vigna farmers. Vigna CWRs are considered treasure troves in this respect. Yet, unlike with CWRs of cereals (e.g., maize and sorghum), studies on the adaptive response to Striga stress of Vigna CWR taxa have mostly been limited over the past decades. Similar factors, as argued for drought stress, may explain this status. Only two notable studies (Oyatomi et al., 2016; Tignegre et al., 2013) have reported the adaptive response to Striga of Vigna CWR taxa this far.
Tignegre et al. (2013) evaluated 108 cowpea genotypes comprising wild relatives (45), landraces (31), and improved (32) genotypes against three races of S. gesnerioides including races 1 and 5 in Burkina Faso. They used a combination of field plots located in Striga hotspots and pots artificially inoculated with Striga. They used the number of days to 50% flowering (FL) of the host plant, the number of Striga shoots per host plant (NSSP), and a Striga vigour score (SVIG) based on the Striga plant size for evaluation (Tignegre et al., 2013). The authors have reported differential responses of the assessed genotypes to Striga races in pot experiments. Results from the field experiment confirmed this aspect, where the genotypes demonstrated site-specific responses, indicating the existence of different Striga races. Fifty-one genotypes conferring resistance to Striga have been identified, including six CWR genotypes of V. unguiculata subsp. unguiculata var. spontanea (Schweinf.) Pasquet (now V. unguiculata subsp. unguiculata), namely, NS4, TV365-P41a, B12 07a, B32 03a, SP369A-P39b, and 2,300-P45 (Tignegre et al., 2013). Mechanisms for response and resistance genes could be explored from these genotypes for their use in cowpea breeding.
In Nigeria, Oyatomi et al. (2016) assessed the agro-morphological response to Striga of 350 accessions of wild Vigna belonging to 45 different species originating from 32 countries. A field trial was used where planting holes were artificially inoculated with seeds of S. gesnerioides. The accessions were classified based only on the number of Striga emergence as follows: resistant (no Striga emergence in the plot), moderately resistant (1–2 Striga emergence per plot), susceptible (3–4 Striga per plot), and highly susceptible (≥5 Striga emergence/plot). Twenty-one genotypes from 11 wild Vigna species, including V. glabrescens, V. marina, V. oblongifolia, V. parkeri, V. racemosa, V. reticulata, and V. vexillata have been identified as resistant to S. gesnerioides (Oyatomi et al., 2016). These can also be explored for their potential use in Vigna crop breeding for resistance to S. gesnerioides.
From the two studies, it would appear that specific populations of Vigna CWR taxa from areas of high diversity may be sources of Striga-resistance, especially those from the same natural range as the parasite, suggesting co-evolution. For example, four of the six genotypes identified as key potential sources of resistance to S. gesnerioides by Tignegre et al. (2013) were from West Africa, the hotspot for this parasite. Similarly, most of the taxa conferring resistance to S. gesnerioides identified by Oyatomi et al. (2016) originated from West and Central Africa. Mbuvi et al. (2017) used the same reasoning when selecting novel sources of Striga-resistance in sorghum. The authors used genotypes from northeastern Sudan, the natural range of the S. hermonthica that also harbours the highest wild sorghum diversity (Mbuvi et al., 2017).
Considering that Striga attack and damage take place underground way before the parasite emerges, Rubiales et al. (2006) contend that several tubercles may be established in a susceptible host plant but may fail to emerge or fully develop due to competition for nutrients. In such cases, susceptibility may be confounded with resistance if only the total number of emerged-parasite shoots or parasite size is considered (Rubiales et al., 2006), as used by Oyatomi et al. (2016). Further, the Striga score based on Striga plant height alone without paying particular attention to the host-plant health condition and vigour (e.g., Tignegre et al., 2013) may be misleading (Rubiales et al., 2006), suggesting the need to include a severity score based on the host plant’s general condition (i.e., damage rating score) (Dossa et al., 2023; Sawadogo et al., 2021b). Here, we present a selection of common screening methods and effective selection indices for Striga. Pot experiments and field trials, or a combination of these are the common methods, whereas the number of emerged-parasite shoots per host plant or plot is the most widely used index (Table 3).
Table 3. Selected studies for potential use in screening for adaptive response to Striga stress in Vigna CWRs.
Remarkable efforts supporting in situ conservation and use enhancement of Vigna CWRs have been made over the past two decades, albeit through few studies. We anticipate an increasing trend going forward to 2050, given the growing global interest in healthy and nutrient-dense diets that are eco-friendly, less costly, and climate-resilient in a changing climate. The inherent desirable traits in Vigna CWR taxa, including tolerance to drought and resistance to Striga gesnerioides are important in this respect. Moreover, most Vigna CWR taxa have various socio-cultural uses for acceptability as novel crops, and most could also have diverse food and pharmaceutical industrial applications. We conclude that Vigna CWRs provide a beacon of hope for sustainable and resilient food systems’ contribution in the face of climate change. This could be achieved by using Vigna CWRs as sources of genetic material for improving the resilience, quantity, and quality of domesticated Vigna taxa, as wild edible plants, or as a new profile of plants for neo-domestication, allowing farmers and consumers to switch to different crops. Addressing critical knowledge gaps highlighted in this paper would be crucial for decision-making in the conservation and use enhancement of Vigna CWRs for global food security and rural livelihood improvement.
LM: Methodology, Writing – original draft, Writing – review & editing. RI: Supervision, Writing – review & editing, Conceptualization. EA: Supervision, Writing – review & editing. SA: Supervision, Writing – review & editing. KS: Supervision, Writing – review & editing. CA: Supervision, Writing – review & editing. ACA: Writing – review & editing. AEA: Conceptualization, Supervision, Writing – review & editing.
The author(s) declare that financial support was received for the research, authorship, and/or publication of this article. This research was part of a PhD study for the first author (2018-2022) through a scholarship from the Regional Universities Forum for Capacity Building in Agriculture (RUFORUM) through the Intra-Africa-Partnership for Training Regional academic exchange for enhanced skills in fragile ecosystems management in Africa (REFORM).
The authors are grateful to the RUFORUM for providing a scholarship to LM. They are also appreciative of the reviewers’ invaluable comments made on the draft manuscript.
The authors declare that the research was conducted in the absence of any commercial or financial relationships that could be construed as a potential conflict of interest.
All claims expressed in this article are solely those of the authors and do not necessarily represent those of their affiliated organizations, or those of the publisher, the editors and the reviewers. Any product that may be evaluated in this article, or claim that may be made by its manufacturer, is not guaranteed or endorsed by the publisher.
The Supplementary material for this article can be found online at: https://www.frontiersin.org/articles/10.3389/frsus.2025.1453170/full#supplementary-material
SUPPLEMENTARY TABLE 1 | Global diversity, distribution, biology and conservation status of Vigna species.
1. ^https://powo.science.kew.org/ (accessed August 28, 2022)
2. ^https://www.ildis.org/LegumeWeb/ (accessed August 29, 2022)
3. ^https://africanplantdatabase.ch/ (accessed August 30, 2022)
Abdelghany, G., Wurm, P., Hoang, L. T. M., and Bellairs, S. M. (2021). Commercial cultivation of Australian wild Oryza spp.: a review and conceptual framework for future research needs. Agronomy 12:42. doi: 10.3390/agronomy12010042
Agbicodo, E. M., Fatokun, C. A., Muranaka, S., Visser, R. G. F., and Linden Van Der, C. (2009). Breeding drought tolerant cowpea: constraints, accomplishments, and future prospects. Euphytica 167, 353–370. doi: 10.1007/s10681-009-9893-8
Aguirre-Gutiérrez, J., van Treuren, R., Hoekstra, R., and van Hintum, T. J. L. (2017). Crop wild relatives range shifts and conservation in Europe under climate change. Divers. Distrib. 23, 739–750. doi: 10.1111/ddi.12573
Aimutis, W. R. (2022). Plant-based proteins: the good, bad, and ugly. Annu. Rev. Food Sci. Technol. 13, 1–17. doi: 10.1146/annurev-food-092221-041723
Ajayi, A. T., Gbadamosi, A. E., Olumekun, V. O., and Nwosu, P. O. (2020). GT biplot analysis of shoot traits indicating drought tolerance in cowpea [Vigna unguiculata (L.) Walp] accessions at vegetative stage. Int. J. Biosci. Technol. 13, 18–33. doi: 10.5281/zenodo.4019163
Al-Atawneh, N., Amri, A., Assi, R., and Maxted, N. (2007). “Management plans for promoting in situ conservation of local agrobiodiversity in the West Asia Centre of plant diversity” in Crop Wild Relative Conservation and Use. eds. N. Maxted, B. Ford-Lloyd, S. Kell, and M. Dulloo (Wallingford: CAB International), 340–363.
Alercia, A., López, F., Marsella, M., and Cerutti, A. L. (2021). Descriptors for crop wild relatives conserved in situ (CWRI v.1). International treaty on plant genetic resources for food and agriculture. Rome: FAO.
Alidu, M. S. (2018). Evaluation of cowpea genotypes for drought tolerance using the pot screening approach. Asian Res. J. Agric. 10, 1–11. doi: 10.9734/arja/2018/45806
Al-Khayri, J. M., Rashmi, R., Toppo, V., Chole, P. B., Banadka, A., Sudheer, W. N., et al. (2023). Plant secondary metabolites: the weapons for biotic stress management. Metabolites 13:716. doi: 10.3390/metabo13060716
Allen, E., Gaisberger, H., Magos Brehm, J., Maxted, N., Thormann, I., Lupupa, T., et al. (2019). A crop wild relative inventory for southern Africa: A first step in linking conservation and use of valuable wild populations for enhancing food security. Plant Genetic Res. 17, 128–139. doi: 10.1017/S1479262118000515
Allendorf, T. D., and Yang, J. (2013). The role of ecosystem services in park-people relationships: the case of Gaoligongshan nature reserve in Southwest China. Biol. Conserv. 167, 187–193. doi: 10.1016/j.biocon.2013.08.013
Alonge, S. O., Lagoke, S. T. O., and Ajakaiye, C. O. (2005a). Cowpea reactions to Striga gesnerioides I. Effect on growth. Crop Prot. 24, 565–573. doi: 10.1016/j.cropro.2004.08.014
Alonge, S. O., Lagoke, S. T. O., and Ajakaiye, C. O. (2005b). Cowpea reactions to Striga gesnerioides II. Effect on grain yield and nutrient composition. Crop Prot. 24, 575–580. doi: 10.1016/j.cropro.2004.08.015
Álvarez Muñiz, C., Rubio Teso, M. L., Magos Brehm, J., Ralli, P., Palmé, A., and Dulloo, E. (2020). A list of crop wild relative in situ conservation case studies. Available at: https://more.bham.ac.uk/farmerspride/wpcontent/uploads/sites/19/2020/05/Crop_wild_Relative_in_situ_case_studies.Pdf (Accessed May 19, 2020).
Amkul, K., Wang, L., Somta, P., Wang, S., and Cheng, X. (2019). Construction of a high-density linkage map and genome dissection of bruchid resistance in zombi pea (Vigna vexillata (L.) A. Rich). Sci. Rep. 9:11719. doi: 10.1038/s41598-019-48239-5
Amusan, I. O., Rich, P. J., Menkir, A., Housley, T., and Ejeta, G. (2008). Resistance to Striga hermonthica in a maize inbred line derived from Zea diploperennis. New Phytol. 178, 157–166. doi: 10.1111/j.1469-8137.2007.02355.x
Assogbadjo, A. E., Chadare, F. J., Manda, L., and Sinsin, B. (2021). A 20-year journey through an orphan African baobab (Adansonia digitata L.) towards improved food and nutrition security in Africa. Front. Sust. Food Syst. 5, 1–17. doi: 10.3389/fsufs.2021.675382
Atera, E., and Itoh, K. (2011). Evaluation of ecologies and severity of Striga weed on rice in sub-Saharan Africa. Agric. Biol. J. N. Am. 2, 752–760. doi: 10.5251/abjna.2011.2.5.752.760
Atis, I., Atak, M., Can, E., and Mavi, K. (2011). Seed coat color effects on seed quality and salt tolerance of red clover (Trifolium pratense). Int. J. Agric. Biol. 13, 1560–8530.
Azam-Ali, S. N. (2021). The ninth revolution: Transforming food Systems for Good. In the ninth revolution: Transforming food Systems for Good. Hackensack, NJ: World Scientific Publishing Company.
Bartlett, M. E., Moyers, B. T., Man, J., Subramaniam, B., and Makunga, N. P. (2023). The power and perils of De Novo domestication using genome editing. Ann. Rev. Plant Biol. 74, 727–750. doi: 10.1146/annurev-arplant-053122
Basu, S., Ramegowda, V., Kumar, A., and Pereira, A. (2016). Plant adaptation to drought stress. F1000Research 5:1554. doi: 10.12688/f1000research.7678.1
Beebe, S. E., Rao, I. M., Blair, M. W., and Acosta-Gallegos, J. A. (2013). Phenotyping common beans for adaptation to drought. Front. Physiol. 4:35. doi: 10.3389/fphys.2013.00035
Bellon, M. R., Dulloo, E., Sardos, J., Thormann, I., and Burdon, J. J. (2017). In situ conservation—harnessing natural and human-derived evolutionary forces to ensure future crop adaptation. Evol. Appl. 10, 965–977. doi: 10.1111/eva.12521
Beshir, H. M., Bueckert, R., and Tar’an, B. (2016). Effect of temporary drought at different growth stages on snap bean pod quality and yield. Afr. Crop. Sci. J. 24:317. doi: 10.4314/acsj.v24i3.8
Bhadkaria, A., Narvekar, D. T., Gupta, N., Khare, A., and Bhagyawant, S. S. (2022). “Moth bean (Vigna aconitifolia (Jacq.) Marechal) seeds: A review on nutritional properties and health benefits” in Discover food (Cham: Springer Nature).
Bisht, I. S., Bhat, K. V., Lakhanpaul, S., Latha, M., Jayan, P. K., Biswas, B. K., et al. (2005). Diversity and genetic resources of wild Vigna species in India. Genet. Resour. Crop. Evol. 52, 53–68. doi: 10.1007/s10722-005-0286-0
Bisht, I. S., and Singh, M. (2013). “Asian Vigna” in Genetic and genomic resources of grain legume improvement. ed. H. D. Upadhyaya (Amsterdam: Elsevier), 237–267.
Bohra, A., Kilian, B., Sivasankar, S., Caccamo, M., Mba, C., McCouch, S. R., et al. (2021). Reap the crop wild relatives for breeding future crops. Trends Biotechnol. 40, 412–431. doi: 10.1016/j.tibtech.2021.08.009
Botanga, C. J., and Timko, M. P. (2006). Phenetic relationships among different races of Striga gesnerioides (Willd.) Vatke from West Africa. Genome 49, 1351–1365. doi: 10.1139/G06-086
Boukar, O., Abberton, M., Oyatomi, O., Togola, A., Tripathi, L., and Fatokun, C. (2020). Introgression breeding in cowpea [Vigna unguiculata (L.) Walp.]. Front. Plant Sci. 11:567425. doi: 10.3389/fpls.2020.567425
Boukar, O., Massawe, F., Muranaka, S., Franco, J., Maziya-Dixon, B., Singh, B., et al. (2011). Evaluation of cowpea germplasm lines for protein and mineral concentrations in grains. Plant Genetic Res. Characterisation Utilisation 9, 515–522. doi: 10.1017/S1479262111000815
Brozynska, M., Furtado, A., and Henry, R. J. (2015). Genomics of crop wild relatives: expanding the gene pool for crop improvement. Plant Biotechnol. J. 14, 1070–1085. doi: 10.1111/pbi.12454
Carvalho, M., Matos, M., Castro, I., Monteiro, E., Rosa, E., Lino-Neto, T., et al. (2019). Screening of worldwide cowpea collection to drought tolerant at a germination stage. Sci. Hortic. 247, 107–115. doi: 10.1016/j.scienta.2018.11.082
Casas, A., Otero-Arnaiz, A., Pérez-Negrón, E., and Valiente-Banuet, A. (2007). In situ management and domestication of plants in Mesoamerica. Annal. Bot. 100, 1101–1115. doi: 10.1093/aob/mcm126
Castagnino, F., Estévez, R. A., Caillaux, M., Velez-Zuazo, X., and Gelcich, S. (2023). Local ecological knowledge (LEK) suggests overfishing and sequential depletion of Peruvian coastal groundfish. Marine Coastal Fisher. 15:10272. doi: 10.1002/mcf2.10272
Castañeda-Álvarez, N. P., Khoury, C. K., Achicanoy, H. A., Bernau, V., Dempewolf, H., Eastwood, R. J., et al. (2016). Global conservation priorities for crop wild relatives. Nat. Plants 2:16022. doi: 10.1038/NPLANTS.2016.22
Castelli, A., and Mikić, A. (2019). Vigna lanceolata in the fire-stick farming and the Australian aboriginal culture. Ratarstvo i povrtarstvo 56:19176. doi: 10.5937/ratpov56-19716
Catarino, S., Rangel, J., Darbyshire, I., Costa, E., Duarte, M. C., and Romeiras, M. M. (2021). Conservation priorities for African Vigna species: unveiling Angola’s diversity hotspots. Global Ecol. Conserv. 25:e01415. doi: 10.1016/j.gecco.2020.e01415
CBD (2005). Handbook of the convention on biological diversity including its Cartagena protocol on biosafety convention on biological diversity. 3rd Edn. Montreal, QC: Secretariat of the Convention on Biological Diversity.
CBD (2010). The Strategic Plan for Biodiversity (2011–2020) and the Aichi Biodiversity Targets. Montreal, QC: Secretariat of the Convention on Biological Diversity.
CBD (2022). Kunming-Montreal global biodiversity framework. CBD/COP/15/L25, UN convention on biological diversity. Secretariat of the convention on biological diversity. Montreal, QC: Secretariat of the Convention on Biological Diversity.
Chamoli, N., Prabha, D., and Chauhan, J. S. (2021). Correlation in seed coat colour and pod colour of French bean collected form Garhwal Himalayas. J. Mountain Res. 16:35. doi: 10.51220/jmr.v16i3.35
Cissoko, M., Boisnard, A., Rodenburg, J., Press, M. C., and Scholes, J. D. (2011). New Rice for Africa (NERICA) cultivars exhibit different levels of post-attachment resistance against the parasitic weeds Striga hermonthica and Striga asiatica. New Phytol. 192, 952–963. doi: 10.1111/j.1469-8137.2011.03846.x
Cohn, A. S., Newton, P., Gil, J. D. B., Kuhl, L., Samberg, L., and Ricciardi, V. (2017). Smallholder Agriculture and Climate Change. Annu. Rev. Environ. Resour. 42, 347–75. doi: 10.1146/annurev-environ-102016-060946
Cortés, A. J., Monserrate, F. A., Ramírez-Villegas, J., Madriñán, S., and Blair, M. W. (2013). Drought tolerance in wild plant populations: the case of common beans (Phaseolus vulgaris L.). PLoS One 8:e62898. doi: 10.1371/journal.pone.0062898
Coyne, C. J., Kumar, S., von Wettberg, E. J. B., Marques, E., Berger, J. D., Redden, R. J., et al. (2020). Potential and limits of exploitation of crop wild relatives for pea, lentil, and chickpea improvement. Legume Sci. 2:e36. doi: 10.1002/leg3.36
Cui, Q., Xiong, H., Yufeng, Y., Eaton, S., Imamura, S., Santamaria, J., et al. (2020). Evaluation of drought tolerance in Arkansas cowpea lines at seedling stage. HortScience 55, 1132–1143. doi: 10.21273/HORTSCI15036-20
Dafaallah, A. B. (2019). Biology and physiology of Witchweed (Striga spp.): a review. IJAMR 3, 42–51.
Dai, A. (2013). Increasing drought under global warming in observations and models. Nat. Clim. Chang. 3, 52–58. doi: 10.1038/nclimate1633
Dakora, F. D. (2013). Biogeographic distribution, nodulation and nutritional attributes of underutilized indigenous African legumes. Acta Hortic. 979, 53–64. doi: 10.17660/ActaHortic.2013.979.3
David, O. A., Obiakara, M. C., Fabolude, G. O., Akomolafe, G. F., and Ajiboye, M. D. (2022). Habitat suitability and dispersal of invasive Striga species under climate change in Africa. Afr. J. Ecol. 60, 1143–1154. doi: 10.1111/aje.13064
Davis, A. P., Chadburn, H., Moat, J., O’Sullivan, R., Hargreaves, S., and Lughadha, E. N. (2019). High extinction risk for wild coffee species and implications for coffee sector sustainability. Sci. Adv. 5:eaav3473. doi: 10.1126/sciadv.aav3473
Delgado-Salinas, A., Lavin, M., Snak, C., and Lewis, G. P. (2022). Systematics of Vigna subgenus Lasiospron (Leguminosae: Papilionoideae: Phaseolinae). Syst. Bot. 47, 97–124. doi: 10.1600/036364422X16442668423428
Delgado-Salinas, A., Thulin, M., Pasquet, R., Weeden, N., and Lavin, M. (2011). Vigna (Leguminosae) sensu lato: the names and identities of the American segregate genera. Am. J. Bot. 98, 1694–1715. doi: 10.3732/ajb.1100069
Dempewolf, H., Baute, G., Anderson, J., Kilian, B., Smith, C., and Guarino, L. (2017). Past and future use of wild relatives in crop breeding. Crop Sci. 57, 1070–1082. doi: 10.2135/cropsci2016.10.0885
Dempewolf, H., Eastwood, R. J., Guarino, L., Khoury, C. K., Müller, J. V., and Toll, J. (2014). Adapting agriculture to climate change: A global initiative to collect, conserve, and use crop wild relatives. Agroecol. Sust. Food Syst. 38, 369–377. doi: 10.1080/21683565.2013.870629
Devi, P. I., Manjula, M., and Bhavani, R. V. (2022). Agrochemicals, environment, and human health. Ann. Rev. Environ. Res. 47, 399–421. doi: 10.1146/annurev-environ-120920
Diamond, J. (2002). Evolution, consequences and future of plant and animal domestication. Nature 418:issue 6898. doi: 10.1038/nature01019
Dong, S., Jiang, Y., Dong, Y., Wang, L., Wang, W., Ma, Z., et al. (2019). A study on soybean responses to drought stress and rehydration. Saudi J. Biol. Sci. 26, 2006–2017. doi: 10.1016/j.sjbs.2019.08.005
Dossa, E. N., Shimelis, H., Shayanowako, A. I. T., and Laing, M. D. (2023). A meta-analysis of the effects of Striga control methods on maize, sorghum, and major millets production in sub-Saharan Africa. Crop Sci. 63, 460–479. doi: 10.1002/csc2.20889
Drucker, A. G., Mponya, N. K., Grazioli, F., Maxted, N., Brehm, J. M., and Dulloo, E. (2023). Community-level incentive mechanisms for the conservation of crop wild relatives: a Malawi case study. Plan. Theory 12:1030. doi: 10.3390/plants12051030
Dulloo, M. E., Labokas, J., Iriondo, J. M., Maxted, N., Lane, A., Laguna, E., et al. (2008). Genetic reserve location and design. In conserving plant genetic diversity in protected. Areas: Population Management of Crop Wild Relatives. Wallingford: CABI.
Dulloo, E., Maxted, N., Shave, J., Pungulani, L., Hamisy, W., Munkombwe, G., et al. (2021). Crop wild relatives in the South African development community. Policy brief no. 49. Rome: Alliance of Bioversity International and CIAT. Available at: https://cgspace.cgiar.org/items/e413b3b5-fc51-46ed-a034-0d77f6e9976d (Accessed December 27, 2024).
Editorial, N. (2024). Down-to-earth drought resistance. Nat. Plants 10, 525–526. doi: 10.1038/s41477-024-01686-z
Ejeta, G. (2007). “The Striga scourge in Africa: A growing pandemic” in Integrating new Technologies for Striga Control: Towards ending the witch-Hunt. eds. G. Ejeta and J. Gressel, vol. 53 (London: World Scientific), 3–16.
Emeghebe, A. M., Ellis-Jones, J., Schulz, S., Chikoye, D., Douthwaite, B., Kureh, I., et al. (2004). Farmers’ perception of the Striga problem and its control in northern Nigeria. Exp. Agric. 40, 215–232. doi: 10.1017/S0014479703001601
Engels, J. M. M., and Ebert, A. W. (2021a). A critical review of the current global ex situ conservation system for plant agrobiodiversity. I. History of the development of the global system in the context of the political/legal framework and its major conservation components. Plan. Theory 10:557. doi: 10.3390/plants10081557
Engels, J. M. M., and Ebert, A. W. (2021b). A critical review of the current global ex situ conservation system for plant agrobiodiversity. II. Strengths and weaknesses of the current system and recommendations for its improvement. Plan. Theory 10:1904. doi: 10.3390/plants10091904
Engels, J. M. M., Maggioni, L., Maxted, N., and Dulloo, M. E. (2008). “Complementing in situ conservation with ex situ measures” in Conserving plant genetic diversity in protected areas: Population Management of Crop Wild Relatives. eds. J. M. Iriondo and N. Maxted (Wallingford: CABI Publishing), 169–181.
Engels, J. M. M., and Thormann, I. (2020). Main challenges and actions needed to improve conservation and sustainable use of our crop wild relatives. Plan. Theory 9, 1–26. doi: 10.3390/plants9080968
Enriquez, J. P., and Archila-Godinez, J. C. (2022). “Social and cultural influences on food choices: A review” in Critical reviews in food science and nutrition. eds. V. S. Govindarajan and T. Nadu (New York, NY: Taylor and Francis Ltd.), 3698–3704.
Fagandini Ruiz, F., Bazile, D., Drucker, A. G., Tapia, M., and Chura, E. (2021). Geographical distribution of quinoa crop wild relatives in the Peruvian Andes: a participatory mapping initiative. Environ. Dev. Sustain. 23, 6337–6358. doi: 10.1007/s10668-020-00875-y
Fang, X., Turner, N. C., Yan, G., Li, F., and Siddique, K. H. M. (2010). Flower numbers, pod production, pollen viability, and pistil function are reduced and flower and pod abortion increased in chickpea (Cicer arietinum L.) under terminal drought. J. Exp. Bot. 61, 335–345. doi: 10.1093/jxb/erp307
Fang, Y., and Xiong, L. (2015). General mechanisms of drought response and their application in drought resistance improvement in plants. Cell. Mol. Life Sci. 72, 673–689. doi: 10.1007/s00018-014-1767-0
FAO (2009). International treaty on plant genetic resources for food and agriculture. Rome: FAO. Available at: https://www.fao.org/3/i0510e/i0510e.pdf (Accessed December 4, 2021).
FAO (2010). The second report on the state of the World's plant genetic resources for food and agriculture. Commission on genetic resources for food and agriculture food and agriculture Organization of the United Nations. Rome: FAO.
FAO (2011). Second global plan of action for plant genetic resources for food and agriculture. Commission on Genetic Resources for Food and Agriculture. Rome: FAO.
FAO (2018). Voluntary guidelines for the conservation and sustainable use of crop wild relatives and wild food plants. Rome: FAO.
Farooq, M., Gogoi, N., Barthakur, S., Baroowa, B., Bharadwaj, N., Alghamdi, S. S., et al. (2017). Drought stress in grain legumes during reproduction and grain filling. J. Agron. Crop Sci. 203, 81–102. doi: 10.1111/jac.12169
Fatokun, C. A., Boukar, O., and Muranaka, S. (2012). Evaluation of cowpea (Vigna unguiculata (L.) Walp.) germplasm lines for tolerance to drought. Plant Genetic Res. Characterisation Utilisation 10, 171–176. doi: 10.1017/S1479262112000214
Feng, Q., Niu, Z., Zhang, S., Wang, L., Qun, S., Yan, Z., et al. (2023). Mung bean protein as an emerging source of plant protein: a review on production methods, functional properties, modifications and its potential applications. J. Sci. Food Agric. 104, 2561–2573. doi: 10.1002/jsfa.13107
Fernández-Aparicio, M., Delavault, P., and Timko, M. P. (2020). Management of infection by parasitic weeds: a review. Plan. Theory 9, 1–26. doi: 10.3390/plants9091184
Fernández-Aparicio, M., Flores, F., and Rubiales, D. (2012). Escape and true resistance to crenate broomrape (Orobanche crenata Forsk.) in grass pea (Lathyrus sativus L.) germplasm. Field Crop Res. 125, 92–97. doi: 10.1016/j.fcr.2011.09.003
Fernie, A. R., and Yan, J. (2019). De novo domestication: an alternative route toward new crops for the future. Mol. Plant 12, 615–631. doi: 10.1016/j.molp.2019.03.016
Ferrari, L., Panaite, S. A., Bertazzo, A., and Visioli, F. (2022). Animal- and plant-based protein sources: A scoping review of human health outcomes and environmental impact. Nutrients 14:115. doi: 10.3390/nu14235115
Flores-Saavedra, M., Plazas, M., Vilanova, S., Prohens, J., and Gramazio, P. (2023). Induction of water stress in major Solanum crops: a review on methodologies and their application for identifying drought tolerant materials. Sci. Hortic. 318:112105. doi: 10.1016/j.scienta.2023.112105
Ford-Lloyd, B. V., Schmidt, M., Armstrong, S. J., Barazani, O., Engels, J., Hadas, R., et al. (2011). Crop wild relatives - undervalued, underutilized and under threat? Bioscience 61, 559–565. doi: 10.1525/bio.2011.61.7.10
Foyer, C. H., Lam, H. M., Nguyen, H. T., Siddique, K. H. M., Varshney, R. K., Colmer, T. D., et al. (2016). Neglecting legumes has compromised human health and sustainable food production. Nat. Plants 2:16112. doi: 10.1038/NPLANTS.2016.112
Francavilla, A., and Joye, I. J. (2020). Anthocyanins in whole grain cereals and their potential effect on health. Nutrients 12:922. doi: 10.3390/nu12102922
Gasparini, K., Moreira, J., Peres, L. E. P., and Zsögön, A. (2021). De novo domestication of wild species to create crops with increased resilience and nutritional value. Curr. Opin. Plant Biol. 60:102006. doi: 10.1016/j.pbi.2021.102006
George, N. A., Coorey, R., Dixon, K., and Bennett, S. J. (2023). A review of the de novo domestication and cultivation of edible Australian native plants as food crops. J. Agric. Sci. 161, 778–793. doi: 10.1017/S0021859624000078
Geyik, Ö., Hadjikakou, M., and Bryan, B. A. (2022). Climate-friendly and nutrition-sensitive interventions can close the global dietary nutrient gap while reducing GHG emissions. Nat. Food 4, 61–73. doi: 10.1038/s43016-022-00648-y
Ghanem, M. E. (2022). Africa’s forgotten crops could offset growing food insecurity. Nature 605:30. doi: 10.1038/d41586-022-01185-1
Godfray, H. C. J. (2014). The challenge of feeding 9-10 billion people equitably and sustainably. J. Agric. Sci. 152, 2–S8. doi: 10.1017/S0021859613000774
Godfray, H. C. J., Aveyard, P., Garnett, T., Hall, J. W., Key, T. J., Lorimer, J., et al. (2018). Meat consumption, health, and the environment. Science 361:aam5324. doi: 10.1126/science.aam5324
Godfray, H. C. J., Beddington, J. R., Crute, I. R., Haddad, L., Lawrence, D., Muir, J. F., et al. (2010). Food security: the challenge of feeding 9 billion people. Science 327, 812–818. doi: 10.1126/science.1185383
Goettsch, B., Urquiza-Haas, T., Koleff, P., Acevedo Gasman, F., Aguilar-Meléndez, A., Alavez, V., et al. (2021). Extinction risk of Mesoamerican crop wild relatives. Plants People Planet 3, 775–795. doi: 10.1002/ppp3.10225
Gogoi, A., Baruah, N., Poudel, M., Gupta, R., Baruah, G., and Kumar Borah, B. (2022). Parasitic plants as vectors for pathogens. London: IntechOpen.
Gore, P. G., Tripathi, K., Pratap, A., Bhat, K. V., Umdale, S. D., Gupta, V., et al. (2019). Delineating taxonomic identity of two closely related Vigna species of section Aconitifoliae: V. Trilobata (L.) Verdc. And V. Stipulacea (Lam.) Kuntz in India. Genet. Resour. Crop. Evol. 66, 1155–1165. doi: 10.1007/s10722-019-00767-9
Gorim, L. Y., and Vandenberg, A. (2017). Evaluation of wild lentil species as genetic resources to improve drought tolerance in cultivated lentil. Frontiers. Plant Sci. 8:1129. doi: 10.3389/fpls.2017.01129
Guadarrama-Escobar, L. M., Hunt, J., Gurung, A., Zarco-Tejada, P. J., Shabala, S., Camino, C., et al. (2024). Back to the future for drought tolerance. New Phytologist 242:19619. doi: 10.1111/nph.19619
Haddaway, N. R., Woodcock, P., Macura, B., and Collins, A. (2015). Making literature reviews more reliable through application of lessons from systematic reviews. Conserv. Biol. 29, 1596–1605. doi: 10.1111/cobi.12541
Hajjar, R., and Hodgkin, T. (2007). The use of wild relatives in crop improvement: A survey of developments over the last 20 years. Euphytica 156, 1–13. doi: 10.1007/s10681-007-9363-0
Harlan, J. R., and De, J. M. J. (1971). Toward a rational classification of cultivated plants. Wet Source: Taxon 20, 509–517.
Harouna, D. V., Venkataramana, P. B., Matemu, A. O., and Alois Ndakidemi, P. (2020). Agro-morphological exploration of some unexplored wild Vigna legumes for domestication. Agronomy 10:111. doi: 10.3390/agronomy10010111
Harouna, D. V., Venkataramana, P. B., Ndakidemi, P. A., and Matemu, A. O. (2018). Under-exploited wild Vigna species potentials in human and animal nutrition: a review. Glob. Food Sec. 18, 1–11. doi: 10.1016/j.gfs.2018.06.002
Haruna, P., Asare, A. T., Asare-Bediako, E., and Kusi, F. (2018). Farmers and agricultural extension officers perception of Striga gesnerioides (Willd.) Vatke parasitism on cowpea in the upper east region of Ghana. Adv. Agric. 2018, 1–11. doi: 10.1155/2018/7319204
Hayatu, M., Shehu, M., and Haruna, H. (2016). Effect of different levels of Striga gesnerioides on the growth and yield of some local and improved cowpea (Vigna unguiculata (L) Walp) varieties. J. Pure Appl. Sci. 9:76. doi: 10.4314/bajopas.v9i1.12
Herbold, T., and Engels, J. M. M. (2023). Genebanks at risk: Hazard assessment and risk management of national and international Genebanks. Plan. Theory 12:874. doi: 10.3390/plants12152874
Heusala, H., Sinkko, T., Mogensen, L., and Knudsen, M. T. (2020). Carbon footprint and land use of food products containing oat protein concentrate. J. Clean. Prod. 276:122938. doi: 10.1016/j.jclepro.2020.122938
Heywood, V. H. (2019). Conserving plants within and beyond protected areas – still problematic and future uncertain. Plant Div. 41, 36–49. doi: 10.1016/j.pld.2018.10.001
HLPE. (2023). Reducing inequalities for food security and nutrition. Rome, CFS HLPE-FSN. Available at: https://openknowledge.fao.org/server/api/core/bitstreams/3b32bc6c-b4e8-46b3-bdae-acc32afe222f/content (Accessed December 17, 2023).
Huang, K., Mellor, K. E., Paul, S. N., Lawson, M. J., Mackey, A. J., and Timko, M. P. (2012). Global changes in gene expression during compatible and incompatible interactions of cowpea (Vigna unguiculata L.) with the root parasitic angiosperm Striga gesnerioides. BMC Genomics 13:402. doi: 10.1186/1471-2164-13-402
Hunter, D., Guarino, L., Khoury, C., and Dempewolf, H. (2011). “A community divided: lessons from the conservation of crop wild relatives around the world” in Agrobiodiversity conservation: Securing the diversity of crop wild relatives and landraces. eds. N. Maxted, E. Dulloo, B. Ford-Llyod, L. Frese, and J. Iriondo (Wallingford: CABI International), 298–304.
IPCC (2021). “Summary for Policymakers” in Climate Change 2021: The Physical Science Basis: Contribution of Working Group I to the Sixth Assessment Report of the Intergovernmental Panel on Climate Change. eds. V. Masson-Delmotte, P. Zhai, and A. Pirani (Geneva: Intergovernmental Panel on Climate Change).
Iriondo, J. M., Ford-Lloyd, B., De Hond, L., Kell, S. P., Lefèvre, F., Korpelainen, H., et al. (2008). “Plant population monitoring methodologies for the in situ genetic conservation of CWR” in Conserving plant genetic diversity in protected areas: Population Management of Crop Wild Relatives. eds. J. M. Iriondo, N. Maxted, and M. E. Dulloo (Wallingford: CABI Publishing), 88–123.
Iriondo, J. M., Magos Brehm, J., Dulloo, M. E., and Maxted, N. (2021). Crop Wild Relative Population Management Guidelines. Farmer’s Pride: Networking, partnerships and tools to enhance in situ conservation of European plant genetic resources. Available at: https://more.bham.ac.uk/farmerspride/wp-content/uploads/sites/19/2021/07/Crop_Wild_Relative_Population_Management_Guidelines.pdf (Accessed July 19, 2021).
Iseki, K., Takahashi, Y., Muto, C., Naito, K., and Tomooka, N. (2018). Diversity of drought tolerance in the genus Vigna. Front. Plant Sci. 9:729. doi: 10.3389/fpls.2018.00729
IUCN (2019). Recognising and reporting other effective area-based conservation measures Recognising and reporting other effective area-based conservation measures. Gland: IUCN, International Union for Conservation of Nature.
Jamil, M., Kountche, B. A., and Al-Babili, S. (2021). Current progress in Striga management. Plant Physiol. 185, 1339–1352. doi: 10.1093/plphys/kiab040
Jaradat, A. A. (2015). “Beyond biodiversity: ecosystem Services of Crop Wild Relatives” in Crop wild relatives and climate change. eds. P. Redden and R. Yadav (New York, NY: John Wiley & Sons, Inc.), 336–349.
Jarvis, S., Fielder, H., Hopkins, J., Maxted, N., and Smart, S. (2015). Distribution of crop wild relatives of conservation priority in the UK landscape. Biol. Conserv. 191, 444–451. doi: 10.1016/j.biocon.2015.07.039
Jarvis, A., Lane, A., and Hijmans, R. J. (2008). The effect of climate change on crop wild relatives. Agric. Ecosyst. Environ. 126, 13–23. doi: 10.1016/j.agee.2008.01.013
Jayathilake, C., Visvanathan, R., Deen, A., Bangamuwage, R., Jayawardana, B. C., Nammi, S., et al. (2018). Cowpea: an overview on its nutritional facts and health benefits. J. Sci. Food Agric. 98, 4793–4806. doi: 10.1002/jsfa.9074
Jensen, E. S., Peoples, M. B., Boddey, R. M., Gresshoff, P. M., Henrik, H. N., Alves, B. J. R., et al. (2012). Legumes for mitigation of climate change and the provision of feedstock for biofuels and biorefineries. A review. Agron. Sust. Dev. 32:56. doi: 10.1007/s13593-011-0056-7
Jensen, M., and Pliscoff, P. (2023). Endemic crop wild relatives in Chile towards the end of the 21st century: protected areas and agricultural expansion. Crop Sci. 63, 2241–2254. doi: 10.1002/csc2.20962
Jhu, M. Y., Kawa, D., and Brady, S. M. (2023). The genetic basis of plants’ battle against witchweeds: linking immune responses to distinct resistance mechanisms. J. Exp. Bot. 74, 4903–4909. doi: 10.1093/jxb/erad305
Jhu, M. Y., and Sinha, N. R. (2022). Parasitic plants: an overview of mechanisms by which plants perceive and respond to parasites. Annu. Rev. Plant Biol. 73, 433–455. doi: 10.1146/annurev-arplant-102820-100635
Kanagavel, A., Parvathy, S., Tapley, B., Nirmal, N., Selvaraj, G., Raghavan, R., et al. (2020). Are local and traditional ecological knowledge suitable tools for informing the conservation of threatened amphibians in biodiversity hotspots? Herpetol. Bullet. 153, 3–13. doi: 10.33256/HB153.313
Kansaye, A., Kansaye, L., Mallé, A., Nebie, B., and Donigolo, C. (2022). Evaluation of West African Sorghum varieties for resistance to Striga in the Sudano-Sahelian zone of Mali. Int. J. Innov. Sci. Res. Technolo. 7:1878.
Kapazoglou, A., Gerakari, M., Lazaridi, E., Kleftogianni, K., Sarri, E., Tani, E., et al. (2023). Crop wild relatives: A valuable source of tolerance to various abiotic stresses. Plan. Theory 12:328. doi: 10.3390/plants12020328
Kasso, M., and Balakrishnan, M. (2013). Ex situ conservation of biodiversity with particular emphasis to Ethiopia. ISRN Biodiv. 2013, 1–11. doi: 10.1155/2013/985037
Kell, S. P., Ford-Lloyd, B. V., Brehm, J. M., Iriondo, J. M., and Maxted, N. (2017). Broadening the base, narrowing the task: prioritizing crop wild relative taxa for conservation action. Crop Sci. 57, 1042–1058. doi: 10.2135/cropsci2016.10.0873
Kell, S.P., Maxted, N., and Bliz, M. (2011). European crop wild relative threat assessment: Knowledge gained and lessons Learnt. In N. Maxted, Dulloo, E., Ford-Lloyd, B. V., and Frese, L., Agrobiodiversity conservation: Securing the diversity of crop wild relatives and landraces. Wallingford: CAB International.
Khan, Z. R., Midega, C. A. O., Hassanali, A., Pickett, J. A., and Wadhams, L. J. (2007). Assessment of different legumes for the control of Striga hermonthica in maize and sorghum. Crop Sci. 47, 730–736. doi: 10.2135/cropsci2006.07.0487
Khatun, M., Sarkar, S., Era, F. M., Islam, A. K. M. M., Anwar, M. P., Fahad, S., et al. (2021). Drought stress in grain legumes: effects, tolerance mechanisms and management. Agronomy 11:374. doi: 10.3390/agronomy11122374
Khoury, C. K., Brush, S., Costich, D. E., Curry, H. A., de Haan, S., Engels, J. M. M., et al. (2022). Crop genetic erosion: understanding and responding to loss of crop diversity. New Phytol. 233, 84–118. doi: 10.1111/nph.17733
Khoury, C. K., Carver, D., Greene, S. L., Williams, K. A., Achicanoy, H. A., Schori, M., et al. (2020a). Crop wild relatives of the United States require urgent conservation action. Proc. Natl. Acad. Sci. 117, 33351–33357. doi: 10.1073/pnas.2007029117
Khoury, C. K., Carver, D., Kates, H. R., Achicanoy, H. A., van Zonneveld, M., Thomas, E., et al. (2020b). Distributions, conservation status, and abiotic stress tolerance potential of wild cucurbits (Cucurbita L.). Plants People Planet 2, 269–283. doi: 10.1002/ppp3.10085
Kim, S. K., Jung, J., Jung, J. H., Lee, B. W., Kang, S. S., Roh, G. S., et al. (2018). Antidiabetic effects of Vigna nakashimae extract in humans: a preliminary study. J. Altern. Complement. Med. 24, 249–253. doi: 10.1089/acm.2017.0114
Konrad, W., Burkhardt, J., Ebner, M., and Roth-Nebelsick, A. (2015). Leaf pubescence as a possibility to increase water use efficiency by promoting condensation. Ecohydrology 8, 480–492. doi: 10.1002/eco.1518
Kooyers, N. J. (2015). The evolution of drought escape and avoidance in natural herbaceous populations. Plant Sci. 234, 155–162. doi: 10.1016/j.plantsci.2015.02.012
Kopecká, R., Kameniarová, M., Černý, M., Brzobohatý, B., and Novák, J. (2023). Abiotic stress in crop production. Int. J. Mol. Sci. 24:6603. doi: 10.3390/ijms24076603
Kountche, B. A., Jamil, M., Yonli, D., Nikiema, M. P., Blanco-Ania, D., Asami, T., et al. (2019). Suicidal germination as a control strategy for Striga hermonthica (Benth.) in smallholder farms of sub-Saharan Africa. Plants, People, Planet 1, 107–118. doi: 10.1002/ppp3.32
Kroschel, J. (2001). “A technical manual for parasitic weed research and extension” in A technical manual for parasitic weed research and extension. ed. J. Kroschel (Cham: Springer).
Labastida, D., Ingvarsson, P. K., and Rendón-Anaya, M. (2023). Dissecting the genetic basis of drought responses in common bean using natural variation. Front. Plant Sci. 14:1143873. doi: 10.3389/fpls.2023.1143873
Lado, A., Umar Sani, F., Usman Yahaya, S., and Kwalle Karaye, A. (2018). Efficacy of Parkia biglobosa fruit powder on the control of Striga in cowpea cropping systems in the Sudan-savanna, Nigeria. Heliyon 4:e00733. doi: 10.1016/j.heliyon.2018.e00733
Lala, S., Amri, A., and Maxted, N. (2018). Towards the conservation of crop wild relative diversity in North Africa: checklist, prioritisation and inventory. Genet. Resour. Crop. Evol. 65, 113–124. doi: 10.1007/s10722-017-0513-5
Leakey, R. R. B., Tientcheu Avana, M.-L., Awazi, N. P., Assogbadjo, A. E., Mabhaudhi, T., Hendre, P. S., et al. (2022). The future of food: domestication and commercialization of indigenous food crops in Africa over the third decade (2012–2021). Sustain. For. 14:2355. doi: 10.3390/su14042355
Leandre, S. P., Francis, K., Richard, A., Joseph, B., Jean, B. T., Jeremy, T. O., et al. (2018). Screening for resistance to Striga gesnerioides and estimation of yield loss among cowpea (Vigna unguiculata (L.) Walp.) progenies in the upper east region of Ghana. Afr. J. Agric. Res. 13, 1430–1442. doi: 10.5897/ajar2018.13083
Leduc, A. O. H. C., De Carvalho, F. H. D., Hussey, N. E., Reis-Filho, J. A., Longo, G. O., and Lopes, P. F. M. (2021). Local ecological knowledge to assist conservation status assessments in data poor contexts: a case study with the threatened sharks of the Brazilian northeast. Biodivers. Conserv. 30, 819–845. doi: 10.1007/s10531-021-02119-5
Leimona, B., van Noordwijk, M., de Groot, R., and Leemans, R. (2015). Fairly efficient, efficiently fair: lessons from designing and testing payment schemes for ecosystem services in Asia. Ecosyst. Serv. 12, 16–28. doi: 10.1016/j.ecoser.2014.12.012
Lewis, G., Schrire, B., Mackinder, B., and Lock, M. (2005). Legumes of the world. Royal Bot. Gardens 55:251. doi: 10.2307/25065563
Li, D. Z., and Pritchard, H. W. (2009). The science and economics of ex situ plant conservation. Trends Plant Sci. 14, 614–621. doi: 10.1016/j.tplants.2009.09.005
Li, J., and Timko, M. P. (2009). Gene-for-gene resistance in Striga-cowpea associations. Science 325:1094. doi: 10.1126/science.1174754
Lira, R., Téllez, O., and Dávila, P. (2009). The effects of climate change on the geographic distribution of Mexican wild relatives of domesticated Cucurbitaceae. Genet. Resour. Crop. Evol. 56, 691–703. doi: 10.1007/s10722-008-9394-y
Lu, Y. H., Wu, K. M., Wyckhuys, K. A. G., and Guo, Y. Y. (2009). Potential of mungbean, Vigna radiata as a trap crop for managing Apolygus lucorum (Hemiptera: Miridae) on Bt cotton. Crop Prot. 28, 77–81. doi: 10.1016/j.cropro.2008.08.018
Lucrèce, K. M., Judith, A. F., Huguette, A. B., Fidèle, A. M., Pierre, D. H., and Djimon, G. J. (2021). Vigna comosa Baker aerial Part’s extracts effects on hyperglycemia and hepatic Glucose’s liberation. Asian J. Pharm. Res. nd Dev. 9, 11–17. doi: 10.22270/ajprd.v9i2.965
Magos Brehm, J., Gaisberger, H., Kell, S., Parra-Quijano, M., Thormann, I., Dulloo, M. E., et al. (2022). Planning complementary conservation of crop wild relative diversity in southern Africa. Divers. Distrib. 28, 1358–1372. doi: 10.1111/ddi.13512
Magos Brehm, J., Kell, S., Thormann, I., Gaisberger, H., Dulloo, M. E., and Maxted, N. (2019). New tools for crop wild relative conservation planning. Plant Genet. Res. Characterisation Utilisation 17, 208–212. doi: 10.1017/S1479262118000527
Magos Brehm, J., Maxted, N., Ford-Lloyd, B. V., and Martins-Loução, M. A. (2008). National inventories of crop wild relatives and wild harvested plants: case-study for Portugal. Genet. Resour. Crop. Evol. 55, 779–796. doi: 10.1007/s10722-007-9283-9
Makaza, W., En-nahli, Y., and Amri, M. (2023). Harnessing plant resistance against Striga spp. parasitism in major cereal crops for enhanced crop production and food security in sub-Saharan Africa: a review. Food Secur. 15, 1127–1149. doi: 10.1007/s12571-023-01345-9
Mammadov, J., Buyyarapu, R., Guttikonda, S. K., Parliament, K., Abdurakhmonov, I. Y., and Kumpatla, S. P. (2018). Wild relatives of maize, rice, cotton, and soybean: treasure troves for tolerance to biotic and abiotic stresses. Front. Plant Sci. 9:886. doi: 10.3389/fpls.2018.00886
Manda, L., Idohou, R., Assogbadjo, A. E., and Agbangla, C. (2022). Climate change reveals contractions and expansions in the distribution of suitable habitats for the neglected crop wild relatives of the genus Vigna (Savi) in Benin. Front. Conserv. Sci. 3:870041. doi: 10.3389/fcosc.2022.870041
Manda, L., Salako, K. V., Kataya, A., Affossogbe, S. A. T., Njera, D., Mgoola, W. O., et al. (2023). Co-management brings hope for effective biodiversity conservation and socio-economic development in Vwaza Marsh wildlife Reserve in Malawi. Front. Conserv. Sci. 4:1124142. doi: 10.3389/fcosc.2023.1124142
Mandizvo, T., and Odindo, A. O. (2019). Seed mineral reserves and vigour of Bambara groundnut (Vigna subterranea L.) landraces differing in seed coat colour. Heliyon 5:e01635. doi: 10.1016/j.heliyon.2019.e01635
Mandumbu, R., Mutengwa, C., Mabasa, S., and Mwenje, E. (2019). Challenges to the exploitation of host plant resistance for Striga management in cereals and legumes by farmers in sub-Saharan Africa: a review. Acta Agric. Scand. Sect. B 69, 82–88. doi: 10.1080/09064710.2018.1494302
Masteling, R., Lombard, L., de Boer, W., Raaijmakers, J. M., and Dini-Andreote, F. (2019). Harnessing the microbiome to control plant parasitic weeds. Curr. Opin. Microbiol. 49, 26–33. doi: 10.1016/j.mib.2019.09.006
Maxted, N., Amri, A., Castañeda-Álvarez, N. P., Dias, S., Dulloo, M. E., Fielder, H., et al. (2016). “Joining up the dots: a systematic perspective of crop wild relative conservation and use” in Enhancing crop gene pool use: Capturing wild relative and landrace diversity for crop improvement. eds. N. Maxted, M. E. Dulloo, and B. V. Ford-Lloyd (Wallingford: CABI), 87–124.
Maxted, N., Ford-Lloyd, B. V., Jury, S., Kell, S., and Scholten, M. (2006). Towards a definition of a crop wild relative. Biodivers. Conserv. 15, 2673–2685. doi: 10.1007/s10531-005-5409-6
Maxted, N., Iriondo, J. M., De Hond, L., Dulloo, M. E., Lefèvre, F., Asdal, A., et al. (2008a). “Genetic reserve management” in Conserving plant genetic diversity in protected areas: Population Management of Crop Wild Relatives. eds. J. M. Iriondo, N. Maxted, and M. E. Dulloo (Wallingford: CABI Publishing), 65–87.
Maxted, N., Iriondo, J. M., Dulloo, M. E., and Lane, A. (2008b). “Introduction: the integration of pgr conservation with protected area management” in Conserving plant genetic diversity in protected areas: Population Management of Crop Wild Relatives (pp. 1–22). eds. J. M. Iriondo, N. Maxted, and M. E. Dulloo (Wallingford: CABI), 1–22.
Maxted, N., and Kell, S. (2009). Establishment of a global network for the in situ conservation of crop wild relatives: status and needs. Rome: FAO Commission of Plant Genetic Resources and Agriculture.
Maxted, N., Kell, S., Ford-Lloyd, B., Dulloo, E., and Toledo, Á. (2012). Toward the systematic conservation of global crop wild relative diversity. Crop Sci. 52, 774–785. doi: 10.2135/cropsci2011.08.0415
Maxted, N., Kell, S., Toledo, Á., Dulloo, E., Heywood, V., Hodgkin, T., et al. (2010). A global approach to crop wild relative conservation: securing the gene pool for food and agriculture. Kew Bull. 65, 561–576. doi: 10.1007/s12225-011-9253-4
Maxted, N., Mabuza-Diamini, P., Moss, H., Padulosi, S., Jarvis, A., and Guarino, L. (2004). An ecogeographic study: African Vigna. Rome: International Plant Genetic Resources Centre.
Maxted, N., and Magos Brehm, J. (2023). Maximizing the crop wild relative resources available to plant breeders for crop improvement. Front. Sust. Food Syst. 7:1010204. doi: 10.3389/fsufs.2023.1010204
Maxted, N., and McFarlane, D. (2019). Vigna desmodioides. The IUCN Red List of Threatened Species 2019: e.T170431A1313658. Available at: http://dx.doi.org/10.2305/IUCN.UK.2019-3.RLTS.T170431A1313658.en. IUCN, Gland, Switzerland (Accessed December 23, 2024).
Maxted, N., Scholten, M., Codd, R., and Ford-Lloyd, B. (2007). Creation and use of a national inventory of crop wild relatives. Biol. Conserv. 140, 142–159. doi: 10.1016/j.biocon.2007.08.006
Mbuvi, D. A., Masiga, C. W., Kuria, E., Masanga, J., Wamalwa, M., Mohamed, A., et al. (2017). Novel sources of witchweed (Striga) resistance from wild sorghum accessions. Front. Plant Sci. 8:116. doi: 10.3389/fpls.2017.00116
McCouch, S., Baute, G. J., Bradeen, J., Bramel, P., Bretting, P. K., Buckler, E., et al. (2013). Agriculture: feeding the future. Nature 499, 23–24. doi: 10.1038/499023a
McFarlane, D., and Maxted, N. (2019a). Vigna bosseri. The IUCN Red List of Threatened Species 2019: e.T170429A1313646. Available at: http://dx.doi.org/10.2305/IUCN.UK.2019-3.RLTS.T170429A1313646.en. IUCN, Gland, Switzerland (Accessed December 23, 2024).
McFarlane, D., and Maxted, N. (2019b). Vigna dolomitica. The IUCN Red List of Threatened Species 2019: e.T170432A1313664. Available at: http://dx.doi.org/10.2305/IUCN.UK.2019-3.RLTS.T170432A1313664.en. IUCN, Gland, Switzerland (Accessed December 23, 2024).
McFarlane, D., and Maxted, N. (2019c). Vigna laurentii. The IUCN Red List of Threatened Species 2019: e.T170447A1314016. Available at: http://dx.doi.org/10.2305/IUCN.UK.2019-3.RLTS.T170447A1314016.en. IUCN, Gland, Switzerland (Accessed December 23, 2024).
Mehrabi, Z., Ellis, E. C., and Ramankutty, N. (2018). The challenge of feeding the world while conserving half the planet. Nat. Sust. 1, 409–412. doi: 10.1038/s41893-018-0119-8
Meilleur, B. A., and Hodgkin, T. (2004). In situ conservation of crop wild relatives: status and trends. Biodivers. Conserv. 13, 663–684. doi: 10.1023/B:BIOC.0000011719.03230.17
Moray, C., Game, E. T., and Maxted, N. (2014). Prioritising in situ conservation of crop resources: A case study of African cowpea (Vigna unguiculata). Sci. Rep. 4:5247. doi: 10.1038/srep05247
Muchero, W., Roberts, P. A., Diop, N. N., Drabo, I., Cisse, N., Close, T. J., et al. (2013). Genetic architecture of delayed senescence, biomass, and grain yield under drought stress in cowpea. PLoS One 8:e70041. doi: 10.1371/journal.pone.0070041
Muñoz, N., Liu, A., Kan, L., Li, M. W., and Lam, H. M. (2017). Potential uses of wild germplasms of grain legumes for crop improvement. Int. J. Mol. Sci. 18:328. doi: 10.3390/ijms18020328
Mutyasira, V. (2023). Transforming Africa’s food systems: a smallholder farmers’ perspective. Global Soc. Challenges J. 2, 51–63. doi: 10.1332/lpzj2396
Mwale, S. E., Ochwo-Ssemakula, M., Sadik, K., Achola, E., Okul, V., Gibson, P., et al. (2017). Response of cowpea genotypes to drought stress in Uganda. Am. J. Plant Sci. 8, 720–733. doi: 10.4236/ajps.2017.84050
Mwangangi, I. M., Büchi, L., Haefele, S. M., Bastiaans, L., Runo, S., and Rodenburg, J. (2021). Combining host plant defence with targeted nutrition: key to durable control of hemiparasitic Striga in cereals in sub-Saharan Africa? New Phytol. 230, 2164–2178. doi: 10.1111/nph.17271
Myers, S. S., Smith, M. R., Guth, S., Golden, C. D., Vaitla, B., Mueller, N. D., et al. (2017). Climate change and global food systems: potential impacts on food security and undernutrition. Annu. Rev. Public Health 38, 259–277. doi: 10.1146/annurev-publhealth-031816-044356
Nadeem, M., Li, J., Yahya, M., Sher, A., Ma, C., Wang, X., et al. (2019). Research Progress and perspective on drought stress in legumes: a review. Int. J. Mol. Sci. 20:2541. doi: 10.3390/ijms20102541
Nair, R. M., Pujar, M., Cockel, C., Scheldeman, X., Vandelook, F., van Zonneveld, M., et al. (2023). Global strategy for the conservation and use of Vigna. Bonn: Global Crop Diversity Trust.
Nduche, M., Magos Brehm, J., Abberton, M., Omosun, G., and Maxted, N. (2021). West African crop wild relative checklist, prioritization and inventory. Gent. Resources 2, 55–65. doi: 10.46265/genresj.eifl1323
Nduche, M. U., Magos Brehm, J., Parra Quijano, M., and Maxted, N. (2022). In situ and ex situ conservation gap analyses of West African priority crop wild relatives. Genet. Resour. Crop. Evol. 70, 333–351. doi: 10.1007/s10722-022-01507-2
Nkomo, G. V., Sedibe, M. M., and Mofokeng, M. A. (2021). Production constraints and improvement strategies of cowpea (Vigna unguiculata L. Walp.) genotypes for drought tolerance. Int. J. Agron. 2021, 1–9. doi: 10.1155/2021/5536417
Nzioki, H. S., Oyosi, F., Morris, C. E., Kaya, E., Pilgeram, A. L., Baker, C. S., et al. (2016). Striga biocontrol on a toothpick: A readily deployable and inexpensive method for smallholder farmers. Front. Plant Sci. 7, 1–8. doi: 10.3389/fpls.2016.01121
Ogiso-Tanaka, E., Chankaew, S., Yoshida, Y., Isemura, T., Marubodee, R., Kongjaimun, A., et al. (2023). Unique salt-tolerance-related QTLs, evolved in Vigna riukiuensis (Na(+) Includer) and V. Nakashimae (Na(+) excluder), shed light on the development of super-salt-tolerant Azuki bean (V. angularis) cultivars. Cultivars. Plants 12:680. doi: 10.3390/plants12081680
Ogundeji, A. A., and Okolie, C. C. (2022). Perception and adaptation strategies of smallholder farmers to drought risk: a scientometric analysis. Agriculture 12:1129. doi: 10.3390/agriculture12081129
Ogunkanmi, A. L., Ogundipe, O. T., Omoigui, L. O., Odeseye, A. O., and Fatokun, C. A. (2019). Morphological and SSR marker characterization of wild and cultivated cowpeas (Vigna unguiculata L Walp). J. Agric. Sci. 64, 367–380. doi: 10.2298/JAS1904367O
Oguz, M. C., Aycan, M., Oguz, E., Poyraz, I., and Yildiz, M. (2022). Drought stress tolerance in plants: interplay of molecular, biochemical and physiological responses in important development stages. Physiologia 2, 180–197. doi: 10.3390/physiologia2040015
Ohlson, E. W., and Timko, M. P. (2020). Race structure of cowpea witchweed (Striga gesnerioides) in West Africa and its implications for Striga resistance breeding of cowpea. Weed Sci. 68, 125–133. doi: 10.1017/wsc.2020.3
Omoigui, L. O., Kamara, A. Y., Alunyo, G. I., Bello, L. L., Oluoch, M., Timko, M. P., et al. (2017). Identification of new sources of resistance to Striga gesnerioides in cowpea Vigna unguiculata accessions. Genet. Resour. Crop. Evol. 64, 901–911. doi: 10.1007/s10722-016-0410-3
Omoigui, L. O., Kamara, A. Y., Moukoumbi, Y. D., Ogunkanmi, L. A., and Timko, M. P. (2017). Breeding cowpea for resistance to Striga gesnerioides in the Nigerian dry savannas using marker-assisted selection. Plant Breed. 136, 393–399. doi: 10.1111/pbr.12475
Østerberg, J. T., Xiang, W., Olsen, L. I., Edenbrandt, A. K., Vedel, S. E., Christiansen, A., et al. (2017). Accelerating the domestication of new crops: feasibility and approaches. Trends Plant Sci. 22, 373–384. doi: 10.1016/j.tplants.2017.01.004
Oswald, A., and Ransom, J. K. (2004). Response of maize varieties to Striga infestation. Crop Prot. 23, 89–94. doi: 10.1016/S0261-2194(03)00173-X
Oyatomi, O., Fatokun, C., Boukar, O., Abberton, M., and Ilori, C. (2016). “Screening wild Vigna species and cowpea (Vigna unguiculata) landraces for sources of resistance to Striga gesnerioides” in Enhancing crop gene pool use: Capturing wild relative and landrace diversity for crop improvement. eds. S. Dias and M. E. Dulloo (Wallingford: CABI), 27–31.
Padulosi, S., Heywood, V., Hunter, D., and Jarvis, A. (2011). “Underutilized species and climate change: current status and outlook” in Crop adaptation to climate change. eds. S. S. Yadav, R. J. Redden, J. L. Hatfield, H. Lotze-Campen, and A. E. Hall (New York, NY: Wiley-Blackwell), 507–521.
Parker, C. (2012). Parasitic weeds: a world challenge. Weed Sci. 60, 269–276. doi: 10.1614/ws-d-11-00068.1
Parker, C. (2014). The continuing threat from parasitic weeds. Outlooks Pest Manage. 25, 237–242. doi: 10.1564/v25_jun_11
Parker, C. (2021). A personal history in parasitic weeds and their control. Plan. Theory 10:249. doi: 10.3390/plants10112249
Pawera, L., Łuczaj, Ł., Pieroni, A., and Polesny, Z. (2017). Traditional plant knowledge in the white Carpathians: ethnobotany of wild food plants and crop wild relatives in the Czech Republic. Hum. Ecol. 45, 655–671. doi: 10.1007/s10745-017-9938-x
Petitpierre, B., Boserup, J., Möhl, A., Rometsch, S., and Aubry, S. (2023). Importance of agriculture for crop wild relatives conservation in Switzerland. Global Ecol. Conserv. 46:e02588. doi: 10.1016/j.gecco.2023.e02588
Pironon, S., Etherington, T. R., Borrell, J. S., Kühn, N., Macias-Fauria, M., Ondo, I., et al. (2019). Potential adaptive strategies for 29 sub-Saharan crops under future climate change. Nat. Clim. Chang. 9, 758–763. doi: 10.1038/s41558-019-0585-7
Pootakham, W., Sonthirod, C., Naktang, C., Yundaeng, C., Yoocha, T., Kongkachana, W., et al. (2022). Genome assemblies of Vigna reflexo-pilosa (Créole bean) and its progenitors, Vigna hirtella and Vigna trinervia, revealed homoeolog expression bias and expression-level dominance in the allotetraploid. GigaScience 12:giad050. doi: 10.1093/gigascience/giad050
Popoola, J. O., Aremu, B. R., Daramola, F. Y., Ejoh, A. S., and Adegbite, A. E. (2015). Morphometric analysis of some species in the genus Vigna (L.) Walp: implication for utilization for genetic improvement. J. Biol. Sci. 15, 156–166. doi: 10.3923/jbs.2015.156.166
Porch, T., Beaver, J., Debouck, D., Jackson, S., Kelly, J., and Dempewolf, H. (2013). Use of wild relatives and closely related species to adapt common bean to climate change. Agronomy 3, 433–461. doi: 10.3390/agronomy3020433
Powell, B., Thilsted, S. H., Ickowitz, A., Termote, C., Sunderland, T., and Herforth, A. (2015). Improving diets with wild and cultivated biodiversity from across the landscape. Food Secur. 7, 535–554. doi: 10.1007/s12571-015-0466-5
Pratap, A., Malviya, N., Gupta, S., Tomar, R., Pandey, V. R., and Prajapati, U. (2018). Field characterization of endemic wild Vigna accessions collected from biodiversity hotspots of India to identify promising genotypes for multiple agronomic and adaptive traits. Legum. Res. 4723, 1–10. doi: 10.18805/A-4723
PricewaterhouseCoopers. (2013). Crop wild relatives: A valuable resource for crop development. PricewaterhouseCooper. Available at: https://pwc.blogs.com/files/pwc-seed-bank-analysis-for-msb-0713.pdf (Accessed April 24, 2019).
Rahimi, M., Houshmand, S., Khodambashi, M., Shiran, B., and Mohammady, S. (2016). Effect of drought stress on agro-morphological traits of lentil (Lens culinaris Medik.) recombinant inbred lines. J. Agric. Res. 41, 207–219. doi: 10.3329/bjar.v41i2.28216
Ramatsetse, K. E., Ramashia, E. S., and Mashau, M. E. (2023). A review on health benefits, antimicrobial and antioxidant properties of Bambara groundnut (Vigna subterranean). Int. J. Food Prop. 26, 91–107. doi: 10.1080/10942912.2022.2153864
Rao, I. M., Beebe, S. E., and Polania, J. A. (2013). Can Tepary bean be a model for improvement of drought resistance in common bean. Afr. Crop. Sci. J. 21:v23i4. doi: 10.4314/acsj.v21i4
Ratnayake, S. S., Kariyawasam, C. S., Kumar, L., Hunter, D., and Liyanage, A. S. U. (2021). Potential distribution of crop wild relatives under climate change in Sri Lanka: implications for conservation of agricultural biodiversity. Curr. Res. Environ. Sust. 3:100092. doi: 10.1016/j.crsust.2021.100092
Reyes, J. A. O., Delfin, E. F., Reyes, M. E. C., and Ocampo, E. T. M. (2022). Physiological and yield responses of selected Mungbean genotypes to terminal drought. Philippine J. Sci. 151, 739–750. doi: 10.56899/151.02.16
Rhodes, L. (2016a). Vigna keraudrenii. The IUCN Red List of Threatened Species 2016: e.T170444A1313998. Available at: http://dx.doi.org/10.2305/IUCN.UK.2016-3.RLTS.T170444A1313998.en IUCN, Gland, Switzerland (Accessed December 23, 2024).
Rhodes, L. (2016b). Vigna monantha. The IUCN Red List of Threatened Species 2016: e.T170444A1313998. Available at: http://dx.doi.org/10.2305/IUCN.UK.2016-3.RLTS.T170444A1313998.en. IUCN, Gland, Switzerland (Accessed December 23, 2024).
Riordan, E. C., and Nabhan, G. P. (2019). Trans situ conservation of crop wild relatives. Crop Sci. 59, 2387–2403. doi: 10.2135/cropsci2019.06.0356
Ronald, M., Charles, M., Stanford, M., and Eddie, M. (2017). Predictions of the Striga scourge under new climate in southern Africa: a perspective. J. Biol. Sci. 17, 194–201. doi: 10.3923/jbs.2017.194.201
Ross-Ibarra, J., Morrell, P. L., and Gaut, B. S. (2007). Plant domestication, a unique opportunity to identify the genetic basis of adaptation. PNAS 104, 8641–8648. doi: 10.1073/pnas.0700643104
Ruben, R., Cavatassi, R., Lipper, L., Smaling, E., and Winters, P. (2021). Towards food systems transformation—five paradigm shifts for healthy, inclusive and sustainable food systems. Food Secur. 13, 1423–1430. doi: 10.1007/s12571-021-01221-4
Rubiales, D. (2003). Parasitic plants, wild relatives and the nature of resistance. New Phytol. 160, 459–461. doi: 10.1046/j.1469-8137.2003.00929.x
Rubiales, D. (2023). Managing root parasitic weeds to facilitate legume reintroduction into Mediterranean rain-fed farming systems. Soil Systems 7:99. doi: 10.3390/soilsystems7040099
Rubiales, D., Fernández-Aparicio, M., Vurro, M., and Eizenberg, H. (2018). Editorial: advances in parasitic weed research. Front. Plant Sci. 9, 7–9. doi: 10.3389/fpls.2018.00236
Rubiales, D., Pérez-de-Luque, A., Fernández-Aparico, M., Sillero, J. C., Román, B., Kharrat, M., et al. (2006). Screening techniques and sources of resistance against parasitic weeds in grain legumes. Euphytica 147, 187–199. doi: 10.1007/s10681-006-7399-1
Rubio Teso, M.L., Álvarez Muñiz, C., Gaisberger, H., Kell, S., Lara-Romero, C., and Magos Brehm, J. (2020). In situ plant genetic resources in Europe: crop wild relatives. Available at: https://more.bham.ac.uk/farmerspride/wp-content/uploads/sites/19/2020/10/D1.2_In_situ_PGR_in_Europe_crop_wild_relatives.pdf (Accessed October 19, 2020).
Rubio Teso, M. L., Lara-Romero, C., Rubiales, D., Parra-Quijano, M., and Iriondo, J. M. (2022). Searching for abiotic tolerant and biotic stress resistant wild lentils for introgression breeding through predictive characterization. Front. Plant Sci. 13:817849. doi: 10.3389/fpls.2022.817849
Runo, S., and Kuria, E. K. (2018). Habits of a highly successful cereal killer, Striga. PLoS Pathogens 14:e1006731. doi: 10.1371/journal.ppat.1006731
Safari, J. G., Nkua, A. J., and Masanyiwa, Z. S. (2021). Household food security among Hadza hunter-gatherers in Mkalama district, Tanzania. Agric. Food Secur. 10, 1–13. doi: 10.1186/s40066-021-00293-x
Saha, D., Choyal, P., Mishra, U. N., Dey, P., Bose, B., Gupta, N. K., et al. (2022). Drought stress responses and inducing tolerance by seed priming approach in plants. Plant Stress 4:100066. doi: 10.1016/j.stress.2022.100066
Salako, V. K., Fandohan, B., Kassa, B., Assogbadjo, A. E., Idohou, A. F. R., Gbedomon, R. C., et al. (2014). Home gardens: an assessment of their biodiversity and potential contribution to conservation of threatened species and crop wild relatives in Benin. Genet. Resour. Crop. Evol. 61, 313–330. doi: 10.1007/s10722-013-0035-8
Salifou, M., Tignegre, J. B. L. S., Tongoona, P., Offei, S., Ofori, K., and Danquah, E. (2017). Differential responses of 15 cowpea genotypes to three Striga hot spots in Niger. Int. J. Biol. Chem. Sci. 11:1413. doi: 10.4314/ijbcs.v11i4.2
Sallam, A., Alqudah, A. M., Dawood, M. F. A., Baenziger, P. S., and Börner, A. (2019). Drought stress tolerance in wheat and barley: advances in physiology, breeding and genetics research. Int. J. Mol. Sci. 20:137. doi: 10.3390/ijms20133137
Samejima, H., and Sugimoto, Y. (2018). Recent research progress in combatting root parasitic weeds. Biotechnol. Biotechnol. Equip. 32, 221–240. doi: 10.1080/13102818.2017.1420427
Satori, D., Hammond, E., Gemma, H., Christopher, M., Nicola, C., Leitch, I. J., et al. (2021). Prioritising crop wild relatives to enhance agricultural resilience in sub-Saharan Africa under climate change. Plants, People, Planet 4, 269–282. doi: 10.1002/ppp3.10247
Sawadogo, P., Ouedraogo, T. J., Dieni, Z., Batieno, T. B. J., Sawadogo, N., Poda, S. L., et al. (2021a). Differential and comparative screening of cowpea varieties to Striga gesnerioides (Willd.) Vatke for race specific identification in Burkina Faso. Afr. Crop. Sci. J. 29, 101–118. doi: 10.4314/acsj.v29i1.7
Sawadogo, P., Sawadogo, N., Ouedraogo, T. J., Dieni, Z., Batieno, T. B. J., Zongo, H., et al. (2021b). Indigenous knowledge of Striga gesnerioides (Willd.) Vatke, in Burkina Faso. Afr. J. Agric. Res. 17, 57–65. doi: 10.5897/AJAR2020.15159
Sawadogo, T. C., Yonli, D., Sourabie, S., Zerbo, P., Traoré, H., and Boussim, J. I. (2022). Aqueous extracts from indigenous plant in Burkina Faso with bio-herbicide properties to reduce Striga hermonthica (Del.) Benth propagation. CABI Agric. Biosci. 3:129. doi: 10.1186/s43170-022-00129-z
Scholes, J. D., and Press, M. C. (2008). Striga infestation of cereal crops - an unsolved problem in resource limited agriculture. Curr. Opin. Plant Biol. 11, 180–186. doi: 10.1016/j.pbi.2008.02.004
Scott, D., and Freckleton, R. P. (2022). Crop diversification and parasitic weed abundance: a global meta-analysis. Sci. Rep. 12:19413. doi: 10.1038/s41598-022-24047-2
Seleiman, M. F., Al-Suhaibani, N., Ali, N., Akmal, M., Alotaibi, M., Refay, Y., et al. (2021). Drought stress impacts on plants and different approaches to alleviate its adverse effects. Plan. Theory 10, 1–25. doi: 10.3390/plants10020259
Senapati, N., Stratonovitch, P., Paul, M. J., and Semenov, M. A. (2019). Drought tolerance during reproductive development is important for increasing wheat yield potential under climate change in Europe. J. Exp. Bot. 70, 2549–2560. doi: 10.1093/jxb/ery226
Shigaeva, J., Darr, D., Sulaiman, N., and Polesny, Z. (2019). Ethnobotany of Wild Plants and Crop Wild Relatives in the Walnut-Fruit Forests of Kyrgyzstan. Tropentag 2019: Filling gaps and removing traps for sustainable resources management. Available at: https://www.researchgate.net/publication/335972104_Ethnobotany_of_Wild_Plants_and_Crop_Wild_Relatives_in_the_Walnut-Fruit_Forests_of_Kyrgyzstan (Accessed September 18, 2019).
Showler, A. T. (2013). Plant-arthropod interactions affected by water deficit stress through association with changes in plant free amino acid accumulations. Molecular Approaches in Plant Abiotic Stress eds. Gaur, B. R. K. and Sharma, P. (Boca Raton, Florida: CRC Press), 299–352.
Singh, A., Dubey, P. K., Chaurasia, R., Dubey, R. K., Pandey, K. K., Singh, G. S., et al. (2019). Domesticating the undomesticated for global food and nutritional security: four steps. Agronomy 9:491. doi: 10.3390/agronomy9090491
Smýkal, P., Nelson, M. N., Berger, J. D., and Von Wettberg, E. J. B. (2018). The impact of genetic changes during crop domestication. Agronomy 8:119. doi: 10.3390/agronomy8070119
Solberg, S. Ø., Brodal, G., von Bothmer, R., Meen, E., Yndgaard, F., Andreasen, C., et al. (2020). Seed germination after 30 years storage in permafrost. Plan. Theory 9, 1–9. doi: 10.3390/plants9050579
Solberg, S. O., Yndgaard, F., and Palmè, A. (2017). Morphological and phenological consequences of ex situ conservation of natural populations of red clover (Trifolium pratense L.). Plant Genetic Res. Characterisation Utilisation 15, 97–108. doi: 10.1017/S1479262115000416
Somta, P., Dachapak, S., Yimram, T., Srinives, P., and Poonchaivilaisak, S. (2019). Genetic diversity of zombi pea (Vigna vexillata) assessed by microsatellite markers. Acta Hortic. 1241, 143–150. doi: 10.17660/ActaHortic.2019.1241.21
Spallek, T., Mutuku, M., and Shirasu, K. (2013). The genus Striga: a witch profile. Mol. Plant Pathol. 14, 861–869. doi: 10.1111/mpp.12058
Springmann, M., Clark, M., Mason-D’Croz, D., Wiebe, K., Bodirsky, B. L., Lassaletta, L., et al. (2018). Options for keeping the food system within environmental limits. Nature 562, 519–525. doi: 10.1038/s41586-018-0594-0
Steiner, A., Aguilar, G., Bomba, K., Bonilla, J. P., Campbell, A., Echeverria, R., et al. (2020). Actions to transform food systems under climate change. Wageningen: CGIAR Research Program on Climate Change, Agriculture and Food Security (CCAFS).
Stolton, S., Maxted, N., Ford-Lloyd, B., Kell, S., and Dudley, N. (2006). Food stores. Using Protected Areas to secure Crop Genetic Diversity. In Siteresources. New York, NY: Worldbank.
Su, C., Liu, H., Wafula, E. K., Honaas, L., de Pamphilis, C. W., and Timko, M. P. (2020). SHR4z, a novel decoy effector from the haustorium of the parasitic weed Striga gesnerioides, suppresses host plant immunity. New Phytol. 226, 891–908. doi: 10.1111/nph.16351
Sulaiman, N., Aziz, M. A., Stryamets, N., Mattalia, G., Zocchi, D. M., Ahmed, H. M., et al. (2023). The importance of becoming tamed: wild food plants as possible novel crops in selected food-insecure regions. Horticulturae 9:171. doi: 10.3390/horticulturae9020171
Swanton, C. J., Nkoa, R., and Blackshaw, R. E. (2015). Experimental methods for crop–weed competition studies. Weed Sci. 63, 2–11. doi: 10.1614/ws-d-13-00062.1
Syfert, M. M., Castañeda-Álvarez, N. P., Khoury, C. K., Särkinen, T., Sosa, C. C., Achicanoy, H. A., et al. (2016). Crop wild relatives of the brinjal eggplant (Solanum melongena): poorly represented in genebanks and many species at risk of extinction. Am. J. Bot. 103, 635–651. doi: 10.3732/ajb.1500539
Tadele, Z. (2019). Orphan crops: their importance and the urgency of improvement. Planta 250, 677–694. doi: 10.1007/s00425-019-03210-6
Takahashi, Y., Muto, C., Iseki, K., Naito, K., Somta, P., Pandiyan, M., et al. (2018). A new taxonomic treatment for some wild relatives of mungbean (Vigna radiata (L.) Wilcz.) based on their molecular phylogenetic relationships and morphological variations. Genet. Resour. Crop. Evol. 65, 1109–1121. doi: 10.1007/s10722-017-0599-9
Takahashi, Y., Sakai, H., Yoshitsu, Y., Muto, C., Anai, T., Pandiyan, M., et al. (2019). Domesticating Vigna stipulacea: a potential legume crop with broad resistance to biotic stresses. Front. Plant Sci. 10:1607. doi: 10.3389/fpls.2019.01607
Takahashi, Y., Somta, P., Muto, C., Iseki, K., Naito, K., Pandiyan, M., et al. (2016). Novel genetic resources in the genus Vigna unveiled from gene bank accessions. PLoS One 11, 1–18. doi: 10.1371/journal.pone.0147568
Takahashi, Y., and Tomooka, N. (2020). “Taxonomy of Mungbean and its relatives” in The Mungbean genome. eds. R. M. Nair, R. Schafleitner, and S.-H. Lee (Cham: Springer International Publishing), 27–41.
Tejada-Alvarado, J. J., Meléndez-Mori, J. B., Vilca-Valqui, N. C., Neri, J. C., Ayala-Tocto, R. Y., Huaman-Huaman, E., et al. (2023). Impact of wild Solanaceae rootstocks on morphological and physiological response, yield, and fruit quality of tomato (Solanum lycopersicum L.) grown under deficit irrigation conditions. Heliyon 9:e12755. doi: 10.1016/j.heliyon.2022.e12755
Teka, H. B. (2014). Advance research on Striga control: a review. African J. Plant Sci. 8, 492–506. doi: 10.5897/AJPS2014.1186
Těšitel, J. (2016). Functional biology of parasitic plants: a review. Plant Ecol. Evol. 149, 5–20. doi: 10.5091/plecevo.2016.1097
Thomas, C. D., and Gillingham, P. K. (2015). The performance of protected areas for biodiversity under climate change. Biol. J. Linn. Soc. 115, 718–730. doi: 10.1111/bij.12510
Tignegre, J. B. S., Ouedraogo, J. T., Melis, R., Tongoona, P., Sibiya, J., Makanda, I., et al. (2013). Identification of new sources of resistance to Striga gesnerioides in cowpea germplasm. Plant Breed. 132, 330–336. doi: 10.1111/pbr.12055
Tilman, D., Balzer, C., Hill, J., and Befort, B. L. (2011). Global food demand and the sustainable intensification of agriculture. Proc. Natl. Acad. Sci. USA 108, 20260–20264. doi: 10.1073/pnas.1116437108
Tippe, D. E., Rodenburg, J., Schut, M., van Ast, A., Kayeke, J., and Bastiaans, L. (2017). Farmers’ knowledge, use and preferences of parasitic weed management strategies in rain-fed rice production systems. Crop Prot. 99, 93–107. doi: 10.1016/j.cropro.2017.05.007
Tirnaz, S., Zandberg, J., Thomas, W. J. W., Marsh, J., Edwards, D., and Batley, J. (2022). Application of crop wild relatives in modern breeding: an overview of resources, experimental and computational methodologies. Front. Plant Sci. 13:1008904. doi: 10.3389/fpls.2022.1008904
Tomasini, S., and Theilade, I. (2019). Local ecological knowledge indicators for wild plant management: autonomous local monitoring in Prespa, Albania. Ecol. Indic. 101, 1064–1076. doi: 10.1016/j.ecolind.2019.01.076
Tomooka, N., Kaga, A., Isemura, T., and Vaughan, D. (2011). “Vigna” in Wild crop relatives: Genomic and breeding resources: Legume crops and forages. ed. C. Kole (Berlin Heidelberg: Springer), 291–311.
Tomooka, N., Naito, K., Kaga, A., Sakai, H., Isemura, T., Ogiso-Tanaka, E., et al. (2014). Evolution, domestication and neo-domestication of the genus Vigna. Plant Genetic Res. Characterisation Utilisation 12, S168–S171. doi: 10.1017/S1479262114000483
Tomooka, N., Vaughan, D. A., Moss, H., and Maxted, N. (2002). The Asian Vigna: genus Vigna subgenus Ceratotropis genetic resources. Berlin: Springer.
Trenberth, K. E., Dai, A., Van Der Schrier, G., Jones, P. D., Barichivich, J., Briffa, K. R., et al. (2014). Global warming and changes in drought. Nat. Clim. Chang. 4, 17–22. doi: 10.1038/nclimate2067
Tripathi, K., Gore, P. G., Pandey, A., Nayar, E. R., Gayacharan, C., Pamarthi, R. K., et al. (2021). Morphological and nutritional assessment of Vigna vexillata (L.) A. Rich.: a potential tuberous legume of India. Genet. Resour. Crop. Evol. 68, 397–408. doi: 10.1007/s10722-020-01023-1
Tyack, N., Dempewolf, H., and Khoury, C. K. (2020). The potential of payment for ecosystem services for crop wild relative conservation. Plan. Theory 9, 1–14. doi: 10.3390/plants9101305
Ullah, A., and Farooq, M. (2021). The challenge of drought stress for grain legumes and options for improvement. Arch. Agron. Soil Sci. 68, 1601–1618. doi: 10.1080/03650340.2021.1906413
Umdale, S. D., Gaikwad, N. B., Kadam, S., Ahire, M. L., Mundada, P. S., and Bhat, K. V. (2023). Genetic diversity of Asian Vigna species (subgenus Ceratotropis; genus Vigna) in India based on ITS2 sequences data. Plant Mol. Biol. Report. 41, 454–469. doi: 10.1007/s11105-023-01377-7
United Nations (2015). Transforming our world: the 2030 agenda for sustainable development. Cham: Springer Publishing Company.
Van Delft, G. J., Graves, J. D., Fitter, A. H., and Van Ast, A. (2000). Striga seed avoidance by deep planting and no-tillage in sorghum and maize. Int. J. Pest Manag. 46, 251–256. doi: 10.1080/09670870050206019
van Dijk, M., Morley, T., Rau, M. L., and Saghai, Y. (2021). A meta-analysis of projected global food demand and population at risk of hunger for the period 2010–2050. Nat. Food 2, 494–501. doi: 10.1038/s43016-021-00322-9
van Munster, M. (2020). Impact of abiotic stresses on plant virus transmission by aphids. Viruses 12:216. doi: 10.3390/v12020216
van Zonneveld, M., Kindt, R., McMullin, S., Achigan-Dako, E. G., N’Danikou, S., Hsieh, W. H., et al. (2023). Forgotten food crops in sub-Saharan Africa for healthy diets in a changing climate. Proc. Natl. Acad. Sci. USA 120:e2205794120. doi: 10.1073/pnas.2205794120
van Zonneveld, M., Rakha, M., Tan, S., Chou, Y. Y., Chang, C. H., Yen, J. Y., et al. (2020). Mapping patterns of abiotic and biotic stress resilience uncovers conservation gaps and breeding potential of Vigna wild relatives. Sci. Rep. 10:2111. doi: 10.1038/s41598-020-58646-8
Verma, S. K., Singh, C. K., Taunk, J., Gayacharan, C. J., Kalia, S., Dey, N., et al. (2022). Vignette of Vigna domestication: from archives to genomics. Front. Genet. 13:960200. doi: 10.3389/fgene.2022.960200
Vermeulen, S. J., Campbell, B. M., and Ingram, J. S. I. (2012). Climate change and food systems. Ann. Rev. Environ. Res. 37, 195–222. doi: 10.1146/annurev-environ-020411-130608
Vincent, H., Amri, A., Castañeda-Álvarez, N. P., Dempewolf, H., Dulloo, E., Guarino, L., et al. (2019). Modeling of crop wild relative species identifies areas globally for in situ conservation. Commun. Biol. 2:136. doi: 10.1038/s42003-019-0372-z
Vissoh, P. V., Gbèhounou, G., Ahanchédé, A., Kuyper, T. W., and Röling, N. G. (2004). Weeds as agricultural constraint to farmers in Benin: results of a diagnostic study. NJAS - Wageningen J. Life Sci. 52, 305–329. doi: 10.1016/S1573-5214(04)80019-8
von Wettberg, E., Davis, T. M., and Smýkal, P. (2020). Editorial: wild plants as source of new crops. Front. Plant Sci. 11:591554. doi: 10.3389/fpls.2020.591554
Wainwright, W., Drucker, A. G., Maxted, N., Brehm, J. M., Ng’uni, D., and Moran, D. (2019). Estimating in situ conservation costs of Zambian crop wild relatives under alternative conservation goals. Land Use Policy 81, 632–643. doi: 10.1016/j.landusepol.2018.11.033
Walls, S. C. (2018). Coping with constraints: achieving effective conservation with limited resources. Front. Ecol. Evol. 6:24. doi: 10.3389/fevo.2018.00024
Watteyn, C., Reubens, B., Bolaños, J. B. A., Campos, F. S., Silva, A. P., Karremans, A. P., et al. (2023). Cultivation potential of Vanilla crop wild relatives in two contrasting land use systems. Eur. J. Agron. 149:126890. doi: 10.1016/j.eja.2023.126890
Willett, W., Rockström, J., Loken, B., Springmann, M., Lang, T., Vermeulen, S., et al. (2019). Food in the Anthropocene: the EAT–lancet commission on healthy diets from sustainable food systems. Lancet 393, 447–492. doi: 10.1016/S0140-6736(18)31788-4
Wohlin, C. (2014). “Guidelines for snowballing in systematic literature studies and a replication in software engineering,” in Proceedings of the 18th International Conference on Evaluation and Assessment in Software Engineering - EASE ‘14.
Wood, W., Gore, J., Catchot, A., Cook, D., Dodds, D., and Krutz, L. J. (2017). Effect of leaf pubescence on tarnished plant bug (Hemiptera: Miridae) ability to cause damage and yield loss in cotton. J. Cotton Science 21, 122–127. doi: 10.56454/edox4776
Yang, J., Kornet, R., Diedericks, C. F., Yang, Q., Berton-Carabin, C. C., Nikiforidis, C. V., et al. (2022). Rethinking plant protein extraction: albumin—from side stream to an excellent foaming ingredient. Food Struct. 31:100254. doi: 10.1016/j.foostr.2022.100254
Yoshida, S., Cui, S., Ichihashi, Y., and Shirasu, K. (2016). The Haustorium, a specialized invasive organ in parasitic plants. Annu. Rev. Plant Biol. 67, 643–667. doi: 10.1146/annurev-arplant-043015-111702
Zaki, H. E. M., and Radwan, K. S. A. (2022). Response of potato (Solanum tuberosum L.) cultivars to drought stress under in vitro and field conditions. Chemical and biological technologies. Agriculture 9:266. doi: 10.1186/s40538-021-00266-z
Zhang, H., Li, Y., and Zhu, J.-K. (2018). Developing naturally stress-resistant crops for a sustainable agriculture. Nat. Plants 4, 989–996. doi: 10.1038/s41477-018-0309-4
Zhang, H., Mittal, N., Leamy, L. J., Barazani, O., and Song, B. H. (2017). Back into the wild—apply untapped genetic diversity of wild relatives for crop improvement. Evol. Appl. 10, 5–24. doi: 10.1111/eva.12434
Zhang, Y., Song, H., Wang, X., Zhou, X., Zhang, K., Chen, X., et al. (2020). The roles of different types of trichomes in tomato resistance to cold, drought, whiteflies, and botrytis. Agronomy 10:411. doi: 10.3390/agronomy10030411
Zhao, C., Liu, B., Piao, S., Wang, X., Lobell, D. B., Huang, Y., et al. (2017). Temperature increase reduces global yields of major crops in four independent estimates. Proc. Natl. Acad. Sci. USA 114, 9326–9331. doi: 10.1073/pnas.1701762114
Zsögön, A., Cermak, T., Voytas, D., and Peres, L. E. P. (2017). Genome editing as a tool to achieve the crop ideotype and de novo domestication of wild relatives: case study in tomato. Plant Sci. 256, 120–130. doi: 10.1016/j.plantsci.2016.12.012
Keywords: biodiversity, climate-resilient food systems, crop wild relatives, drought stress, ecosystem services, in situ conservation, Striga stress, Zero hunger
Citation: Manda L, Idohou R, Agoyi EE, Agbahoungba S, Salako KV, Agbangla C, Adomou AC and Assogbadjo AE (2025) Progress of in situ conservation and use of crop wild relatives for food security in a changing climate: a case of the underutilised Vigna Savi. Front. Sustain. 6:1453170. doi: 10.3389/frsus.2025.1453170
Received: 22 June 2024; Accepted: 23 January 2025;
Published: 12 February 2025.
Edited by:
Zujun Yang, University of Electronic Science and Technology of China, ChinaReviewed by:
Eric Von Wettberg, University of Vermont, United StatesCopyright © 2025 Manda, Idohou, Agoyi, Agbahoungba, Salako, Agbangla, Adomou and Assogbadjo. This is an open-access article distributed under the terms of the Creative Commons Attribution License (CC BY). The use, distribution or reproduction in other forums is permitted, provided the original author(s) and the copyright owner(s) are credited and that the original publication in this journal is cited, in accordance with accepted academic practice. No use, distribution or reproduction is permitted which does not comply with these terms.
*Correspondence: Leonard Manda, bG1hbmRhOEBnbWFpbC5jb20=
Disclaimer: All claims expressed in this article are solely those of the authors and do not necessarily represent those of their affiliated organizations, or those of the publisher, the editors and the reviewers. Any product that may be evaluated in this article or claim that may be made by its manufacturer is not guaranteed or endorsed by the publisher.
Research integrity at Frontiers
Learn more about the work of our research integrity team to safeguard the quality of each article we publish.