- 1Institute of Plant Breeding and Genetic Resources, Hellenic Agricultural Organization DIMITRA (ELGO-DIMITRA), Thessaloniki, Greece
- 2University Center of International Programmes of Studies, International Hellenic University, Thessaloniki, Greece
- 3Laboratory of Ichthyology & Fisheries, Department of Animal Production, School of Agriculture, Faculty of Agriculture, Forestry & Natural Environment, Aristotle University of Thessaloniki, Thessaloniki, Greece
Introduction: Aquaponics provide multiple benefits due to the simultaneous yield of vegetables and fish, however they are characterized by increased greenhouse gas emissions owing to intensive production system. The most appropriate method for quantifying the environmental effects of these systems is Life Cycle Assessment with which the identification of hotspots and the suggestion of improved production plans can be achieved. The purpose of the present study was to evaluate the environmental impact of a pilot high-tech aquaponic system utilized for the simultaneous production of baby lettuce and rocket as well as rainbow trout, in indicators such as Global Warming Potential.
Materials and methods: To achieve this goal, data on inputs and outputs were collected from 12 case studies that were implemented, combining different fertilizer treatments, substrate choices, plant species cultivated and water source provision. Life Cycle Assessment was performed using SimaPro v.9.4.0.2 software.
Results: The results showcase that the optimal case studies include the cultivation of baby lettuce and rocket in perlite substrate using wastewater from fish and partial use of synthetic fertilizers. Indicatively, Global Warming Potential of these cases was calculated at 21.18 and 40.59 kg CO2-eq/kg of vegetable respectively. The parameter with the greatest impact on most of the environmental indicators was electricity consumption for the operation of the oxygen supply pump for the fish tanks, while greenhouse infrastructure had the greatest impact in Abiotic Depletion and Human Toxicity impact categories. In an alternative production scenario tested where renewable energy sources were used, system impacts were reduced by up to 50% for Global Warming Potential and 86% for Eutrophication impact. The results of this study aspire to constitute a significant milestone in environmental impact assessments of aquaponic production systems and the adoption of more sustainable farming practices.
1 Introduction
The rapid growth of the world population which reached 7.94 billion in 2022 and is predicted to reach 9.69 billion by 2050, intensifies the increase in the feeding needs of people throughout the world (United Nations Department of Economic and Social Affairs, 2022). This noticeable increase in the feeding requirements of the world population must also be accompanied by ensuring food production from sustainable systems, which use fewer or reusable resources, but with higher efficiency (Pawlak and Kołodziejczak, 2020). Various innovative solutions have been sought towards this direction, such as the application of aquaponics, which combines the rearing of fish for consumption and the simultaneous production of vegetables with the utilization of reared fish waste, as fertilizer for crops (Masabni and Niu, 2022). This system adheres with the concept of circular economy, with promising results in terms of reducing inputs such as synthetic fertilizers, which are replaced by nitrates produced by the fish waste through the process of nitrification, with the contribution of nitrifying bacteria and a combination of filters and wastewater processing (Campanati et al., 2022). While these types of systems appear to have a positive effect on the supply chain in terms of environmental measures, there are still gaps in whether they can match or even surpass alternative production systems (Greenfeld et al., 2022).
Aquaponic systems can support a variety of fish species, with rainbow trout (Oncorhynchus mykiss), common carp (Cyprinus carpio), African catfish (Clarias gariepinus) and Nile tilapia (Oreochromis niloticus), being the most widely used (Vasdravanidis et al., 2022). Moreover, silver catfish (Rhamdia quelen) and carnivorous hybrid walleye (Sander vitreus x Sander canadensis, aka saugeye) have also been proved suitable for aquaponics under specific conditions of growth (Maucieri et al., 2018; Jaeger et al., 2019; Baßmann et al., 2020; Birolo et al., 2020; Ghamkhar et al., 2020; Pinho et al., 2021). Several low-tech fish farming systems have been studied (Birolo et al., 2020; Maucieri et al., 2020; Bordignon et al., 2022a), as well as a few large-scale systems (Elnour et al., 2023), that enable production in degraded or even urban environments (Dos Santos, 2016). The different setups can be distinguished according to the type of water circulation system and the distribution of nutrients, into coupled aquaponic systems (CAPS), where there is continuous water circulation between the hydroponic system and the fish culture and decoupled aquaponic systems (DAPS), with a clear separation of the two systems and continuous control of the direction and movement of nutrients (Goddek et al., 2019).
At the same time, the plant species that can be grown in a satisfactory manner among these systems are both edible vegetables such as lettuce (Lactuca sativa L.), spinach (Spinachia oleracea), cauliflower (Brassica oleracea), cucumber (Cucumis sativus) and tomato (Solanum lycopersicum), and ornamental species such as moneywort (Lysimachia nummularia L.) and fishmint (Houttuynia cordata) (Maucieri et al., 2018; Jaeger et al., 2019; Arakkal Thaiparambil and Radhakrishnan, 2022). Both fish and vegetable species are usually chosen according to the geographical location, commercial activities, financial balance and the usual nutritional needs of the population (Bosma et al., 2017). With the appropriate market plan, depending on the choice of fish and vegetable species, high value-added income and great market competitiveness for the producer are forecasted (Miličić et al., 2017). Common hydroponic systems that can be used in aquaponics are raft systems (RAFT) which are also called Deep Water Culture systems (DWC), where plants are grown in styrofoam or plastic trays in tanks filled with nutrient-rich water and Media-Filled Beds Systems (MFBS), where various solid substrates are used in grow beds to support and grow cultivated plants (Forchino et al., 2017).
The importance of aquaponic systems lies in a number of innovative practices, such as the use of fish culture wastewater after treatment processes with filters and microbial communities for solid waste removal and nitrate enrichment (Arakkal Thaiparambil and Radhakrishnan, 2022), avoiding the discharge in the environment. This fact leads both to the protection of the environment from disposal of harmful waste, and to the saving of water and fertilizers, as water reuse efficiency can rise up to 95–99% in relation to conventional farming practices (Dalsgaard et al., 2013), while the use of fertilizers can be reduced by up to 77.7% (Suhl et al., 2018). In addition, the symbiosis of fish, microbial community and vegetables compose a simplified ecosystem that under controlled and stable conditions constitutes a sustainable farming practice, with multiple economic and environmental benefits (Blidariu and Grozea, 2011).
In order to quantitatively assess the degree of environmental sustainability of these systems, the application of Life Cycle Assessment (LCA) method (ISO 14040, 2006) is crucial, as it provides a holistic estimation of the direct and indirect effects derived from the use of inputs and the operation of the production systems (Nemecek et al., 2016). The most common applications of LCA in aquaponic systems focus on their comparison with pure hydroponics (Jaeger et al., 2019; Chen et al., 2020), system simulations and theoretical approaches (Forchino et al., 2017), small-scale systems (Maucieri et al., 2018), and research scale facilities (Ghamkhar et al., 2020). In all these cases, the environmental impacts are investigated under the prism of different parameters, such as stocking density of fish and different allocation levels and choices (Boxman et al., 2017; Bordignon et al., 2022a). LCA constitutes the keystone reducing environmental burden of aquaponics through the detailed recording of every input and every operating parameter of the systems, which is a necessary prerequisite for accurate and transparent results. The increased interest in conducting environmental analyses in aquaponic systems is also reflected by the increase in published studies in recent years (Greenfeld et al., 2022).
In this paper, LCA has been conducted on a high-tech pilot application of a vertically integrated aquaponic system. The originality of the study is reflected by the system’s arrangement and design, as the fish farming system is located in the underground area of the greenhouse and utilizes exclusively harvested rainwater for growing baby leaf vegetables (lettuce and rocket), in different fertilization treatments. The existing system was evaluated in terms of its environmental impacts and alternative scenarios were also tested in order to propose an improved cultivation plan. To the best of our knowledge there has been no other LCA study on a vertical high-tech aquaponic system using rainwater for irrigation. Hence the primary aim of this study is to analyze the total impact of the system in various environmental impact categories and decisively advance the current efforts to approach sustainability in aquaponic systems.
2 Materials and methods
The examination of the environmental performance of the constructed aquaponic system was carried out using the LCA method, according to ISO standards 14,040, 14,044 and 14,067 (ISO 14040, 2006; ISO 14044, 2006; ISO 14067, 2018). The conduction of LCA includes four distinct stages, which are: goal and scope definition, inventory analysis, impact assessment and interpretation. In the first stage, the purpose of conducting the study is determined, while the functional unit that will be used, must also be defined. In the second stage, the detailed quantities of inputs and outputs that were used and observed during the production process are recorded. In the third stage, the quantitative calculation of the effects of the system on the selected environmental indicators is carried out, while in the fourth and last stage, the observed results are analyzed and interpreted.
2.1 Goal and scope
The purpose of the present study is to profile the environmental burden caused by 12 different production case studies in the high-tech aquaponic system integrated as a pilot application and aimed at the simultaneous production of baby lettuce and rocket with rainbow trout. For the different cases developed, all inputs and practices associated with effects on specific environmental indicators, as well as products and nutrient emissions from fish sludge, were recorded. The findings of this work are expected to highlight the various hotspots of each production case study and lead to the proposal of an improved cultivation plan in aquaponics systems, that concerns both producers and researchers.
2.1.1 Functional unit
The selection and use of the appropriate functional unit is one of the primary stages in conducting LCA, as it contributes to the comparison between the results of various studies and constitutes a point of convergence between inputs and outputs (ISO 14044, 2006). Different functional units may lead to different results (Ravani et al., 2023), hence the selection should be based on established selections of previous publications on similar themes of research and the action proposed by official guidelines.
Aquaponic systems are characterized by a relative complexity in presenting the results, due to the simultaneous production of more than one product. In order to obtain meaningful insights into the environmental performance of the multifunctional aquaponic system under study, 1 kg of vegetable (1 kg baby lettuce or 1 kg baby rocket, depending on the case study) was chosen to be defined as the functional unit. Trout was considered a co-product and mass allocation was applied for data analysis and extraction of the results. Allocation was calculated based on the mass proportion between the two outputs, namely vegetable and trout. The total amount of the products was summed, the sum was set as 100%, and then the share of each product was calculated for allocation. Given that the allocation choice has a significant impact in the extracted results (Michiels et al., 2021), mass allocation was chosen in agreement with other works studying similar aquaponic applications (Forchino et al., 2017; Bordignon et al., 2022a).
2.1.2 System boundaries
The boundaries’ selection of the aquaponic system was based on the primary goal set for this study and the specific characteristics of the structure of the system under examination. The production of leafy vegetables was highly associated with the production of fish through the utilization of fish wastewater. Thus, system boundaries included both the processes and the inputs used for the operation of the fish farming system, and the growth of plants in the greenhouse area, the outputs of the two parts of the system, as well as the emissions produced from fish waste (N, P emissions to water). The assessment was characterized as cradle-to-gate and included the direct emissions released to water and soil as well as CO2 emissions released from the fish during the production process and the indirect emissions accompanying the inputs inserted to the system, i.e., those released during the production of the raw materials. Particular stages were not included in the assessment, such as trout hatchery phase, a practice followed by other studies (Aubin et al., 2009; Boxman et al., 2017), post-harvest treatments and seeds production, due to low/neutral impact in the results of the assessment and wide variation in post-harvest product handling scenarios (Ghamkhar et al., 2020). The particular methodology for defining cradle-to-gate system boundaries was adopted by other studies evaluating the performance of aquaponic systems in a comprehensive manner (Boxman et al., 2017; Jaeger et al., 2019; Chen et al., 2020; Ghamkhar et al., 2020). The boundaries of the system and all materials included are depicted in Figure 1.
2.2 Inventory analysis
2.2.1 System description
The development of the aquaponic system took place at the facilities of the Institute of Plant Breeding and Genetic Resources of ELGO-DIMITRA, in Thermi Thessaloniki, Greece in July 2022. The greenhouse of the Institute (40°32′N, 22°59′E, 15 m) covered an area of 106.4 m2 and was utilized for the plant cultivation. It should be clarified that in order to save space and improve the efficiency of land use, the fish farming system was developed in an underground area of 52.6 m2, that was built right below the greenhouse, and the area was specifically designed for the needs of the pilot application. The system is considered high tech, as innovative technological tools were used for the automatic control of the fish and plant growth systems. Specifically, there were sensors to control the conditions of the two cultivation areas, with the aim of the automatic operation of the systems (maintenance of constant water level, temperature in the fish rearing tanks, automated cooling systems in the greenhouse, etc.), without requiring human intervention. The whole system is characterized as decoupled aquaponics. Fish were reared in Recirculating Aquaculture System (RAS), while vegetables cultivation was took place in DWC. In an intuitive manner, this means that fish farming was developed in a closed loop system, with the water being recirculated in the underground area and the nitrate-enriched water exiting the system in an upward direction, into the greenhouse, in the vegetable cultivation tanks, whenever it was deemed necessary.
The selected fish species was rainbow trout, which is a cosmopolitan species that constitutes the most valuable and highly cultivated freshwater fish in the country (Vasdravanidis et al., 2022), while two baby vegetable crops, lettuce and rocket (Eruca sativa Mill.) were selected as the optimal choice, due to the short biological cycle and widespread appeal to consumers. The first growing season lasted 21–34 days, depending on the case study performed. The substrates used for vegetables cultivation were peat and perlite. Peat is a common substrate for cultivation in greenhouses (Atzori et al., 2021), while perlite was chosen as an inert support material from which no additional nutrients are provided to the plants, which contributes to better evaluation of the nutrient solutions. Sowing was carried out by putting 2 to 3 seeds in each position, in trays of 300 positions (dimensions: 0.33 × 0.67 m). A total of 15 trays were sown with baby lettuce in peat and an additional 15 with baby lettuce in perlite, while the same experimental design was applied for baby rocket cultivation. After sowing, the trays were placed in a closed pre-germination chamber, with constant temperature and humidity, until the germination of the majority of the seeds was observed. Seeds in peat germinated earlier than those in perlite and were placed in the cultivation tanks four days after sowing, in contrast to the perlite trays which were ready to exit the chamber seven days after sowing and were also placed directly into the tanks in the greenhouse.
In the underground area where fish were farmed, there were (i) 2 rearing tanks with a capacity of 1,500 L each, (ii) a mechanical filter for separating solid and liquid waste, (iii) a biological filter with colonies of Nitrosomonas sp. and Nitrobacter sp. Bacteria for the conversion of ammonia waste into nitrates and (iv) two sump tanks for storing water enriched with nitrates, making it available at all times for use in the greenhouse. Also, a UV lamp was used for sterilizing the water that went up into the greenhouse. The rainwater that was used in the system, was initially collected in an outdoor steel tank with a capacity of 14 m3 and after filtering by a triple resin filter for microparticles (5–20 μm) removal, was stored in the basement in a plastic tank with a capacity of 1 m3. The water was continuously recirculated in the basement by two water pumps, while there was also oxygen supply to the tanks by air stones. The operation of the entire system was monitored on a 24 h basis by the IoT system, which kept the growth environment conditions constant and recorded measurements of various parameters (temperature, humidity, pH, oxygen, solar radiation).
In the greenhouse area there were six stainless cultivation tanks (capacity of 500 L each), that were filled with water at the beginning of the growing season. After the end of the experiment, tanks were refilled only with the amount of nutrient solution that had been consumed, facilitating the next crop to be installed directly, avoiding water waste and deposition of fertilizers in the environment. Oxygen pumps and recirculating pumps were installed inside the cultivation tanks and operated constantly in a 24 h basis, to provide oxygen in the root-zone of the plants for prevention of bacterial growth due to anaerobic conditions, the coverage of the oxygen needs of the root and the even distribution of fertilizers and nutrients in the solution. Three treatments were implemented, with 2 tanks for each treatment and an even distribution of 5 baby lettuce trays and 5 baby rocket trays in each tank. The treatments were as follows: 1st treatment—Hoagland: water originated from the rainwater tank, enriched exclusively with synthetic fertilizers in the concentrations desired for the crop. 2nd treatment—Mix: the fish water was analyzed and enriched with synthetic fertilizers until nutrients reached the desired concentration for the cultivation. 3rd treatment—Fish: the wastewater from the fish farm is filtered (in the solid waste and biological filters) and after being analyzed to determine its concentrations of nitrate ions and macronutrients, it was used directly in the tanks without further treatment or enrichment with chemical fertilizers. In the three tanks with different nutrient solutions, trays with peat substrate were placed, while in the other three tanks trays with perlite substrate were used. For better representation of the experimental design, an illustration of the greenhouse cultivation and the underground area is presented in Figure 2.
2.2.2 Production case studies
The different production case studies resulting from the different combinations of plants, treatments and substrates are depicted in Table 1. Two different substrates were used to investigate whether there is a difference in their environmental and quality performance. The total cultivation period refers to the days needed for the plants to germinate in dark conditions in a specially designed closed chamber, with 20 ± 2°C temperature and 70 ± 10% relative humidity, as well as the time needed for their growth in the greenhouse, until the leaves were ready for harvest. Each production case study consisted of 5 replications (5 sowing trays per case study) and the total yield per case study was calculated as the sum of the yield of the 5 trays. Input consumptions for each case study were calculated in a similar manner, as the sum of the inputs used for the five trays.
2.2.3 Collected data
The data collected were related to input consumptions to produce baby leaves and trout weight gain for the total cultivation period of each case study. SimaPro software v.9.4.0.2 was used for input and background data recording, as well as the analysis and the extraction of the results.
For all case studies, input parameters were related to system construction materials (greenhouse or/and basement structure materials, cultivation tanks and rainwater collection tanks), substrate amount used per case study, fertilizers consumed, plastic seed trays, water, as well as the energy used for the equipment operation (recirculation pumps, oxygen supply, wet panel for cooling and air-condition and humidifier for seed germination). During calculations, the amount of construction materials was adjusted according to the lifetime of each material and the cultivation area. In case studies where water from fish farming was used (Case studies LPtH, LPtF, LPrM, LPrF, RPtM, RPtF, RPrM, RPrF), in addition to the aforementioned inputs, the inventory analysis included all input consumptions for the operation of the fish farming system. These included the construction materials of the basement, the plastic pipes, the plastic tanks, the fish feed, the energy consumption for the lighting, the operation of all pumps, the air conditioner used to keep the temperature constant at 18°C, and the dehumidifier. All the amounts of inputs were extrapolated to the area of the greenhouse occupied by each case study and its total cultivation period. Transportation for all materials was also considered and was calculated by inserting the appropriate input categories in SimaPro software.
The outputs include two products, the difference in the total weight of the 1,080 farmed fish during the experiment, which was obtained from sample measurements at the beginning and end of the experiment and the yield of the harvested lettuce and rocket baby leaves. Furthermore, the emissions of nutrients released in the water (nitrogen and phosphorus), from the mechanical filter operation, were also calculated. The wastewater resulted from cleaning the mechanical filter consisted of solid waste that was applied as fertilizer in other cultivations in the field. Emissions in water are related to nitrogen and phosphorus escaping from sludge during the mechanical filter operation. To calculate the nutrients (N, P) produced as emissions to water, coefficients from the Ecoinvent database were used. CO2 emissions from the fish were monitored through a meteorological sensor. The amounts of inputs, outputs and emissions analyzed and monitored are described in detail in Table 2.
2.3 Impact assessment
To estimate the environmental impact of the system, the CML-IA baseline v.3.07 (EU25) method was used, which is implemented in SimaPro software and is often used in environmental impact assessment studies in greenhouse cultivation (Ravani et al., 2023), aquaculture systems (Henriksson et al., 2012) and the combination of these two systems, in aquaponics (Boxman et al., 2017; Chen et al., 2020). Eleven midpoint impact categories were studied for the examination of the environmental performance of the system, at an intermediate level of detail. The examined impact categories were Abiotic Depletion (AD, kg Sb-eq), Abiotic Depletion-fossil fuels (Adf, MJ), Global Warming (GWP100, kg CO2-eq), Ozone Layer Depletion (ODP, kg CFC-11-eq), Human Toxicity (HT, kg 1,4-DB-eq), Freshwater Aquatic Ecotoxicity (FAE, kg 1,4-DB-eq), Marine Aquatic Ecotoxicity (MAE, kg 1,4-DB-eq), Terrestrial Ecotoxicity (TE, kg 1,4-DB-eq), Photochemical Oxidation (PO, kg C2H4-eq), Acidification (AF, kg SO2-eq), and Eutrophication (ET, kg PO4-eq). As energy appears to have a primary role in the overall function of the system, Cumulative energy demand v.1.11 method was applied to calculate a robust indicator for energy focused assessments. Cumulative Energy Demand (CED) is an expression of the amount of direct and indirect energy consumed during the production process (Huijbregts et al., 2010) and was used to investigate the usage of energy for the best-case studies. The impact factors for all calculations and analyses were derived from Ecoinvent 3 database, that is included in SimaPro software.
2.4 Interpretation
For better interpretation of the results, a sensitivity analysis was performed to assess the impact of alternative choices of inputs that constitute hotspots in the initial analysis and the impact of alternative experimental design. This method may lead to better understanding of the influence of specific parameters in the overall environmental impact categories and the identification of integrated techniques for mitigating the burdens of the systems (Elnour et al., 2023). For the sensitivity analysis, different levels of allocation were tested, in a scenario where, fish with a higher weight gain had been used for the experiment instead of fish fry. In a second basis of the sensitivity analysis, electricity from the grid was replaced by electricity produced by photovoltaics. Electricity has already been presented as a major hotspot in several aquaponics studies (Greenfeld et al., 2022), so it was important to check the sensitivity of the results due to this parameter. Since photovoltaics facility is already implemented besides the greenhouse and could be used in future research, it was important to test its environmental effect when providing electricity for the aquaponic system.
3 Results and discussion
3.1 Vegetables yield and fish growth
For baby lettuce and rocket, the total yield was measured as the total fresh weight of baby leaves harvested from the five trays of each case study, at the end of the cultivation phase and ranged from 1.18–2.76 kg for lettuce leaves and 0.34–1.78 kg for rocket leaves. Regarding baby lettuce cultivation, in each of the examined comparisons, no statistically significant differences were observed between Hoagland and Mix. The yield ranged from 2.27 to 2.51 kg/m2 in Mix with perlite and peat, respectively. For baby rocket, while taking into account the inability of Fish-Perlite to adapt to the specific treatment which resulted in low quality production, the lowest yield was in Mix-Perlite with 1.08 kg/m2, and the highest in Hoagland-Peat with 1.62 kg/m2, while there was a statistically significant difference between the two yields. In the treatments where only fish water was used that contained 83.44 mg/L NO3- concentration, both the weight of the leaves and their quality were unsatisfactory, as they were weak and not suitable for consumption. The degraded production of the plants was due to the fact that P and K were limited, as a result of their removal from the system during the mechanical filtration of the solid waste of the fish.
Fish weight showcased a steady increase during the experiments and was measured at 0.12 gr per fish per day. The initial weight of 1,080 fish was measured at 7.94 kg (7.35 gr per fish). On the 16th day of the experiment, the final weight of the fish was measured at 9.88 kg, on the 17th day at 10 kg and on the 25th day at 11.05 kg. The final fish output was calculated as the difference between the initial and final weight of the fish in kilograms and extrapolated for 1.1 m2. The weight gain of the fish was measured at 0.24 kg for 16 days (case studies LPrM, RPrM), 0.26 kg for 17 days (case studies LPtM, LPtF, RPtM, RPtF) and 0.39 kg for 25 days (case studies LPrF, RPrF). It can be concluded that the number of days each experiment lasted affects the results by producing more outputs. However, it is important to state that the larger amount of product does not correspond to disproportionately higher consumptions.
The importance of growth rate of fish in optimizing the system is highlighted by other studies as well. There could be a significant difference in the results for different growth rates of fish, since it affects the allocation for the co-products. In a study of aquaponics with tilapia, fish growth rate was calculated at 0.99 g/day in a one-month period, however it was noted that the full 180 day growth of the fish should have been considered to avoid underestimation of fish value in aquaponics (Chen et al., 2020). Stocking density was another factor that was tested for its possible effect in trout yield, but no significant differences were found in fish growth, that was measured at 331 g after 117 days (Bordignon et al., 2022b).
The emissions of nitrogen and phosphorus in water from wastewater produced due to the operation of the mechanical filter, were calculated based on the values of the nutrients found to be included in the wastewater entering a waste treatment plant and presented in the study of Verdoodt (2018). Emissions in water from aquaponics appears to be a critical pollutant, which has also been studied by other works (Forchino et al., 2017; Bordignon et al., 2022b). Specifically, the quantities of nutrients concentration in the present study, are 0.028 kg N/m3 of water and 0.0031 kg P/m3 of water, thus the final values for this study were extrapolated based on the amount of water exiting the mechanical filter during the experiment that was measured at 0.006 m3 in 16–17 days and 0.009 m3 in 25 days for 1.1 m2. The corresponding values were 0.00518 kg of N and 0.0023 kg of P per kg of lettuce in a floating system of another study (Forchino et al., 2017). The amount of emissions in water is based on the growth stage and number of fish, since more and older fish produce higher amounts of metabolic products and excrement, so there is greater need to clean solid residues from the mechanical filter, thus higher emissions to water.
3.2 Aquaponic system’s life cycle impact assessment
The results of the analysis for the quantification of the environmental impacts of the case studies are presented at two levels. Due to large amount of data, the environmental impacts are presented comparatively for the three case studies with baby lettuce in peat, then separately for the three case studies for baby lettuce in perlite and the corresponding procedure was carried out for the baby rocket as well. It is showcased that for baby lettuce, the optimal scenario appears to be the Lettuce-Perlite-Mix case. This case had lower environmental burden than the treatment with plain fish water, as presented in Figure 3B. Thus, the detailed impact assessment results for this case study were presented separately. As already mentioned before, there was no production of good-quality rocket leaves in the treatment with plain fish water, hence the impact assessment results for the Rocket-Perlite-Mix case study were presented instead, to achieve uniformity and convergence in the presentation of the results with those of case study LPrM. All the analyses were carried out with an emphasis on the leafy vegetable which was considered the main product to be received by the pilot aquaponic system with fish as secondary/co-product. However it should be clarified that as mentioned above, the allocation to the two products has already been considered.
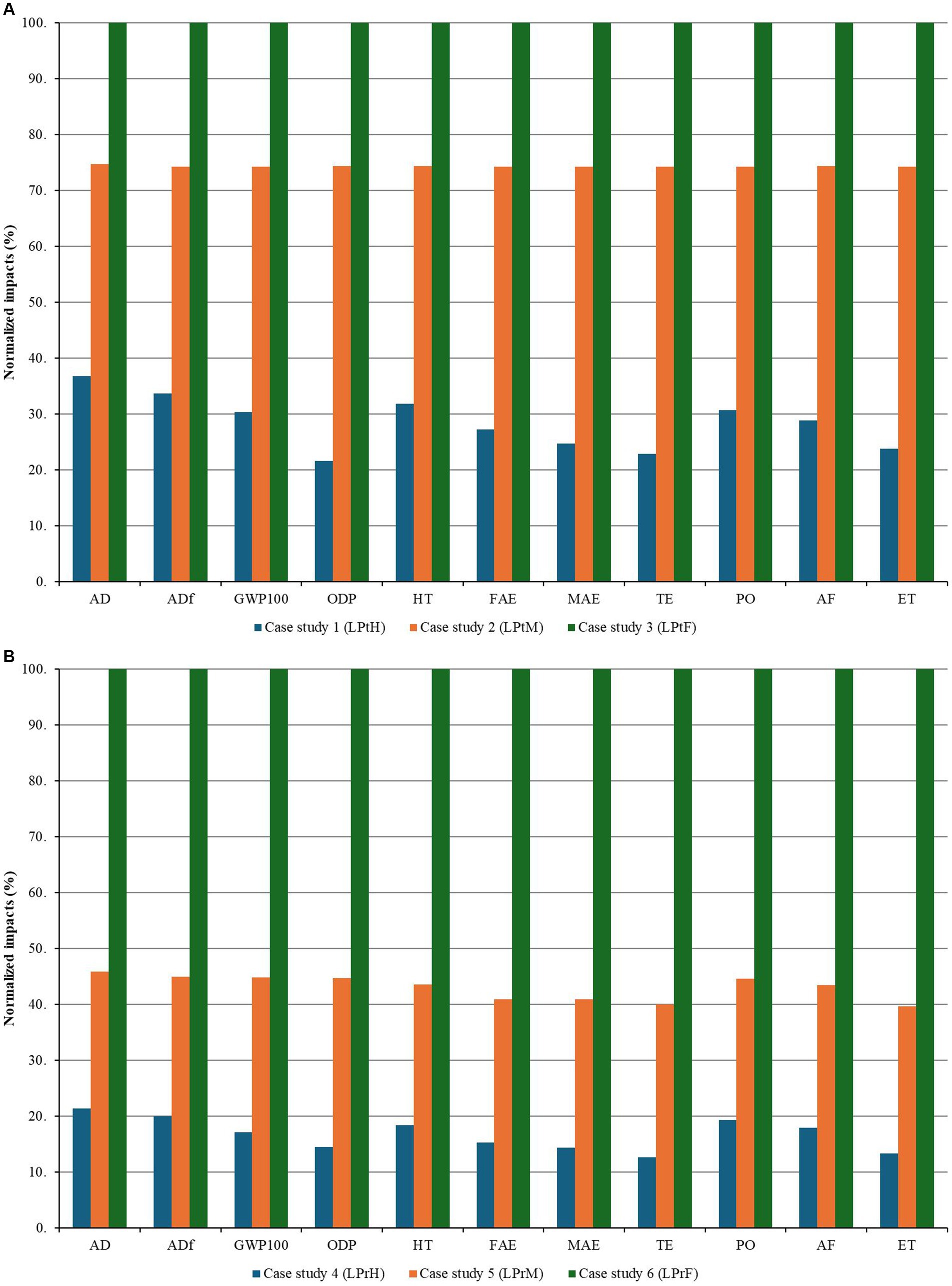
Figure 3. Comparison of environmental impacts of baby lettuce-peat-different nutrient solution cases (A) and baby lettuce-perlite-different nutrient solution cases (B).
As presented in Figures 3, 4, between case studies LPtH-LPtM-LPtF, LPrH-LPrM-LPrF, RPtH-RPtM-RPtF, RPrH-RPrM-RPrF there is a large difference in all impact categories between the three treatments, both for baby lettuce and rocket. The treatments where no fish water was utilized and were a representation of purely hydroponic cultivation in a greenhouse, without a second product (fish) (Case studies LPtH, LPrH, RPtH and RPrH), had the lowest environmental impact. This phenomenon has been found in other studies as well (Greenfeld et al., 2021) and is attributed to the operation of multiple machinery and equipment that is needed for fish rearing system. Among the cases where fish water was used (Mix–Fish), Mix treatments seem to be environmentally friendlier, due to the partial use of fertilizers and the achievement of higher yield. This finding is more pronounced in baby lettuce, while in baby rocket the difference between the Mix and Fish treatments with a peat substrate is smaller, which is due to the low yields observed in this specific plant species for both treatments. An important finding is that in all case studies where perlite was used as cultivation substrate, the environmental impact in mix case studies was much less than in fish case studies. On the other hand, in all cases with peat substrate, the difference in the effects between mix and fish treatments was comparatively smaller than the corresponding ones for perlite. In order to search for the causes for this phenomenon, the effects of the inputs and outputs on the systems with the two different substrates were investigated in detail. The examination indicated that the very low yield observed in the perlite substrate and fish water treatments in baby lettuce and even more so in baby rocket, led to greater impacts for case studies LPrF and RPrF, even though the input amounts were almost the same. The plants could not absorb sufficient amounts of all the necessary nutrients from the plain fish water, while they also lacked the supply of nutrients from the substrate, as perlite is an inorganic substrate, unlike peat which is organic and can be an initial aid in plant nutrition. This fact led to much higher values of environmental indicators in the treatments with perlite and fish water (GWP100 in Case study LPrF: 47.2 kg CO2-eq/kg of baby lettuce) than in the treatments with peat and fish water (GWP100 in Case study LPtF: 26.3 kg CO2-eq/kg of baby lettuce).
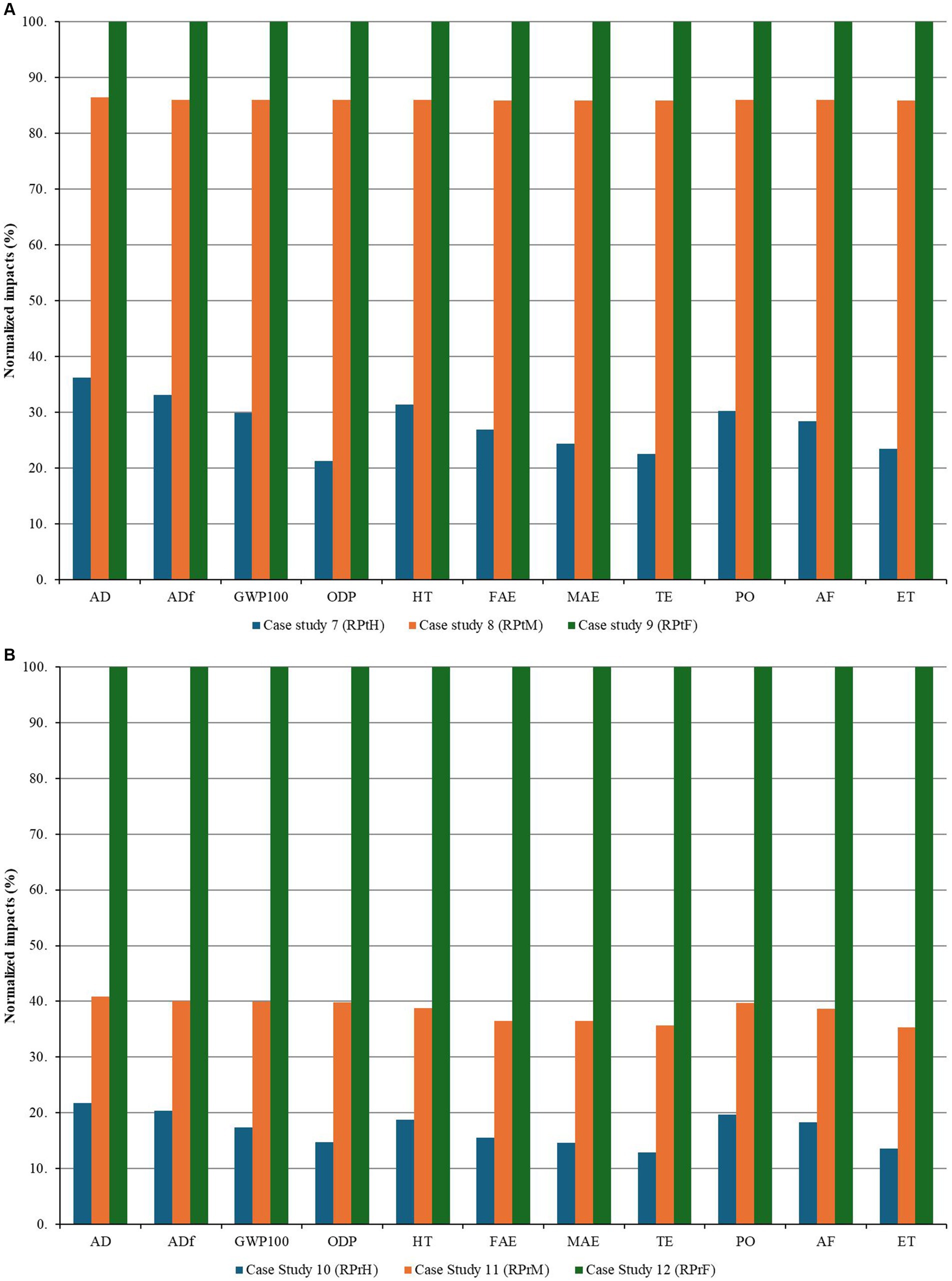
Figure 4. Comparison of environmental impacts of baby rocket-peat-different nutrient solution cases (A) and baby rocket-perlite-different nutrient solutions cases (B).
For Mix treatments, regarding carbon footprint (GWP), no remarkable differences were observed between case studies LPtM-LPrM and RPtM-RPrM that had different substrates, where carbon footprint was calculated at 19.5 and 21.2 kg CO2-eq/kg of baby lettuce for case study LPtM and LPrM respectively, and 33.5 and 40.6 kg CO2-eq/kg of baby rocket for case studies RPtM and RPrM, respectively. In fact, it appears that in peat case studies, carbon footprint was slightly smaller due to the corresponding slightly higher yield observed in the peat substrate scenarios. Due to this difference in yields, corresponding differences also emerged for some of the rest of the environmental indicators, where the values of the indicators were lower in the case studies with peat compared to those with perlite, in both baby lettuce and rocket. Cases 5 (LPrM) and 11 (RPrM) were considered to be more suitable from an environmental point of view for the present cropping system. The full analysis for these two cases is depicted in Figures 5, 6 respectively.
In studies that used vegetable and in particular lettuce as a functional unit, the carbon footprint showed a huge variability, due to a plethora of experimental designs, choice of the examined boundaries, climatic conditions that led to an increase in energy consumption, etc. (Greenfeld et al., 2022). In a study where mass allocation between products was applied, 1 kg of lettuce was calculated to be 6.47 kg CO2-eq (Jaeger et al., 2019), while in a different system where lettuce was grown in a tilapia and bass aquaponic system, greenhouse gas emissions were calculated at 4.45 kg CO2-eq (Hollmann, 2017). In a large-scale production system, 54 ton of lettuce and 0.75 ton of goldfish resulted in the production of 29,568 kg CO2-eq (Greenfeld et al., 2021). Finally in a study of a small-scale aquaponic system for the production of 1 kg leafy greens, carbon footprint was calculated at 49.50 kg CO2-eq (Valappil, 2021) which is comparable with the findings of the present study.
The results for all studied environmental indicators are similar for both plant cultivations in Mix treatment with perlite substrate, where the main hotspot in all indicators is the electricity spent to operate the electrical equipment for the fish rearing system. Same findings are showcased in many studies, where electricity consumption is accounted for more than 80% in most of environmental impact categories (Elnour et al., 2023), while scenarios excluding impacts of electricity and heat may lead to more than 91% decrease in GWP and AC (Ghamkhar et al., 2020). The largest impact on all indicators is occupied by the electricity used to provide oxygen in fish tanks and cool the area where fish were reared, while the next largest impacts are occupied by the electricity to operate the water recirculation pumps in the fish rearing system. As can be seen in Figures 5, 6, there is no difference in the identified hotspots between the two cultivated plants. Overall, the electricity consumed for the machinery operation both in the underground and greenhouse area contributed 55% to the total carbon footprint impact. This fact strongly demonstrates the need to find alternative sources of energy, which will result in reduced greenhouse gas emissions to achieve the least possible burden on the environment while ensuring the benefits of double production in aquaponic systems, with the help of eco-design tools like LCA (Forchino et al., 2018).
For the selected case studies LPrM and RPrM, the effects of the inputs on the carbon footprint indicator were analyzed and presented in Figure 7. The electricity was cumulatively presented and categorized as electricity for the operation of the fish farming system (39% of total impacts), electricity for the operation of the greenhouse (9%) and electricity for the operation of the seed germination chamber (7%).
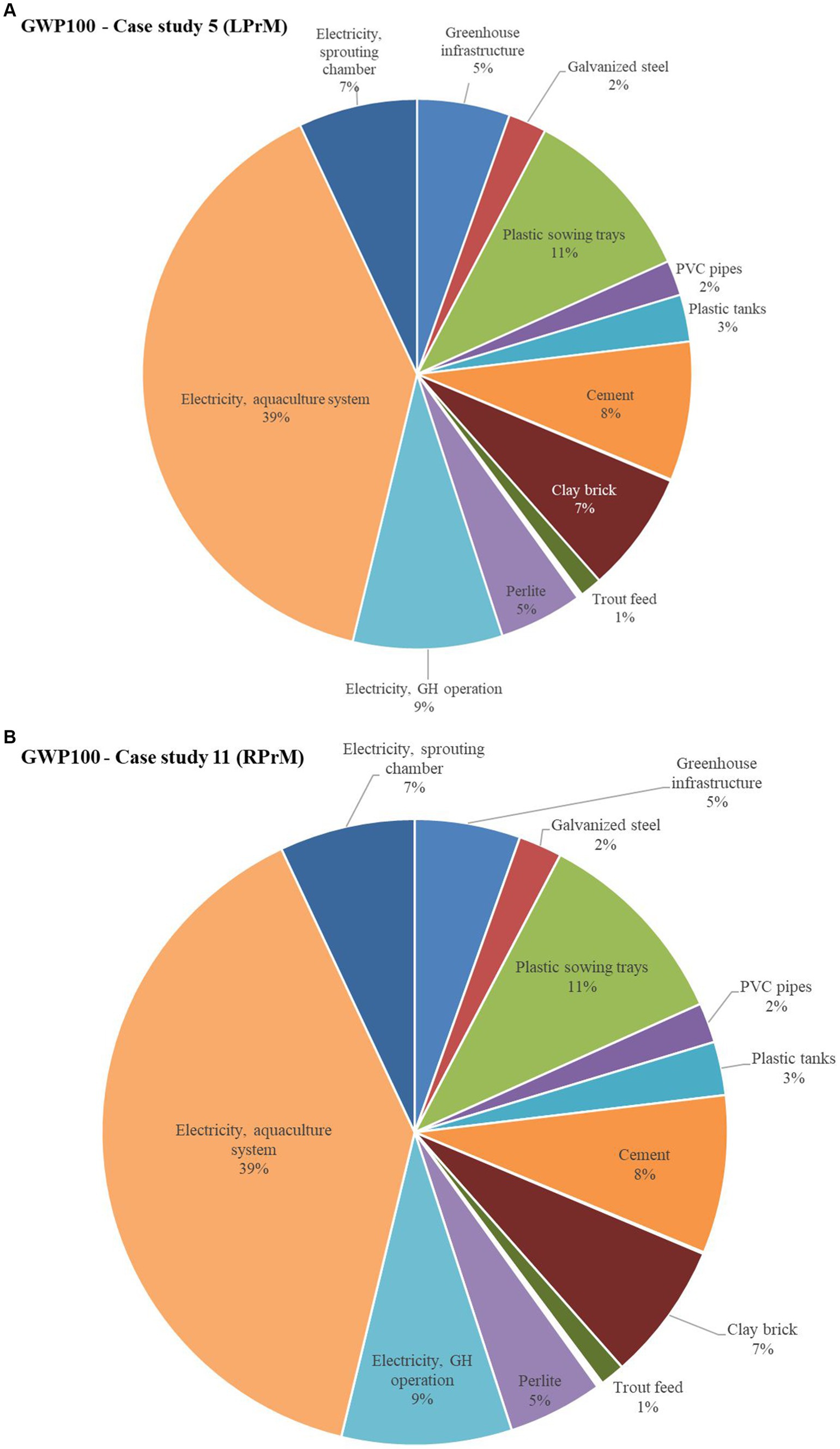
Figure 7. Share of carbon footprint values for different inputs in Case study 5-LPrM (A) and Case study 11-RPrM (B).
3.3 Cumulative energy demand calculation
CED was calculated for the two selected cases and was 359.0 MJ/kg of baby lettuce produced in Case study LPrM and 687.9 MJ/kg of baby rocket in Case study RPrM. The largest CED share for both cases is occupied by electricity consumed for the operation of the underground area for the fish rearing system and more particularly, the operation of the oxygen supply pump. This was the most substantial hotspot for all other impact categories and along with energy consumed for the operation of all machinery in the underground fish rearing area, it contributed to 40% of the total CED value for both case studies. The share of inputs in CED is presented in Figure 8, where all parameters with contribution less than 1% are excluded from the presentation of the results.
Due to increased energy consumption in aquaponics systems, similar findings of hotspots in CED coinciding with hotspots in GWP is also observed in other studies (Forchino et al., 2017; Kalvakaalva et al., 2023). In these studies, it is reported that CED is affected by the season of operation of the aquaponic system, due to lower production of fish or vegetable and high energy requirements in spring and winter, where it was calculated at 296 and 454 kWh/kg dry weight of tilapia, respectively, (Kalvakaalva et al., 2023). In small-scale low-tech aquaponic systems, CED is much lower and calculated at 161 MJ, due to lower energy consumption (Bordignon et al., 2022b).
3.4 Alternative scenarios—sensitivity analysis
For the sensitivity analysis, two different alternative scenarios, each for Case studies LPrM and RPrM from the initial experiment, were considered to test whether the results will be affected by different parameters of the production cases. Initially, once it was identified that the operation of the fish farming system is the most important hotspot for carbon footprint, it was necessary to find solutions to utilize the area in the best possible way to minimize its impact. Production of larger size fish was studied as an optimal option, and data on fish growth rate, amount of feed provided, and amount of water discharged into the environment by the operation of the mechanical filter were collected after the experiment described in this study was completed, when fish had larger size and the operation and maintenance of the system continued. All other inputs used in the alternative scenarios were the same as in the original case studies, as they would be used unchanged in a future setup of the experiment.
Thus, an alternative scenario was developed in which it was assumed that the fish were received at an older age, when the initial weight of the 1,080 fish was 108.0 kg instead of 7.94 kg in the actual experiment (100 gr weight per fish instead of 7.35 gr). The weight of the fish after 16 days was measured at 145.4 kg. The amount of feed consumed by the fish was still 3% of their weight, so the total amount of feed consumed during the 16 days of the system operation would be 60.0 kg. The above values refer to the aquaculture system that could support 4 plant cultivation tanks of a total 8.8 m2 area, therefore the appropriate distribution of the quantities was made to 1.1 m2 which was the cultivation area for each case study. The amount of wastewater lost from the system during the operation of the mechanical filter with extrapolation for 1.1 m2 was 0.03 m3. Based on these data, the environmental analysis for the alternative scenario was carried out and its effects on the considered environmental indicators were calculated with the same method that the actual case studies were analyzed (CML-IA baseline v.3.07 EU25).
It was calculated that in the alternative system for Case study LPrM, the carbon footprint was 22.2 kg CO2-eq/kg of baby lettuce, i.e., about 55% lower than the actual case study. The corresponding value of alternative scenario 11 for baby rocket was calculated at 27.1 kg CO2-eq/kg of baby rocket, i.e., 70% less than the actual case study. The reduction is also significant in the remaining environmental indicators, where it ranges from 40–61% for scenario 5 and 62–75% for scenario 11, as shown and detailed in Table 3. Hotspots identified were the same as in the initial cases, with electricity occupying the first place in the effects of all indicators. The substantial reduction of the effects of the alternative systems on the environmental indicators highlights the need to conduct the experiments in the optimal conditions to achieve the optimal efficiency of the system. The parameters that affect the values of the indicators, i.e., the inputs, can be modified, especially in closed controlled systems, such as greenhouses, which justifies the importance of these systems in the sustainability approach (Achour et al., 2021).
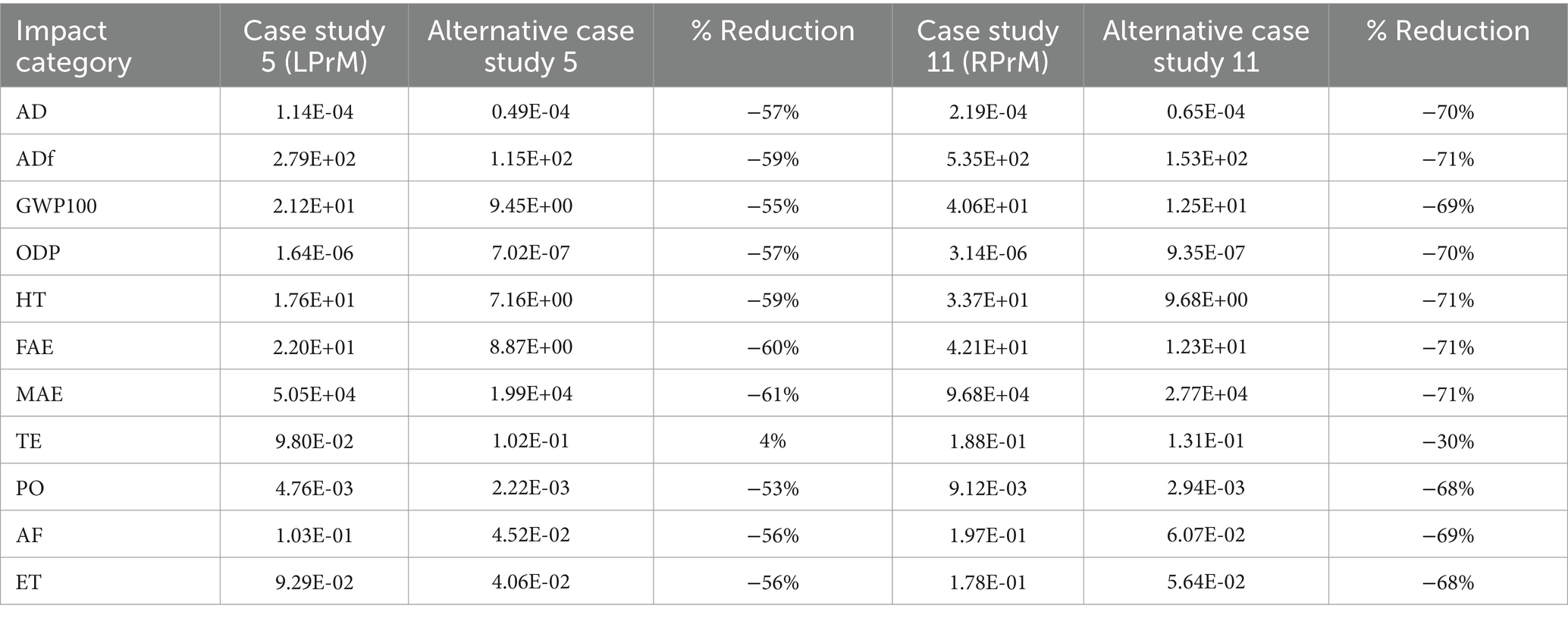
Table 3. Sensitivity analysis results for the alternative scenario with higher fish body weight value.
For the second level of sensitivity analysis, the electricity extracted from the grid and used as an input to the system under consideration, was replaced in the second alternative scenario with energy produced by photovoltaics. The choice of alternative forms of energy is used by similar works in the field (Elnour et al., 2023) and may contribute to the assimilation of the sensitivity degree occurred when performing the LCA method. The type of photovoltaics used as an energy source for the alternative scenario concerned grid-connected low voltage electricity with a 3 kWp building integrated photovoltaic (PV) module in Greece (Swiss Centre for Life Cycle Inventories, 2023), while the appropriate adjustments were made to the specific registration in SimaPro so that could meet the energy needs of the system under consideration. The results of the alternative scenario with photovoltaics are presented in Table 4.
The results indicate that for the second alternative scenario of the two original case studies (Table 4), the only indicator that showed an increase in its value was AD where there was a 31% increase. The impact of photovoltaics use and especially mono-crystalline cells is increased in this category, due to the use of fossil fuels for energy generation during their manufacturing processes (Dubey et al., 2013). The percentages of reduction in the second alternative scenario are almost the same for both case studies as for the specific level of sensitivity analysis only one parameter was changed (replacement of grid energy with energy from photovoltaics), without actually modifying its value (the amount of energy required to operate the system remains the same). This comes in contrast to the first level of analysis where multiple parameters were modified (fish weight, fish feed, N, P emissions to the environment) and the distribution of products also changed, due to increasing fish production. Modification of energy parameter in systems is proved crucial in other studies as well, where the switch to photovoltaic energy leads to a decrease in the GWP (Bordignon et al., 2022b). An important finding of this alternative scenario is the 50% reduction of carbon footprint indicator, which can lead to further decision-making and inclusion of photovoltaic panels in agricultural production.
4 Conclusion
In the present study the LCA results of a pilot high-tech aquaponic system in a vertical arrangement were presented. Various experimental designs with different combinations of parameters were examined in order to identify the optimal production plan in terms of its environmental performance. The parameters were chosen in such a way that the operation of the aquaponic system is based on the principles of circular economy and reuse of resources, with the least possible waste. These included the use of rainwater, water enriched with nitrate ions from farmed fish waste, the use of peat and perlite substrates, the use of synthetic fertilizers in different concentrations and the use of two leafy baby vegetables. From the results of the analysis, it was found that the cultivation of baby lettuce and rocket in rainwater hydroponic systems has the lowest environmental impact, however, the comparison with fish water treatments cannot reject the latter as environmentally unfriendly, as duplicative production and reuse of aquaculture waste and resources need to be taken into account.
The cases with perlite substrate and utilization of water from the fish waste are the optimal choice for the specific experimental design. The main hotspot of these cases is the consumption of electricity to operate the machinery of the fish rearing area and more specifically, oxygen supply pump in the fish tanks. The carbon footprint in the Lettuce-Perlite-Mix (LPrM) case was calculated at 21.18 kg CO2-eq/kg of baby lettuce, while in Rocket-Perlite-Mix (RPrM) case it was calculated at 40.59 kg CO2-eq/kg of baby rocket. The effects of the systems on the rest of the environmental indicators studied were similar for the two cultivated vegetable species and in all indicators (Table 5). The largest share of effects was occupied by electricity, which accounted for 55% of the effects. This fact increases the need to find solutions to reduce the impact of the use of electrical energy on the environment.
An alternative scenario was considered where electricity supplied by the grid was replaced by electricity supplied by photovoltaics. The values of all environmental indicators were notably decreased, while indicatively for carbon footprint, its value reduction was 50%. Other solutions include the replacement of the oxygen supply pump with alternative machinery and further optimization of the system. The next steps include the study of the system from an economic point of view, in order to establish its degree of sustainability and formulating the optimal cultivation plan with the lowest burdens on the environment and the greatest possible economic benefit that can be suggested to producers.
Data availability statement
The original contributions presented in the study are included in the article/supplementary material, further inquiries can be directed to the corresponding author.
Ethics statement
The ethics approval for the experimental use of the rainbow trout (Oncorhynchus mykiss) was granted by the University of Western Macedonia local ethics committee given the protocol code 101, whereas fish receipt was confirmed by the Department of Aquaculture Development, Greek Ministry of Rural Development and Food, Decision number 167/41275/02-06-2022.
Author contributions
MR: Writing – original draft, Visualization, Software, Methodology, Investigation, Formal analysis, Data curation. IC: Writing – review & editing, Validation, Investigation, Data curation. NM: Writing – review & editing, Supervision. IG: Writing – review & editing, Resources. GN: Writing – review & editing, Supervision, Resources, Project administration, Funding acquisition, Conceptualization.
Funding
The author(s) declare that financial support was received for the research, authorship, and/or publication of this article. This research was co-financed by the European Regional Development Fund of the European Union and Greek national funds through the Operational Program Competitiveness, Entrepreneurship and Innovation, under the call RESEARCH-CREATE-INNOVATE (project code: T1EDK-00756).
Conflict of interest
The authors declare that the research was conducted in the absence of any commercial or financial relationships that could be construed as a potential conflict of interest.
Publisher’s note
All claims expressed in this article are solely those of the authors and do not necessarily represent those of their affiliated organizations, or those of the publisher, the editors and the reviewers. Any product that may be evaluated in this article, or claim that may be made by its manufacturer, is not guaranteed or endorsed by the publisher.
References
Achour, Y., Ouammi, A., and Zejli, D. (2021). Technological progresses in modern sustainable greenhouses cultivation as the path towards precision agriculture. Renew. Sust. Energ. Rev. 147:111251. doi: 10.1016/j.rser.2021.111251
Arakkal Thaiparambil, N., and Radhakrishnan, V. (2022). Challenges in achieving an economically sustainable aquaponic system: a review. Aquac. Int. 30, 3035–3066. doi: 10.1007/s10499-022-00946-z
Atzori, G., Pane, C., Zaccardelli, M., Cacini, S., and Massa, D. (2021). The role of peat-free organic substrates in the sustainable management of soilless cultivations. Agronomy 11:1236. doi: 10.3390/agronomy11061236
Aubin, J., Papatryphon, E., van der Werf, H. M. G., and Chatzifotis, S. (2009). Assessment of the environmental impact of carnivorous finfish production systems using life cycle assessment. J. Clean. Prod. 17, 354–361. doi: 10.1016/j.jclepro.2008.08.008
Baßmann, B., Harbach, H., Weißbach, S., and Palm, H. W. (2020). Effect of plant density in coupled aquaponics on the welfare status of African catfish, Clarias gariepinus. J World Aquac Soc 51, 183–199. doi: 10.1111/jwas.12574
Birolo, M., Bordignon, F., Trocino, A., Fasolato, L., Pascual, A., Godoy, S., et al. (2020). Effects of stocking density on the growth and flesh quality of rainbow trout (Oncorhynchus mykiss) reared in a low-tech aquaponic system. Aquaculture 529:735653. doi: 10.1016/j.aquaculture.2020.735653
Blidariu, F., and Grozea, A. (2011). Increasing the economical efficiency and sustainability of indoor fish farming by means of aquaponics-review. Sci. Papers Animal Sci. Biotechnol. 44:1.
Bordignon, F., Gasco, L., Birolo, M., Trocino, A., Caimi, C., Ballarin, C., et al. (2022a). Performance and fillet traits of rainbow trout (Oncorhynchus mykiss) fed different levels of Hermetia illucens meal in a low-tech aquaponic system. Aquaculture 546:737279. doi: 10.1016/j.aquaculture.2021.737279
Bordignon, F., Sturaro, E., Trocino, A., Birolo, M., Xiccato, G., and Berton, M. (2022b). Comparative life cycle assessment of rainbow trout (Oncorhynchus mykiss) farming at two stocking densities in a low-tech aquaponic system. Aquaculture 556:738264. doi: 10.1016/j.aquaculture.2022.738264
Bosma, R. H., Lacambra, L., Landstra, Y., Perini, C., Poulie, J., Schwaner, M. J., et al. (2017). The financial feasibility of producing fish and vegetables through aquaponics. Aquac. Eng. 78, 146–154. doi: 10.1016/j.aquaeng.2017.07.002
Boxman, S. E., Zhang, Q., Bailey, D., and Trotz, M. A. (2017). Life cycle assessment of a commercial-scale freshwater aquaponic system. Environ. Eng. Sci. 34, 299–311. doi: 10.1089/ees.2015.0510
Campanati, C., Willer, D., Schubert, J., and Aldridge, D. C. (2022). Sustainable intensification of aquaculture through nutrient recycling and circular economies: more fish, less waste, blue growth. Rev. Fisheries Sci. Aquaculture 30, 143–169. doi: 10.1080/23308249.2021.1897520
Chen, P., Zhu, G., Kim, H. J., Brown, P. B., and Huang, J. Y. (2020). Comparative life cycle assessment of aquaponics and hydroponics in the Midwestern United States. J. Clean. Prod. 275:122888. doi: 10.1016/j.jclepro.2020.122888
Coroiu, P. (2017). Baby-leaf NFT production and water management in aquaponic system. Dissertation/master’s thesis : University of Padova. https://hdl.handle.net/20.500.12608/24446
Dalsgaard, J., Lund, I., Thorarinsdottir, R., Drengstig, A., Arvonen, K., and Pedersen, P. B. (2013). Farming different species in RAS in Nordic countries: current status and future perspectives. Aquac. Eng. 53, 2–13. doi: 10.1016/j.aquaeng.2012.11.008
Dos Santos, M. J. P. L. (2016). Smart cities and urban areas—aquaponics as innovative urban agriculture. Urban For. Urban Green. 20, 402–406. doi: 10.1016/j.ufug.2016.10.004
Dubey, S., Jadhav, N. Y., and Zakirova, B. (2013). Socio-economic and environmental impacts of silicon based photovoltaic (PV) technologies, Energy Procedia, Elsevier Ltd., 322–334, 33
Elnour, M., Haller, H., and Martin, M. (2023). Life cycle assessment of a retail store aquaponic system in a cold-weather region. Front. Sustainability 3:51091. doi: 10.3389/frsus.2022.1051091
Forchino, A. A., Gennotte, V., Maiolo, S., Brigolin, D., Mélard, C., and Pastres, R. (2018). “Eco-designing Aquaponics” in A case study of an experimental production system in Belgium, Procedia CIRP, vol. 69 (Elsevier B.V.), 546–550.
Forchino, A. A., Lourguioui, H., Brigolin, D., and Pastres, R. (2017). Aquaponics and sustainability: the comparison of two different aquaponic techniques using the life cycle assessment (LCA). Aquac. Eng. 77, 80–88. doi: 10.1016/j.aquaeng.2017.03.002
Ghamkhar, R., Hartleb, C., Wu, F., and Hicks, A. (2020). Life cycle assessment of a cold weather aquaponic food production system. J. Clean. Prod. 244:118767. doi: 10.1016/j.jclepro.2019.118767
Goddek, S., Joyce, A., Kotzen, B., and Burnell Editors, G. M. (2019) in Aquaponics food production systems. eds. S. Goddek, A. Joyce, B. Kotzen, and G. M. Burnell (Cham: Springer international publishing).
Greenfeld, A., Becker, N., Bornman, J. F., Spatari, S., and Angel, D. L. (2021). Monetizing environmental impact of integrated aquaponic farming compared to separate systems. Sci. Total Environ. 792:148459. doi: 10.1016/j.scitotenv.2021.148459
Greenfeld, A., Becker, N., Bornman, J. F., Spatari, S., and Angel, D. L. (2022). Is aquaponics good for the environment?—evaluation of environmental impact through life cycle assessment studies on aquaponics systems. Aquac. Int. 30, 305–322. doi: 10.1007/s10499-021-00800-8
Henriksson, P. J. G., Guinée, J. B., Kleijn, R., and De Snoo, G. R. (2012). Life cycle assessment of aquaculture systems-a review of methodologies. Int. J. Life Cycle Assess. 17, 304–313. doi: 10.1007/s11367-011-0369-4
Hollmann, R. E. (2017). An aquaponics life cycle assessment: Evaluating an inovative method for growing local fish and lettuce. Dissertation/master’s thesis : University of Colorado Denver. https://dashboard.digital.auraria.edu/downloads/b580bca2-60c8-4eea-a715-8432296f5d01
Huijbregts, M. A. J., Hellweg, S., Frischknecht, R., Hendriks, H. W. M., Hungehbühler, K., and Hendriks, A. J. (2010). Cumulative energy demand as predictor for the environmental burden of commodity production. Environ. Sci. Technol. 44, 2189–2196. doi: 10.1021/es902870s
ISO 14040 (2006). Environmental management—life cycle assessment—principles and framework (ISO 14040). Geneva. Available at: https://www.iso.org/standard/37456.html (Accessed November 9, 2023).
ISO 14044 (2006). Environmental management—Life cycle assessment—Requirements and guidelines. International organisation for standardisation: Geneva, Switzerland.
ISO 14067 (2018). Greenhouse gases — Carbon footprint of products — Requirements and guidelines for quantification. International organisation for standardisation: Geneva, Switzerland.
Jaeger, C., Foucard, P., Tocqueville, A., Nahon, S., and Aubin, J. (2019). Mass balanced based LCA of a common carp-lettuce aquaponics system. Aquac. Eng. 84, 29–41. doi: 10.1016/j.aquaeng.2018.11.003
Kalvakaalva, R., Smith, M., Prior, S. A., Runion, G. B., Ayipio, E., Blanchard, C., et al. (2023). Life cycle assessment of a decoupled biofloc aquaponics facility across seasons. J. Clean. Prod. 429:139356. doi: 10.1016/j.jclepro.2023.139356
Masabni, J., and Niu, G. (2022). Aquaponics, Plant factory basics, applications and advances, (Elsevier), 167–180
Maucieri, C., Forchino, A. A., Nicoletto, C., Junge, R., Pastres, R., Sambo, P., et al. (2018). Life cycle assessment of a micro aquaponic system for educational purposes built using recovered material. J. Clean. Prod. 172, 3119–3127. doi: 10.1016/j.jclepro.2017.11.097
Maucieri, C., Nicoletto, C., Zanin, G., Xiccato, G., Borin, M., and Sambo, P. (2020). Composition and quality traits of vegetables grown in a low-tech aquaponic system at different fish stocking densities. J. Sci. Food Agric. 100, 4310–4318. doi: 10.1002/jsfa.10475
Michiels, F., Hubo, L., and Geeraerd, A. (2021). Why mass allocation with representative allocation factor is preferential in LCA when using residual livestock products as organic fertilizers. J. Environ. Manag. 297:113337. doi: 10.1016/j.jenvman.2021.113337
Miličić, V., Thorarinsdottir, R., Dos Santos, M., and Hančič, M. T. (2017). Commercial aquaponics approaching the European market: to consumers’ perceptions of aquaponics products in Europe. Water 9:20080. doi: 10.3390/w9020080
Nemecek, T., Jungbluth, N., Canals, L. M., and Schenck, R. (2016). Environmental impacts of food consumption and nutrition: where are we and what is next? Int. J. Life Cycle Assess. 21, 607–620. doi: 10.1007/s11367-016-1071-3
Pawlak, K., and Kołodziejczak, M. (2020). The role of agriculture in ensuring food security in developing countries: considerations in the context of the problem of sustainable food production. Sustainability 12:35488. doi: 10.3390/su12135488
Pinho, S. M., David, L. H., Garcia, F., Keesman, K. J., Portella, M. C., and Goddek, S. (2021). South American fish species suitable for aquaponics: a review. Aquac. Int. 29, 1427–1449. doi: 10.1007/s10499-021-00674-w
Ravani, M., Georgiou, K., Tselempi, S., Monokrousos, N., and Ntinas, G. (2023). Carbon footprint of greenhouse production in EU—how close are we to green deal goals? Sustain. For. 16:191. doi: 10.3390/su16010191
Stathopoulou, P., Tsoumalakou, E., Levizou, E., Vanikiotis, T., Zaoutsos, S., and Berillis, P. (2021). Iron and potassium fertilization improve rocket growth without affecting tilapia growth and histomorphology characteristics in aquaponics. Appl. Sci. 11:25681. doi: 10.3390/app11125681
Suhl, J., Dannehl, D., Baganz, D., Schmidt, U., and Kloas, W. (2018). An innovative suction filter device reduces nitrogen loss in double recirculating aquaponic systems. Aquac. Eng. 82, 63–72. doi: 10.1016/j.aquaeng.2018.06.008
Swiss Centre for Life Cycle Inventories (2023). Ecoinvent Database. Available at: https://ecoinvent.org/ (Accessed November 10, 2023).
United Nations Department of Economic and Social Affairs (2022). World population prospects 2022: Summary of results. New York. https://www.un.org/development/desa/pd/sites/www.un.org.development.desa.pd/files/wpp2022_summary_of_results.pdf
Valappil, G. (2021). Environmental and economic implications of small-scale Canadian aquaponics: A life cycle study. Dissertation/master’s thesis : University of Waterloo. http://hdl.handle.net/10012/16951
Vasdravanidis, C., Alvanou, M. V., Lattos, A., Papadopoulos, D. K., Chatzigeorgiou, I., Ravani, M., et al. (2022). Aquaponics as a promising strategy to mitigate impacts of climate change on rainbow trout culture. Animals 12:92523. doi: 10.3390/ani12192523
Verdoodt, S. (2018). Life cycle assessment of aquaponics: A case study in the urban environment. Dissertation/master’s thesis : Ghent University. https://lib.ugent.be/catalog/rug01:002785243
Keywords: product carbon footprint, aquaponics, greenhouse, vertical system, greenhouse gas emissions, sustainable production
Citation: Ravani M, Chatzigeorgiou I, Monokrousos N, Giantsis IA and Ntinas GK (2024) Life cycle assessment of a high-tech vertical decoupled aquaponic system for sustainable greenhouse production. Front. Sustain. 5:1422200. doi: 10.3389/frsus.2024.1422200
Edited by:
George Tsalidis, Brunel University London, United KingdomReviewed by:
Michael Martin, Swedish Environmental Research Institute (IVL), SwedenMugahid Elnour, KU Leuven, Belgium
Copyright © 2024 Ravani, Chatzigeorgiou, Monokrousos, Giantsis and Ntinas. This is an open-access article distributed under the terms of the Creative Commons Attribution License (CC BY). The use, distribution or reproduction in other forums is permitted, provided the original author(s) and the copyright owner(s) are credited and that the original publication in this journal is cited, in accordance with accepted academic practice. No use, distribution or reproduction is permitted which does not comply with these terms.
*Correspondence: Georgios K. Ntinas, Z250aW5hc0BlbGdvLmdy