- 1ifeu—Institut für Energie- und Umweltforschung Heidelberg gGmbH, Heidelberg, Germany
- 2Material Flow Management and Resource Economy, Institute IWAR, Technical University of Darmstadt, Darmstadt, Germany
- 3Fraunhofer Research Institution for Materials Recycling and Resource Strategies IWKS, Alzenau, Germany
Context: Bulk mineral waste materials such as construction and demolition waste are Germany’s largest waste stream. Despite the availability of high-quality recycling pathways such as road base layers, waste concrete is predominantly recycled into lower-quality recycling pathways like earthworks or unbound road construction. This is due to low demand for recycled aggregates in road base layers and frost protection layers, especially in public procurement.
Purpose: This study assesses the environmental consequences of increasing high-quality recycling of waste concrete in the near future to provide decision support for public procurement in Germany. The focus lies on climate change due to its importance for decision-makers. However, 17 other impact categories were assessed to avoid problem shifting.
Methods: Life cycle assessment (LCA) is applied with background data from ecoinvent 3.9.1. Impact assessment was conducted at midpoint level using IPCC 2021 and ReCiPe Midpoint (H). Foreground data were taken from literature and expert interviews. In line with the goal of this LCA, a consequential modeling approach was followed to account for changes in the material flow system. Substitution creates a cascade effect previously omitted in consequential LCA studies, in which lower quality recycling materials replace higher quality recycling materials in their respective utilization pathways.
Results and discussion: Increasing the high-quality recycling of waste concrete into road base layers causes a reduction in environmental impacts for all 18 impact categories, as it replaces natural aggregate and avoids backfilling of mixed mineral waste and excavated earth through substitution effects. Transport distances and ferrous metal recovery were identified as hot spots. Sensitivity analyses show that only transport is a significant issue.
Conclusion: Increasing the high-quality recycling of waste concrete in Germany is recommended in terms of environmental impacts. Lower-quality recycling is environmentally feasible only in cases where the avoided transport distances for natural aggregates and backfilling are significantly lower than the additional transport distances for high-quality recycling.
1 Introduction
Recycling of demolition waste in Germany is stagnating with high recycling rates but predominantly inferior recycling pathways (BBS, 2023). This is largely due to a lack of acceptance of recycled aggregates (RA) for high-quality applications such as concrete and road base layers (incl. frost protection layers)—especially in public procurement (Hinzmann et al., 2019; Mettke et al., 2019). Natural aggregates (NA) are the predominant type of aggregate used in high-quality applications. Increasing the demand for RA in public procurement for road base layers could increase the high-quality recycling of demolition waste and would also have an important signaling effect for the private construction industry. 60 Mio. t of construction and demolition waste (CDW) were produced in Germany in 2020, of which 47.3 Mio. t were recycled into RA for road construction, earthworks, and other applications. In addition, 129.2 Mio. t of waste soil and stones were produced, which are largely backfilled (BBS, 2023). The non-hazardous fraction of the CDW waste group is further distinguished by the European Waste Catalog (EWC) (European Commission, 2014) into the waste types waste concrete (EWC 17 01 01), bricks (EWC 17 01 02), tiles and ceramics (EWC 17 01 03), and mixtures of concrete, bricks, tiles, and ceramics (EWC 17 01 07)—from here on referred to as mixed mineral waste. The detailed allocation of the different CDW fractions to specific recycling pathways is not documented (BBS, 2023) and therefore the absolute potential for material flow shifts in this system is unknown.
Few life cycle assessment (LCA) studies on mineral waste management in Germany exist in peer-reviewed journals or in gray literature (Weil et al., 2006; Faulstich et al., 2017). A total of four consequential LCA (CLCA) studies assessing CDW management (Butera et al., 2015; Turk et al., 2015; Hossain et al., 2016; Iodice et al., 2021) were identified by two recent literature reviews (Dierks et al., 2021; Bayram and Greiff, 2023). None of these studies addresses the cascade effect modeled in the present study, instead assuming landfilling of the waste concrete as the alternative to high-quality recycling. This is not representative of the German context, where landfilling is almost nonexistent for concrete waste (BBS, 2023). CLCA studies for the German context are missing entirely, representing a major research gap for robust decision support. The goal of this life cycle assessment is to close this research gap by quantifying the environmental consequences, especially in regard to climate change, of substituting NA with RA made from waste concrete in unbound road base layers in Germany. As this shift in material flows can mainly be achieved by increasing the demand in public procurement, this life cycle assessment is aimed at public procurement bodies in Germany. It is intended to support decision-making regarding an increase in demand for RA in public procurement for high-quality utilization pathways, most notably unbound road base layers, in the near future. This life cycle assessment does not address individual procurement bodies, but rather the entirety of all public procurement for unbound road base layers in Germany. We further address decision-makers in private procurement, demolition and recycling companies and other stakeholders in the system of bulk mineral waste recycling. Policy makers area also addressed, as incentives, e.g., subsidizing mineral waste recycling or products containing recycled materials, and deterrents, e.g., taxing NAs or landfilling and backfilling of mineral waste, may have an important steering effect toward increased circular economy in the construction sector (Tošić et al., 2015).
2 Materials and methods
This section comprises the scope definition—including the definition of the function and the functional unit, the spatial, temporal and technological system boundaries, the data quality requirements, and the choice of impact categories—as well as the life cycle inventory analysis.
2.1 Functional unit and system boundary
The main function of the investigated system is the management (i.e., disposal or recycling) of waste concrete. This waste material is directly affected by an increase in demand for RA in base layers. As this LCA refers to one ton of waste material for illustration purposes, the functional unit is defined as the management of 1 t of waste concrete in Germany. The change in demand for RA is assumed to take place in the near future (ca. 2024–2029) and will be in effect for several years. The specific focus lies on the change in recycling pathways caused by a change in demand for high-quality RA, not an increase or decrease of total demand for aggregate. The reference flow is consequently defined as 1 t of waste concrete.
Because the objective of this LCA is decision support, we follow a consequential inventory modeling approach. The consequential modeling approach “attempts to provide information on the environmental burdens that occur, directly or indirectly, as a consequence of a decision (usually represented by changes in demand for a product)” (UNEP-SETAC, 2011). This means that the results do not reflect the environmental impacts of products (e.g., NA or RA), but rather the environmental consequences of changing the material flow system by substituting NA with RA in road base layers. In this modeling approach, allocation is avoided by means of substitution. Furthermore, background datasets representing marginal providers, i.e., technologies affected by a small-scale (relative to the affected market), long-term change in supply or demand, are used instead of average technology mixes.
The scope of this LCA includes the processes that are assumed to be affected by a change in the material flow system as visualized in Figure 1. We assume that the waste concrete is processed in a stationary processing plant to produce additional high-quality aggregate for use in unbound base layers. CDW is represented in this LCA by the two most common waste types in this category, i.e., waste concrete and mixed mineral waste. Soil and stones (EWC 17 05 04) are also included as they are affected by the cascade effect. We exclude bituminous mixtures (EWC 17 03 02), as this waste group is already effectively recycled into asphalt and road base layers. The supply of waste concrete and mixed mineral waste is assumed to be constrained by the demolition activity, i.e., no additional demolition will take place with the purpose of producing additional waste concrete. Therefore, the increase in demand for RA in road base layers will affect the other utilization pathways. The overall demand for aggregates is assumed to be unaffected by the choice for NA or RA, meaning that an increased demand for RA is always accompanied by an equivalent decrease in demand for NA.
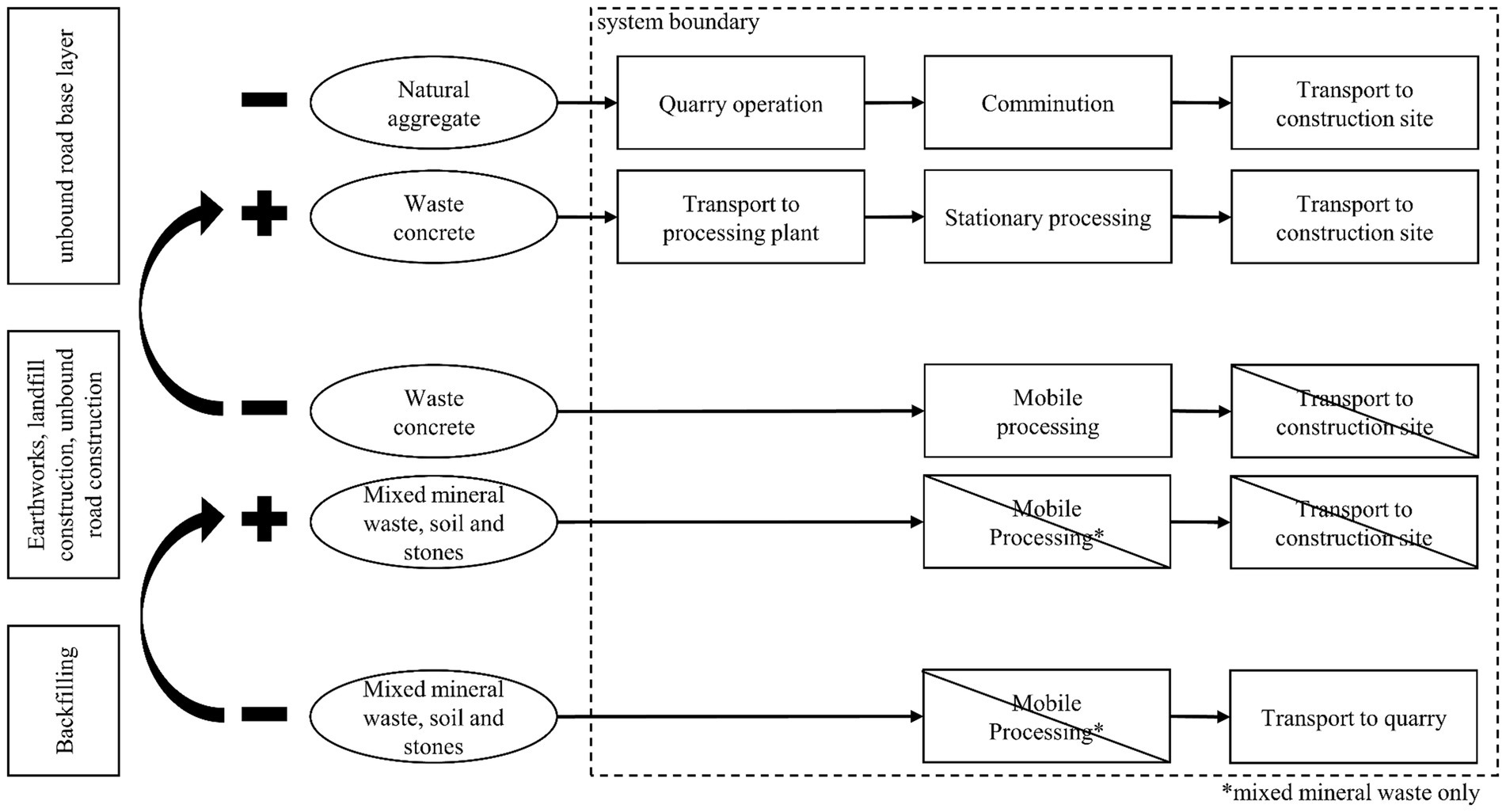
Figure 1. System boundary and changes (±) caused by the substitution of NA by RA made from waste concrete in road base layers. Crossed out processes are omitted.
As this LCA investigates changes in the material flow system of the construction and demolition waste group from lower to higher recycling pathways, the following processes are subject to change in each recycling pathway:
• Transport of the waste material from the waste generation site to the stationary processing plant or transport of the mobile processing plant to and from the waste generation site.
• Processing of the waste material in the stationary or mobile processing plant.
• Transport of the produced RA from stationary or mobile processing to the consuming construction site.
• Avoided production of substituted NA incl. transport from quarry to consuming construction site if applicable.
“Embodied impacts” of the waste material originating, e.g., from the life cycle of the demolished building or the demolition process are excluded from the system boundaries, because they are assumed to be unaffected by the change in recycling pathways. As CDW is typically handled on-site by excavators using screening buckets, a fine fraction is already separated on-site before transport or mobile processing. This fine fraction is assumed to be backfilled regardless of the recycling pathway of the remaining material and is therefore excluded from the system boundaries. We assume that the primary and secondary materials considered for each recycling pathway have similar technical properties and do not differ significantly in their handling during and after installation. This assumption is justified as recycling materials have to adhere to the same specifications as primary materials according to the German technical terms of delivery for construction material mixtures for the production of unbound layers in road construction (Forschungsgesellschaft für Straßen- und Verkehrswesen, 2020). For this reason, processes that occur after processing and transport to the construction site such as the installation into the structure, the use phase of the material in the structure and the disposal or further recycling at the subsequent (second) end-of-life are also excluded from the system boundaries. For the processing of concrete and mixed mineral waste for lower-quality recycling pathways (i.e., qualified applications in earthworks, landfill construction, unbound road construction or backfilling in quarries, sand and gravel pits and surface mines), processing in a mobile processing plant was assumed based on expert interviews.
Expert interviews confirmed that in each recycling pathway, only the recycled materials of highest quality available are used. This means that mixed mineral waste is generally not accepted for use in road base layers in Germany; although a mass fraction of up to 30% recycled bricks is technically and legally feasible (Forschungsgesellschaft für Straßen- und Verkehrswesen, 2020). Furthermore, we assume comparable bulk and compacted densities of the various mineral waste materials. Based on the assumed compacted densities of NA (2.56 t/m3) and RA (2.25 t/m3) as well as the assumption that their technical properties and further life cycles are nearly identical, 0.88 t of NA are substituted by 1 t of RA from waste concrete. During processing, around 4.8% mass losses occur in terms of ferrous metals for recycling and inert waste. This is a simplification, as the material compositions of waste concrete and mixed mineral waste vary. Based on these assumptions, about 0.84 t of NA are replaced by 1 t of waste concrete. We assume the following cascade effect, visualized in Figure 1, to take place:
• In the “road base layer” recycling pathway, 1 t of RA from waste concrete substitutes about 0.84 t of NA. This reduces the production and transport of NA. Instead, 1 t of additional waste concrete is transported from the waste generation site to the stationary processing plant, where it is processed before being transported to the construction site for use as road base layer material.
• Without this material flow shift, the waste concrete would be used in the medium-quality recycling pathway, i.e., for qualified earthworks, landfill construction and unbound road construction. Here, the mobile processing of waste concrete is reduced by 1 t. The unchanged demand for recycled material in this recycling pathway is expected to be satisfied by RA from mixed mineral waste as well as soil and stones. As a simplification, transport to the construction site is omitted in this recycling pathway based on the assumption that the transport distance is the same for all secondary materials. Furthermore, processing of mixed mineral waste as well as soil and stones is assumed to be the same for medium-quality recycling and backfilling and is therefore also omitted (see Figure 1).
• The 1 t of mixed mineral waste or soil and stones that is now used in the medium-quality recycling pathway does not have to be backfilled in quarries or surface mines. Simultaneously, the decreased production of NA reduces the demand for backfilling material. Expert interviews further revealed that quarries demand substantial acceptance fees for backfilling material, indicating an already low demand-to-supply ratio. We therefore assume that the secondary material is not replaced by primary material in this recycling pathway. The process of backfilling itself is omitted to avoid double counting, as avoided recultivation of quarries is already included in the avoided quarry operation process module.
This cascade effect is a simplification, as the recycling category “earthworks, landfill construction and unbound road construction” includes a broad spectrum of applications with different levels of technical requirements.
The net changes to the material flow system consist of (1) the reduction in production and transport of NA for road base layers, (2) the additional transport of waste concrete to the stationary processing plant, its processing and its transport to a construction site, (3) the avoided mobile processing of waste concrete, and (4) the avoided transport of mixed mineral waste as well as soil and stones to a backfilling site. Landfilling of bulk mineral waste in dedicated landfills is not considered to be affected by this cascade effect, as only 5.5% of CDW and 14.3% of soil and stones were landfilled in Germany in 2020 (BBS, 2023).
2.2 Data collection and data quality
The studied material flow changes in the foreground system are based on literature data and 12 semi-structured expert interviews with mineral waste recyclers, logistics experts, and researchers, which were analyzed in aggregated form to protect the experts’ personal and corporate information. Energy demands of the processing machines are based on data sheets as documented in Supplementary Tables 3, 4.
Background inventory data were taken from ecoinvent 3.9.1 (Wernet et al., 2016). The consequential system model (“Substitution, consequential, long-term”) was selected, as attributional background data is not appropriate for CLCA studies and can have a significant impact on the results (Weidema, 2017). Wherever possible, the background system data were selected to be representative of the German or European context. According to the goal of the study, the inventory data should be representative of processes in the near future. However, the background data of the transforming processes in the ecoinvent database relate exclusively to the past. This increases the uncertainty of the results, as is usual in LCA. Life cycle inventory (LCI) and life cycle impact assessment (LCIA) were calculated modularly using the ActivityBrowser (Steubing et al., 2020). The overall calculation and sensitivity analyses were then implemented via a spreadsheet.
2.3 Life cycle inventory analysis
This section addresses important choices made in regard to the LCI. The complete LCI data incl. parameters and data sources can be found in Supplementary Tables 2–5.
For lower-quality recycling pathways, diesel-powered mobile processing plants were chosen as the affected processing technology. Higher quality waste concrete recycling for road base layers can technically take place in semi-mobile processing plants. This is feasible for demolition projects with very large amounts of material for processing. Under our assumption that RA from concrete from large recycling projects is already used predominantly in road base layers, as it is likely cheaper to produce due to lower transport efforts than RA from smaller demolition projects, we assume this fraction of the waste concrete supply to be constrained. Stationary processing plants are generally larger with a greater throughput, may have more elaborate equipment such as wind sifters and allow for intermediate storage of RA, which makes quality control for high-quality RA applications more feasible. For this reason, stationary processing plants were selected as the affected technology for processing waste concrete in the recycling pathway for unbound base layers. Blengini and Garbarino (2010) similarly identified stationary processing plants as the producer of high-quality RA for road construction in an Italian context. The process chains of the two types of processing as visualized in Figure 2 as well as the transfer coefficients are based on Heyn and Mettke (2010) and were corroborated through expert interviews. The processing comprises comminution of the material, magnetic separation of iron scrap, manual screening for other impurities, and sieving. Stationary processing further utilizes a wind sifter. The product is a RA with a grain size distribution of 0/45 mm. Larger particles will be re-fed into the comminution. As the process modules for stationary and mobile CDW processing plants are largely the same, the two types of processing plants differ mainly in regard to their power source. According to expert interviews, stationary processing plants are either electrically operated stationary processing plants or diesel-powered mobile or semi-mobile plants operated in a fixed location. Newer stationary plants are more likely to be electrically powered. We therefore assumed electrically powered plants as the affected stationary processing plant type in Germany. The amounts of iron scrap and waste for disposal contained in the input waste streams vary significantly between demolition projects. As a simplification, we assume that the recovery rates for ferrous metal for each waste material do not change between recycling pathways. Expert interviews revealed that due to its economic feasibility and the potential hazard that reinforcement steel poses to construction equipment, the recovery of ferrous metals is necessary even if the recycled material is backfilled. Nevertheless, this assumption is assessed in a sensitivity analysis, as lower quality processing might constitute a slightly lower amount of iron scrap removed from the waste material. NA is assumed to be produced as gravel from crushed rock, which is commonly used in road base layers in Germany, whereas round gravel is usually reserved for concrete production.
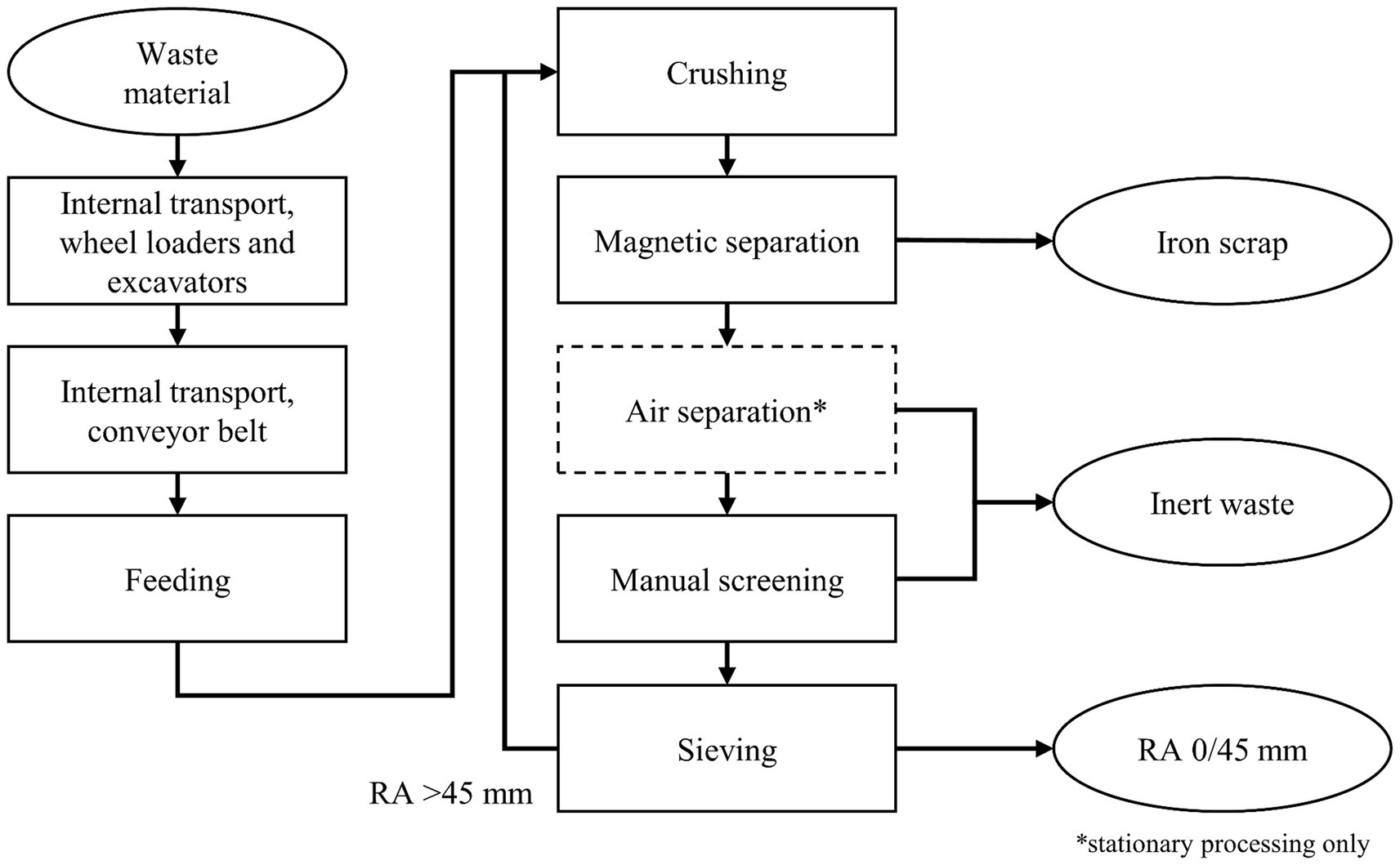
Figure 2. Process chain of stationary and mobile processing of waste concrete and mixed mineral waste based on Heyn and Mettke (2010).
The mode of transport for all materials in the foreground system is modeled as diesel trucks (16–32 tons, Euro 6), as they are unlikely to be replaced in the mid-term. It is possible that in certain cases with especially long distances, waste materials, RA or NA may be transported by train and/or ship in addition to lorry—however, these modes of transport are already rare and apply only in special cases. Due to a lack of robust literature data, the transport distances were estimated based on expert interviews. Transport distances can vary significantly between different regions in Germany, depending on, e.g., regional availability of rock and gravel deposits and the level of urbanization. Quarries and other backfilling sites are generally located outside of urban centers, while processing plants for mineral waste are usually located in the outskirts of urban centers and are therefore likely closer to both demolition and construction sites. The transport distance between the quarry and the construction site was estimated at an average of 30–100 km. This estimate is consistent with the distances modeled in LCA studies for other European countries such as France (Guignot et al., 2015; Chebbi et al., 2016), Italy (Borghi et al., 2018; Pantini et al., 2018), Denmark (Butera et al., 2015), Belgium (Di Maria et al., 2018), and Serbia (Marinković et al., 2010). The average transport distance from the waste generation site to the backfilling site was equally estimated at 30–100 km, with the same considerations regarding the distance as for quarries. This estimate is also corroborated by distances to backfilling sites or landfills used in LCAs for different European countries, e.g., Denmark (Butera et al., 2015), Portugal (Coelho and de Brito, 2012), and Belgium (Di Maria et al., 2018). However, other studies have estimated longer [e.g., Basti (2018) for Italy] or shorter distances [e.g., Faleschini et al. (2017) for Italy and Mercante et al. (2012) for Spain]. The average distances from the waste generation site to the treatment plant and from the treatment plant to the construction site where the RA is used were estimated at 20–30 km. The distance to the treatment plant is consistent with studies for France (Guignot et al., 2015; Chebbi et al., 2016), Italy (Blengini and Garbarino, 2010; Borghi et al., 2018), Denmark (Butera et al., 2015), and Belgium (Di Maria et al., 2018) with other studies for Italy (Blengini, 2009) and Spain (Mercante et al., 2012) using lower estimates. The distance from the processing plant to the construction site is consistent with studies for France (Guignot et al., 2015), Denmark (Butera et al., 2015), and Belgium (Di Maria et al., 2018), while Borghi et al. (2018) gave a lower estimate for the Lombardy region in Italy. An overview of the transport distances for mineral waste transport used in different LCA studies can be found in Supplementary Table 6. The baseline transport distances were modeled as 25 km each from the waste generation site of the concrete to the processing plant and further to the consuming construction site and as 50 km each from the quarry to the construction site and from the waste generation site of the mixture to the backfilling site. The transport distance for the mobile processing plant is assumed to be the same as for the distance from the waste generation site to the stationary processing plant (25 km). The mobile plant weighing 40 t is transported back and forth once per 10,000 t of material, resulting in 0.2 tkm per ton of processed material. This estimate based on expert interviews differs from the estimate of Blengini and Garbarino (2010), who estimated a distance 100 km for 5,000 t of material. Transport is a potential significant issue in LCA studies on mineral waste management according to the body of literature (Levis et al., 2011; Martínez et al., 2013; Miliutenko et al., 2013; Vossberg et al., 2014; Butera et al., 2015; Guignot et al., 2015; Wu et al., 2015; Borghi et al., 2018; Di Maria et al., 2018; Mah et al., 2018; Pantini et al., 2018; Wang et al., 2018; Yazdanbakhsh, 2018; Zhang et al., 2018, 2019; Amato et al., 2019; Fort and Cerny, 2020; Jain et al., 2020; Ram et al., 2020). The assumptions in regard to transport distances are therefore assessed in a sensitivity analysis.
3 Results
The LCIA was conducted at the midpoint for climate change (GWP100) using IPCC 2021 as well as for 17 additional impact categories using ReCiPe 2016 Midpoint (H) (Huijbregts et al., 2017). IPCC 2021 was chosen for climate change, because ReCiPe 2016 Midpoint (H) deviates slightly from the average characterization factors given in the IPCC Sixth Assessment Report (IPCC AR6 Working Group I, 2023). The optional normalization and weighting of impact categories was omitted. This article focuses on climate change because of its significance for decision-makers in public policy making and procurement in Germany. Results for all 18 impact categories can be found in Supplementary Table 7.
As illustrated in Figure 3, increasing the recycling quality of 1 t of waste concrete results in a net reduction of greenhouse gas (GHG) emissions of 12.3 kg CO2e. The recovery of ferrous scrap in stationary and mobile processing has the greatest impact on the GHG emissions (28.7 kg CO2e). However, since we assume that the transfer coefficient is the same for all recycling pathways, it is canceled out by the avoided ferrous metal recovery in mobile processing and not shown in Figure 3. The additional transport and stationary processing of waste concrete causes an additional 10.4 kg CO2e, which is offset by its avoided mobile processing (−2.3 kg CO2e), the avoided production and transport of NA (−11.5 kg CO2e) and the avoided transport of mixed mineral waste as well as soil and stones (−9.0 kg CO2e). The GHG emissions from stationary processing of waste concrete are largely determined by the electricity demand for crushing and sorting. On-site transport using excavators contributes much less to GHG emissions. The facility itself is not significant in terms of GHG emissions. For the avoided mobile processing of mixed mineral waste, it was assumed that the energy requirement is mainly supplied by diesel. Combustion and upstream processes of diesel fuel account for 82% of GHG emissions within mobile processing. Electricity demand, transport to the construction site and the life cycle of the mobile processing plant have a significantly lower share of the GHG emissions associated with mobile processing. The emissions from mobile processing are higher than those from stationary processing due to the use of diesel fuel compared to the mostly renewable marginal electricity providers in Germany. Emissions associated with diesel fuel demand are the largest contributor to GHG emissions from NA production (34%). Other significant sources of GHG emissions in NA production are factory buildings, machines and electricity demand. Overall, the GHG emissions from stationary and avoided mobile processing as well as for NA production are small in comparison to the emissions from transport.
Figure 4 shows the relative LCIA results for six exemplary impact categories, which were evaluated to identify possible problem shifting. Transport is the highest contributing factor in all six impact categories. NA production has large impacts in regard to mineral resource scarcity and land use. Stationary and mobile processing have relatively low contributions to all six impact categories. The difference in land use impacts for stationary and mobile processing of CDW stem only from upstream processes, as no direct land use elementary flows were modeled. Land use and transformation elementary flows for the facilities are the same for stationary and mobile processing plants, because the same background dataset was used. Thus, the reduction in land use impacts from avoided mobile processing is likely overestimated. This is not a potential significant issue, because land use contributions from stationary and mobile processing are small compared to the overall results. While the contributions vary across impact categories, environmental impacts are reduced due to the increase in high-quality recycling of waste concrete in all six impact categories. Thus, no problem shifting is expected.
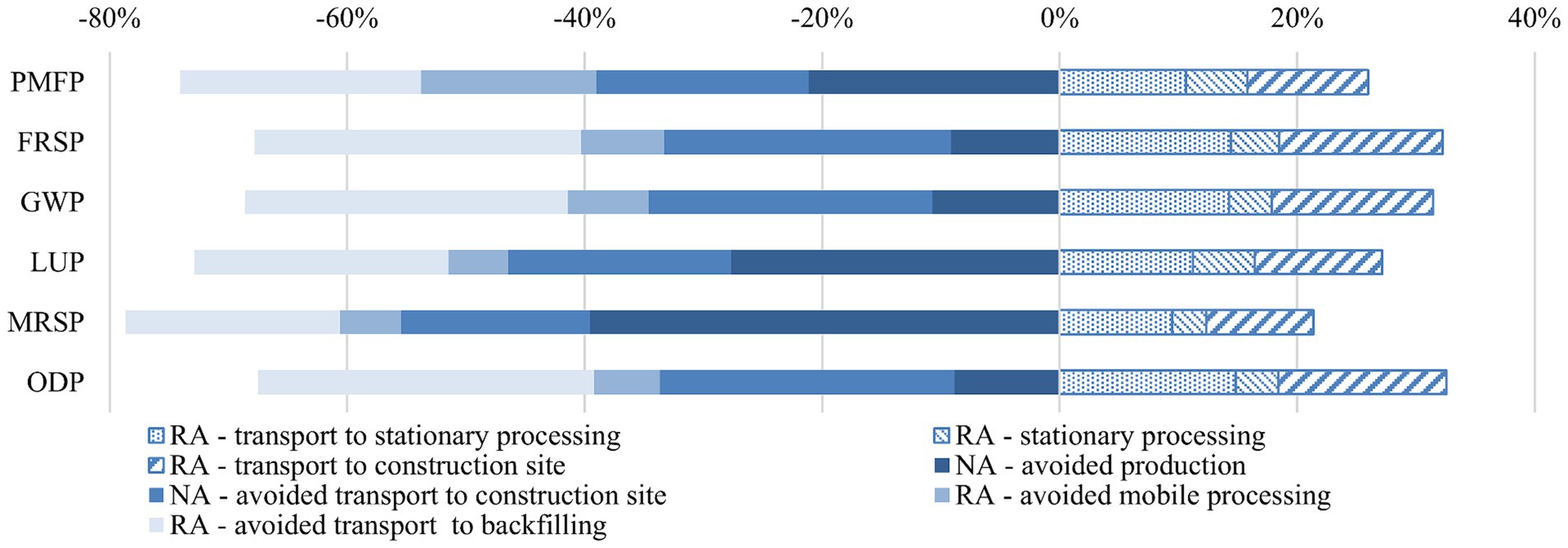
Figure 4. Relative LCIA results for six impact categories. PMFP, Particulate matter formation potential; FRSP, Fossil resource scarcity potential; GWP, Global warming potential; LUP, Land use potential; MRSP, Mineral resource scarcity potential; ODP, Ozone depletion potential; and TAP, Terrestrial acidification potential.
4 Discussion
This section discusses the robustness and possible limitations of the LCA results in terms of completeness, consistency and sensitivity. On this basis, conclusions and recommendations are formulated.
4.1 Completeness
According to our own assessment, all information and data relevant to the goal and the scope of the investigation are generally available. An unavoidable exception is the fact that there is no data for future processes. Therefore, data for existing technologies were used in the foreground and background models. As the analyzed system lies in the near future, this limitation is not considered to be critical in the present LCA. Potential leaching of heavy metals from NA and RA was not assessed. As summarized in Dierks et al. (2021), the following challenges exist in regard to assessing leaching in LCA: (a) NA may also be contaminated with heavy metals due to geogenic background pollution. The type and severity of the contamination depends on the respective type of mineral and its origin. (b) It is not possible to draw conclusions about the leaching behavior from the pollutants contained in a material. Leaching and the distribution of contaminants in the environment are dependent on a variety of factors such as pH, soil type, and material use—therefore, the same material may leach differently depending on the location and type of application. (c) Leaching can occur over the span of centuries. The quantification of such long-term environmental impacts represents a particular challenge in LCA. The issue of asbestos in CDW could not be considered due to lack of data. The impact categories human toxicity and ecotoxicity must be interpreted with these limitations in mind.
4.2 Consistency
Data collection was performed using a consistent spreadsheet and with a consistent level of detail. In line with the consequential modeling approach, allocation was avoided by substitution and marginal technology mixes were used. Background data were taken from a uniform source (ecoinvent 3.9.1). However, the datasets in ecoinvent 3.9.1 do not have a uniform temporal representativeness and level of detail. ReCiPe 2016 Midpoint (H) was consistently used for all impact categories with the exception of climate change, for which ReCiPe 2016 Midpoint (H) deviates from the characterization factor established in IPCC AR6 (IPCC AR6 Working Group I, 2023). GWP 100 was assessed using the IPCC 2021 LCIA method. The optional components ordering, weighing and normalization were omitted for all results. The modular impact assessment was carried out in a consistent spreadsheet. Overall, the assumptions, methods and data are in line with the objective and the scope of the study.
4.3 Sensitivity
A variety of parameters can potentially affect the conclusions if both their contribution to the LCIA results and their uncertainty are high. A summary of identified potential significant issues and our reasoning for or against sensitivity checks for each parameter can be found in Supplementary Table 8. Sensitivity analyses and checks were conducted on the following parameters: transport distances, ferrous metal recovery, substitution factor in road base layer and choice of stationary vs. mobile processing for high-quality RA from concrete. The two most relevant sensitivity analyses and checks, i.e., transport and ferrous metal recovery, are documented below. The other sensitivity analyses can be found in Supplementary Figures 2–4, as we found that those parameters are not significant issues.
4.3.1 Transport distances
The contribution of transport is greatest both in terms of the additional emissions due to the high-quality processing of waste concrete and in the avoided production of NA and the avoided backfilling of mixed mineral waste, if the recovery of ferrous metal is omitted. Dierks et al. (2021) found that in life cycle assessments on the subject of mineral waste management, transport often represents a significant issue. Transport distances for mineral waste, RA and NA in Germany are subject to a high degree of uncertainty and regional specificity. For this reason, a sensitivity analysis was conducted on the parameter transport distances. There are four relevant instances transport in the system under investigation, which are shown in Figure 1:
• Additional transport of waste concrete from the waste generation site to a stationary processing plant.
• Additional transport of RA from the stationary processing plant to the road construction site where it is utilized as base layer material.
• Avoided transport of mixed mineral waste or soil and stones from the was generation site to a quarry or surface mine for backfilling.
• Avoided transport of NA from a quarry to the road construction site where it would have been utilized as base layer material.
These transport distances do not contribute equally to the results, as there are some material losses during RA processing; hence only about 0.95 t of RA is transported from stationary processing to road construction per 1 t of waste concrete. As we assume a substitution factor of 0.88 t of NA per t of RA, the transport of NA from the quarry contributes even less per kilometer. For this reason, the break-even point between the additional and avoided transport distances is not fixed but decreases with total distances. For this sensitivity analysis, the combined additional transport distances (20, 30, 50, and 100 km) were therefore plotted against the combined avoided transport distances (0–100 km).
The results of the sensitivity analysis in Figure 5 show that increasing the demand for RA in base layers reduces GHG emissions irrespective of the avoided transport distances for combined additional distances of 20 km in the high-quality recycling route. For combined additional transport distances of 30, 50, and 100 km, GHG emissions are reduced if the avoided transport distances are at least ca. 5, 27, and 82 km, respectively. This shows that while transport distances should generally be classified as a significant issue, increasing the demand for high-quality RA will be advantageous in terms of GHG emissions unless the avoided transport distances are significantly lower (by about 18–25 km combined, depending on the total distances) than the additional ones. This is only feasible in regions with high availability of NA from crushed stone such as Stuttgart or Mannheim. For use in northern Germany, e.g., Hamburg, NA needs to be transported over long distances, which means that even long transport distances for RA are ecologically feasible.
4.3.2 Ferrous metal recovery
Figure 6 shows the results for the sensitivity analysis for decreased ferrous metal recovery during low-quality mobile processing. It compares the baseline results, which are set at −100% to indicate a reduction in emissions, with a scenario in which during mobile processing, 10% less ferrous metal is recovered compared to its stationary counterpart. This would improve the environmental benefits of increasing high-quality stationary recycling in most impact categories, as it would allow for the recovery and subsequent recycling of more ferrous scrap. Exceptions are carcinogenic human toxicity (HTP-C), ionizing radiation (IR) and marine eutrophication potential (MEP) with increases in net emissions of ca. 3,500, 700, and 110%, respectively. All of these increases are related to the increased production of recycled steel via the electric arc furnace route and reduced production of the marginal steel technology, the primary converter route. The results show that even a small change in this parameter can significantly impact the results. However, for most impact categories, this impact only increases the robustness of the original conclusion. Furthermore, recycling iron scrap after it has been removed from the mineral waste fraction is not strictly necessary for high-quality RA utilization and does not need to be a focus of this study. It is therefore not a significant issue within the scope of this LCA.
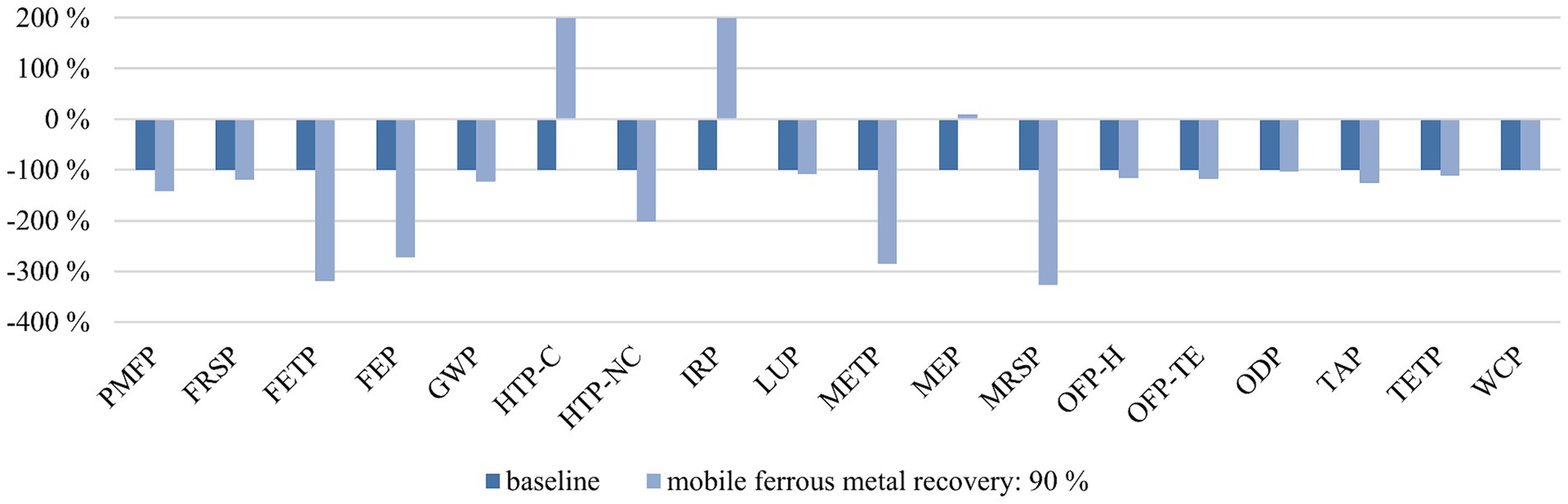
Figure 6. Sensitivity analysis: ferrous metal recovery (18 impact categories). Values for HTP-C (ca. 3,400%) and IRP (600%) for 90% ferrous metal recovery were cut off for improved readability.
4.4 Limitations
In principle, the results of this life cycle assessment can be classified as robust in terms of completeness, sensitivity, and consistency. When using the results of this study, it should be noted that two important issues, leaching and asbestos, could not be considered. We recommend addressing these issues on a case-by-case basis to supplement the results of this LCA for decision-making. The consistency check did not result in any limitations with regard to the conclusions and recommendations. The sensitivity check revealed that transport distances may be a significant issue in specific regions. The conclusions and recommendations were adjusted accordingly.
Demolition selectivity may be affected by increased demand for (and thus economic feasibility of) high-quality RA. This could not be addressed in this LCA. Further, soil and stones such as excavated earth could be processed in gravel processing plants to produce gravel and potentially clay as a raw material. The potential CO2 uptake that could possibly be achieved by carbonation due to enriching RA with CO2 is not addressed in this LCA, as it is not yet clear how much CO2 can be carbonated using different types of aggregates.
This study does not address the application of RA in recycled concrete. As there is not yet an established market for recycled concrete in Germany, it is currently not a significant utilization path for RA. However, this is likely to change in the future, with changes to the federal regulations for recycled concrete made in 2023 allowing significant amounts of RA type 2, i.e., RA including up to 30% masonry bricks and similar materials, which are currently utilized in low-quality recycling pathways. This could significantly affect the system of demolition waste recycling, with recycled concrete and base layer applications potentially competing for waste concrete. The environmental implications of the choice for and against the utilization of waste concrete in recycled concrete and road base layer, respectively, should be investigated in future studies.
4.5 Conclusion and recommendations
In this study, we conducted a consequential LCA on increasing high-quality recycling of waste concrete and, by extension, mixed mineral waste in Germany. This LCA is intended as decision support for decision-makers in aggregate procurement, especially in the public sector. To a lesser degree, it addresses recyclers, demolition companies and other stakeholders in the system of bulk mineral waste recycling as well as policy makers.
Per ton of material, the GHG savings from high-quality recycling of bulk mineral waste materials such as waste concrete and mixed mineral waste are small compared to the recycling of other materials. This is due to the fact that processing mineral waste and producing NA require much lower energy and material requirements than, e.g., pyrometallurgical or electrolytic processes. However, due to the large amounts of mineral waste produced in Germany, the high-quality recycling of demolition waste and other mineral waste and mineral by-products (e.g., steelmaking slag) can present an important building block on the way to climate neutrality. While expert interviews revealed that the use of RA in road base layers is still limited in Germany, there is no exact data on the share of waste concrete that is already utilized in this recycling pathway. We are therefore unable to exactly quantify the total potential for environmental impact reduction through this material flow shift.
Substituting NA with RA in unbound base layers and frost protection layers in Germany is advantageous in all 18 impact categories addressed in this study. Therefore, no problem shifting is expected with respect to the investigated impact categories. We therefore recommend considering RA in public and private procurement in most cases. Low-quality recycling is only feasible in terms of GHG emissions if the combined avoided transport distances are at least ca. 18–25 km shorter than the additional transport distances. This is unlikely to be the case for average distances in entire regions but could be the case for specific demolition projects located in regions where NA availability is high.
Data availability statement
The original contributions presented in the study are included in the article/Supplementary material; further inquiries can be directed to the corresponding author.
Author contributions
CD: Conceptualization, Data curation, Formal analysis, Visualization, Writing – original draft. TH: Data curation, Writing – review & editing. TM: Data curation, Writing – review & editing. VZ: Supervision, Writing – review & editing.
Funding
The author(s) declare that financial support was received for the research, authorship, and/or publication of this article. The research for this study was partly conducted within the research projects “WieBauin – Wiederverwendung Baumaterialien innovativ” (FKZ 033L209A) and “RessStadtQuartier – Urbanes Stoffstrommanagement: Instrumente für die ressourceneffiziente Entwicklung von Stadtquartieren” (FKZ 033W109A), both funded by the German Federal Ministry of Education and Research.
Acknowledgments
We would like to thank our colleague Joachim Reinhardt for his valuable insights. We further thank the experts from mineral waste recycling, logistics, and research who agreed to be interviewed for our research.
Conflict of interest
CD was employed by ifeu—Institut für Energie- und Umweltforschung Heidelberg gGmbH.
The remaining authors declare that the research was conducted in the absence of any commercial or financial relationships that could be construed as a potential conflict of interest.
Publisher’s note
All claims expressed in this article are solely those of the authors and do not necessarily represent those of their affiliated organizations, or those of the publisher, the editors and the reviewers. Any product that may be evaluated in this article, or claim that may be made by its manufacturer, is not guaranteed or endorsed by the publisher.
Supplementary material
The Supplementary material for this article can be found online at: https://www.frontiersin.org/articles/10.3389/frsus.2024.1417637/full#supplementary-material
References
Amato, A., Gabrielli, F., Spinozzi, F., Galluzzi, L. M., Balducci, S., and Beolchini, F. (2019). Strategies of disaster waste management after an earthquake: a sustainability assessment. Resour. Conserv. Recycl. 146, 590–597. doi: 10.1016/j.resconrec.2019.02.033
Basti, A. (2018). Sustainable management of debris from the L'Aquila earthquake: environmental strategies and impact assessment. Detritus 2:112. doi: 10.31025/2611-4135/2018.13661
Bayram, B., and Greiff, K. (2023). Life cycle assessment on construction and demolition waste recycling: a systematic review analyzing three important quality aspects. Int. J. Life Cycle Assess. 28, 967–989. doi: 10.1007/s11367-023-02145-1
BBS (2023). Mineralische Bauabfälle Monitoring 2020: Bericht zum Aufkommen und zum Verbleib mineralischer Bauabfälle im Jahr 2020, Bundesverband Baustoffe – Steine und Erden e. V., Kreislaufwirtschaft Bau.
Blengini, G. A. (2009). Life cycle of buildings, demolition and recycling potential: a case study in Turin, Italy. Build. Environ. 44, 319–330. doi: 10.1016/j.buildenv.2008.03.007
Blengini, G. A., and Garbarino, E. (2010). Resources and waste management in Turin (Italy): the role of recycled aggregates in the sustainable supply mix. J. Clean. Prod. 18, 1021–1030. doi: 10.1016/j.jclepro.2010.01.027
Borghi, G., Pantini, S., and Rigamonti, L. (2018). Life cycle assessment of non-hazardous construction and demolition waste (CDW) management in Lombardy region (Italy). J. Clean. Prod. 184, 815–825. doi: 10.1016/j.jclepro.2018.02.287
Butera, S., Christensen, T. H., and Astrup, T. F. (2015). Life cycle assessment of construction and demolition waste management. Waste Manag. 44, 196–205. doi: 10.1016/j.wasman.2015.07.011
Chebbi, W., Yazoghli-Marzouk, O., Dauvergne, M., Lumiere, L., and Jullien, A. (2016). Environmental assessment of EAF slag in different "end of 2nd life". Int. J. Adv. Mech. Civil Eng. 3, 65–70.
Coelho, A., and de Brito, J. (2012). Influence of construction and demolition waste management on the environmental impact of buildings. Waste Manag. 32, 532–541. doi: 10.1016/j.wasman.2011.11.011
Di Maria, A., Eyckmans, J., and van Acker, K. (2018). Downcycling versus recycling of construction and demolition waste: combining LCA and LCC to support sustainable policy making. Waste Manag. 75, 3–21. doi: 10.1016/j.wasman.2018.01.028
Dierks, C., Hagedorn, T., Campitelli, A., Bulach, W., and Zeller, V. (2021). Are LCA studies on bulk mineral waste management suitable for decision support? A critical review. Sustain. For. 13:4686. doi: 10.3390/su13094686
European Commission (2014). Commission decision—of 18 December 2014—amending decision 2000/532/EC on the list of waste pursuant to directive 2008/98/EC of the European Parliament and of the council—(2014/955/EU) (2014/955/EU).
Faleschini, F., Zanini, M. A., Hofer, L., Zampieri, P., and Pellegrino, C. (2017). Sustainable management of demolition waste in post-quake recovery processes: the Italian experience. Int. J. Disast. Risk Reduct. 24, 172–182. doi: 10.1016/j.ijdrr.2017.06.015
Faulstich, M., Flamm, M., Sauter, A., and Zeller, Z. (2017). Bewertung der Substitution von industriellen Nebenprodukten der Stahlerzeugung durch Primärrohstoffe beim Einsatz im Straßen- und Wegebaubau, Clausthal-Zellerfeld. Available at: http://www.fehs.de/pressebereich/ (Accessed September 18, 2017).
Forschungsgesellschaft für Straßen- und Verkehrswesen (2020). Technische Lieferbedingungen für Baustoffgemische zur Herstellung von Schichten ohne Bindemittel im Straßenbau: TL SoB-StB 20. 2020th Edn. Köln: FGSV Verlag GmbH.
Fort, J., and Cerny, R. (2020). Transition to circular economy in the construction industry: environmental aspects of waste brick recycling scenarios. Waste Manag. 118, 510–520. doi: 10.1016/j.wasman.2020.09.004
Guignot, S., and Touzé, S., Weid, F. von der, Ménard, Y., and Villeneuve, J. (2015). Recycling construction and demolition wastes as building materials: a life cycle assessment. J. Ind. Ecol. 19, 1030–1043. doi: 10.1111/jiec.12262
Heyn, S., and Mettke, A. (2010). Ökologische Prozessbetrachtungen—RC-Beton (Stofffluss, Energieaufwand, Emissionen), Brandenburgische Technische Universität Cottbus.
Hinzmann, M., Araujo Sosa, A., and Hirschnitz-Garbers, M. (2019). Stärkung der Kreislaufführung von mineralischen Baustoffen mittels freiwilliger Selbstverpflichtung: Akteursperspektiven auf Bedarfe und Optionen.
Hossain, M. U., Poon, C. S., Lo, I. M., and Cheng, J. C. (2016). Comparative environmental evaluation of aggregate production from recycled waste materials and virgin sources by LCA. Resour. Conserv. Recycl. 109, 67–77. doi: 10.1016/j.resconrec.2016.02.009
Huijbregts, M. A. J., Steinmann, Z. J. N., Elshout, P. M. F., Stam, G., Verones, F., Vieira, M., et al. (2017). ReCiPe2016: a harmonised life cycle impact assessment method at midpoint and endpoint level. Int. J. Life Cycle Assess. 22, 138–147. doi: 10.1007/s11367-016-1246-y
Iodice, S., Garbarino, E., Cerreta, M., and Tonini, D. (2021). Sustainability assessment of construction and demolition waste management applied to an Italian case. Waste Manag. 128, 83–98. doi: 10.1016/j.wasman.2021.04.031
IPCC AR6 Working Group I (2023). “The Earth’s energy budget, climate feedbacks and climate sensitivity” in Climate change 2021: The physical science basis: Working group I contribution to the sixth assessment report of the intergovernmental panel on climate change, Cambridge, Cambridge University Press. ed. Masson-Delmotte, V., pp. 923–1054.
Jain, S., Singhal, S., and Pandey, S. (2020). Environmental life cycle assessment of construction and demolition waste recycling: a case of urban India. Resour. Conserv. Recycl. 155:104642. doi: 10.1016/j.resconrec.2019.104642
Levis, J. W., Barlaz, M. A., Tayebali, A., and Ranjithan, S. R. (2011). Quantifying the greenhouse gas emission reductions associated with recycling hot mix asphalt. Road Mater. Pavement Des. 12, 57–77. doi: 10.1080/14680629.2011.9690352
Mah, C. M., Fujiwara, T., and Ho, C. S. (2018). Life cycle assessment and life cycle costing toward eco-efficiency concrete waste management in Malaysia. J. Clean. Prod. 172, 3415–3427. doi: 10.1016/j.jclepro.2017.11.200
Marinković, S., Radonjanin, V., Malešev, M., and Ignjatović, I. (2010). Comparative environmental assessment of natural and recycled aggregate concrete. Waste Manag. 30, 2255–2264. doi: 10.1016/j.wasman.2010.04.012
Martínez, E., Nuñez, Y., and Sobaberas, E. (2013). End of life of buildings: three alternatives, two scenarios. A case study. Int. J. Life Cycle Assess. 18, 1082–1088. doi: 10.1007/s11367-013-0566-4
Mercante, I. T., Bovea, M. D., Ibáñez-Forés, V., and Arena, A. P. (2012). Life cycle assessment of construction and demolition waste management systems: a Spanish case study. Int. J. Life Cycle Assess. 17, 232–241. doi: 10.1007/s11367-011-0350-2
Mettke, A., Filonenko, M., and Fischer, J. (2019). Ressourceneffizienz und Umweltkommunikation: Nutzung von mineralischen Sekundärrohstoffen am Beispiel des Landes Berlin. Available at: https://www.dbu.de/OPAC/ab/DBU-Abschlussbericht-AZ-32867_01-Hauptbericht.pdf (Accessed March 15, 2024).
Miliutenko, S., Björklund, A., and Carlsson, A. (2013). Opportunities for environmentally improved asphalt recycling: the example of Sweden. J. Clean. Prod. 43, 156–165. doi: 10.1016/j.jclepro.2012.12.040
Pantini, S., Borghi, G., and Rigamonti, L. (2018). Towards resource-efficient management of asphalt waste in Lombardy region (Italy): identification of effective strategies based on the LCA methodology. Waste Manag. 80, 423–434. doi: 10.1016/j.wasman.2018.09.035
Ram, V. G., Kishore, K. C., and Kalidindi, S. N. (2020). Environmental benefits of construction and demolition debris recycling: evidence from an Indian case study using life cycle assessment. J. Clean. Prod. 255:120258. doi: 10.1016/j.jclepro.2020.120258
Steubing, B., De, K. D., Haas, A., and Mutel, C. L. (2020). The activity browser—an open source LCA software building on top of the brightway framework. Softw. Impact. 3:100012. doi: 10.1016/j.simpa.2019.100012
Tošić, N., Marinković, S., Dašić, T., and Stanić, M. (2015). Multicriteria optimization of natural and recycled aggregate concrete for structural use. J. Clean. Prod. 87, 766–776. doi: 10.1016/j.jclepro.2014.10.070
Turk, J., Cotič, Z., Mladenovič, A., and Šajna, A. (2015). Environmental evaluation of green concretes versus conventional concrete by means of LCA. Waste Manag. 45, 194–205. doi: 10.1016/j.wasman.2015.06.035
UNEP-SETAC (2011). Global guidance principles for life cycle assessment databases: A basis for greener processes and products. UNEP-SETAC, Shonan Guidance Principles.
Vossberg, C., Mason-Jones, K., and Cohen, B. (2014). An energetic life cycle assessment of C&D waste and container glass recycling in Cape Town. S.A. Resour. Conserv. Recycl. 88, 39–49. doi: 10.1016/j.resconrec.2014.04.009
Wang, T., Wang, J., Wu, P., Wang, J., He, Q., and Wang, X. (2018). Estimating the environmental costs and benefits of demolition waste using life cycle assessment and willingness-to-pay: a case study in Shenzhen. J. Clean. Prod. 172, 14–26. doi: 10.1016/j.jclepro.2017.10.168
Weidema, B. P. (2017). Estimation of the size of error introduced into consequential models by using attributional background datasets. Int. J. Life Cycle Assess. 22, 1241–1246. doi: 10.1007/s11367-016-1239-x
Weil, M., Jeske, U., and Schebek, L. (2006). Closed-loop recycling of construction and demolition waste in Germany in view of stricter environmental threshold values. Waste Manag. Res. 24, 197–206. doi: 10.1177/0734242X06063686
Wernet, G., Bauer, C., Steubing, B., Reinhardt, J., Moreno-Ruiz, E., and Weidema, B. P. (2016). The ecoinvent database version 3 (part I): overview and methodology. Int. J. Life Cycle Assess. 21, 1218–1230. doi: 10.1007/s11367-016-1087-8
Wu, H., Duan, H., Wang, J., Wang, T., and Wang, X. (2015). Quantification of carbon emission of construction waste by using streamlined LCA: a case study of Shenzhen, China. J. Mater. Cycles Waste Manag. 17, 637–645. doi: 10.1007/s10163-015-0404-9
Yazdanbakhsh, A. (2018). A bi-level environmental impact assessment framework for comparing construction and demolition waste management strategies. Waste Manag. 77, 401–412. doi: 10.1016/j.wasman.2018.04.024
Zhang, C., Hu, M., Dong, L., Gebremariam, A., Miranda-Xicotencatl, B., Di Maio, F., et al. (2019). Eco-efficiency assessment of technological innovations in high-grade concrete recycling. Resour. Conserv. Recycl. 149, 649–663. doi: 10.1016/j.resconrec.2019.06.023
Keywords: life cycle assessment, consequential LCA, mineral waste, construction and demolition waste, recycled aggregate
Citation: Dierks C, Hagedorn T, Mack T and Zeller V (2024) Consequential life cycle assessment of demolition waste management in Germany. Front. Sustain. 5:1417637. doi: 10.3389/frsus.2024.1417637
Edited by:
Idiano D’Adamo, Sapienza University of Rome, ItalyReviewed by:
Asela Kulatunga, University of Exeter, United KingdomFlora Faleschini, University of Padua, Italy
Copyright © 2024 Dierks, Hagedorn, Mack and Zeller. This is an open-access article distributed under the terms of the Creative Commons Attribution License (CC BY). The use, distribution or reproduction in other forums is permitted, provided the original author(s) and the copyright owner(s) are credited and that the original publication in this journal is cited, in accordance with accepted academic practice. No use, distribution or reproduction is permitted which does not comply with these terms.
*Correspondence: Christian Dierks, christian.dierks@ifeu.de