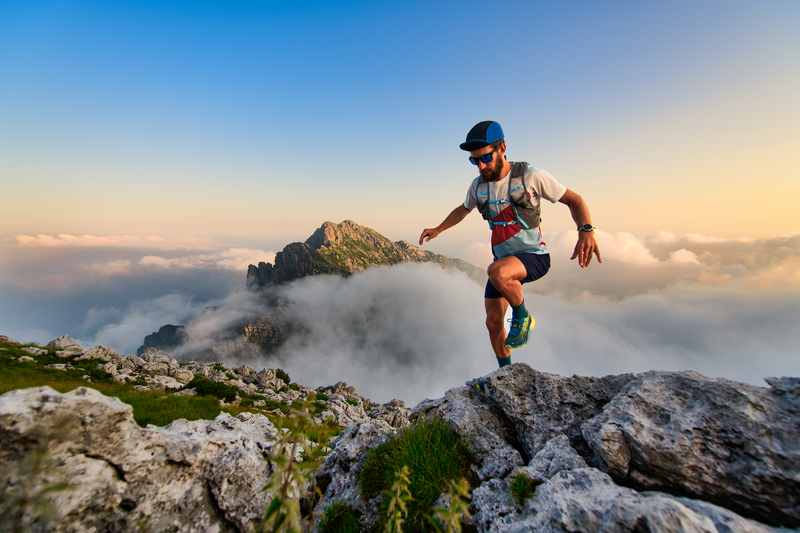
95% of researchers rate our articles as excellent or good
Learn more about the work of our research integrity team to safeguard the quality of each article we publish.
Find out more
ORIGINAL RESEARCH article
Front. Sustain. , 25 July 2024
Sec. Waste Management
Volume 5 - 2024 | https://doi.org/10.3389/frsus.2024.1417207
This article is part of the Research Topic Circular Economy and Food Systems: Challenges and Opportunities in Waste Management, with Reference to Developing Economies View all 8 articles
Valorization of peanut shells has recently gained prominence in the context of thermally converting agricultural waste into biochar, a carbon-rich byproduct with significant potential as a soil amendment. The present study delves into understanding the influence of slow (450°C and 500°C) and fast (550°C and 600°C) pyrolysis temperatures with a resident time of 60 and 30 minutes, respectively, on the physico-chemical properties of peanut shell biochar produced in a low-cost kiln. Results of the Scanning Electron Microscopy analysis revealed that increased pyrolysis temperature increased porosity and surface roughness with crystalline deposits. Thermogravimetric analysis showed that increased temperatures contributed to enhanced thermal stability but reduced biochar yield. Pyrolysis temperatures of 450, 500, 550, and 600°C exhibited 32.19, 29.13, 21.8, and 19.43 percent conversion efficiency with organic carbon content of 11.57, 6.48, 8.64, and 7.76 percent, respectively. The intensities of functional groups (C-H and C-O) declined, whereas the intensity of C=C and stable carbon content increased with the rise in temperatures. The concentrations of heavy metals in all biochar samples were below permissible limits outlined by international biochar initiatives. The study concluded that slow pyrolysis at 450°C for 60 minutes resident time is an ideal pyrolytic condition for producing peanut shell biochar in terms of qualitative and quantitative characteristics.
Soil is a dynamic realm that is pivotal in the natural geochemical cycle. It encompasses decaying organic matter, plant nutrient cycles, and carbon sequestration (Holatko et al., 2022). Soil organic matter (SOM) comprises soil organic carbon (SOC), which constitutes approximately 45–60% of the total mass of SOM (Lal, 2016). The maintenance of the SOC pool is paramount for optimal soil structure, aeration, water retention, nutrient retention, and rhizosphere processes (Chander et al., 2023). Soil management has a significant impact on whether carbon is released into the atmosphere or remains in the soil (Ray et al., 2022; Singh et al., 2022). Agricultural waste can be used to create customized carbon-based materials due to its high oxygenated functional groups and low condensation levels (Bai et al., 2020; Zhang et al., 2023).
Thus, regenerative agriculture practices such as the incorporation of crop residues into soil, mulching, composting, biochar production, and their subsequent application in the soil will increase the SOC and build resilience in the agriculture sector (Jat et al., 2022; Venkatesh et al., 2023). In this context, biochar, a carbon-rich product obtained through the pyrolysis of organic materials, has garnered attention for its myriad applications in soil amendment, carbon sequestration, and waste management (Murtaza et al., 2021; Hu et al., 2022; Kumar et al., 2024; Yadav et al., 2021). It is anticipated that chemical fertilizer usage will decrease when biochar is applied as a soil amendment because it can enhance soil quality (Doran and Zeiss., 2000; Das et al., 2021; Osman et al., 2022).
Globally, peanut cultivation covers 327 lakh hectares with a production of 539 lakh tonnes annually. India ranks first in the cultivation of peanuts (45.53 lakh hectares). It is the world’s second-largest producer (101 lakh tonnes) with a productivity of 1863 kg ha−1 (Crop Outlook Reports of Andhra Pradesh: Maize, 2022). Peanut shells, which account for 30% of the weight of peanuts, are expelled as waste during deshelling processing (Singh, 2004). Although the peanut industry produces approximately 11 million tonnes of waste (shells) annually, its potential uses remain largely untapped (Verheijen et al., 2010). It is currently used on a limited scale for producing value-added products viz., biodiesel, bioethanol, carbon nanosheets, and building material through industrialized processes (Duc et al., 2019; Zheng et al., 2023). The peanut shells have a unique composition of cellulose (45%), hemicellulose (6%), and lignin (36%), making them suitable for recycling and can be used as a soil amendment. However, due to high lignin content, peanut shells have a slow decomposition rate in the natural environment. Thus, producing biochar through pyrolysis using a low-cost kiln offers an effective solution for recycling peanut shells (Gupta et al., 2022; Kumar et al., 2023), which has demonstrated positive impacts on soil health, as evidenced by various studies (Omidi et al., 2017; Fall et al., 2018).
Pyrolysis techniques and residence time are crucial for the quantitative and qualitative aspects of biochar production (Tan et al., 2018; Moradi et al., 2019; Ong et al., 2021; Mukherjee et al., 2022; Joshi et al., 2023). Studies were conducted on producing biochar from peanut shells using different methods such as pyrolyzing units, muffle furnaces, fixed bed glass reactors, and microwave-based pyrolysis. However, more research is needed on the characterization of biochar produced from peanut shells using low-cost kilns and the economic feasibility of the process (Abbhishek et al., 2021). Slow pyrolysis performed under lower temperatures (<400–500°C) and with long contact times often results in a high yield of biochar (35%) (Meyer et al., 2011). Faster pyrolysis operates at higher temperatures (<800°C) and gives a high yield of combustible gases in relation to the solid biochar (12%) (Laird et al., 2009; Bruun, 2011; Abdullah et al., 2023).
Centralized peanut shell recycling procedures limit the scaling up of valorization and carbon sequestration potential of peanut shells, so the need for low-cost biochar production technology becomes imperative. The present prudent study uses an extensive range of analytical techniques viz., scanning electron microscopy coupled with energy diffraction (SEM–EDX), fourier transform infrared spectroscopy (FTIR), X-ray diffraction (XRD), thermogravimetric analysis (TGA) and elemental analysis characterize peanut shell biochar produced through fast and slow pyrolysis at different temperature in a low-cost pyrolytic kiln (Venkatesh et al., 2013; Pasumarthi et al., 2024) that functions based on direct up-draft principle.
The peanut shells used in this study were collected from the International Crops Research Institute for the Semi-Arid Tropics (ICRISAT) farm, located at the Patancheru campus (geographical coordinates: 17° 15’ N latitude and 77° 35′ E longitude). After collection, the shells underwent a meticulous cleaning process to remove any adhering dirt or foreign materials. They were stored in a shaded environment to preserve their integrity and moisture content until their use in biochar preparation.
An inexpensive, indigenously fabricated portable single-barrel kiln was used for biochar preparation. The kiln is 88 cm in length, 81 cm in circumference, and 58 cm in diameter. An opening with a lid for feeding the feedstock with a dimension of 31× 31 cm is provided on the upper surface of the kiln. In addition, to aid in the incinerating process, the bottom surface of the kiln has about 20 apertures with a diameter of 3.2 ± 0.1 cm (Pasumarthi et al., 2024). A wire mesh was placed in the bottom of the kiln, and the peanut shells were weighed and fed into the biochar kiln. Different pyrolytic temperatures and resident times were applied: slow pyrolysis at 450°C and 500°C for 60 min and fast pyrolysis at 550°C and 600°C for 30 min. The samples are abbreviated as follows: GB-450 (biochar produced at 450°C with 60 min resident time), GB-500 (biochar produced at 500°C with 60 min resident time), GB-550 (biochar produced at 550°C with 30 min resident time), and GB-600 (biochar produced at 600°C with 30 min resident time). The below formula was used to calculate the yield of the biochar produced. The process of the thermo-chemical conversion of peanut shells to biochar using a kiln is illustrated in Figure 1.
Figure 1. Schematic presentation of the operational pyrolysis process for biochar production. (A) Biomass feeding point; (B) Shade dried residues; (C) Bottom perforations; (D) ignition and fire point; (E) initial air flow; (F) Heat transfer process between hot gases and residues; (G) Hot gas exhaust.
WRB, weight of raw biomass; WBC, weight of biochar.
The analysis of biochar samples encompassed the determination of various parameters, including organic carbon -OC (Walkley-Black method), total nitrogen-N, phosphorus-P (Setter et al., 2020), potassium-K (Olsen and Sommers, 1982; Helmke and Sparks, 1996), sulfur-S (Tabatabai, 1996), boron-B (Keren, 1996), zinc (Zn), iron (Fe), copper (Cu), magnesium (Mg), sodium (Na), calcium (Ca), and manganese (Mn) (Lindsay and Norvell, 1978; Yu et al., 2019). The investigations followed standard procedures at the Charles Renard Analytical Laboratory (CRAL) at ICRISAT.
The biochar samples derived from peanut shells were analyzed using SEM and EDX. The Quanta FEG 250 SEM model from Eindhoven, Netherlands, was used with a resolution range capacity of a minimum of 1 nm to examine the surface morphology of biochar samples. The instrument supported with xT microscope control V6 2.8 software was used to investigate the pore size of the biochar samples.
The biochar samples were analyzed using Fourier transform infrared spectroscopy (FTIR; Shimadzu, Japan) to examine their surface chemistry or functional groups. The method employed was the KBr pellet technique, recording 50 scans per sample, and each spectrum had an infrared range of 4,000–400 cm−1 as per the protocol by Liu et al. (2015).
The peanut shell biochar samples were subjected to thermogravimetric analysis (TGA) using a thermogravimetric instrument (TGA-DSC, Model: Discovery SDT 650). The protocol employed for the TGA study was adopted from Kumar et al. (2019).
The crystalline constituents of the biochar samples were determined by XRD analysis using an X-ray diffractometer (Make: Bruker D8 Advance) at a diffraction angle of 10–80° (Meili et al., 2019).
In accordance with Jindo and Sonoki (2019), the biochar samples were subjected to the chemical oxidation method (Edinburgh stability tool) to test for stability. Using a TCN analyzer (Skalar PRIMACS SNC 100 Carbon/Nitrogen analyzer), the carbon content of the dried residue was determined using the Dumas dry combustion method. The following formula was used to determine the stable carbon.
The presence of heavy metals has been tested using Inductively Coupled Plasma Atomic Emission Spectroscopy (ICP-AES).
Statistical analysis such as standard deviation (SD), coefficient of variation (CV), and Standard error of the mean (SEM±) to estimate the significant difference between the treatments were performed using SPSS 17.0 version statistical tool.
Biochar was produced from peanut shells using a low-cost portable biochar Kiln through a slow and fast pyrolysis process. It was observed that the quantity of biochar produced was less under a fast pyrolysis process, i.e., 550°C and 600°C for 30 minutes, compared to a slow pyrolysis process, i.e., 450°C, 500°C, for 60 minutes. In a study conducted by Cheng et al. (2021), it was observed that the polygeneration process was applied to cotton stalk at six different pyrolysis temperatures, and the yield distributions of biochars, polylignous acids, and gas were investigated. As the pyrolysis temperature was increased from 300 to 500°C, the biochar yield decreased from 46.71 to 33.15%. The percentage of biochar yields obtained at 450°C, 500°C, 550°C, and 600°C pyrolytic temperatures are 32.19, 29.13, 21.8, and 19.43, respectively. In a study conducted by Sun et al. (2017), it was observed that an increase in pyrolysis temperature resulted in a decrease in biochar yield. The study aimed to determine the influence of pyrolysis temperature and residence time on the physiochemical characteristics of biochar. These findings are similar to a study conducted by Zhao et al. (2018). In a study conducted by Nazir et al. (2021) on peanut shell biochar production using a pyrolyzing unit, the yields were 41, 36, and 33% at 250°C, 400°C, and 500°C, respectively. According to Selvarajoo and Oochit (2020), biochar yield reduced from 54.83 to 26.67% as the pyrolysis temperature increased from 300 to 900°C. This phenomenon is due to the extensive decomposition of lignocellulosic components at higher temperatures, leading to a reduction in biochar yield. Therefore, it is recommended to use lower temperatures (slow pyrolysis) to achieve higher biochar yield.
The composition of biochar is greatly affected by the pyrolysis temperature; with higher temperatures, the intensity of certain elements reduces due to volatilization or transformation. The nitrogen content was found to decrease with an increase in temperature during both fast and slow pyrolysis methods; a similar trend was observed in a study on peanut shell biochar production at 250°C, 400°C, and 500°C using pyrolyzing units (Nazir et al., 2021). However, some elements, such as potassium, peaked at intermediate temperatures, suggesting complex interactions during biochar formation. This data is vital for understanding the nutrient profile of biochar and its potential application in soil amendment or other uses (Pariyar et al., 2020).
In a study conducted by Omotade et al. (2020), the effect of pyrolysis temperature on the nutrient content of biochar derived from corn cobs, poultry litter, cow dung, and peanut shells was investigated. The biochar was produced at different temperatures of 300°C, 400°C, 500°C, and 600°C with a residence time of 3 h, using a heating rate of 10°C per minute. The highest nutrient contents (N, P, K, Ca, Mg, and S) were observed in corn cob biochar prepared at the lowest temperature, i.e., 300°C. Results from the present study are in agreement with those of Omotade et al. (2020). It was observed that the nutrient content of the biochar prepared by fast pyrolysis at 600°C with a resident time of 30 min was higher compared to fast pyrolysis at 550°C except for OC, B, Cu, and Mn (Table 1). The fast pyrolysis process, having a temperature of 550°C and 600°C and a residence time of 30 min, yielded less biochar with less organic carbon (8.64 and 7.76%) than biochar derived at 450°C. It was evident from these results that both fast and slow pyrolysis methods lead to a decrease in yield and organic carbon content with an increase in temperature (Table 1). Biochar produced at 450°C, 500°C, 550°C, and 600°C has OC content of 11.57, 6.48, 8.64, and 7.76%, respectively.
Studies have shown that the fixed carbon content of biochar increases with pyrolysis temperature. However, high temperatures or fast pyrolysis processes can result in a loss of carbon (Sun et al., 2017; Dahal et al., 2018; Dai et al., 2019; Yuan et al., 2019). According to a recent meta-analysis study on biochar carbon content, it was found that biochar produced at a lower temperature has a higher organic carbon content, which is consistent with other studies that have reported similar findings (Wijitkosum and Jiwnok, 2019). This phenomenon can be attributed to the breakdown of aromatic rings at increased temperatures ranging from 500 to 600°C, leading to a reduction in organic carbon content. N, P, Ca, and Mg content decreases with increasing temperature, indicating potential volatilization or transformation of these elements at higher temperatures, whereas potassium content peaks at 500°C and decreases at 600°C. Elements such as sulfur (total-S), zinc (total-Zn), boron (total-B), iron (total-Fe), copper (total-Cu), manganese (total-Mn), and sodium (total-Na) also showed varied concentrations at different temperatures (Pariyar et al., 2020).
The biochar samples’ surface morphology and pore size have varied with temperature. The macropore size increased with an increase in temperature. Variations were observed in surface morphology and macropore size, as the peanut shell biomass has cellulose, hemicellulose, and lignin, which respond differently to increased temperature under anoxic conditions. The observations were similar to the findings from experiments by Tanquilut et al. (2019) and Woźniak et al. (2021). The study by Yaashikaa et al. (2020) found that the surface morphology of biochar samples varied depending on the pyrolysis method and temperature. The surface morphology and macropores of the biochar prepared under slow and fast pyrolysis conditions are shown in Figure 2. Peanut shell biochar exhibits a combination of oval to slightly irregular macropore shapes. At 450°C, the structure is slightly denser with fewer visible cavities, suggesting that the lower temperature has not fully degraded the peanut shells, resulting in a less porous biochar, indicative of a preliminary stage of pyrolysis where the organic components are beginning to break down. The surface topology becomes more intricate as the temperature increases to 500°C. There is an increased prevalence of interconnected macropores, suggesting an enhancement in biochar porosity. This temperature fosters a more evolved pyrolysis process, leading to a higher degree of carbonization and pore development (Liu et al., 2015). The biochar produced at 550°C (GB-550) showed an even more fragmented morphological characteristic. The diversity in the particle shapes ranged from slender shards to more substantial fragments, hinting at a differential degradation rate and further breakdown of the organic material in the peanut shells.
Figure 2. Scanning electron microscopy of peanut shell-derived biochar samples at different pyrolysis temperatures: 450 (GB-450), 500 (GB-500), 550 (GB-550), and 600 (GB-600).
At the highest temperature of 600°C, the biochar exhibited high porosity, with the pores appearing more irregular in dimension and varied in size. The crystalline depositions become more pronounced at this temperature, possibly because of the mineral content or residues solidifying after most volatile components have been expelled (Weidner et al., 2022). The pyrolysis temperature determines peanut shell biochar’s surface morphology and porosity. As the temperature increases, the biochar undergoes more extensive carbonization, enhancing porosity and distinct structural features. These observations underscore the significance of optimizing pyrolysis temperatures to tailor the properties of biochar for specific applications (Leng et al., 2021). The porosity of biochar increases with temperature, which can enhance its water retention capacity, serving as habitat for microbial communities and improve soil health. It was observed that fast pyrolysis with less resident time generated biochar with uniform oval-shaped and bigger macropores compared with biochar produced through slow pyrolysis with more resident time.
The FTIR provides insights into the functional groups present on the biochar surfaces. The presence of hydroxyl, carbonyl, and aromatic groups can be ascertained, allowing for an understanding of biochar’s chemical properties and potential interactions in environmental applications. The FTIR spectra (Figure 3) revealed that the peak near 2,920 cm−1 corresponding to aliphatic C–H stretching, became less pronounced with higher pyrolysis temperatures, indicating the reduction or elimination of aliphatic compounds, which is in accordance with the findings by Antonangelo et al. (2019). These results are similar to previous research findings on the pyrolysis of bamboo conducted by Zheng et al. (2017), Qin et al. (2020), and Hadey et al. (2022).
Figure 3. FTIR spectra of peanut shell biochar at different pyrolysis temperatures 450 (GB-450), 500 (GB-500), 550 (GB-550), and 600 (GB-600).
The intensity of peaks observed around 1700 cm−1 in the spectra for GB-450 and GB-500, which are attributed to the presence of carbonyl or carboxylic groups, decreased significantly as the temperature increased, which may signify a loss of more volatile components and decomposition of carboxylic materials. The high concentration of carbonyl functional groups in the peanut shell biochar implies that cellulose and hemicellulose have been broken down into volatile compounds. The results published by Sahoo et al. (2021) and Hadey et al. (2022) are in agreement with the findings of the present study. Peaks in the region of 1,600 cm−1 are associated with aromatic C=C stretching vibrations. These peaks retain their intensity or become slightly more defined during fast pyrolysis at 550°C, suggesting that the stabilization of aromatic structures during pyrolysis is in agreement with the results reported by Bayartsengel et al. (2021) on biochar produced from various biowastes and the findings of Reza et al. (2020) and Zhang et al. (2020).
As the temperature increased, the peak at 1030 cm−1, representing C-O stretching vibrations, appeared less intense. This suggests that the C-O groups had undergone thermal degradation. This finding is consistent with Chellappan et al. (2018) research, which also showed similar trends in the thermal decomposition of materials derived from biomass. The reduction in peaks associated with aliphatic C– H stretching with increased pyrolysis temperature suggests that dehydration and decarboxylation reactions are prevalent, leading to a more carbon-rich and less reactive biochar (Li and Chen, 2018). The persistence and slight enhancement of aromatic C=C structures at higher temperatures indicate the formation of a more stable carbon network, characteristic of the charring process that enhances the fixed carbon content of biochar (Qin et al., 2022). The changes observed in the FTIR results indicate organic compounds’ decomposition and transformation within the biochar.
Overall, the FTIR results of peanut shell biochar elucidate a decreasing trend in the functional group intensity with increasing pyrolysis temperatures except for C=C, which increased with increasing pyrolysis temperature. These changes depict the decomposition and transformation of the organic compounds within the biochar, leading to increased carbonization and aromaticity.
The XRD patterns of biochar produced from peanut shells at different temperatures and residence times showed crystalline phases. The presence of these crystalline phases, as well as inorganic minerals from the original organic material, can be detected using XRD (Bai et al., 2020). As shown in Figure 4, the detection of peaks ranging from 2ϴ ≈ 24o and 28o were predominantly linked to the crystalline configurations of calcite (CaCO3) and sylvite (KCl) (Pariyar et al., 2020). Peaks within the 50–75° range suggested the existence of silicates and quartz associated with Mg, Ca, and Mn. XRD analysis indicated various inorganic constituents in the biochar. The results were similar to those of other products of biomass carbonization, such as rose petals (IIkhtiarini and Tjahjanto, 2019) and eucalyptus wood (Fernandes and Mendes, 2020). However, the biochar prepared at a higher temperature (600°C) shows a reduction of wide peaks, which are noticed in the biochar prepared at 450°C. This indicates that the amorphous structure has been reduced by increasing the temperature. Across all pyrolysis temperatures, carbon consistently emerged as the significant component of biochar, aligning with the findings of Chen et al. (2009). The material degradation at higher temperatures and exposure to minerals improves peak distinction. The peak representing calcite (CaCO3) was intensified in slow pyrolysis at 500°C with a residence time of 60 min, and a similar pattern was observed in biochar prepared using fast pyrolysis at 600°C with a residence time of 30 min. The peak at two theta value of 42.5 represents the presence of Fe3O4 (Saeed et al., 2021), and the intensity decreased with an increase in temperature beyond 500°C, this might be due to destabilization and loss of oxygen at higher temperatures. This is in alignment with the elemental analysis of the present study in which Fe intensity decreased with increasing temperature. The peak at two theta value of 36.5, which was in biochar prepared at 450°C has disintegrated with an increase in temperature to 500°C and above.
Figure 4. X-ray diffractogram of biochar prepared from peanut shell biochar samples at different pyrolysis temperatures 450 (GB-450), 500 (GB-500), 550 (GB-550), and 600 (GB-600).
The EDX analysis results are given in Table 2 and Figure 5. The elements carbon (C), oxygen (O), potassium (K), magnesium (Mg), and calcium (Ca) are considered important minerals present in biochar for their use as soil amendment (Zaitun et al., 2022). The EDX results indicated that carbon was the dominant element, followed by O, N, Mg, and K, which was also observed in the study undertaken by Reza et al. (2023). No significant difference was observed in carbon weight and atomic percentages with slow and fast pyrolysis at varying temperatures. However, biochar prepared using fast pyrolysis at 550°C with 30 min of resident time has slightly higher carbon weight and atomic percentages, i.e., 89.2 and 92%, respectively. Biochar prepared using fast pyrolysis at 600°C has a higher content of N, O, Mg, and K than those produced at 450, 500, and 550°C. Zhao et al. (2018) showed that the properties of rapeseed stem biochar were significantly impacted by the pyrolysis conditions, such as temperature, residence time, and heating rate. The study reported that N, Mg, and K contents decreased as the temperature increased during slow and fast pyrolysis. However, the fast pyrolysis process at 550°C resulted in lower N content than the slow pyrolysis process (Table 2).
Figure 5. Electron dispersive X-ray (EDX) of biochar prepared from peanut shell biochar samples at different pyrolysis temperatures 450 (GB-450), 500 (GB-500), 550 (GB-550), and 600 (GB-600).
The thermogravimetric analysis (TGA) analysis of biochar derived from peanut shells at various pyrolysis temperatures (450–600°C) is shown in Figure 6. The consistent change in the weight (or weight loss) was observed at all four temperatures, which indicates the loss of bound moisture or some light volatile components that are consistent across the samples (Tomczyk et al., 2020; Das et al., 2021). The TGA curve of the biochar derived at 450°C and 500°C (slow pyrolysis) reveals that degradation starts at approximately 274.33°C (29.02% weight loss), 286.46°C (19.74% weight loss), and culminates at approximately 361.08°C and 358.77°C, respectively. The biochar produced at higher temperatures exhibited less percentage weight loss during thermal degradation (TGA) compared to those produced at low temperatures. This means that slow pyrolysis showed increased thermal resistance with increasing temperatures. On the other hand, the TGA (thermogravimetric analysis) curve of the biochar derived at 550°C, and 600°C (fast pyrolysis) showed that degradation started at approximately 366.69°C (30.96% weight loss), and 352.07°C (19.77% weight loss), and degradation process culminated at around 486.96°C, and 493.40°C, respectively. The thermal stability of biochar increased with higher pyrolysis temperatures, which align with the findings of Cárdenas et al. (2022). The weight loss percentage decreased as the pyrolysis temperature increased. The most stable biochar was produced at 500°C and 600°C with a residence time of 60 and 30 min, respectively. The results are in agreement with the findings by Leng et al. (2019), which indicate a significant change in the rate of weight loss around 119.13°C. The sample degradation has initiated at 362.07°C and terminated at 493.40°C in the case of the biochar produced at 600°C. Weight considerations showed a loss of approximately 1.61 mg, resulting in a pronounced percentage reduction of 30.96%. From the TGA graphs, it can be inferred that as the pyrolysis temperature increases, the stability of the biochar also increases. As the pyrolysis temperature increases during both slow and fast pyrolysis, the stability of biochar has increased, which was evident from the decrease in weight loss when heated to 1,200°C. It was observed that the thermal destabilization of slow pyrolyzed biochar initiated at low temperatures compared to biochar prepared above 500°C. This indicated the importance of temperature in achieving thermally stable biochar. Li and Chen (2018) conducted a study on the production of biochar from switchgrass, biosolids, and water oak, revealing the requirement of elevated combustion temperatures to achieve significant mass loss in biochar derived at higher pyrolysis temperatures.
Figure 6. TGA of biochar samples prepared from peanut shells at different pyrolysis temperatures, 450 (GB-450), 500 (GB-500), 550 (GB-550), and 600 (GB-600).
The results indicate a clear trend, where the percentage of stable carbon in biochar increases with the pyrolysis temperature (Figure 7). Stable carbon content is an important indicator of the biochar’s quality and its potential for long-term carbon sequestration in soil. The increased stable carbon content at higher temperatures suggests a higher degree of carbonization and the formation of aromatic carbon structures resistant to decomposition (Pasumarthi et al., 2024).
Figure 7. Influence of pyrolysis temperature on the stable carbon content of peanut shell biochar, bars on the column indicates standard error mean; alphabets with different letters on the column shows significant difference as per Duncan’s multiple range test.
The data presented in Table 3 indicates that the concentrations of copper (Cu), chromium (Cr), lead (Pb), and nickel (Ni) in peanut shell biochar increased with the pyrolysis temperature from 450°C to 600°C. Cadmium (Cd) was not detected in biochar prepared at any temperature. All heavy metal concentrations in the biochar are below the permissible limits set by the International Biochar Initiative (Nzediegwu et al., 2019). Therefore, the low levels of potentially toxic elements suggest that the biochar produced using peanut shells could be used as a soil amendment.
The production economics of biochar using a kiln is given in Table 4. The cost of six kilns is about 108.4 USD, which is inclusive of the drum, perforations, and handle fitting. The production economics was calculated considering 200 working days per annum and 100 kg biomass utilization per day. The conversion efficiency of biomass to biochar varies with temperature, highest at 450°C (32.19%) and lowest at 600°C (19.43%). The economic analysis indicates that producing biochar from peanut shells is most economically feasible at a pyrolysis temperature of 450°C, fetching the highest Benefit–Cost Ratio (BCR) of 1.51. As the temperature increases, there is a marked decline in profitability, primarily due to a decrease in conversion efficiency. Having the existing technologies to produce biofuels, carbon nanosheets, cellulolytic enzymes, and building materials, a significant quantity of peanut shells remains unutilized in a sustainable manner due to the lack of low-cost decentralized value-addition technologies. The present study stands unique as peanut shell biochar was produced using a low-cost pyrolytic kiln, which addresses multiple issues like deteriorating soil health, elevated greenhouse gas emissions, and societal perseverance towards biochar application. As per the computed economics of peanut shell biochar production in the present study, kiln-based biochar production at 450°C with 60 min of resident time is suitable for decentralized production in smallholder farmers’ fields.
The study stands unique for using a portable kiln for peanut shell biochar production at 450°C, 500°C, 550°C, and 600°C with 60 and 30 min of resident time, respectively, and estimating its economics. It highlights the critical role of standardizing the pyrolytic conditions to enhance the quality and yield of peanut shell biochar. Higher pyrolysis temperature was found to result in increased porosity, crystalline deposits close to the macropores, structural changes, and increased thermal stability. However, the increase in temperature inversely affected the biochar yield. The FTIR findings reflected peak shifts and alterations across various temperatures, which can be attributed to the thermal degradation and modification of cellulose, hemicellulose, and aliphatic chains. The functional groups C-H and C-O intensities have decreased, and the C=C intensities increased with the increase in temperature but declined at 600°C. The slow pyrolytic method of producing biochar from peanut shells at 450°C was found to be better in terms of quality (11.57% OC) and yield (32.19%). In a nutshell, the present investigation advocates for the practical application of optimized biochar production method at the farm level, taking into account both economic and practical aspects and suggesting additional field research to investigate the long-term carbon sequestration potential of peanut shell biochar.
Looking ahead, the research should focus on conducting long-term studies to explore the effects of biochar application on microbial diversity, plant health, and yield at the system level. Biochar, being a nature-based positive solution, needs to be assessed economically to cater to the process of curtailing carbon footprints and greenhouse gas emissions. The authors look forward to in-depth investigations on the role of catalysts in the optimization of the pyrolysis process, which will scale up biochar production for smallholder farms.
The original contributions presented in the study are included in the article/supplementary material, further inquiries can be directed to the corresponding author/s.
GS: Conceptualization, Investigation, Methodology, Project administration, Resources, Supervision, Visualization, Writing – original draft, Writing – review & editing. RP: Conceptualization, Data curation, Formal analysis, Methodology, Supervision, Validation, Visualization, Writing – original draft, Writing – review & editing. SK: Conceptualization, Formal analysis, Investigation, Methodology, Supervision, Validation, Visualization, Writing – original draft, Writing – review & editing. PC: Formal analysis, Project administration, Supervision, Validation, Writing – review & editing. SR: Writing – review & editing, Methodology, Conceptualization, Supervision, Visualization, Formal analysis, Validation. SM: Formal analysis, Validation, Writing – review & editing. AS: Formal analysis, Validation, Writing – review & editing. HS: Conceptualization, Methodology, Supervision, Writing – review & editing. MR: Formal analysis, Validation, Writing – review & editing. RS: Conceptualization, Formal analysis, Methodology, Supervision, Validation, Writing – review & editing. MJ: Resources, Writing – review & editing. AP: Writing – review & editing, Methodology, Conceptualization, Visualization.
The author(s) declare that no financial support was received for the research, authorship, and/or publication of this article.
We acknowledge the support of the Centralized sophisticated instrument facility, BITS-Pilani, K K BIRLA Goa campus, and ICRISAT-CRA Laboratory for analyzing the biochar samples.
The authors declare that the research was conducted in the absence of any commercial or financial relationships that could be construed as a potential conflict of interest.
All claims expressed in this article are solely those of the authors and do not necessarily represent those of their affiliated organizations, or those of the publisher, the editors and the reviewers. Any product that may be evaluated in this article, or claim that may be made by its manufacturer, is not guaranteed or endorsed by the publisher.
Abbhishek, K., Chander, G., Dixit, S., Kuttippurath, J., Singh, A., and Das, D. (2021). Legume biochar fertilizer can be an efficient alternative to compost in integrated nutrient management of paddy (Oryza sativa L.). J. Soil Sci. Plant Nutr. 21, 2673–2688. doi: 10.1007/s42729-021-00555-4
Abdullah, N., Taib, R. M., Aziz, N. S. M., Omar, M. R., and Disa, N. M. (2023). Banana pseudo-stem biochar derived from slow and fast pyrolysis process. Heliyon 9:e12940. doi: 10.1016/j.heliyon.2023.e12940
Antonangelo, J. A., Zhang, H., Sun, X., and Kumar, A. (2019). Physicochemical properties and morphology of biochars as affected by feedstock sources and pyrolysis temperatures. Biochar 1, 325–336. doi: 10.1007/s42773-019-00028-z
Bai, S., Wang, T., Tian, Z., Cao, K., and Li, J. (2020). Facile preparation of porous biomass charcoal from peanut shell as adsorbent. Sci. Rep. 10:15845. doi: 10.1038/s41598-020-72721-0
Bayartsengel, B., Erdene-Ochir, N., Battulga, S., Tyeliubek, S., Jantsanpurev, E., and Chuluun, B. (2021). Characterization of biochars produced from various biowastes. In 5th International Conference on Chemical Investigation and Utilization of Natural Resource (ICCIUNR-2021) 73–81
Bruun, E. W. (2011). Application of fast pyrolysis biochar to a loamy soil-effects on carbon and nitrogen dynamics and potential for carbon sequestration. Eur. J. Soil Sci. 62, 581–589. doi: 10.1111/j.1365-2389.2011.01377.x
Cárdenas, R., Palma-Ramírez, D., Flores-Vela, A. I., Domínguez-Crespo, M. A., Torres-Huerta, A. M., Dorantes-Rosales, H., et al. (2022). Valorization of sawdust biomass for biopolymer extraction via the green method: comparison with conventional processes. Int. J. Energy Res. 46, 20279–20302. doi: 10.1002/er.8112
Chander, G., Singh, A., Abbhishek, K., Whitbread, A. M., Jat, M. L., Mequanint, M. B., et al. (2023). Consortium of management practices in long-run improves soil fertility and carbon sequestration in drylands of semi-arid tropics. Int. J. Plant Prod. 17, 477–490. doi: 10.1007/s42106-023-00249-0
Chellappan, S., Nair, V., Sajith, V., and Aparna, K. (2018). Synthesis, optimisation and characterisation of biochar based catalyst from sawdust for simultaneous esterification and transesterification. Chin. J. Chem. Eng. 26, 2654–2663. doi: 10.1016/j.cjche.2018.02.034
Chen, X., Sun, X., Jiang, D., Niu, A., Yu, Z., Liu, L., et al. (2009). A two-step hydrothermal synthesis approach to monodispersed colloidal carbon spheres. Res. Lett. 4, 971–976. doi: 10.1007/s11671-009-9343-5
Cheng, J., Hu, S., Sun, G., Geng, Z., and Zhu, M. (2021). The effect of pyrolysis temperature on the characteristics of biochar, pyroligneous acids, and gas prepared from cotton stalk through a polygeneration process. Ind. Crop. Prod. 170:113690. doi: 10.1016/j.indcrop.2021.113690
Crop Outlook Reports of Andhra Pradesh: Maize, (2022). Acharya N.G. Ranga agricultural university., (2022) crop outlook reports of Andhra Pradesh: Maize – January to December 2022. ANGRAU, Lam, Guntur
Dahal, R. K., Acharya, B., and Farooque, A. (2018). Biochar: a sustainable solution for solid waste management in agro-processing industries. Biofuels 12, 237–245. doi: 10.1016/B978-0-443-15206-1.00005-0
Dai, Y., Zhang, N., Xing, C., Cui, Q., and Sun, Q. (2019). The adsorption, regeneration and engineering applications of biochar for removal organic pollutants: a review. Chemosphere 223, 12–27. 4.2. doi: 10.1016/j.chemosphere.2019.01.161
Das, O., Mensah, R. A., George, G., Jiang, L., Xu, Q., Neisiany, R. E., et al. (2021). Flammability and mechanical properties of biocare produced in different pyrolysis reactors. Biomass Bioenergy 152:106197. doi: 10.1016/j.biombioe.2021.106197
Doran, J. W., and Zeiss, M. R. (2000). Soil health and sustainability: managing the biotic component of soil quality. Appl. Soil Ecol. 15, 3–11. doi: 10.1016/S0929-1393(00)00067-6
Duc, P. A., Dharanipriya, P., Velmurugan, B. K., and Shanmugavadivu, M. (2019). Groundnut shell -a beneficial bio-waste. Biocatal. Agric. Biotechnol. 20:101206. doi: 10.1016/j.bcab.2019.101206
Fall, D., Bakhoum, N., Fall, F., Diouf, F., Ndiaye, C., Faye, M. N., et al. (2018). Effect of peanut shell amendment on soil properties and growth of Senegalia senegal (L.) Britton, Vachellia seyal (Delile) P. Hurter, and Prosopis juliflora (Swartz) DC seedlings in salt-affected soils. Ann. For. Sci. 75, 1–11. doi: 10.1007/s13595-018-0714-x
Fernandes, B., and Mendes, K. F. (2020). Impact of pyrolysis temperature on the properties of Eucalyptus wood-derived biochar. Materials 13, 1–13. doi: 10.3390/ma13245841
Gupta, M., Savla, N., Pandit, C., Pandit, S., Gupta, P. K., Pant, M., et al. (2022). Use of biomass-derived biochar in wastewater treatment and power production: a promising solution for a sustainable environment. Sci. Total Environ. 825:153892. doi: 10.1016/j.scitotenv.2022.153892
Hadey, C., Allouch, M., Alami, M., Boukhlifi, F., and Loulidi, I. (2022). Preparation and characterization of biochars obtained from biomasses for combustible briquette applications. Sci. World J. 2022, 1–13. doi: 10.1155/2022/2554475
Helmke, P. A., and Sparks, D. (1996). “Lithium, sodium, potassium, rubidium, and cesium” in Methods of soil analysis: Part 3—Chemical methods. ed. D. L. Sparks (Madison, WI: SSSA and ASA), 551–574.
Holatko, J., Hammerschmiedt, T., Latal, O., Kintl, A., Mustafa, A., Baltazar, T., et al. (2022). Deciphering the effectiveness of humic substances and biochar modified digestates on soil quality and plant biomass accumulation. Agronomy 12:1587. doi: 10.3390/agronomy12071587
Hu, S., Cheng, J., Wang, W., Zhu, Y., Kang, K., Zhu, M., et al. (2022). Preparation and analysis of pyroligneous liquor, charcoal and gas from lacquer wood by carbonization method based on a biorefinery process. Energy 239:121918. doi: 10.1016/j.energy.2021.121918
Nzediegwu, C., Prasher, S., Elsayed, E., Dhiman, J., Mawof, A., Patel, R., et al. (2019). Effect of biochar on heavy metal accumulation in potatoes from wastewater irrigation. Journal of environmental management 232, 153–164.
IIkhtiarini, R. T., and Tjahjanto, S. T. (2019). The effect of parameter combinations (carbonization temperature chemical activator) on degree of graphitization, aromaticity, and Fungsional Group of Rose Petal (Rosa sp) based-activated carbon. Materials Sci. Engin. 546, 1–8. doi: 10.1088/1757-899X/546/2/022007
Jat, M. L., Chakraborty, D., Ladha, J. K., Parihar, C. M., Datta, A., Mandal, B., et al. (2022). 4.4 carbon sequestration potential, challenges, and strategies for climate action in smallholder agricultural systems of South Asia. Crop Environ. 1, 86–101. doi: 10.1016/j.crope.2022.03.005
Jindo, K., and Sonoki, T. (2019). Comparative assessment of biochar stability using multiple indicators. Agronomy 9:254. doi: 10.3390/agronomy9050254
Joshi, M., Bhatt, D., and Srivastava, A. (2023). Enhanced adsorption efficiency through biochar modification: a comprehensive review. Ind. Eng. Chem. Res. 62, 13748–13761. doi: 10.1021/acs.iecr.3c02368
Keren, R. (1996). “Boron” in Methods of soil analysis, Part 3–Chemical Methods. ed. D. L. Sparks (Madison, WI, USA: SSSA and ASA), 603–626.
Kumar, N. V., Sawargaonkar, G. L., Rani, C. S., Pasumarthi, R., Kale, S., Ram Prakash, T., et al. (2024). Harnessing the potential of pigeonpea and maize feedstock biochar for carbon sequestration, energy generation, and environmental sustainability. Bioresour. Bioprocess. 11:5. doi: 10.1186/s40643-023-00719-3
Kumar, N. V., Sawargaonkar, G. L., Rani, C. S., Singh, A., Prakash, T. R., Triveni, S., et al. (2023). Comparative analysis of Pigeonpea stalk biochar characteristics and energy use under different biochar production methods. Sustain. For. 15:14394. doi: 10.3390/su151914394
Kumar, M., Upadhyay, S. N., and Mishra, P. K. (2019). A comparative study of the thermochemical characteristics of lignocellulosic biomasses. Bioresource Technol. Rep. 8:100186. doi: 10.1016/j.biteb.2019.100186
Laird, D. A., Brown, R. C., Amonette, J. E., and Lehmann, J. (2009). Review of the pyrolysis platform for coproducing bio-oil and biochar. Biofuels Bioprod. Biorefin. 3, 547–562. doi: 10.1002/bbb.169
Lal, R. (2016). Biochar and soil carbon sequestration. Agricultural and environmental applications of biochar. Advances Barriers 63, 175–197. doi: 10.2136/sssaspecpub63.2014.0042.5
Leng, L., Huang, H., Li, H., Li, J., and Zhou, W. (2019). Biochar stability assessment methods: a review. Sci. Total Environ. 647, 210–222. doi: 10.1016/j.scitotenv.2018.07.402
Leng, L., Xiong, Q., Yang, L., Li, H., Zhou, Y., Zhang, W., et al. (2021). An overview of engineering the surface area and porosity of biochar. Sci. Total Environ. 763:144204. doi: 10.1016/j.scitotenv.2020.144204
Li, S., and Chen, G. (2018). Thermogravimetric, thermochemical, and infra-red spectral characterisation of feedstocks and biochar derived at different pyrolysis temperatures. Waste Manag. 78, 198–207. doi: 10.1016/j.wasman.2018.05.048
Lindsay, W. L., and Norvell, W. A. (1978). Development of a DTPA soil test for zinc, Iron, manganese, and copper. Soil Sci. Soc. Am. J. 42, 421–428. doi: 10.2136/sssaj1978.03615995004200030009x
Liu, Y., He, Z., and Uchimiya, M. (2015). Comparison of biochar formation from various agricultural by-products by FTIR spectroscopy. Mod. Appl. Sci. 9:4. doi: 10.5539/mas.v9n4p246
Meili, L., Godoy, R. P. S., Soletti, J. I., Carvalho, S. H. V., Ribeiro, L. M. O., Silva, M. G. C., et al. (2019). Cassava (Manihot esculenta Crantz) stump biochar: physical/chemical characteristics and dye affinity. ChemEng Commun. 206, 829–841. doi: 10.1080/00986445.2018.1530991
Meyer, S., Glaser, B., and Quicker, P. (2011). Technical, economical, and climate-related aspects of biochar production technologies: a literature review. Environ. Sci. Technol. 45, 9473–9483. doi: 10.1021/es201792c
Moradi, F., Moosavi, A. A., and Baghernejad, M. (2019). Determining the organochemical composition of sugarcane bagasse-derived biochar as a function of pyrolysis temperature using proximate and Fourier transform infrared red. J. Therm. Anal. Calorim. 138, 331–342. doi: 10.1007/s10973-019-08186-9
Mukherjee, A., Patra, B. R., Podder, J., and Dalai, A. K. (2022). Synthesis of biochar from lignocellulosic biomass for diverse industrial applications and energy harvesting: effects of pyrolysis conditions on the physicochemical properties of biochar. Front. Materials 9:870184. doi: 10.3389/fmats.2022.870184
Murtaza, G., Ditta, A., Ullah, N., Usman, M., and Ahmed, Z. (2021). Biochar for managing nutrient-impaired and metal-contaminated soils: preparation, applications, and prospects. J. Soil Sci. Plant Nutr. 21, 2191–2213. doi: 10.1007/s42729-021-00514-z
Nazir, A., Laila, U.-E., Bareen, F.-E., Hameed, E., and Shafiq, M. (2021). Sustainable Management of Peanut Shell through biochar and its application as soil ameliorant. Sustain. For. 13:13796. doi: 10.3390/su132413796
Olsen, S. R., and Sommers, L. E. (1982). “Phosphorus” in Methods of soil analysis, vol. 9. 2nd ed. Agronomy Monogr (Madison, WI, USA: SSSA, ASA and SSSA), 403–430.
Omidi, J., Abdolmohammadi, S., Hatamzadeh, A., and Khomami, A. M. (2017). Application of peanut shell composts in replacement with peat on growth indices and physical and chemical properties of violet growth media (Viola spp.) in outdoorenvironments. Front. Microbiol. 3, 68–72. doi: 10.11648/j.fem.20170305.11
Omotade, İ., Momoh, S., Oluwafemi, B., and Agboola, E. (2020). Comparative analysis of nutrients composition in biochar produced from different feedstocks at varying pyrolysis temperature. Environ. Res. Technol. 3, 64–70. doi: 10.35208/ert.747833
Ong, H. C., Yu, K. L., Chen, W. H., Pillejera, M. K., Bi, X., Tran, K. Q., et al. (2021). Variation of lignocellulosic biomass structure from torrefaction: a critical review. Renew. Sust. Energ. Rev. 152:111698. doi: 10.1016/j.rser.2021.111698
Osman, A. I., Fawzy, S., Farghali, M., El-Azazy, M., Elgarahy, A. M., Fahim, R. A., et al. (2022). Biochar for agronomy, animal farming, anaerobic digestion, composting, water treatment, soil remediation, construction, energy storage, and carbon sequestration: a review. Environ. Chem. Lett. 20, 2385–2485. doi: 10.1007/s10311-022-01424-x
Pariyar, P., Kumari, K., Jain, M. K., and Jadhao, P. S. (2020). Evaluation of changes in biochar properties derived from different feedstocks and pyrolysis temperatures for environmental and agricultural applications. Sci. Total Environ. 713:136433. doi: 10.1016/j.scitotenv.2019.136433
Pasumarthi, R., Sawargaonkar, G., Kale, S., Kumar, N. V., Choudhari, P. L., Singh, R., et al. (2024). Innovative bio-pyrolytic method for efficient biochar production from maize and pigeonpea stalks and their characterization. J. Clean. Prod. 448:141573. doi: 10.1016/j.jclepro.2024.141573
Qin, Y., Yin, X., Xu, X., Yan, X., Bi, F., and Wu, W. (2020). Specific surface area and electron donating capacity determine biochar's role in methane production during anaerobic digestion. Bioresour. Technol. 303:122919. doi: 10.1016/j.biortech.2020.122919
Qin, F., Zhang, C., Zeng, G., Huang, D., Tan, X., and Duan, A. (2022). Lignocellulosic biomass carbonisation for biochar production and biochar reactivity characterisation. Renew. Sust. Energ. Rev. 157:112056. doi: 10.1016/j.rser.2021.112056
Ray, R. L., Khan, N., Abeysingha, N. S., Farooq, S., Singh, S. K., and Umair, M. (2022). Quantifying surface soil organic carbon distribution globally during the COVID-19 pandemic using satellite data. Geocarto Int. 37, 12149–12170. doi: 10.1080/10106049.2022.2063412
Reza, M. S., Afroze, S., Bakar, M. S., Saidur, R., Aslfattahi, N., Taweekun, J., et al. (2020). Biochar characterisation of invasive Pennisetum purpureum grass: effect of pyrolysis temperature. Biochar 2, 239–251. doi: 10.1007/s42773-020-00048-0
Reza, M. S., Taweekun, J., Afroze, S., Siddique, S. A., Islam, M. S., Wang, C., et al. (2023). Investigation of thermochemical properties and pyrolysis of barley waste as a renewable energy source. Sustain. For. 15:1643. doi: 10.3390/su15021643
Saeed, A. A. H., Harun, N. Y., Sufian, S., Bilad, M. R., Zakaria, Z. Y., Jagaba, A. H., et al. (2021). Pristine and magnetic Kenaf Fiber biochar for Cd2+ adsorption from aqueous solution. Int. J. Environ. Res. Public Health 18:7949. doi: 10.3390/ijerph18157949
Sahoo, S. S., Vijay, V. K., Chandra, R., and Kumar, H. (2021). Production and characterisation of biochar produced by slow pyrolysis of pigeon pea stalks and bamboo. Cleaner Engin. Technol. 3:100101. doi: 10.1016/j.clet.2021.100101
Selvarajoo, A., and Oochit, D. (2020). Effect of pyrolysis temperature on product yields of palm fibre and its biochar characteristics. Materials Sci. Energy Technol. 3, 575–583. doi: 10.1016/j.mset.2020.06.003
Setter, C., Borges, F. A., Cardoso, C. R., Mendes, R. F., and Oliveira, T. J. P. (2020). Energy quality of pellets produced from coffee residue: characterization of the products obtained via slow pyrolysis. Ind. Crop. Prod. 154:112731. doi: 10.1016/j.indcrop.2020.112731
Singh, A. L. (2004). Growth and physiology of peanut. Peanut Res. India 6, 178–212. Available at: https://www.researchgate.net/profile/Amrit-Singh-2/publication/284027853_Growth_and_physiology_of_Groundnut/links/57726f8408aeeec38953e0be/Growth-and-physiology-of-Groundnut.pdf
Singh, H., Northup, B. K., Rice, C. W., and Prasad, P. V. (2022). Biochar applications influence soil physical and chemical properties, microbial diversity, and crop productivity: a meta-analysis. Biochar 4:8. doi: 10.1007/s42773-022-00138-1
Sun, X., Shan, R., Li, X., Pan, J., Liu, X., Deng, R., et al. (2017). Characterization of 60 types of Chinese biomass waste and the resultant biocare in terms of their candidacy for soil application. GCB Bioenergy 9, 1423–1435. doi: 10.1111/gcbb.12435
Tabatabai, M. A. (1996). “Sulfur” in Methods of soil analysis: Part 3–chemical methods. ed. D. L. Sparks (Madison, WI, USA: SSSA and ASA), 921–960.
Tan, Z., Zouj, Z. L., and Huang, Q. (2018). Morphology, pore size distribution, and nutrient characteristics in biocare under different pyrolysis temperatures and atmospheres. J. Material Cycles Waste Manag. 20, 1036–1049. doi: 10.1007/s10163-017-0666-5
Tanquilut, M. R. C., Elairia, J. C., Amongo, R. M. C., Suministrado, D. C., Yaptenco, K. F., and Elauria, M. M. (2019). Biomass characterisation of pigeonpea (Cajanus cajan) wood for thermochemical conversion. Philippine J. Agric. Biosyst. Engin. 15, 39–52.
Tomczyk, A., Sokołowska, Z., and Boguta, P. (2020). Biochar physicochemical properties: pyrolysis temperature and feedstock type effects. Rev. Environ. Sci. Biotechnol. 19, 191–215. doi: 10.1007/s11157-020-09523-3
Venkatesh, B., Reddy, M. M., Sawargaonkar, G. L., Sarada, C., Padmaja, B., Gopalakrishnan, S., et al. (2023). Bio-inoculated nutrient management Influenceon soil nutrient availability pattern and growth of hybrid Pigeonpea (ICPH 2740) under establishment methods and crop geometry. Legum. Res. 46, 1490–1495. doi: 10.18805/LR-5188
Venkatesh, G., Srinivasarao, C., Venkateswarlu, B., Gopinath, K. A., Prasad, J. N. V. S., Reddy, B. S., et al. (2013). Operational process for biochar preparation from castor bean stalk and its characterization for soil application. Indian J. Dryland Agric. Res. Develop. 28, 21–26.
Verheijen, F., Jeffery, S., Bastos, A. C., Van der Velde, M., and Diafas, I. (2010). Biochar application to soils. A critical scientific review of effects on soil properties, processes, and functions. EUR 24099, 2183–2207.
Weidner, E., Karbassiyazdi, E., Altaee, A., Jesionowski, T., and Ciesielczyk, F. (2022). Hybrid metal oxide/biochar materials for wastewater treatment technology: a review. ACS Omega 7, 27062–27078. doi: 10.1021/acsomega.2c02909
Wijitkosum, S., and Jiwnok, P. (2019). Elemental composition of biochar obtained from agricultural waste for soil amendment and carbon sequestration. Appl. Sci. 9:3980. doi: 10.3390/app9193980
Woźniak, M., Ratajczak, I., Wojcieszak, D., Waśkiewicz, A., Szentner, K., Przybył, J., et al. (2021). Chemical and structural characterization of maize Stover fractions in aspect of possible applications. Materials 14:1527. doi: 10.3390/ma14061527
Yaashikaa, P. R., Kumar, P. S., Varjani, S., and Saravanan, A. (2020). A critical review on the biochar production techniques, characterization, stability and applications for circular bioeconomy. Biotechnol. Rep. 28:e00570. doi: 10.1016/j.btre.2020.e00570
Yadav, V. K., Yadav, K. K., Tirth, V., Gnanamoorthy, G., Gupta, N., Algahtani, A., et al. (2021). Extraction of value-added minerals from various agricultural, industrial, and domestic wastes. Materials 14:6333. doi: 10.3390/ma14216333
Yu, S., Park, J., Kim, M., Ryu, C., and Park, J. (2019). Characterization of biochar and byproducts from slow pyrolysis of hinoki cypress. Bioresources. Technol. Rep. 6, 217–222. doi: 10.1016/j.biteb.2019.03.009
Yuan, P., Wang, J., Pan, Y., Shen, B., and Wu, C. (2019). Review of biochar for the management of contaminated soil: preparation, application, and prospects. Sci. Total Environ. 659, 473–490. doi: 10.1016/j.scitotenv.2018.12.400
Zaitun, Z., Halim, A., Sa’dah, Y., and Cahyadi, R. (2022). Surface morphology properties of biochar feedstock for soil amendment. IOP Conf. Ser. Earth Environ. Sci. 951:012034. doi: 10.1088/1755-1315/951/1/012034
Zhang, Y., Sam, E. K., Liu, J., and Lv, X. (2023). Biomass-based/derived value-added porous absorbents for oil/water separation. Waste Biomass Valorization 14, 3147–3168. doi: 10.1007/s12649-023-02112-9
Zhang, C., Zhang, Z., Zhang, L., Li, Q., Li, C., Chen, G., et al. (2020). Evolution of the functionalities and structures of biochar in poplar pyrolysis over a wide temperature range. Bioresour. Technol. 304:123002. doi: 10.1016/j.biortech.2020.123002
Zhao, B., O'Connor, D., Zhang, J., Peng, T., Shen, Z., Tsang, D. C., et al. (2018). Effect of pyrolysis temperature, heating rate, and residence time on rapeseed stem-derived biochar. J. Clean. Prod. 174, 977–987. doi: 10.1016/j.jclepro.2017.11.013
Zheng, H., Wang, X., Chen, L., Wang, Z., Xia, Y., Zhang, Y., et al. (2017). Enhanced growth of halophyte plants in biochar-amended coastal soil: roles of nutrient availability and rhizosphere microbial modulation. Plant Cell Environ. 41, 517–532. doi: 10.1111/pce.12944
Keywords: peanut shells, biochar, slow and fast pyrolysis, SEM–EDX, FTIR, TGA
Citation: Sawargaonkar G, Pasumarthi R, Kale S, Choudhari P, Rakesh S, Mutnuri S, Singh A, Sudini H, Ramaraju M, Singh R, Padhee AK and Jat ML (2024) Valorization of peanut shells through biochar production using slow and fast pyrolysis and its detailed physicochemical characterization. Front. Sustain. 5:1417207. doi: 10.3389/frsus.2024.1417207
Received: 14 April 2024; Accepted: 08 July 2024;
Published: 25 July 2024.
Edited by:
Silvia Quispe Prieto, Jorge Basadre Grohmann National University, PeruReviewed by:
Ming-Qiang Zhu, Northwest A&F University, ChinaCopyright © 2024 Sawargaonkar, Pasumarthi, Kale, Choudhari, Rakesh, Mutnuri, Singh, Sudini, Ramaraju, Singh, Padhee and Jat. This is an open-access article distributed under the terms of the Creative Commons Attribution License (CC BY). The use, distribution or reproduction in other forums is permitted, provided the original author(s) and the copyright owner(s) are credited and that the original publication in this journal is cited, in accordance with accepted academic practice. No use, distribution or reproduction is permitted which does not comply with these terms.
*Correspondence: Rajesh Pasumarthi, cmFqZXNoLnBhc3VtYXJ0aGlAaWNyaXNhdC5vcmc=
†These authors have contributed equally to this work
Disclaimer: All claims expressed in this article are solely those of the authors and do not necessarily represent those of their affiliated organizations, or those of the publisher, the editors and the reviewers. Any product that may be evaluated in this article or claim that may be made by its manufacturer is not guaranteed or endorsed by the publisher.
Research integrity at Frontiers
Learn more about the work of our research integrity team to safeguard the quality of each article we publish.