- Institute for Technical Microbiology, Mannheim University of Applied Sciences, Mannheim, Germany
The issue of used rubber tires is becoming an ever-greater problem for the environment. Often these are disposed of in an illegal manner. Whether on forest paths, fields, or other unsuitable areas—illegal disposal of used tires is a punishable offense and a risk to people and the environment. Nevertheless, the number of cases increases from year to year. This is partly caused by the lack of suitable recycling options for waste tires. Reuse does take place but mainly in the form of downcycling, with the majority currently either being incinerated for energy recovery or, as shredded tires, used as substrate or filler material in roads and sporting grounds. Several reclamation technologies have been developed in the past, using for example mechanical, thermal energy and/or chemicals, aiming to provide a better solution to the waste tire problem, however, most processes cause some form of rubber degradation that limits reuse to low value applications. Only devulcanisation using a biotechnological approach with microorganisms and/or enzymes shows currently promise to reuse waste rubber for high value applications such as new tires. This review provides an overview of the technological development of different recycling options and their potential benefit to the circular economy.
1 Introduction
The sources of rubber waste can vary by region, generally the largest source is used or discarded tires that contain vulcanized rubber, typically making up to 50% of total rubber waste. Other major sources are rubber waste from automotive and conveyor belt manufacturing industries, as well as municipal solid wastes, such as clothing and shoe soles (Cummins, 2019). Based on these sources, waste rubber types range from natural rubber (NR) to synthetic rubbers such as styrene-butadiene rubber (SBR), nitrile-butadiene rubber (NBR), ethylene-propylene-diene monomer (EPDM), polyurethane (PU) and silicone rubber. All these rubber products and materials are a challenging source of waste and realizing a suitable and sustainable way to reuse waste rubber has been a global challenge for many years. Traditionally, waste rubber, i.e., end-of-life (EOL) tires, were dumped on landfills where they can form a hazardous ecosystem as potentially harmful substances used during the manufacture of tires, such as antioxidants and antiozonants [e.g., butylated hydroxyanisole, BHA and butylated hydroxytoluene, BHT (Armada et al., 2022) as well as 6PPD, N-(1,3-dimethylbutyl)-N′-phenyl-p-phenylenediamine (Hu et al., 2022)], as well as heavy metals can leach into the groundwater. Additionally, these dumped tires can act as a breeding ground for mosquitos, forming a further threat for the environment and human health (Yee, 2008; González et al., 2020). Given the lack of suitable recycling options, and regulation, huge landfill sites appeared and developed over the past centuries. Currently, the EU scraps a total of 3.6 million tons of worn tires per year, with global numbers reaching one billion tons (Cummins, 2019). An additional environmental problem emerged, with tire stockpiles forming inextinguishable fire hazards that have excellent properties to sustain combustion, and once ignited, burning thousands and millions of tires, as for example happened regularly in the US in the 1980s and 90s and more recently in Seseña, Spain in 2016 and in the world's largest tire dump in Sulaibiya, Kuwait in 2020. Rubber tires though do not easily ignite since rubber does not conduct heat very well and ignition therefore requires prolonged periods at very high temperature, and indeed a number of these accidents were caused by either very poor management and/or malfunctions at tire collection and destruction sites, or by arsonists. These fires typically last for a long time, from several days to months, a fire involving 10 million tires in Heyope, Wales began in 1989 and still smoldered 15 years later as the tires were packed too densely for firefighters to extinguish. All these fires caused significant damage to local ecosystems, including contamination of residential drinking water wells and contaminating soils with carbon black, as well as releasing harmful greenhouse gases including carbon dioxide, carbon monoxide and sulfur oxides into the atmosphere (Downard et al., 2015; Singh et al., 2015). As a result of these increasing problems associated with EOL tires, the United Nations Environmental Programme (UNEP) recognizes that waste tires are becoming a burgeoning problem for national and local governments to ensure a sustainable management of solid waste (Jain, 2016). Until around 20 years ago, up to and partially more than 50% of EOL tires were either dumped illegally or disposed in landfill sites. Legislation started to act upon that, with e.g., stockpiling of whole tires in landfills being banned in the EU in 2003 and shredded tires in 2006 according to the EU landfill directive 1999/31/EC. However, because of the lack of suitable reuse options for waste rubber, these large landfill sites partially still remain. It is estimated that there are currently 4 billion such tires in landfills and stockpiles worldwide (Cummins, 2019).
One problem of reusing or recycling waste rubber, apart from its immense quantity, is that rubber is a thermosetting polymeric material that unlike thermoplastic materials, cannot be remolded by simply reheating it. The sulfur that is used to crosslink rubber polymers during vulcanization, creates very strong chemical bonds and products that do not easily decompose. Various methods have been suggested to break these bonds and allow to revulcanise rubber by adding fresh sulfur to create new rubber products. An overview of these technologies and their pathways to potential end products are illustrated in Figure 1. Most of these methods, however, do not only break the sulfur crosslinks but also the carbon bonds. This typically leads to low value rubber products, e.g., rubber mats for playgrounds or roofing materials, where the initial physical characteristics of the rubber get destroyed, at least partially. To allow the reuse of rubber for high value products, such as rubber tires, the sulfur crosslinks need to be removed selectively which is a very challenging process. Some of the potential methods and their value for a circular economy will be discussed here with a focus on biotechnological solutions.
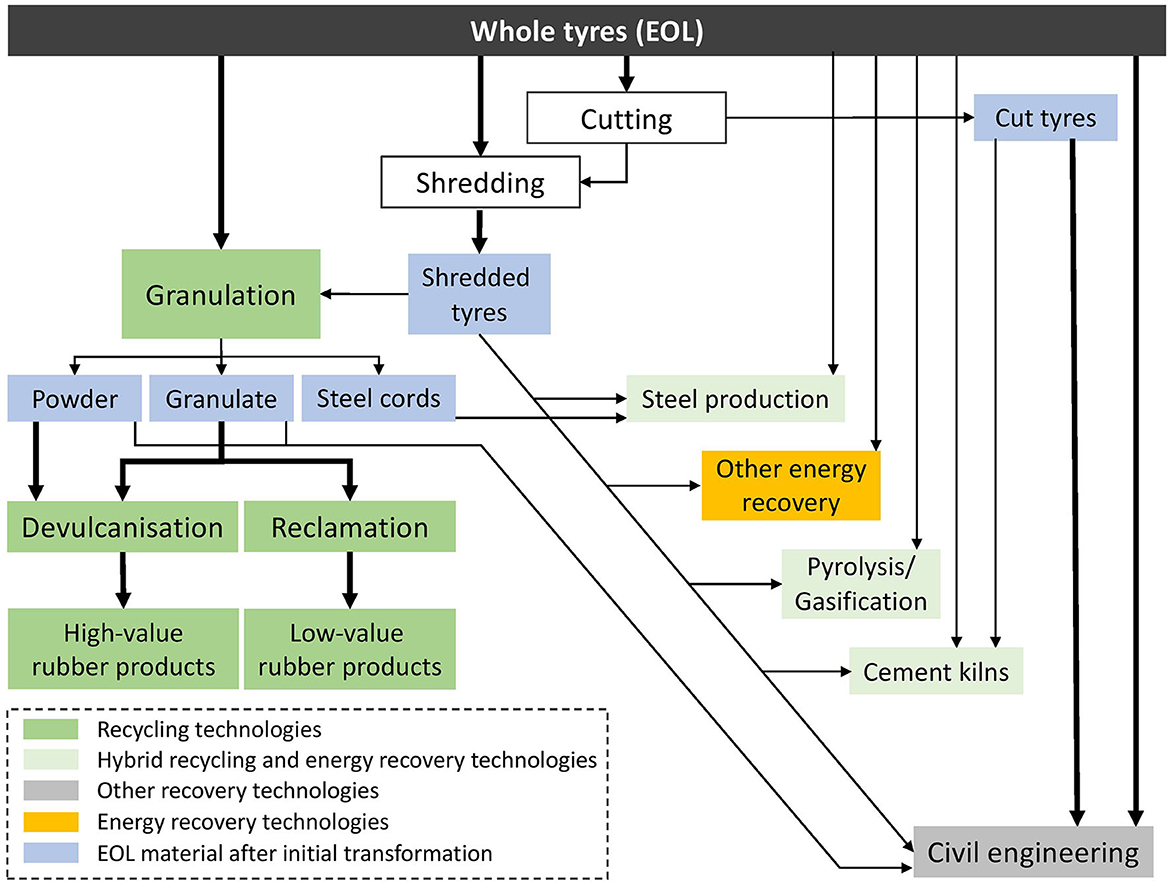
Figure 1. Different recovery routes of end-of-life rubber tires (initial stages of transformation pre-application). Modified after Cummins (2019).
2 Waste rubber collection and traditional recovery options
The first step in rubber recycling and reuse is collection of rubber waste materials. In 2019, 95% of waste tires were collected and treated for material recycling and energy recovery in Europe, with the remaining 5% either being stocked and waiting for treatment or not being able to be tracked down (European Tyre and Rubber Manufacturers' Association, 2021). Until the 2010's, a substantial amount of these tires, estimated at up to 10% (ETRMA, 2015), was exported, primarily to China, where the tires were burned as an energy source. The introduction of the waste import ban in China in 2018 heavily restricted this disposal option and increased the pressure to find alternative disposal routes. This is currently gaining even more importance as Europe heads toward a waste export ban. Surely, in terms of a circular economy, rubber waste combustion, either via export to other countries outside the EU or within the EU, has never been an ideal solution. It helps to decrease amounts of rubber waste, however, can lead to a production of greenhouse gases such as carbon dioxide and sulfur oxides if not handled and managed correctly. Also, the calorific value of burning a tire is approximately only 25%−30% of the energy needed to produce a tire (Snyder, 1998), therefore not very energy efficient. Regardless, in Europe close to 1.5 million tires are being burned every year (European Tyre and Rubber Manufacturers' Association, 2021), primarily in cement production where shredded or whole tires are added to the slurry as it enters the cement kiln. As waste rubber tires form a cheap alternative to the usual heat sources, typically oil, gas or petroleum coke, they are a welcomed addition to the heat process by most cement manufacturers. A recent economic and environmental assessment of using waste tires as fuel in cement clinker production reported a cost reduction of around 25%, when a combination of 40% scrap tires and 60% petroleum coke was used instead of 100% coke (Castañón et al., 2021). Additionally, in the same study and using the same test conditions, a reduction of 17% NOx, 28% SO2 and up to 60% CO at the exit of the kiln was observed, showing that there is also potential environmental benefit when cement kilns are operated efficiently. There is another technological advantage of using waste tires as fuel in cement kilns, as it is not necessary to remove the metal reinforcements in the tires prior to incineration as they can partially replace the iron additions used as a flux in the composition of cement raw mix (Castañón et al., 2021). Further research is required, however, to fully determine all the potential environmental implications of using tires as fuel and under which conditions EU limits, e.g., 500 mg/Nm3 NOx and 50 mg/Nm3 SO2 according to Directive 2010/75/EU for cements kilns co-incinerating waste, can be met.
The economic viability of utilizing waste tires in cement kilns depends on a number of factors, such as the ability to obtain gate or tipping fees. Although alternative fuels, e.g., plastic waste, may lead to greater competition, the comparable high calorific value of tires make them an appealing option.
From a sustainability perspective, environmental benefits depend on the original heat source being used. Industries can transition from fossil fuel to waste tires for a share of their energy demand and generate less greenhouse gas or other polluting emissions. Alternative fuels, such as waste tires, can therefore be a more sustainable option to decrease air pollution.
Another traditional disposal route for used tires is material recovery, in particular crumbing and reusing the resulting tire crumb as infill in compounds for road, sports, and playground surfaces. In Europe, around every third EOL tire is currently being used for that recycling route (Cummins, 2019). Scrap tire rubber can be for example incorporated into asphalt paving mixes using two different methods, a wet and a dry process. In the wet process, crumb rubber typically acts as an asphalt cement modifier to improve the rheological properties of asphalt binder. while in the dry process, crumb rubber is used to modify bitumen or as a partial substitute for aggregates (Lim et al., 2021; Riekstins et al., 2021; Hussein et al., 2022; Li et al., 2022). Both technologies can play an important role for the circular economy by reducing resource consumption and by alleviating environmental pollution, however, their application is part of a long ongoing debate around the safety of crumbed rubber and the potential release of carcinogenic substances used during the tire manufacture, such as antioxidants and antiozonants as well as polycyclic aromatic hydrocarbons (PAHs), into the environment. Over the last decade numerous articles concluded that crumbed rubber infill poses no threat to human health (Simcox et al., 2011; Pavilonis et al., 2014; Oomen and De Groot, 2017; Schneider et al., 2020a,b,c), but as the concerns remain and as crumbed rubber is part of the microplastic classification, it is likely only a question of time for this application to be restricted or even fully banned in the EU. Indeed, after long discussions the European Commission and the EU member states decided to prohibit the use of granules and mulches as infill if they exceed 20 mg/kg PAHs to make playing on artificial sport pitches and playgrounds safer. Recent studies, however, showed over-standard content of PAHs in rubber crumb in a large number of samples (Armada et al., 2022; Grynkiewicz-Bylina et al., 2022). Furthermore, the European Chemical Agency (ECHA) also proposed wide-ranging restrictions on intentional uses of microplastics. Indeed, on 25th September 2023, the European Commission announced to adopt measures that restrict microplastics intentionally added to products under the EU chemical legislation REACH (European Commission, 2023). An international policy review on the current state of regulations for artificial turf and rubber crumb infill has also been published very recently by Zuccaro et al. (2022). If this market is being completely ruled out in the future, however, current recycling alternatives would basically be incineration or illegal landfill, or exports as described above. Therefore, additional recovery technologies for waste rubber have been developed over the last years, including its use in construction materials such as cementitious concrete, asphalt concrete and granular materials for earth structure. Various studies have shown advantageous properties when rubber crumb was mixed into the compounds, as recently reviewed by Mushunje et al. (2018), Mohajerani et al. (2020), and Singh et al. (2023).
As the granulation of used tires requires advanced treatment and processing steps, the financial investment for granulation and its applications can generally be higher than for other recycling methods. In addition, some markets for ground rubber applications, such as use in artificial sports fields, have already declined due to the ongoing debate about the possible release of carcinogenic substances during tire production. Recent measures by the European Commission, such as the restriction of intentionally added microplastics to products, will further hinder this application.
Granulation is considered material recycling and is therefore high up in the hierarchy of rubber waste management. However, the above-mentioned uncertainty about potential health and environmental hazards associated with certain applications of rubber granules will most likely further limit this recovery method in the future.
Beside energy and material recovery, a third recovery option for waste tires is oil recovery, also known as pyrolysis. Here, EOL tires are decomposed into oil, gas, steel, and char using high temperatures and pressure under anoxic conditions. Though this process traditionally only had minor importance with < 1% of waste tires being used to extract oil and gas (ETRMA, 2017), this technology has gained traction more recently with large chemical companies investing substantial resources to make this process more cost-effective and process oil and gas from EOL tires a lucrative alternative to usual oil and gas. Pyrolysis also generates valuable by-products such as carbon black that can be blended into various materials, e.g., plastic and ethylene-vinyl acetate (EVA) foam. The potential of waste tire pyrolysis has been reviewed in the past (Martínez et al., 2013; Williams, 2013) and also again more recently (Dick et al., 2020; Hoang et al., 2020; Campuzano et al., 2023).
All products of the pyrolysis process, including high-quality carbon black, oil, syngas as well as residual steel have various potential applications. Currently, however, the economic viability of pyrolysis is still quite low in relation to virgin or traditional materials. However, with increasing oil and gas prices and the increasing level of technical feasibility, pyrolysis might become more important in the near future. Indeed, particularly the development of tire-derived material carbon black is in the process of commercialization by a number of European companies.
Still, from an environmental point of view, pyrolysis can cause significant air pollution if environmental standards are not upheld, therefore high environmental standards with continuous monitoring of emissions are crucial while developing this technology further.
3 Retreading and reuse of waste tires
As an alternative to the recovery options described above, the lifetime of some tires can potentially be extended with a process called retreading. Here, the waste tire is stripped off its tread and replaced by a new one. Truck tires for examples are typically retreaded up to three times which can be very profitable as it only requires 30% of the energy and 25% of the raw materials needed in comparison to produce new tires (Sienkiewicz et al., 2012), therefore also significantly reducing the amount of waste tires pilling up in the environment (Dabić-Ostojić et al., 2014; Ounsaneha et al., 2020). Since life cycle studies in the literature for tires are rare, it is very difficult to fully assess the environmental benefits from retreading. One such attempt was made by Gutowski et al. (2011), they calculated a 68% energy saving for a retreaded heavy-duty truck tire (compared to the production of a new one), using life cycle inventory data for the materials and manufacturing phase only. However, they also pointed out that, if the use phase was also considered, depending on the increased rolling resistance of a retreaded tire as well as its reduced lifetime, energy savings reduce to 28% or even become unfavorable if the rolling resistance fraction is larger (depending on driving behavior), e.g., 33%, and expected lifetime is less.
Waste tires can also be repurposed and directly recycled retaining their original form in several civil engineering applications such as garden planters, playground equipment, outdoor furniture, road embankments, erosion barriers, artificial reefs and many others (Sienkiewicz et al., 2012). Most of these applications, however, are not widely practiced currently because of the potential negative impacts of leachates on the environment.
Though retreading is in line with the circular economy and to expand the lifetime of products, it is currently only an established practice for truck and aircraft tires but not for automobile tires because of economic profitability. The market for civil engineering applications of whole or shredded tires also remains small, however, these applications often offer economic advantages over traditional materials. Additionally, using waste tires in civil engineering can create products with a high added value due to the advantageous technical properties of rubber tires.
Despite some potential improvements in environmental impact compared to alternative construction materials, such as the use of stone, gravel and sand, the overall performance of such applications in construction can be considered lower than other recycling routes for used tires due to the materials they replace.
4 Recycling technologies
In terms of the waste hierarchy of the circular economy, if the reuse of materials is not possible, recycling is the next desirable option. For both routes, reuse and recycling, a material should be reused for its originally intended purpose. In the case of waste tires, recycling would mean the use of recycled tire rubber as a compounding ingredient for new tires. Multiple recycling technologies have emerged over the past years but many fall short for this desired recycling goal. As mentioned before, to recycle rubber crumb, the S–S and C–S crosslinks between the polymer chains of the rubber need to be broken in a process called desulfurization (see Figure 2). Most technologies developed to date achieve this to some extent but also break the carbon chain bonds, resulting in poor physical properties of the recycled rubber crumb and therefore limiting its potential applications and restricting it to low-value rubber products. This process is called reclamation and uses mechanical, thermal energy and/or chemicals to convert vulcanized scrap rubber into a reusable rubber material that can be mixed, processed and vulcanized again (Isayev, 2001). The following provides a short overview of the key reclamation methods developed to date and their current application potential.
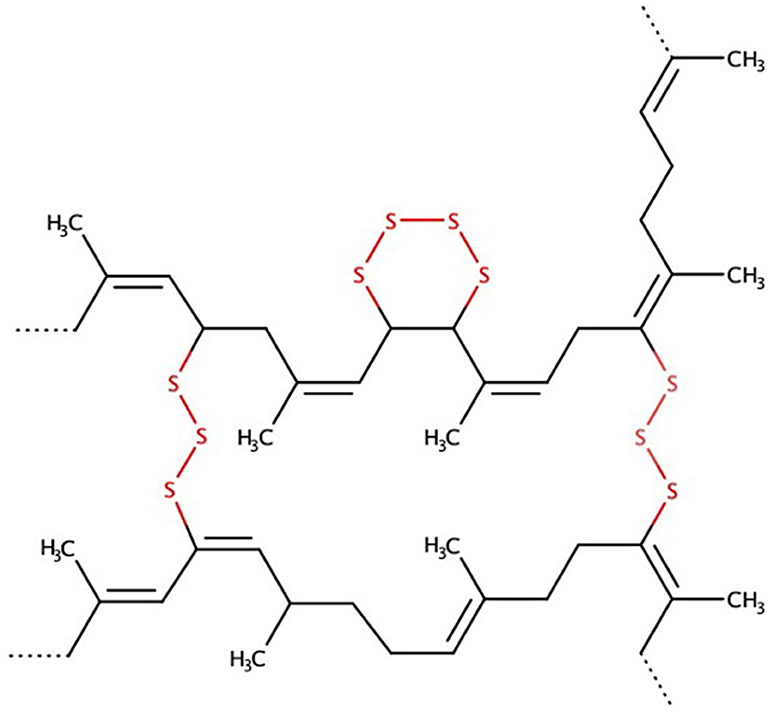
Figure 2. Exemplary chemical structure of vulcanized natural rubber showing the crosslinking of two polymer chains with sulfur. Revulcanisation for applications in high-value rubber products requires selective breakage of these sulfur crosslinks (highlighted in red) while keeping the carbon backbone intact.
4.1 Thermochemical reclamation
One such example is thermomechanical reclamation where a combination of high temperature, ranging from 60 to 220°C, and shear force, e.g., by using an industrial screw extruder, is used to achieve breaking of crosslinks. This process is not selective and as such the polymer backbone gets damaged to some extent as well. Many different parameters and techniques have been trialed to achieve a more selective crosslink scission. It was observed, for example, that an increase of barrel temperature during the thermomechanical reclaiming of ground tire rubber (GTR) had a positive effect on the decrease of screw torque and decrease of Mooney viscosity, improving, for example, the interactions between SBR and reclaimed rubber during compounding (Formela et al., 2016). Contrary, another study achieved the best devulcanisation quality at lower temperatures of 80–100°C and indicated that rubber industry waste could therefore be recycled with low energy consumption (Seghar et al., 2019), however, thermochemical reclamation is rarely used today.
4.2 Microwave reclamation
Similar to the thermochemical method, cleavage of crosslinks can also be achieved by the less aggressive heating effect of microwaves. Again, depending on key parameters such as temperature and length of treatment, selectivity of crosslink scission can suffer, and the polymer chains might experience degradation. Desulfurization rates of up to 85% were reached at high temperatures (180–200°C) but selectivity was low (Simon and Bárány, 2023). In the same study the selectivity of samples treated at 140–160°C was much improved but the decrease in the crosslink density was only moderate. Another study showed promising desulfurization rates at temperatures of 200°C or more, but again also experienced shortening of the polymer as a result of C–C bond breakage (Valdés et al., 2021). Another apparent limitation of the microwave method is that only rubber with sufficient polarity is susceptible to the microwave radiation (Asaro et al., 2018). Rubber with a poor absorptivity of the radiation, such as NR, SBR and EPDM, require suitable additives that absorb microwave radiation, e.g., silica and carbon black. Recent advances and limitations in microwave treatment in waste rubber recycling were reviewed by Formela et al. (2019).
4.3 Ultrasonic reclamation
Breakage of C–S and S–S crosslinks in vulcanized rubber can also be achieved by ultrasonic waves that induce cavitations in the polymer matrix, in this way devulcanising the rubber and making it reprocessable. Since C–C bonds have a higher bond level than S–S and C–S bonds, fewer C–C bonds are broken during the ultrasonic treatment, therefore reducing degradation and mechanical property loss. Research to date on this method is limited and primarily focused on different particle crumb mesh sizes or surface areas and the efficiency of devulcanisation. Under the same amplitude of ultrasonic wave and temperatures of 250°C, smaller mesh sizes (e.g., 30 mesh) showed a better extent of devulcanisation compared with larger mesh sizes such as 10 mesh (Isayev et al., 2014). Generally, advantages of this method are that initial results seem encouraging and that the process can take place within a few seconds and does not require addition of chemicals, but on the downside, it requires a high energy input and is therefore not cost-efficient.
4.4 Chemical reclamation
This method is often coupled with (thermo)mechanical and other methods (e.g., with microwaves). Various chemicals have been reported to act as devulcanising reagents that break the crosslinks between rubber chains, including sulfides, mercaptans, peroxides and ionic liquids (Bockstal et al., 2019). Adding these reagents aims at introducing chemical reactivity to the surface of crumbed rubber, therefore improving the chemical bonding and adhesion of the rubber tire crumb into the virgin tire rubber matrix during compounding. These reactions typically require heat to proceed to the homolytic scissions of the components, however, higher reaction temperatures can also lead to a concurrent degradation of the polymer, resulting in poor mechanical properties of the final product (Bockstal et al., 2019). Molanorouzi and Mohaved (2016) for example tested several sulfide containing chemicals in a microwave process to achieve waste rubber crumb reclamation. The most promising devulcanising reagent was diphenyl disulfide (DPDS) which, at 6 phr and the addition of 30 phr of aromatic oils at 240°C for 3 min, achieved 51% devulcanisation of the crumb. However, the reclaimed rubber exhibited inferior mechanical properties such as significantly reduced tensile strength of 10 MPa and elongation at break of 226%. Mechanical properties remained unaffected in another study, using disulfide oil as chemical agent during mechanical reclamation of waste EPDM rubber (Sabzekar et al., 2016). Additionally, terpenes and solvents were successfully tested as swelling agents to enhance bond-breaking selectivity at low temperature. Guo et al. (2021) for example achieved promising swelling effects below 100°C using terpinene, resulting in good physical properties of the revulcanised rubber including tensile strength of 17 MPa and elongation at break of 401% when 3 phr terpinene were mixed with 100 phr waste rubber crumb.
In summary, chemical reclamation methods show promise in terms of desulfurization efficiency and a wide selection of devulcanising reagents is available, however, most of these chemicals are harmful to the environment and might also cause odor issues with the final products incorporating chemically reclaimed rubber crumb. Therefore, non-sulfured compounds are currently investigated as environment-friendlier alternatives.
4.5 Reclamation using supercritical CO2
Another reclamation technology gained some traction more recently which is the use of supercritical CO2 (scCO2) to devulcanise waste rubber crumb. This method has been proposed as a greener alternative to chemical reclamation and relies on the promise that the CO2, under supercritical conditions, swells the crumb and stretches the sulfide bonds, hence allowing easier bond scission. Indeed, high devulcanisation rates of up to 98.5% were achieved (determined as sol fraction of the reclaimed rubber) (Jiang et al., 2013), however, the treated butyl rubber crumb failed to revulcanise when sulfur was added as curing agent during compounding, potentially due to a shift of the functional groups on the crumb surface caused by remaining diphenyl disulfide that was added as devulcanising agent during reclamation. In general, the devulcanisation with scCO2 is not well understood yet but initial results show some promise as recently reviewed by Asaro et al. (2018).
Generally, reclaimed rubber can have environmental and economic benefits, e.g., by reducing the demand for virgin rubber, by diverting waste rubber materials from landfill or incinerators and thus saving on rubber waste volumes and disposal costs, and by potentially saving energy as further development of reclamation technologies could lead to lower energy requirements compared to manufacturing of virgin rubber. By reducing the demand for natural rubber, which is often associated with deforestation in some regions, the use of reclaimed rubber can also help to protect valuable ecosystems and conserve biodiversity. While reclaimed rubber can offer these various environmental benefits, it also has potential environmental disadvantages and challenges. Primarily, some reclaimed rubber production processes may require the use of chemical additives (e.g., see Section 4.4), such as vulcanising agents, accelerators and plasticizers, which can pose environmental risks if not managed properly. A complete life cycle assessment of individual reclamation technologies and resulting rubber products is necessary, however, to fully demonstrate environmental and economic benefits on a case-by-case basis. Robust data for this purpose are, unfortunately, still very rare.
5 “True” devulcanisation
Though some of the above presented technologies show desulfurization rates close to 100% and therefore claim to demonstrate complete devulcanisation, their value to the circular economy and the global rubber market and its applications remains limited because of the concurrent degradation of the rubber polymer and the resulting poor mechanical properties of the reclaimed rubber. For a general comparison of the pros and cons of the different techniques in rubber waste management and their main applications, see Table 1.
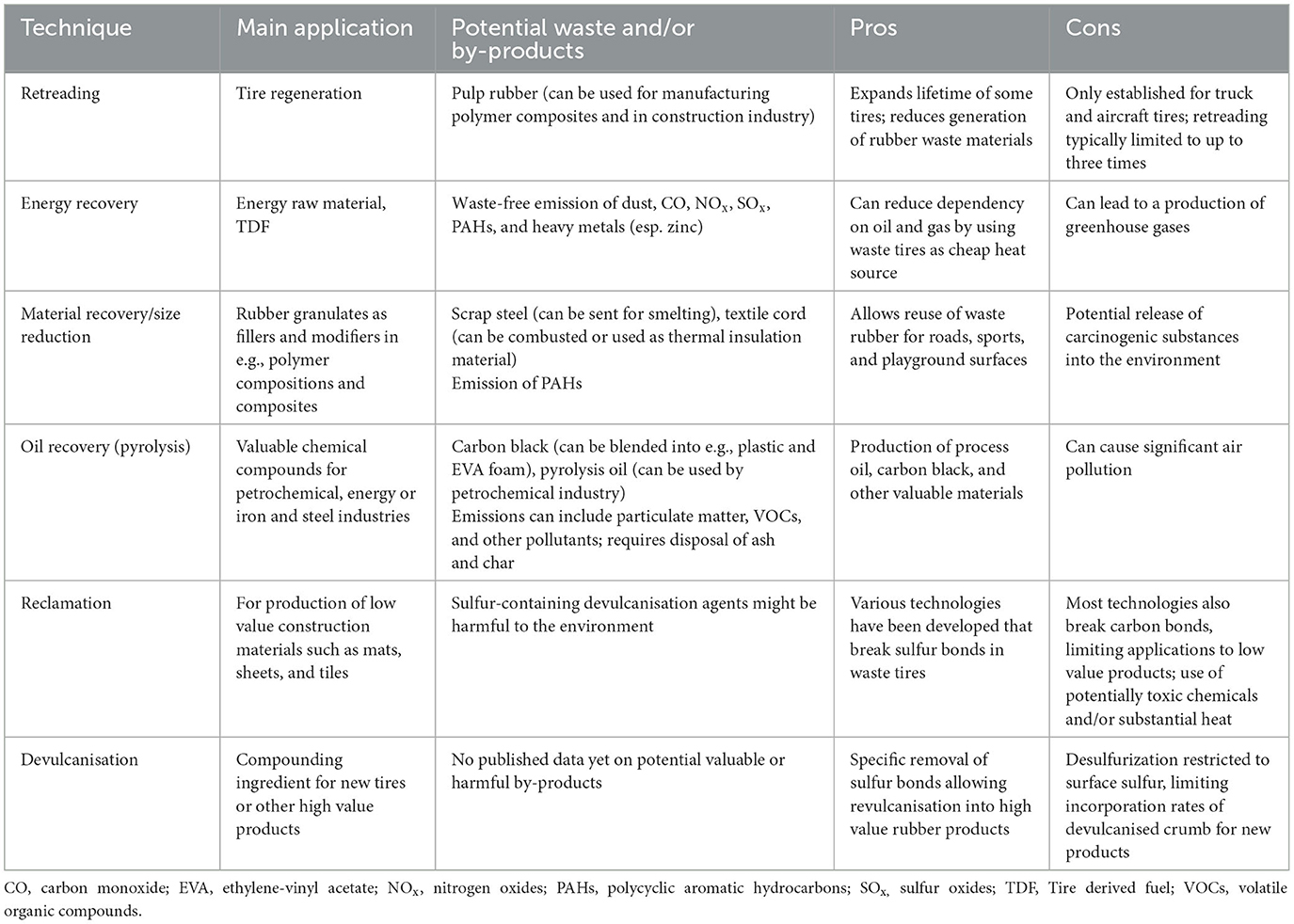
Table 1. Comparison of different rubber waste management techniques, highlighting their main applications, potential waste and by-products, and advantages and disadvantages for the (circular) economy and the environment.
To end the waste rubber problem, the focus therefore needs to shift from recycling to the reuse of rubber waste as a valuable material for high-value products. As mentioned before, reusing waste rubber crumb for these applications requires to break the S–S and C–S crosslinks between the rubber polymer chains while keeping the C–C bonds intact (see Figure 2). This can only be achieved by “true” devulcanisation but not by reclamation. To date the biggest potential to achieve devulcanisation without degrading the rubber polymer has been demonstrated using biotechnological methods using specific microorganisms and desulfurization enzymes. Several key parameters are crucial for a successful and economically feasible devulcanisation.
5.1 Rubber granulation technique and particle size
As these organisms cannot penetrate the rubber matrix, waste rubber materials first need to be reduced in size to supply a fine crumb or powder with a large surface area. Scrap tires are normally first shredded into larger pieces with an average size of ~20 × 20 mm and then ground to fine crumb of typically < 2 mm. Generally, the smaller the crumb, the larger the surface area and therefore more sulfur crosslinks that can be broken down by the desulfurising microorganisms. For microbial desulfurization, typically mesh sizes between 12 (1,680 μm) and 70 mesh (210 μm) have been trialed. The conventional method of grinding waste rubber materials is ambient milling which can be done dry or wet. As the ambient milling generates heat that can lead to some degradation of the rubber surface, wet milling is usually preferred to cool the rubber during the milling process. Alternatively, cryogenic grinding can be used to produce rubber crumb while avoiding heat degradation of the rubber, a technique first developed in the 1960s. Shredded tires are cooled in liquid nitrogen to −196°C, the cold brittle rubber is then processed through a hammer mill, producing rubber particles of various sizes which are subsequently sieved to remove remaining fibers and steel. Ground rubber through milling typically produces rubber crumb with a rough surface while cryogenic ground rubber has a smooth surface (Phadke et al., 1984). A third method, waterjet milling, has been developed more recently. Here, high pressure waterjet systems (e.g., 600 MPa) are used to pulverize tire treads into fine rubber powder without generation of heat or need of chemicals. Hrdlička et al. (2022) analyzed the influence of these three different methods as well as particle size on the properties of SBR, NR and BR and found that waterjet milling showed the biggest promise with best tensile strength (18.4 MPa) and elongation at break (459%) achieved with very fine waterjet ground rubber crumb of 0–100 μm. Current advances in waste tire rubber grinding technologies were recently reviewed by Formela (2021).
Certainly, economics play a large role for selecting the most suitable milling method as well as rubber particle size for subsequent microbial treatment.
5.2 Selecting desulfurizing microorganisms
Several species belonging to both, Bacteria and Archaea, have the ability to selectively break sulfur bonds on the surface of rubber crumb. Mainly sulfur oxidizing species, particularly species of the genus Thiobacillus and thermoacidophiles of the order of Sulfolobales, have been studied in the past. For example, a continuous increase of sulfate in the medium during a 30-day cultivation of Thiobacillus ferreoxidans was used as indication that the sulfur on the surface of GTR was oxidized (Li et al., 2011). Successful devulcanisation was later confirmed by the same authors by examining cure characteristics, swelling behavior and crosslink density, with improvements in mechanical properties being observed for the desulfurized GTR when compared with untreated controls (Li et al., 2012a). This was attributed to the enhanced interfacial interaction between desulfurized GTR and NR. A similar study was then conducted with Sphingomonas sp., also demonstrating a reduction of sulfur content in GTR as well as improved mechanical properties and higher swelling values after cultivation for 20 days (Li et al., 2012b).
Another interesting phylum containing many members of gram-positive bacteria with demonstrated desulfurization capabilities are Actinobacteria. Various species of the genus Gordonia (ex Tsukamura) have been studied more recently in particular. Microbial treatment of vulcanized rubber for 20 days using G. amicalis and dibenzothiophene as substrate resulted in a reduction of surface sulfur content of up to 22.9% but also in a reduction of carbon (by 9.1%) (Hu et al., 2016). FTIR analysis confirmed that not only S–S bonds were broken (producing S=O bonds) but also C=C bonds (transferred into C=O bonds), causing not only desulfurization but also degradation of rubber. Synergistic effects were observed for a mixed consortium of Gordonia sp. and Sphingomonas sp., yielding in a decrease of GTR surface sulfur by 32.4% (Cui et al., 2016). Mechanical properties and the interface coherence between desulfurized GTR and SBR matrix improved as well after microbial treatment and were enhanced when the mixed consortium was used rather than any single bacteria. A similarly high reduction in surface sulfur was also observed for Gordonia desulfuricans by Christofi et al. (2010) ranging between 23 and 35%. Bacterial persistence of Gordonia on rubber crumb, even after vulcanized GTR sterilization, was also shown for G. desulfuricans DSM 44462T (Tatangelo et al., 2016). In another study by the same authors and using the same strain, vulcanisates containing desulfurized GTR showed better mechanical and rheological properties than untreated GTR vulcanisates and even comparable with a NR reference (Tatangelo et al., 2019). Eleven different strains with devulcanisation properties, including four Gordonia strains, were studied by Ghavipanjeh et al. (2018) in a comprehensive study to evaluate biological devulcanisation of GTR and 11 potential factors impacting it. They found that the amount and mesh size of GTR were the most important factors in biological rubber devulcanisation. Surprisingly, they obtained better results with larger particle sizes of 45 mesh than for smaller particles of 60–80 mesh size. Generally, smaller particles provide a larger surface area for the bacteria to attach to and act on, thus a better desulfurization would be expected with these finer particle sizes. The authors, however, observed additional physical and mechanical phenomena by using finer particles such as immersing ability and shear tension that could negatively affect the mixing ability of the rubber particles. This highlighted that efficient mixing can be a key challenge during microbial devulcanisation of GTR, particularly when finer rubber particles are being used.
5.3 Key desulfurization enzymes and pathways
It is generally believed that in rubber devulcanisation the same enzymes are involved that also catalyze the removal of sulfur in fossil fuels. Over the last 20 years, our understanding of biodesulfurization has improved largely, mainly through studying genes involved in the degradation of the model compound dibenzothiophene (DBT) (Mohebali and Ball, 2008; Xu et al., 2009). A number of DBT-desulfurizing rhodococci and other isolates have been reported and extensively studied for their desulfurization capabilities, particularly Rhodococcus sp. IGTS8, the first isolated strain shown to degrade DBT (Kayser and Bielaga-Jonkeas, 1993). Two major DBT desulfurization pathways have been reported, the “Kodama” and the “4S” pathway. Only the 4S pathway involves sequential oxidation of the sulfur part and cleaving of the C–S bonds while keeping the C–C bonds intact (Figure 3B). Three enzymes, DszC, DszA, and DszB, have been identified that catalyze the step-wise reactions that convert DBT to DBT-5-oxide (DBTO), DBT-5, 5′-oxide (DBTO2), 2′-hydroxybiphenyl-2-sulfinate (HPBSi) and 2′-hydroxybiphenyl (HBP) without degradation of either aromatic ring (Denome et al., 1993, 1994). DszC and DszA are flavin-dependent monooxygenases that require FMNH2 or FADH2. Therefore, an additional enzyme, flavin reductase (DszD), is required which catalyzes the reduction of flavins, such as FMN and FAD, by NAD(P)H to form reduced flavins. DszB, a desulfinase, then catalyzes the last step of the pathway in which HPBSi is desulfinated to produce HBP. Despite their similar structures, most DBT-desulfurizing bacteria seem to be unable to desulfurize Benzothiophene (BTH), the other major sulfur constituent of fossil fuels. G. desulfuricans is one of only few microorganisms that has been identified to selectively cleave the sulfur from BTH without breaking the carbon-carbon bonds (Gilbert et al., 1998). Other BTH-desulfurising bacteria reported are Rhodococcus sp. T09 (Matsui et al., 2000), Paenibacillus sp. A11-2 (Konishi et al., 2000), Sinorhizobium sp. KT55 (Tanaka et al., 2001), Gordonia alkalivorans (Alves et al., 2005; Aggarwal et al., 2013; Delegan et al., 2021), Gordonia terrae strain C-6 (Wang et al., 2013) and Rhodococcus sp. strain SA11 (Mohamed et al., 2015). It is believed that the enzymes involved in BTH degradation are similar to the Dsz enzymes responsible for the degradation of DBT in the 4S pathway (Figure 3). However, the analogs of DszA, DszB, and DszC in the biodesulfurization of BTH have not been fully confirmed yet. Although the first operon for BTH biodesulfurization, Bds, has been identified for G. terrae (Wang et al., 2013), a full identification of the involved biodesulfurization enzymes and their regulation is still lacking. Additionally, feedback inhibition mechanisms of sulfate, the most likely sulfurous end product of desulfurization, need to be elucidated for industrially relevant strains to improve process efficiency. It was reported, for example, that the dsz promoter in R. erythropolis IGTS8 is repressed by sulfate (Li et al., 1996) while several studies reported attempts to overcome sulfate repression for Rhodococcus sp. T09 (Matsui et al., 2002; Tanaka et al., 2002; Noda et al., 2003). Also, dszAB genes have been successfully expressed in E. coli in the past, with the recombinant strain growing 4.5 times faster and showing no sulfate repression (Alves et al., 2007).
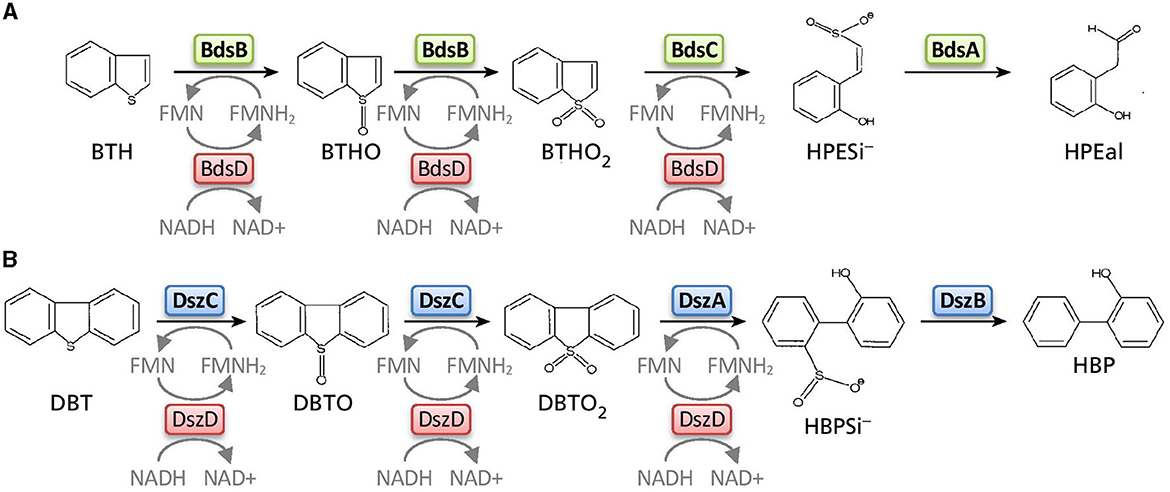
Figure 3. Comparison of the proposed “4S” BTH desulfurization pathway for Gordonia desulfuricans (A) with the DBT desulfurization pathway of Rhodococcus sp. strain IGTS8 (B). BTH, benzothiophene; BTHO, benzothiophene S-oxide; BTHO2, benzothiophene S,S dioxide; HPESi−, (Z)-2-(2H-hydroxyphenyl)-ethen 1-sulphinate; HBPEal, 2-(2H-hydroxyphenyl)-ethan 1-al. For abbreviations of DBT metabolites, see text. Adapted from Gilbert et al. (1998).
Again, life cycle data for microbially devulcanised rubber are, to the best of the author's knowledge, not available yet while these technologies are still being developed. Hence, a full analysis of potential environmental and economic benefits of devulcanised rubber products is not possible at the moment. Given the typical mild conditions used for most biotechnological processes, however, significant energy and cost savings can be expected. Economic feasibility should be achievable as devulcanised rubber, in contrast to reclaimed rubber, can also be used for high value rubber products such as tires, as a selective removal of sulfur crosslinks can be achieved without breaking the carbon backbone of the rubber polymer.
6 Conclusions and recommendations
These studies all show substantial promise that microbial desulfurization of waste tire crumb could be a sustainable approach to deal with the waste tire problem in future, particularly in accordance with shifting to a circular economy where materials are (re)used for their intended purpose and no waste is created. Here, microbial devulcanisation, the only true devulcanisation approach that allows to reuse the waste rubber materials for new high-value rubber products, clearly surpasses alternative recycling routes. For a successful commercialization, a few challenges still must be overcome, however. Our understanding of microbial devulcanisation, e.g., the key enzymes involved and potential feedback inhibition by sulfate released into culture media, need to be studied further to optimize the process. Also, factors that could impact desulfurization rates such as mesh size, GTR amount, required sterility of crumb and bacterial loading, as well as optimal process conditions (media composition, incubation length, temperature, etc.) need to be investigated and determined. For scaling-up biotechnological devulcanisation processes, additional challenges will occur. Given the hydrophobic nature of rubber crumb, mixing will be an important factor for any process using GTR. Efficient mixing will be key, not only to guarantee product homogeneity but also to supply sufficient oxygen levels for aerobic devulcanisation processes. Finally, research gaps in assessing environmental and economic impacts need to be closed for the various recycling options before a wider commercialization.
Overcoming these hurdles will require more in-depth research. Establishing a biotechnological approach to tackle the waste tire problem, however, seems to be within reach over the next few years. Their development should be a major focus now for waste rubber management and perhaps the only possible solution, at least in terms of a circular economy, to counteract the increasing pressure waste tires put on our environment.
Author contributions
DG: Writing – original draft.
Funding
The author(s) declare that no financial support was received for the research, authorship, and/or publication of this article.
Acknowledgments
The author gratefully acknowledges fruitful discussions, particularly on desulfurization enzymes and pathways, with Dr. Jennifer Pratscher while preparing this manuscript.
Conflict of interest
The author declares that the research was conducted in the absence of any commercial or financial relationships that could be construed as a potential conflict of interest.
Publisher's note
All claims expressed in this article are solely those of the authors and do not necessarily represent those of their affiliated organizations, or those of the publisher, the editors and the reviewers. Any product that may be evaluated in this article, or claim that may be made by its manufacturer, is not guaranteed or endorsed by the publisher.
References
Aggarwal, S., Karimi, I. A., and Reinaldi Ivan, G. (2013). In silico modeling and evaluation of Gordonia alkanivorans for biodesulfurization. Mol. Biosyst. 9, 2530. doi: 10.1039/c3mb70132h
Alves, L., Melo, M., Mendonça, D., Simões, F., Matos, J., Tenreiro, R., et al. (2007). Sequencing, cloning and expression of the dsz genes required for dibenzothiophene sulfone desulfurization from Gordonia alkanivorans strain 1B. Enzyme Microb. Technol. 40, 1598–1603. doi: 10.1016/j.enzmictec.2006.11.008
Alves, L., Salgueiro, R., Rodrigues, C., Mesquita, E., Matos, J., Gírio, F. M., et al. (2005). Desulfurization of dibenzothiophene, benzothiophene, and other thiophene analogs by a newly isolated bacterium, Gordonia alkanivorans strain 1B. Appl. Biochem. Biotechnol. 120, 199–208. doi: 10.1385/ABAB:120:3:199
Armada, D., Llompart, M., Celeiro, M., Garcia-Castro, P., Ratola, N., Dagnac, T. J., et al. (2022). Global evaluation of the chemical hazard of recycled tire crumb rubber employed on worldwide synthetic turf football pitches. Sci. Total Environ. 812, 152542. doi: 10.1016/j.scitotenv.2021.152542
Asaro, L., Gratton, M., Seghar, S., and Aït Hocine, N. (2018). Recycling of rubber wastes by devulcanization. Resour. Conserv. Recycl. 133, 250–262. doi: 10.1016/j.resconrec.2018.02.016
Bockstal, L., Berchem, T., Schmetz, Q., and Richel, A. (2019). Devulcanisation and reclaiming of tires and rubber by physical and chemical processes: a review. J. Clean. Prod. 236, 117574. doi: 10.1016/j.jclepro.2019.07.049
Campuzano, F., Martínez, J. D., Agudelo Santamaría, A. F., Sarathy, S. M., and Roberts, W. L. (2023). Pursuing the end-of-life tire circularity: an outlook toward the production of secondary raw materials from tire pyrolysis oil. Energy Fuels 37, 8836–8866. doi: 10.1021/acs.energyfuels.3c00847
Castañón, A. M., Sanmiquel, L., Bascompta, M., Vega, Y., De La Fuente, A., Contreras, V., et al. (2021). Used tires as fuel in clinker production: economic and environmental implications. Sustainability 13, 10455. doi: 10.3390/su131810455
Christofi, N., Morton, J. G., and Bond, D. E. (2010). Rubber Treatment Method. US Patent No. 7,737,191B2.
Cui, X., Zhao, S., and Wang, B. (2016). Microbial desulfurization for ground tire rubber by mixed consortium-Sphingomonas sp., Gordonia sp. Polym. Degrad. Stab. 128, 165–171. doi: 10.1016/j.polymdegradstab.2016.03.011
Cummins, A. (2019). Global ELT Management - A Global State of Knowledge on Regulation, Management Systems, Impacts of Recovery and Technologies. Geneva: World Bus. Counc. Sustain. Dev.
Dabić-Ostojić, S., Miljuš, M., Bojović, N., Glišović, N., and Milenković, M. (2014). Applying a mathematical approach to improve the tire retreading process. Resour. Conserv. Recycl. 86, 107–117. doi: 10.1016/j.resconrec.2014.02.007
Delegan, Y., Kocharovskaya, Y., Frantsuzova, E., Streletskii, R., and Vetrova, A. (2021). Characterization and genomic analysis of Gordonia alkanivorans 135, a promising dibenzothiophene-degrading strain. Biotechnol. Rep. 29, e00591. doi: 10.1016/j.btre.2021.e00591
Denome, S. A., Oldfield, C., Nash, L. J., and Young, K. D. (1994). Characterization of the desulfurization genes from Rhodococcus sp. strain IGTS8. J. Bacteriol. 176, 6707–6716. doi: 10.1128/jb.176.21.6707-6716.1994
Denome, S. A., Olson, E. S., and Young, K. D. (1993). Identification and cloning of genes involved in specific desulfurization of dibenzothiophene by Rhodococcus sp. strain IGTS8. Appl. Environ. Microbiol. 59, 2837–2843. doi: 10.1128/aem.59.9.2837-2843.1993
Dick, T., Agboola, D. O., and O. Ayeni, A. (2020). Pyrolysis of waste tyre for high-quality fuel products: a review. AIMS Energy 8, 869–895. doi: 10.3934/energy.2020.5.869
Downard, J., Singh, A., Bullard, R., Jayarathne, T., Rathnayake, C. M., Simmons, D. L., et al. (2015). Uncontrolled combustion of shredded tires in a landfill – part 1: characterization of gaseous and particulate emissions. Atmos. Environ. 104, 195–204. doi: 10.1016/j.atmosenv.2014.12.059
ETRMA (2015). End-of-Life Tyre Report 2015. Press release (2015). Available online at: https://www.etrma.org/wp-content/uploads/2019/09/elt-report-v9a-final.pdf (accessed February 23, 2023).
ETRMA (2017). Europe - 92% of all End of Life Tyres Collected and Treated in 2017. Press Release (2019). Available online at: https://www.etrma.org/wp-content/uploads/2019/11/20191119-~Europe-92-of-all-End-of-Life-Tyres-collected-and-treated-in-2017.pdf (accessed February 23, 2023).
European Commission (2023). Commission Regulation (EU) amending REACH Regulation as Regards Synthetic Polymer Microparticles. Brussels: European Commission.
European Tyre and Rubber Manufacturers' Association (2021). End of Life Tyres Management (Press release). Brussels: ETRMA.
Formela, K. (2021). Sustainable development of waste tires recycling technologies – recent advances, challenges and future trends. Recycl. Neat Polym. 4, 209–222. doi: 10.1016/j.aiepr.2021.06.004
Formela, K., Cysewska, M., and Haponiuk, J. T. (2016). Thermomechanical reclaiming of ground tire rubber via extrusion at low temperature: efficiency and limits. J. Vinyl Addit. Technol. 22, 213–221. doi: 10.1002/vnl.21426
Formela, K., Hejna, A., Zedler, L., Colom, X., and Canavate, J. (2019). Microwave treatment in waste rubber recycling – recent advances and limitations. Express Polym. Lett. 13, 565–588. doi: 10.3144/expresspolymlett.2019.48
Ghavipanjeh, F., Ziaei Rad, Z., and Pazouki, M. (2018). Devulcanization of ground tires by different strains of bacteria: optimization of culture condition by taguchi method. J. Polym. Environ. 26, 3168–3175. doi: 10.1007/s10924-017-1169-0
Gilbert, S. C., Morton, J., Buchanan, S., Oldfield, C., and McRoberts, A. (1998). Isolation of a unique benzothiophene-desulphurizing bacterium, Gordona sp. strain 213E (NCIMB 40816), and characterization of the desulphurization pathway. Microbiology 144, 2545–2553. doi: 10.1099/00221287-144-9-2545
González, M. A., Rodríguez-Sosa, M. A., Vásquez-Bautista, Y. E., Rosario, E. C., Durán-Tiburcio, J. C., and Alarcón-Elbal, P. M. (2020). A survey of tire-breeding mosquitoes (Diptera: Culicidae) in the Dominican Republic: considerations about a pressing issue. Biomédica 40, 507–515. doi: 10.7705/biomedica.5200
Grynkiewicz-Bylina, B., Rakwic, B., and Słomka-Słupik, B. (2022). Tests of rubber granules used as artificial turf for football fields in terms of toxicity to human health and the environment. Sci. Rep. 12, 6683. doi: 10.1038/s41598-022-10691-1
Guo, L., Ren, D., Wang, W., Hao, K., Guo, X., Liu, F., et al. (2021). Low-temperature mechano-chemical rubber reclamation using terpinene as a swelling agent to enhance bond-breaking selectivity. Polymers 13, 4272. doi: 10.3390/polym13244272
Gutowski, T. G., Sahni, S., Boustani, A., and Graves, S. C. (2011). Remanufacturing and energy savings. Environ. Sci. Technol. 45, 4540–4547. doi: 10.1021/es102598b
Hoang, A. T., Nguyen, T. H., and Nguyen, H. P. (2020). Scrap tire pyrolysis as a potential strategy for waste management pathway: a review. Energy Sources Part Recovery Util. Environ. Eff . 1−18. doi: 10.1080/15567036.2020.1745336
Hrdlička, Z., Brejcha, J., Šubrt, J., Vrtiška, D., Malinová, L., Cadek, D., et al. (2022). Ground tyre rubber produced via ambient, cryogenic, and waterjet milling: the influence of milling method and particle size on the properties of SBR/NR/BR compounds for agricultural tyre treads. Plast. Rubber Compos. 51, 497–506. doi: 10.1080/14658011.2021.2008713
Hu, M., Zhao, S., Li, C., Wang, B., Fu, Y., Wang, Y., et al. (2016). Biodesulfurization of vulcanized rubber by enzymes induced from Gordonia amicalisa. Polym. Degrad. Stab. 128, 8–14. doi: 10.1016/j.polymdegradstab.2016.02.017
Hu, X., Zhao, H. N., Tian, Z., Peter, K. T., Dodd, M. C., Kolodziej, E. P., et al. (2022). Transformation product formation upon heterogeneous ozonation of the tire rubber antioxidant 6PPD (N-(1,3-dimethylbutyl)- N ′-phenyl- p-phenylenediamine). Environ. Sci. Technol. Lett. 9, 413–419. doi: 10.1021/acs.estlett.2c00187
Hussein, S. A., Al-Khafaji, Z., Alfatlawi, T. J. M., and Abbood, A-. K. N. (2022). Improvement of permeable asphalt pavement by adding crumb rubber waste. Open Eng. 12, 1030–1037. doi: 10.1515/eng-2022-0345
Isayev, A. I. (2001). “Recycling of elastomers,” in Encyclopedia of Materials: Science and Technology, eds K. H. J. Buschow, R. W. Cahn, M. C. Flemings, B. Ilschner, E. J. Kramer, et al. (Oxford: Elsevier), 2472–2474.
Isayev, A. I., Liang, T., and Lewis, T. M. (2014). Effect of particle size on ultrasonic devulcanization of tire rubber in twin-screw extruder. Rubber Chem. Technol. 87, 86–102. doi: 10.5254/RCT.13.87926
Jain, A. (2016). Compendium of Technologies for the Recovery of Materials/Energy from End of Life (EoL) Tyres. Osaka: U. N. Environ. Programme - Int. Environ. Technol. Cent.
Jiang, K., Shi, J., Ge, Y., Zou, R., Yao, P., Li, X., et al. (2013). Complete devulcanization of sulfur-cured butyl rubber by using supercritical carbon dioxide. J. Appl. Polym. Sci. 127, 2397–2406. doi: 10.1002/app.37542
Kayser, K., and Bielaga-Jonkeas, B. (1993). Utilization of organosulphur compounds by axenic and mixed cultures of Rhodococcus rhodochrous IGTS8. J. Gen. Microbiol. 139, 3123–3129. doi: 10.1099/00221287-139-12-3123
Konishi, J., Onaka, T., Ishii, Y., and Suzuki, M. (2000). Demonstration of the carbon-sulfur bond targeted desulfurization of benzothiophene by thermophilic Paenibacillus sp. strain A11-2 capable of desulfurizing dibenzothiophene. FEMS Microbiol. Lett. 187, 151–154. doi: 10.1111/j.1574-6968.2000.tb09152.x
Li, F., Zhang, X., Wang, L., and Zhai, R. (2022). The preparation process, service performances and interaction mechanisms of crumb rubber modified asphalt (CRMA) by wet process: a comprehensive review. Constr. Build. Mater. 354, 129168. doi: 10.1016/j.conbuildmat.2022.129168
Li, M. Z., Squires, C. H., Monticello, D. J., and Childs, J. D. (1996). Genetic analysis of the dsz promoter and associated regulatory regions of Rhodococcus erythropolis IGTS8. J. Bacteriol. 178, 6409–6418. doi: 10.1128/jb.178.22.6409-6418.1996
Li, Y., Zhao, S., and Wang, Y. (2011). Microbial desulfurization of ground tire rubber by Thiobacillus ferrooxidans. Polym. Degrad. Stab. 96, 1662–1668. doi: 10.1016/j.polymdegradstab.2011.06.011
Li, Y., Zhao, S., and Wang, Y. (2012a). Improvement of the properties of natural rubber/ground tire rubber composites through biological desulfurization of GTR. J. Polym. Res. 19, 9864. doi: 10.1007/s10965-012-9864-y
Li, Y., Zhao, S., and Wang, Y. (2012b). Microbial desulfurization of ground tire rubber by Sphingomonas sp.: a novel technology for crumb rubber composites. J. Polym. Environ. 20, 372–380. doi: 10.1007/s10924-011-0386-1
Lim, M., Safiuddin, M., Mannan, M., and Resdiansyah (2021). Material properties and environmental benefits of hot-mix asphalt mixes including local crumb rubber obtained from scrap tires. Environments 8, 24. doi: 10.3390/environments8060047
Martínez, J. D., Puy, N., Murillo, R., García, T., Navarro, M. V., Mastral, A. M., et al. (2013). Waste tyre pyrolysis – a review. Renew. Sustain. Energy Rev. 23, 179–213. doi: 10.1016/j.rser.2013.02.038
Matsui, T., Noda, K., Tanaka, Y., Maruhashi, K., and Kurane, R. (2002). Recombinant Rhodococcus sp. strain T09 can desulfurize DBT in the presence of inorganic sulfate. Curr. Microbiol. 45, 240–244. doi: 10.1007/s00284-002-3739-0
Matsui, T., Onaka, T., Tanaka, Y., Tezuka, T., Suzuki, M., Kurane, R., et al. (2000). Alkylated benzothiophene desulfurization by Rhodococcus sp. strain T09. Biosci. Biotechnol. Biochem. 64, 596–599. doi: 10.1271/bbb.64.596
Mohajerani, A., Burnett, L., Smith, J. V., Markovski, S., Rodwell, G., Rahman, M. T., et al. (2020). Recycling waste rubber tyres in construction materials and associated environmental considerations: a review. Resour. Conserv. Recycl. 155, 104679. doi: 10.1016/j.resconrec.2020.104679
Mohamed, M. E-. S., Al-Yacoub, Z. H., and Vedakumar, J. V. (2015). Biocatalytic desulfurization of thiophenic compounds and crude oil by newly isolated bacteria. Front. Microbiol. 6, 112. doi: 10.3389/fmicb.2015.00112
Mohebali, G., and Ball, A. S. (2008). Biocatalytic desulfurization (BDS) of petrodiesel fuels. Microbiology 154, 2169–2183. doi: 10.1099/mic.0.2008/017608-0
Molanorouzi, M., and Mohaved, S. O. (2016). Reclaiming waste tire rubber by an irradiation technique. Polym. Degrad. Stab. 128, 115–125. doi: 10.1016/j.polymdegradstab.2016.03.009
Mushunje, K., Otieno, M., and Ballim, Y. (2018). A review of waste tyre rubber as an alternative concrete consituent material. MATEC Web Conf. 199, 11003. doi: 10.1051/matecconf/201819911003
Noda, K., Watanabe, K., and Maruhashi, K. (2003). Cloning of a rhodococcal promoter using a transposon for dibenzothiophene biodesulfurization. Biotechnol. Lett. 24, 1875–1882. doi: 10.1023/A:1020926107636
Oomen, A. G., and De Groot, G. M. (2017). Evaluation of Health Risks of Playing Sports on Synthetic Turf Pitches with Rubber Granulate. RIVM Report. doi: 10.21945/RIVM-2017-0016
Ounsaneha, W., Buadit, T., and Rattanapan, C. (2020). Assessment of human health impact based on life cycle assessment: a case study of Thai retread tire. IOP Conf. Ser. Mater. Sci. Eng. 773, 012038. doi: 10.1088/1757-899X/773/1/012038
Pavilonis, B. T., Weisel, C. P., Buckley, B., and Lioy, P. J. (2014). Bioaccessibility and risk of exposure to metals and SVOCs in artificial turf field fill materials and fibers: bioaccessibility and risk of exposure to metals and SVOCs. Risk Anal. 34, 44–55. doi: 10.1111/risa.12081
Phadke, A. A., Chakraborty, S. K., and De, S. K. (1984). Cryoground rubber-natural rubber blends. Rubber Chem. Technol. 57, 19–33. doi: 10.5254/1.3535994
Riekstins, A., Baumanis, J., and Barbars, J. (2021). Laboratory investigation of crumb rubber in dense graded asphalt by wet and dry processes. Constr. Build. Mater. 292, 123459. doi: 10.1016/j.conbuildmat.2021.123459
Sabzekar, M., Zohuri, G., Chenar, M. P., Mortazavi, S. M., Kariminejad, M., Asadi, S., et al. (2016). A new approach for reclaiming of waste automotive EPDM rubber using waste oil. Polym. Degrad. Stab. 129, 56–62. doi: 10.1016/j.polymdegradstab.2016.04.002
Schneider, K., Bierwisch, A., and Kaiser, E. (2020a). ERASSTRI - European risk assessment study on synthetic turf rubber infill – part 3: exposure and risk characterisation. Sci. Total Environ. 718, 137721. doi: 10.1016/j.scitotenv.2020.137721
Schneider, K., de Hoogd, M., Haxaire, P., Philipps, A., Bierwisch, A., and Kaiser, E. (2020b). ERASSTRI - european risk assessment study on synthetic turf rubber infill – part 2: migration and monitoring studies. Sci. Total Environ. 718, 137173. doi: 10.1016/j.scitotenv.2020.137173
Schneider, K., de Hoogd, M., Madsen, M. P., Haxaire, P., Bierwisch, A., and Kaiser, E. (2020c). ERASSTRI - European risk assessment study on synthetic turf rubber infill – part 1: analysis of infill samples. Sci. Total Environ. 718, 137174. doi: 10.1016/j.scitotenv.2020.137174
Seghar, S., Asaro, L., Rolland-Monnet, M., and Aït Hocine, N. (2019). Thermo-mechanical devulcanization and recycling of rubber industry waste. Resour. Conserv. Recycl. 144, 180–186. doi: 10.1016/j.resconrec.2019.01.047
Sienkiewicz, M., Kucinska-Lipka, J., Janik, H., and Balas, A. (2012). Progress in used tyres management in the European Union: a review. Waste Manag. 32, 1742–1751. doi: 10.1016/j.wasman.2012.05.010
Simcox, N. J., Bracker, A., Ginsberg, G., Toal, B., Golembiewski, B., Kurland, T., et al. (2011). Synthetic turf field investigation in Connecticut. J. Toxicol. Environ. Health A 74, 1133–1149. doi: 10.1080/15287394.2011.586941
Simon, D. Á., and Bárány, T. (2023). Microwave devulcanization of ground tire rubber and its improved utilization in natural rubber compounds. ACS Sustain. Chem. Eng. 11, 1797–1808. doi: 10.1021/acssuschemeng.2c05984
Singh, A., Spak, S. N., Stone, E. A., Downard, J., Bullard, R. L., Pooley, M., et al. (2015). Uncontrolled combustion of shredded tires in a landfill – part 2: population exposure, public health response, and an air quality index for urban fires. Atmos. Environ. 104, 273–283. doi: 10.1016/j.atmosenv.2015.01.002
Singh, P., Singh, D. N., and Debbarma, S. (2023). Macro- and micro- mechanisms associated with valorization of waste rubber in cement-based concrete and thermoplastic polymer composites: a critical review. Constr. Build. Mater. 371, 130807. doi: 10.1016/j.conbuildmat.2023.130807
Tanaka, Y., Onaka, T., Matsui, T., Maruhashi, K., and Kurane, R. (2001). Desulfurization of benzothiophene by the gram-negative bacterium, Sinorhizobium sp. KT55. Curr. Microbiol. 43, 187–191. doi: 10.1007/s002840010285
Tanaka, Y., Yoshikawa, O., Maruhashi, K., and Kurane, R. (2002). The cbs mutant strain of Rhodococcus erythropolis KA2-5-1 expresses high levels of Dsz enzymes in the presence of sulfate. Arch. Microbiol. 178, 351–357. doi: 10.1007/s00203-002-0466-7
Tatangelo, V., Mangili, I., Caracino, P., Anzano, M., Najmi, Z., Bestetti, G., et al. (2016). Biological devulcanization of ground natural rubber by Gordonia desulfuricans DSM 44462T strain. Appl. Microbiol. Biotechnol. 100, 8931–8942. doi: 10.1007/s00253-016-7691-5
Tatangelo, V., Mangili, I., Caracino, P., Bestetti, G., Collina, E., Anzano, M., et al. (2019). Microbial desulfurization of ground tire rubber (GTR): characterization of microbial communities and rheological and mechanical properties of GTR and natural rubber composites (GTR/NR). Polym. Degrad. Stab. 160, 102–109. doi: 10.1016/j.polymdegradstab.2018.12.021
Valdés, C., Hernández, C., Morales-Vera, R., and Andler, R. (2021). Desulfurization of vulcanized rubber particles using biological and couple microwave-chemical methods. Front. Environ. Sci. 9, 633165. doi: 10.3389/fenvs.2021.633165
Wang, W., Ma, T., Lian, K., Zhang, Y., Tian, H., Ji, K., et al. (2013). Genetic analysis of benzothiophene biodesulfurization pathway of gordonia terrae strain C-6. PLoS ONE 8, e84386. doi: 10.1371/journal.pone.0084386
Williams, P. T. (2013). Pyrolysis of waste tyres: a review. Waste Manag. 33, 1714–1728. doi: 10.1016/j.wasman.2013.05.003
Xu, P., Feng, J., Yu, B., Li, F., and Ma, C. (2009). “Recent developments in biodesulfurization of fossil fuels,” in Biotechnology in China I, eds J.-J. Zhong, F.-W. Bai, and W. Zhang (Berlin: Springer), 255–274. doi: 10.1007/10_2008_16
Yee, D. A. (2008). Tires as habitats for mosquitoes: a review of studies within the Eastern United States. J. Med. Entomol. 45, 581–593. doi: 10.1603/0022-2585(2008)45[581:TAHFMA]2.0.CO;2
Keywords: waste rubber, tire recycling, desulfurization, microbial devulcanisation, circular economy
Citation: Goevert D (2024) The value of different recycling technologies for waste rubber tires in the circular economy—A review. Front. Sustain. 4:1282805. doi: 10.3389/frsus.2023.1282805
Received: 24 August 2023; Accepted: 11 December 2023;
Published: 05 January 2024.
Edited by:
Peng Lin, Delft University of Technology, NetherlandsReviewed by:
Spyridoula Gerassimidou, University of Leeds, United KingdomAntonella Patti, University of Catania, Italy
Copyright © 2024 Goevert. This is an open-access article distributed under the terms of the Creative Commons Attribution License (CC BY). The use, distribution or reproduction in other forums is permitted, provided the original author(s) and the copyright owner(s) are credited and that the original publication in this journal is cited, in accordance with accepted academic practice. No use, distribution or reproduction is permitted which does not comply with these terms.
*Correspondence: Dennis Goevert, ZC5nb2V2ZXJ0JiN4MDAwNDA7aHMtbWFubmhlaW0uZGU=