- 1Department of Civil Engineering, Kyushu University, Fukuoka, Japan
- 2Department of Industrial Engineering, Diponegoro University, Semarang, Indonesia
- 3Urban Institute, Kyushu University, Fukuoka, Japan
Interest in reducing the greenhouse gas emissions from conventional power generation has increased the focus on the potential use of hydrogen to produce electricity. Numerous life-cycle assessment (LCA) studies of hydrogen-based power generation have been published. This study reviews the technological and methodological choices made in hydrogen-based power generation LCAs. A systematic review was chosen as the research method to achieve a comprehensive and minimally biased overview of hydrogen-based power generation LCAs. Relevant articles published between 2004 and 2021 were identified by searching the Scopus and Web of Science databases. Electrolysis from renewable energy resources was the most widely considered type of hydrogen production in the LCAs analyzed. Fuel cell technology was the most common conversion equipment used in hydrogen-based electricity LCAs. A significant number of scenarios examine the use of hydrogen for energy storage and co-generation purposes. Based on qualitative analysis, the methodological choices of LCAs vary between studies in terms of the functional units, allocations, system boundaries, and life-cycle impact assessment methods chosen. These discrepancies were likely to influence the value of the environmental impact results. The findings of the reviewed LCAs could provide an environmental profile of hydrogen-based electricity systems, identify hotspots, drive future research, define performance goals, and establish a baseline for their large-scale deployment.
Introduction
Carbon dioxide (CO2) emissions from fossil fuels have been increasing since the beginning of the Industrial Era. They became the main contributor of anthropogenic emissions to the air from around 1950 and their relative share has kept rising until the present (Friedlingstein et al., 2022). Global CO2 emission reached a historical record of 33.1 gigatons (Gt) in 2018 (IEA, 2021a), at which time, coal-fired power generation alone emitted 10 Gt of CO2 (IEA, 2019a). Renewable energy adoption has been accelerating and is expected to contribute to approximately 30% of all CO2 emissions reduction that occurs between 2017 and 2060 (IEA, 2017). Renewable energy technologies are employed most intensively in the power sector, where it is intended to accelerate the transition to low carbon with applications in the transportation, building, and industry sectors (IEA, 2017). However, power generation with renewable energy sources like solar photovoltaic (PV) and wind power depends on the weather conditions and the time of day (i.e., day or night) and thus the same amount of electricity cannot be distributed constantly. As a consequence, flexible and reliable power generation relying on various energy sources is required to provide electricity when using renewable sources directly is not feasible (Peppas et al., 2021). Since hydrogen is a chemical energy carrier, it may be used as a storage option to balance seasonal fluctuations in providing electrical energy (IEA, 2019b; Peppas et al., 2021).
Numerous strategies have been prepared worldwide to encourage the deployment of hydrogen technologies, involving all stakeholders—government, business developers, investors, and citizens. As of March 2022, 21 governments have released hydrogen strategies, 27 countries have national hydrogen strategies in preparation, and 34 discuss their initial policies and pilot projects (Work Energy Council, 2022). In the IEA's Net Zero by 2050: A Roadmap for the Global Energy Sector, sectoral and technology milestones to guide the global journey to net-zero have been provided, where power is the primarily targeted sector, and hydrogen is one of the critical pillars of decarbonizing the global energy system (IEA, 2021b,c). Recently, Clean Hydrogen Joint Undertaking (JU) – a program intends to encourage research and innovation (R&I) initiatives in the European Union in clean hydrogen solutions and technologies, presents a set of prioritized actions divided into three pillars. The Clean Hydrogen JU Pillar 3, ‘Hydrogen End Uses: Clean heat and Power', aims to support European supply chain actors in developing a portfolio of clean, renewable and flexible heat and power generation solutions for all end user's needs (Clean Hydrogen JU, 2022). This program will aid in developing several hydrogen technologies, which are currently undergone on R&I phase but are projected to contribute to making climate neutrality achievable by 2050.
The use of life cycle assessment (LCA) in R&I stages has gained attention in recent years (Cucurachi et al., 2018) due to its capability to assess environmental impact throughout a product's life cycle. The advantage of LCA is that it evaluates an entire product comprehensively, which prevents any suboptimization that may arise from focusing on only a few processes. LCA enables the comparison of potential environmental impacts from various alternatives (Varun et al., 2009). The European Union even requires LCA as an essential part of the R&I projects for funding proposals (Clean Hydrogen JU, 2022). Regarding emerging hydrogen technology, the FC-HyGuide document, a guideline for conducting LCA of hydrogen technologies, has been provided and recommended by European Union (Lozanovski et al., 2011; Masoni and Zamagni, 2011). Other than FC-HyGuide guidelines, there is a new ongoing EU project developing SH2E LCSA (Life Cycle Sustainability Assessment) guidelines for fuel cell and hydrogen (FCH) systems, including guidelines for LCA (SH2E, 2022).
With the growing number of the hydrogen LCA studies, numerous review paper of hydrogen LCAs have been published. Bhandari et al. (2014) reviewed 21 studies that addressed LCA hydrogen production technologies in which an aggregate comparison from an ecological perspective was discussed. Valente et al. (2016) reviewed more LCA studies on hydrogen energy systems. Koj et al. (2019) performed a review of 32 LCA studies on Power-to-X, where fuels and final use for transport applications were discussed most frequently instead of hydrogen for power generation purposes. Rinawati et al. (2022) placed a stronger emphasis on hydrogen for mobility use LCAs. These studies succeeded in identifying the relevant methodological trends. However, there is a lack of detailed overview about technical aspects on hydrogen for power generation application.
The objective of this review is to synthesize hydrogen for electricity generation LCAs. Our primary goal is to provide an overview of the technological aspects of addressed hydrogen uses for power generation LCAs. Our secondary goal is to analyze the methodological choices in preparing qualitative analyses of hydrogen-based power generation LCAs. Our third goal is to present a quantitative analysis of the environmental impacts of hydrogen-based power generation LCAs.
Materials and methods
This systematic review follows general systematic review principles (Tranfield et al., 2003) and “the STARR-LCA” methodology, which is a standardized technique for assessing and reporting LCA studies (Zumsteg et al., 2012). This methodology is discussed in this section.
Some delimitation criteria were applied in the selection of potential articles. The first delimitation is the origin of publications; only articles published in peer-reviewed journals were considered. The second criterion is year of publication; only articles published in 2000 or later were considered for the review since the first set of International Organization for Standardization (ISO) standards on LCA was completed in 2000. The third criterion is the language of publication; only articles in the English language were considered in this systematic review.
The procedure for selecting potential articles started with a search of the Scopus and Web of Science (WOS) databases using specific keywords and Boolean operators. The combination of keywords and operators was “Life-Cycle Assessment OR Life-Cycle Analysis AND hydrogen AND Power Generation OR electricity Generation.” The initial search generated 400 articles, which included 179 articles from Scopus and 221 publications from the WOS. The selection of articles comprising the systematic review followed several stages, which were conducted according to the method used by Rinawati et al. (2022), as shown in Figure 1. Firstly, the raw data were filtered, considering only papers published in journals (four publications of non-peer-reviewed journal articles were excluded). Then, a series of duplication checks were performed using Excel, which were manually rechecked; 101 duplications were removed using Excel, and seven repeated articles were recognized and eliminated using manual inspection.
Following Becheikh et al. (2006), a two-step practical screening was carried out with a set of inclusion and exclusion criteria adopted from Valente et al. (2016). All papers that reported the environmental implications of hydrogen for power generation applications based on the LCA method were included in this systematic review. We included only process-based LCA studies since they provide a precise and complete basis for analysis. Any variation in the technical features (e.g., feedstock, primary energy source, hydrogen production method, hydrogen storage, and hydrogen-based electricity conversion technology) or methodological choices (e.g., functional units, system boundaries, geographical scope, and impact assessment method) identified a unique case in this systematic review. The exclusion criteria of the articles included articles that (i) did not cover the hydrogen production phase, (ii) had a large number of case studies (more than 30), (iii) performed an environmental evaluation that did not employ the LCA method, (iv) involved hydrogen as a by-product from a background process, and (v) in which the conversion system configuration was combined with another energy storage system like batteries. The inclusion and exclusion criteria were applied in the title and abstract analysis for the first practical screening as well as during the analysis of the full text for the second screening. Based on this process, 228 articles were excluded from the first screening. Subsequently, four relevant articles were found in the literature during the second screening, so a total of 68 potential articles were identified. After the second screening, 47 articles were excluded. This left a total of 21 studies that met all the criteria for inclusion.
The most important information about the methodological choices and technical aspects of the selected hydrogen-based power generation LCA studies was extracted using a modified coding scheme (Muench and Guenther, 2013; Rinawati et al., 2022), as shown in the Supplementary material. We identified a case study or scenario for each variation in technological aspects such as hydrogen production method, feedstock, primary energy source, hydrogen storage, power conversion technology, and geographical scope. The predictive scenarios for sensitivity analysis were not recognized as unique case studies. Based on the data extracted from the 21 LCA studies that met the inclusion criteria, 71 case studies were identified (see Table 1). Furthermore, qualitative analysis of technological aspects in hydrogen-based power generation LCAs were performed based on the number of case studies described in Sections Hydrogen production methods, Hydrogen feedstock and energy sources, Hydrogen storage, Hydrogen-based power generation conversion technologies and applications, and Geographical context. Qualitative analysis of methodological choices were referred to the number of articles due to their homogeneity in a single article. These results provided in Sections System boundary, Functional unit, Allocation, Life-cycle inventory, and Life-cycle impact assessment method.
Results
Scope of the reviewed studies
This section explains the scope of the reviewed hydrogen-based power generation LCAs, and provides an overview of the hydrogen production methods examined, the hydrogen feedstocks and energy sources considered, the hydrogen-based electricity conversion technologies addressed, and the geographical contexts analyzed.
Hydrogen production methods
Hydrogen can be produced by numerous techniques such as thermochemical, electrochemical, photochemical, biochemical, photocatalytic, electrical-thermochemical, photonic-biochemical or photo-electrochemical processes (Balat, 2008; Dincer and Acar, 2014). The hydrogen production methods investigated in the selected hydrogen-based power generation LCAs comprised electrochemical and thermochemical technologies (Figure 2). Other possible hydrogen production technologies—such as photochemical, biochemical, electrical-thermochemical, photonic-biochemical, and electrical-photonic conversion—were not represented among the studies. Forty-one of 71 hydrogen-based power generation LCA case studies evaluated electrochemical conversion, all of which employed the electrolysis technique. In contrast, thermochemical technology was found in the remaining cases (30 of 71 case studies). Steam reforming (SR) was the most common technique among the thermochemical category (17 of 30). In addition to SR, there are other thermochemical technologies such as autothermal reforming (ATR) (6 of 30), gasification (4 of 30), aqueous phase reforming (APR) (2 of 30), and cracking (1 of 30). Electrochemical LCA studies place a major emphasis on energy sources, whereas thermochemical LCA studies focus on feedstocks.
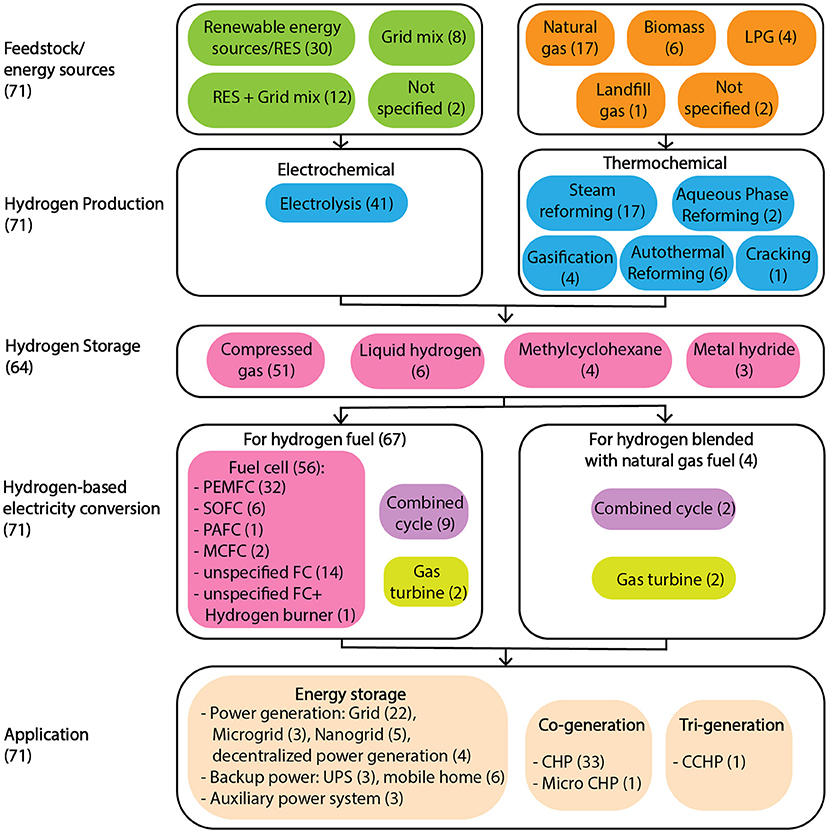
Figure 2. Overview of hydrogen-based power generation LCAs. The number in brackets indicates the number of cases in each particular area.
Hydrogen feedstock and energy sources
Among the electrochemical LCA studies, 73% of them used renewable energy sources (30 of 41 cases), including wind power (5/41), solar photovoltaic (11/41), hydropower (3/41), a combination of solar PV and wind power (2/41), and an unspecified renewable energy source (9/41), as shown in Figure 3. The grid mix, which was generated from non-renewable sources, was considered the energy source for hydrogen extraction in eight cases. In addition, the combination of renewable electricity with the grid mix was investigated in two cases. There was only one case that did not clearly explain its energy source. Among the thermochemical LCA studies, natural gas was the most common hydrogen feedstock (17/30) used in SR (11/17) and ATR (6/17). In addition, there are four cases in which LPG is used as the feedstock for the SR production process. Biomass was used for hydrogen production via gasification (4/30) and aqueous phase reforming (2/30). Only one case considered landfill gas as a hydrogen feedstock in the SR pathway, and two cases did not specify the feedstock that was used in SR and cracking processes.
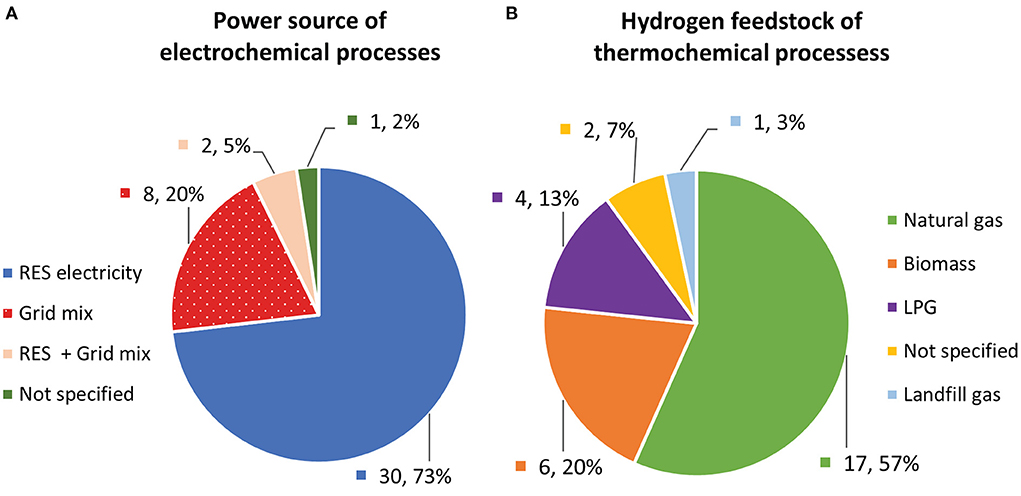
Figure 3. (A) Power sources of electrochemical cases and (B) hydrogen feedstock of thermochemical cases.
Hydrogen storage
Hydrogen contains 143 megajoules (MJ) of energy per kilogram, up to three times greater than liquid hydrocarbon-based fuels (Mazloomi and Gomes, 2012). However, storing the same amount of hydrogen requires a greater volume due to its low volumetric energy density. Conventional techniques for storing hydrogen include compressing it as gas in tanks, as cryogenic liquid, and storing it underground. Recently, material-based or solid-state hydrogen storage—which includes metal hydrides, complex hydrides, chemical hydrides, and adsorbents—has been rapidly developing (Yue et al., 2021). Hydrogen can be stored in gaseous form at higher pressure levels than 700 bar to increase the volumetric energy density, for example at hydrogen refueling stations the hydrogen is compressed to 900 bar to enable fast refueling (Reuß et al., 2017). Liquid hydrogen, which is achieved by cooling its temperature to −253°C, is another option for storing hydrogen. Despite significant improvements to volumetric density, the liquefaction process requires at least 35% of the fuel's energy content (Durbin and Malardier-Jugroot, 2013). Aside from compressing hydrogen in gas and liquid, hydrogen underground storage alternatives such as aquifers, depleted natural gas and oil reserves, and salt caverns are the main options for large-scale medium and long-term hydrogen storage (Yue et al., 2021). Storing hydrogen in a solid state is achieved by combining hydrogen with materials through absorption and adsorption. In adsorption, hydrogen attaches to the surface of material either as hydrogen atoms or hydrogen molecules. In absorption, hydrogen is split into H-atoms, and the H-atoms are then incorporated into chemical compounds (Durbin and Malardier-Jugroot, 2013; Tashie-Lewis and Nnabuife, 2021; Yue et al., 2021).
Our review identified 64 of 71 case studies that considered the storage stage (see Figure 2). The most common form of hydrogen storage was compressed gas (51/64). Liquid hydrogen was considered in six of 64 cases; apart from the two storage alternatives, numerous studies considered material-based hydrogen storage, including methylcyclohexane (MCH) (Ozawa et al., 2019) and metal hydrides for storing hydrogen (Hwang and Chang, 2010).
Hydrogen-based power generation conversion technologies and applications
The selection of hydrogen-based power generation conversion technology is mainly determined by the application for which the electricity is required. Hydrogen can directly react with oxygen in a fuel cell to supply electrical energy to a system or it can be burned in a combustion engine, such as a piston engine or a gas turbine (Tashie-Lewis and Nnabuife, 2021).
A fuel cell is an electrochemical cell that converts the stored chemical energy of hydrogen and oxygen directly into electricity. A fuel cell has four major components: this includes the anode, the cathode, the electrolyte, and the external circuit. At the anode, hydrogen is oxidized into protons and electrons, while at the cathode, oxygen is reduced to oxide ions, which subsequently react to produce water. Depending on the electrolyte, either protons or oxide ions are transmitted through an ion-conducting but electronically insulating electrolyte, while electrons flow around an external circuit delivering electrical energy. Based on the characteristics of the electrolyte, fuel cells can be classified into several types. However, they all run according to the same basic principles (Ormerod, 2003; Mekhilef et al., 2012). The five types of fuel cells considered for application in hydrogen-based power generation systems are alkaline fuel cells (AFC), phosphoric acid fuel cells (PAFC), solid oxide fuel cells (SOFC), molten carbonate fuel cells (MCFC), and proton exchange membrane fuel cells (PEMFC). AFC uses an aqueous solution of either sodium or potassium hydroxide as the electrolyte, and the electrodes are made from carbon with a platinum electrocatalyst. AFC has an operating temperature of around 70°C. PAFC use carbon paper electrodes and phosphoric acid electrolyte and has an operating temperature of up to 200°C. SOFC uses a solid ceramic inorganic as the electrolyte, which operates at high temperatures, typically between 750°C and 1,000°C. MCFC uses a molten potassium lithium carbonate electrolyte and has an operating temperature of around 650°C. PEMFC uses a proton-conducting polymer electrolyte and operates at low temperatures between 60°C and 100°C. Fuel cells generate a range of power from 1 to 10 MW, making them suitable for practically any application that requires electricity (Ormerod, 2003; Mekhilef et al., 2012). Fuel cell can be used in household appliances, transportation, portable power and stationary power generation (such as combined heat and power (CHP), auxiliary power, and backup power) (Cottrell et al., 2011).
The technologies for hydrogen gas turbine power generation are designed for large-scale power generation. These technologies are classified into natural gas–hydrogen co-combustion and hydrogen-fired power generation. A gas turbine generates rotary motion by harnessing the energy contained in a gas—either the kinetic energy of the movement of a flowing gas stream or the potential energy of a gas under pressure. A modern gas turbine has three principal components: this includes a compressor, a combustion chamber, and a turbine stage. The turbine stage is the main energy-producing component, as it drives the compressor while also providing the energy to power the generator and produce electricity. The most important adaptation of the gas turbine cycle, however, is the combined cycle power plant. The efficiency of a gas turbine for power generation is always restricted by the fact that exhaust gases exit the turbine at a high temperature and therefore still contain a significant amount of energy that is not recovered. In a combined cycle power plant, the exhaust from the gas turbine is fed into a heat recovery steam generator, which converts the hot air into steam. Then, the steam is used to power a steam turbine generator, which generates an additional amount of electricity (Breeze, 2019).
The conversion technologies investigated in the hydrogen-based power generation LCAs examined in this study include gas turbines, gas and steam turbines (combined cycle power), and fuel cells, as shown in Figure 2. The fuel cell, which was assessed in 56 case studies, was the most common conversion technology. Among the application of fuel cells to converting hydrogen into electricity, thirty-two case studies assessed the application of PEMFC (Khan et al., 2005; Spitzley et al., 2007; Mori et al., 2014; Oliveira et al., 2015; Valente et al., 2015; Di Marcoberardino et al., 2018; Stropnik et al., 2018; Suwanmanee et al., 2018; Rossi et al., 2020). Of these, Six case studies assessed SOFC (Strazza et al., 2010; Bicer and Khalid, 2020; Di Florio et al., 2021), and two cases assessed MCFC (Lunghi et al., 2004). Only one case investigated PAFC (Zhang et al., 2021). Still, sixteen case studies do not specify the type of fuel cells in their evaluation (Sevencan and Çiftcioglu, 2013; Shimizu et al., 2020; Peppas et al., 2021). This review found fewer cases of hydrogen mono-combustion and co-combustion LCAs addressing either combined cycle or gas turbine conversion. Within the hydrogen mono-combustion category, combined cycle turbines were investigated in nine case studies, and only two case studies discussed gas turbine conversion technologies. Four cases focused on hydrogen-enriched natural gas co-combustion conversion (Walker et al., 2017). Two cases considered gas turbines, and two cases examined gas combined cycle turbines.
As hydrogen plays an important role in a variety of applications for storing and transferring energy, in this review three typical applications of using hydrogen in power generation systems are addressed with the LCA approach—energy storage, co-generation, and tri-generation (Figure 2). Hydrogen-based energy storage has recently attracted increased attention as it can satisfy a wide range of energy storage needs, from controlling short-term system frequencies to balancing the medium and long-term (seasonal) energy supply and demand (Parra et al., 2019). Compared to existing energy storage alternatives such as pumped hydro energy storage and batteries, hydrogen has the advantages of providing a high-capacity means of storing energy, the ability to store energy for a long time, as well as general flexibility (Bocklisch, 2016). To improve efficiency and lower costs, fuel cells and gas turbines can be used as the prime movers for CHP systems known as “co-generation systems,” or it can be used for combined cold heat and power systems, known as “tri-generation systems.” In a co-generation system, the prime mover produces both electricity and heat, with the energy being used for electrical needs and the released heat being used for heating. Tri-generation is an application of co-generation that combines a primary mover with thermally driven equipment to generate cooling (Yue et al., 2021). In this review, some LCAs considered hydrogen as energy storage, including backup power (Sevencan and Çiftcioglu, 2013; Mori et al., 2014; Stropnik et al., 2018), power generation for the electrical grid (Lunghi et al., 2004; Khan et al., 2005; Oliveira et al., 2015; Valente et al., 2015; Walker et al., 2017; Ozawa et al., 2019; Zhang et al., 2021), decentralized power generation (Suwanmanee et al., 2018), power generation for a microgrid (Spitzley et al., 2007; Mori et al., 2021), power generation for nano-grid (Rossi et al., 2020; Di Florio et al., 2021), and auxiliary power (Strazza et al., 2010). Four studies provided LCAs of hydrogen for co-generation (Melamu and von Blottnitz, 2009; Di Marcoberardino et al., 2018; Bicer and Khalid, 2020; Shimizu et al., 2020), but only one study addressed the application of tri-generation systems (Peppas et al., 2021).
Geographical context
The geographical distribution of the LCAs is illustrated in Figure 4, along with their scope. The distribution is calculated based on the number of case studies rather than articles because one study considered cases from various regions (Stropnik et al., 2018) and provided LCAs in both the Norwegian and Moroccan contexts. More than half of the hydrogen-based power generation LCA case studies were examined in a European context (33 of 71). In this category, the countries that are most frequently represented include Italy (17 of 33), Germany (6 of 33), Belgium (4 of 33), Greece (1 of 33), Norway (1 of 33), Spain (1 of 33), and Slovenia (1 of 33). Yet, two case studies in the European context did not specify the country. Thirty-two of the 33 LCA cases on the European context focused on fuel cell applications, and only one case examined a gas turbine in mono-combustion conversion. Twenty-eight of the LCA case studies undertaken in the Asian context, including Japan (16/28), Turkey (6/28), Thailand (4/28), and China (2/28), considered fuel cell utilization and combined cycle turbines for mono-combustion conversion. By contrast, a limited number of case studies can be observed in the North American (7/71) and African (3/71) contexts.
Methodological choices
This qualitative analysis investigated the influencing methodological choices and was based on 21 hydrogen-based power generation LCAs.
System boundary
The system boundary specifies the specific system processes that are evaluated in a study. A one-dimensional definition of the system boundary is as either a “cradle-to-gate” or “cradle-to-grave” study; this classification is therefore insufficient for hydrogen-based power generation LCAs. Furthermore, the definition of the system boundary in hydrogen-based power systems is characterized by two paths. The first path is the hydrogen life cycle, which includes hydrogen production, storage, distribution, and use. In this case, a study is classified as cradle-to-grave if the scope includes hydrogen production and its use for power generation. The second path comprises the conversion system's manufacturing, operation, maintenance, and disposal. A study is labeled as a cradle-to-grave study if its scope covers all phases from manufacturing to disposal; otherwise, it is classified as a cradle-to-gate study.
Regarding the hydrogen life cycle of reviewed studies, hydrogen production and utilization are included in all LCAs (Figure 5). Hydrogen storage is generally included in LCAs, whereas only two of the studies excluded storage. Transportation was only included in eight studies. In terms of the conversion life-cycle equipment, all LCAs incorporated equipment operation. Therefore, equipment manufacturing is generally included in the scope of an LCA, whereas only three studies excluded this phase. Equipment maintenance and the end-of-life phase were only included in six and seven studies, respectively. Due to a lack of data on conversion system disposal and the fact that the studies are comparative LCAs, which would have a similar “end-of-life” phase for the various systems, excluding the end-of-life phase may be deemed an acceptable approximation of the goal of the studies (Di Florio et al., 2021).
Functional unit
The functional unit is an essential element of LCAs. It is a referencing unit for all environmentally relevant flows and impact assessment results. This review found that the functional units defined in LCAs of hydrogen for power generation are not uniform (Table 2). Figure 6 shows the number of times a functional unit was chosen in the reviewed studies. The amount of produced electricity in kilowatt hours (kWh) or megawatt hours (MWh) is the most extensively selected functional unit. Certain LCAs used case-specific of energy output-related functional units, such as the annual energy provided for one, two, or 10 dwellings (Di Marcoberardino et al., 2018), the use of hydrogen technologies in a specific region or area for 1 year (Shimizu et al., 2020), total power generation for 1 year (Sevencan and Çiftcioglu, 2013), and full coverage of energy demands for 20 years (Peppas et al., 2021). Only one study used exergy (in J) as a functional unit. Lastly, only one study used energy (in MJ) as a functional unit. Even though all functional units are energy output-related, a high discrepancy of functional units is seen. The purpose of hydrogen-based electricity technology application is responsible for the differences of functional units selected by reviewed studies. The main product of hydrogen-based power is electricity or electricity and useful heat, depending on their purposes. FC-HyGuide recommends using exergy as functional unit when both electricity and useful heat are generated and utilized (Lozanovski et al., 2011; Masoni and Zamagni, 2011). The exergy is defined as the sum of electricity (in MJ) plus the useful thermal energy (in MJ) times a Carnot coefficient. Adopting exergy could help avoid high discrepancies in selected functional units among the reviewed studies.
Allocation
Allocation rules were considered in LCA studies with multifunctional systems in which multiple outputs were produced. Despite the significance of allocation, many hydrogen-based power LCAs did not provide transparency regarding the allocation method applied. The information provided by the reviewed studies is scarce, since only two studies explicitly mentioned their allocation method. Energy efficiency allocation was selected to calculate the specific energy output in a combined heat and power plant (Bicer and Khalid, 2020). Physical allocation was used to examine the environmental impact of each reference flow of the system, which considers the hydrogen produced at the end of the equipment's life cycle as a by-product (Rossi et al., 2020). Additionally, an expansion system considered a multifunctional system was chosen in which conventional methods of producing electricity and transport fuel were applied, so that all scenarios were compared based on the same set of functions (Melamu and von Blottnitz, 2009).
Life-cycle inventory
The source of data for the life-cycle inventory step and their quality strongly affected the reliability of the assessment. Inputs and outputs for both foreground and background elements were used as representatives for the entire system. A definition of the foreground and background systems must be performed prior to the selection of data sources in LCI stage. The foreground system, according to FC-HyGuide, consist of the main process phases and the related infrastructure processes such as manufacturing. The foreground system is supported by the background system which is made up of processes such as the infrastructure for the supply of energy including power plants and power lines.
In general, there are two types of data used in a LCA study: primary and secondary data. Primary data is recommended to be used for the main processes (foreground system). Primary data is provided from on-site measurement, project partners, manufacturer and/or operator of the system. However, the owner, project partner, manufacturer or operator of the system may not have all of the data required to perform LCA. In that case, secondary data is needed to fill data gaps. Secondary data is also used for the background system. Different data sources can be used for secondary data, such as LCA databases, scientific literature, non-scientific literature, simulations, calculations, assumptions, etc.
The data source distribution for both foreground and background processes is shown in Figure 7. Due to the homogeneity of the data source used in the study, this figure was set according to the source of a single article. “On-site measurement” refers to gathering data from actual measurements either in the field or lab scale. Data from the manufacturer was referred to as “manufacturer,” while “scientific literature” implies published articles. Data from the project partner was classified as “project documentation.” Expert estimation and assumptions were classified in the “other” category. Scientific literature and LCA databases, such as ecoinvent, GaBi, IDEA, BUWAL, and The Argonne's The Greenhouse Gases, Regulated Emissions, and Energy Use in Transportation (GREET) databases were widely used for the foreground process. For the background process, data were generally obtained from the LCA database. The database choice was based on various criteria, such as the geographical context and the LCA software.
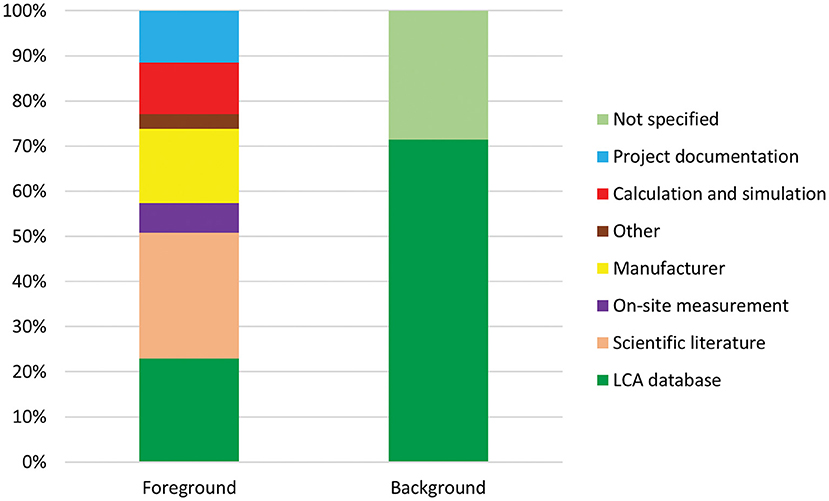
Figure 7. Data source distribution for foreground and background processes of the energy systems used in LCAs.
Life-cycle impact assessment method
There are two distinct impact categories—the midpoint (problem-oriented) approach and the endpoint (damage-oriented) approach. The midpoint approach evaluates the environmental impact in the middle of the environmental cause-and-effect chain. In contrast, the endpoint approach concentrates on the damage that occurs at the end of the chain (i.e., human health, ecosystem quality, and resources) (Guinée, 2002). The midpoint approach provides a more detailed and scientific decision-making foundation, while the endpoint approach is easier to interpret and communicate to decision-makers (Bare et al., 2000; Dong and Ng, 2014). Several of the studies reviewed either applied a midpoint approach (14 of 21) or an endpoint approach (3 of 21), whereas four of the studies utilized both the midpoint and endpoint approaches (Table 2).
The commonly selected impact categories for midpoint and endpoint approaches and the life-cycle impact assessment (LCIA) methods used are illustrated in Figures 8, 9, respectively. These methodological families were recognized without regard for version discrepancies. When a study employed its formula or model in the quantification process, the LCIA method was classified as “other.” Due to the homogeneity of the impact indicators and LCIA methods employed in a study, both figures were set according to the choices of a single article. The secondary axis of the figure indicates the total number of studies considering each impact category. Numerous LCIA methods were applied in the reviewed studies, including Centrum voor Milieukunde Leiden (CML), ReCiPe, IMPACT2002+, Environmental Footprint (EF), LIME2, Intergovernmental Panel on Climate Change (IPCC), GREET, cumulative energy demand (CED), and Eco-indicator 99.
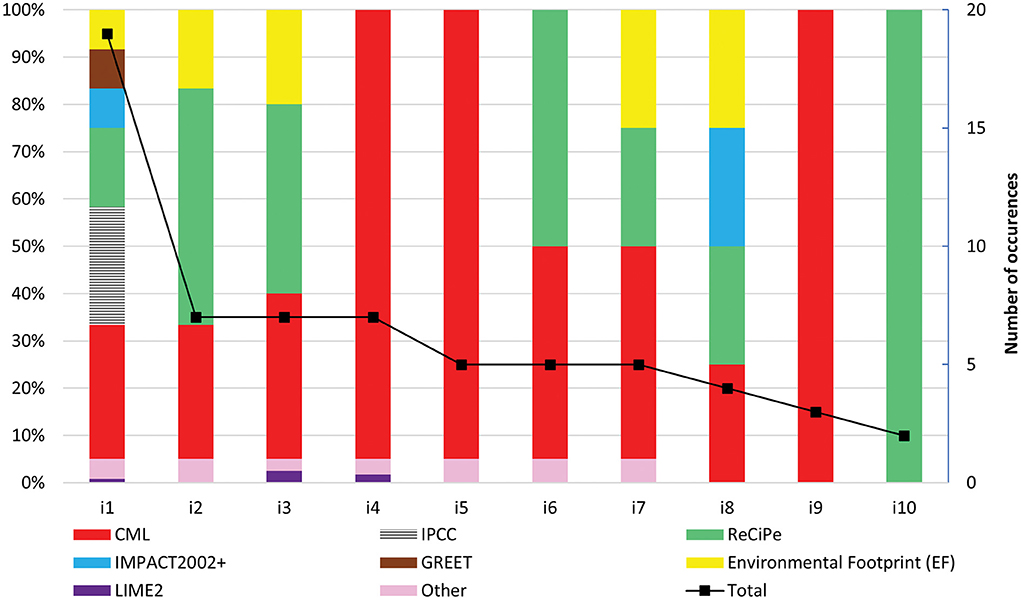
Figure 8. Choice of midpoint impact approach and their respective LCIA methods. i1: global warming potential (GWP), i2: human toxicity potential (HTP), i3: photochemical ozone creation potential (POCP), i4: acidification potential (AP), i5: eutrophication potential (EP), i6: particulate matter formation (PMF), i7: ozone depletion potential (ODP), i8: water depletion (WD), i9: abiotic depletion potential (ADP), and i10: fossil depletion (FD).
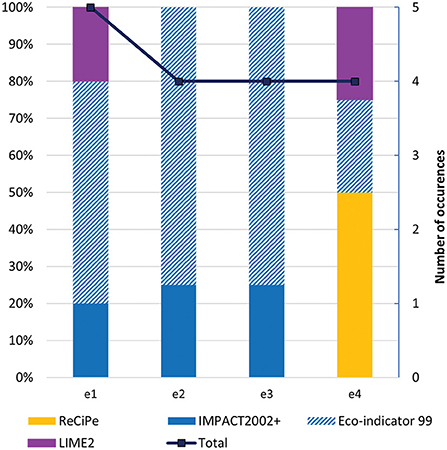
Figure 9. Choice of endpoint impact categories and their respective LCIA methods. e1: human health (HH), e2: ecosystem quality (EQ), e3: resources (R), e4: single score (SS).
Figure 8 presents commonly selected impact categories for the midpoint approach, including global warming potential (GWP), human toxicity potential (HTP), photochemical ozone creation potential (POCP), acidification potential (AP), eutrophication potential (EP), particulate matter formation (PMF), ozone depletion potential (ODP), water depletion (WD), abiotic depletion potential (ADP), and fossil depletion (FD). Based on the impact category choices in the LCIA phase, GWP was the most often examined impact indicator in hydrogen LCAs, followed by HTP and POCP. Regarding the LCIA methods for the midpoint approach, CML was the most widely used method for evaluating GHG emissions. IPCC was used to quantify GWP in three of the studies; CML also played a significant role in quantifying the other midpoint categories, including HTP, POCP, AP, EP, PM, ODP, WD, and ADP. ReCiPe was employed to characterize GWP, HTP, POCP, PM, ODP, WD, and FD. GREET was only used to quantify GHG emissions, whereas LIME2 was utilized to evaluate GWP, POCP, and AP. The EF method was applied to assess GWP, HTP, POCP, ODP, WD, and FD. Other than these impact categories, other midpoints were investigated in several of the reviewed studies, including marine ecotoxicity (METP), freshwater ecotoxicity (FETP), terrestrial ecotoxicity (TETP), terrestrial acidification (TAP), freshwater eutrophication (FEP), marine eutrophication (MEP) (Stropnik et al., 2018; Di Florio et al., 2021; Mori et al., 2021), ionizing radiation (Di Florio et al., 2021; Mori et al., 2021), fossil resources scarcity, mineral resource scarcity, water consumption (Di Florio et al., 2021, urban air pollution (Shimizu et al., 2020), land use (Valente et al., 2015; Di Florio et al., 2021), and energy use (Strazza et al., 2010; Valente et al., 2015; Di Florio et al., 2021). Two authors applied the CED method to evaluate energy use over the life cycle of the system under study (Valente et al., 2015; Di Florio et al., 2021). A few studies addressed endpoint categories, including human health, ecosystem quality, resource damage, and single scores (see Figure 9). Eco-indicator 99 was employed in all the indicators of the endpoint approach. Conversely, ReCiPe was only used to evaluate single-score indicators. IMPACT2002+ was applied to assess human health, ecosystem quality, and resources. In addition, LIME2 was utilized to evaluate human health and single-score indicators. Generally, the selection of the LCIA method for both midpoint and endpoint approaches in the reviewed studies depended on their environmental goals and ecosystem characteristics.
The distribution of the LCA software or tools used during the LCIA phase is shown in Figure 10. Because of homogeneity of the LCA software or tools employed in a study, a pie chart was created according to the choices of a single article. SimaPro and GaBi were widely used in the reviewed LCAs. Open LCA, GREET, and in-house software was utilized at similar frequencies. Many studies explicitly mentioned the software used.
Environmental impact of hydrogen-based power generation lcas
This section presents a quantitative assessment of the LCA results. Studies that did not provide comparable data were excluded from the quantitative analysis for the following reasons: (i) certain LCAs reported impact categories exclusively in graphical data, (ii) certain LCAs reported hydrogen-enriched natural gas power generation, and (iii) several LCAs used case-specific functional units, such as the annual demand for a specific system. The quantitative analysis concentrated on the commonly used midpoint impact categories of hydrogen-based power, including GWP, POCP, AP, and EP. The LCA results are presented according to homogeneous functional units and have been recalculated in the respective equivalency units.
Global warming potential
The analysis of GWP (Figure 11) was based on 19 case studies from eight studies. The GWP of a hydrogen-based power generation system has a median of 289 g/CO2 eq. per kWh of produced electricity and ranges from 17.29 grams of carbon dioxide equivalent (g CO2 eq.) per kWh to 4,040 g CO2 eq. per kWh.
Other impact categories
The other impact results discussed in the LCAs include POCP, AP, EP, and ODP. However, the few case studies that did so prevented a comprehensive quantitative analysis. The results are presented in Table 3.
Discussion
Technical aspects of conversion technology
In this section, the description about the construction of fuel cells is discussed. We do not discuss the construction of gas turbine and combined cycle turbine due to absence of detailed description in the reviewed studies. The amount of electricity generated by a fuel cell is determined by various parameters, including the type of fuel cell, its size, operating temperature, and gas supply pressure. To boost the voltage, individual fuel cells are connected in series to form a stack. A fuel cell stack might comprise a few or hundreds of individual cells, depending on the purpose. The stack construction consists of electrodes, matrixes, and bipolar plates production and their relative assembly (Lunghi and Bove, 2003).
In the case of fuel cells stack/system, FC-HyGuide requests a brief description of the FC system or stack. Information about the major properties needs to be given by stating the FC standard being met, such as: IEC/TS 62282-1 and IEC 62282-2. If no standard applies, FC-HyGuide asks that the following properties be reported: trade name, type of electrolyte used, primary functions, electrical power (rated output), thermal power, efficiency, rated voltage, rated current, range of temperatures and operating temperature, weight, dimensions, duel used and its technical specifications, expected service life, and description of the intended use. Within the reviewed studies, highly detailed description about the construction and operation of selected fuel cells or gas turbine are scarce.
Within the reviewed publications, fuel cells can be used for a wide variety of application, PEMFC for power grid purposes (250 kW, 500 kW), SOFC for auxiliary power system (20 kW), PEMFC for backup power (3 kW), SOFC for CHP (250 kW) and PAFC for power grid (50 MW).
Level of accordance with fc-hyGuide
The result of the evaluation on methodological choices of reviewed studies regarding the level of agreement with a selected set of recommendations from the FC-HyGuide are summarized in Table 4. In this table, the level of accordance is classified as “very high” if more than 90% of studies followed the corresponding FC-HyGuide recommendation, “high” (60–90%), “intermediate” (40–60%), “low” (10–40%) and “very low” (<10%). According to the time of publication of the reviewed studies, the level of agreement is provided in the columns “before FC-HyGuide” and “after FC-HyGuide”. Regarding product system information, it can be seen that the level agreement before and after the publish of the FC-HyGuide is quite similar. Furthermore, a highly satisfying level of agreement is generally obtained both before and after FC-HyGuide in terms of goal and scope definition, despite a contrary tendency in accordance with the use of an attributional modeling approach (from “high” to “intermediate”). On the other hand, dealing with using ISO hierarchy for solving multifunctional process, unsatisfactory level of agreement has found before the release of FC-HyGuide. In regard with LCI, the lack of information about data quality requirement and definition of foreground and background processes are found both before and after the release of FC-HyGuide. While the use of primary data was assessed as intermediate before and after FC-HyGuide. In contrast, a satisfactory level of agreement regarding filling data gaps with secondary data is found both before and after FC-HyGuide. Concerning LCIA, a downward trend is generally observed when comparing the studies before and after FC-HyGuide, despite an increase level of agreement for selecting the LCIA method and reporting non-normalized and non-weighted LCA results. In terms of impact categories, FC-HyGuide recommends the use of midpoint impact categories instead of endpoint categories, the use of GWP, AP, EP, ADP, primary energy demands (renewable and non-renewable), and other categories such as ODP, HTP, LU etc. (Lozanovski et al., 2011; Masoni and Zamagni, 2011). Regarding the LCIA method, FC-HyGuide suggests selecting recommended methods by JRC (European Commission - Joint Research Centre., 2011) or the midpoint CML method. JRC recommends LCIA methods for relevant environmental impact categories, for instance, the IPCC method is endorsed for evaluating GWP. Using the CML method or JRC recommendation reaches an intermediate level of agreement in the reviewed paper published after FC-HyGuide was announced. Despite the intermediate level of agreement in LCIA method with FC-HyGuide, the use of AP, EP and ADP impact categories are “low” since some studies only focused on GWP impact category.
LCA result
This section discusses the LCA results for the various studies included in this systematic review. We only included GWP to ensure that there were enough cases for an in-depth discussion. This measure does not imply that the other impact categories are less important. A wide range of reported results (Figure 11) can be attributed to an outlier above the upper fence. This outlier was derived from a case study reporting a system in which hydrogen was produced through electrolysis from a grid mix and converted into electricity in a PEMFC for UPS application (Stropnik et al., 2018). Moreover, the system boundary of the outlier case study was both cradle-to-grave for the hydrogen life cycle and conversion equipment life cycle, in contrast to other studies that conducted cradle-to-gate LCA analysis excluding the end-of-life phase. Furthermore, different LCA studies of similar hydrogen-based electricity pathways frequently provide divergent results, emphasizing the need to harmonize the LCA methodology in hydrogen-based electricity studies.
These discrepancies between the environmental impact results were likely caused by variations in technical and methodological choices in preparing the LCA such as functional units, allocation, system boundaries, and LCIA methods. These include options regarding hydrogen pathways and conversion equipment for technical decisions. Furthermore, a robust comparison of the environmental results from various studies with different methodologies cannot be conducted without a harmonization procedure.
Finally, it is essential to note that LCA merely provides an environmental profile of hydrogen-based power generation technologies, but other aspects are equally important, such as socioeconomic factors. Integrating LCA research with economic analysis, such as life cycle costing or techno-economic assessments, is highly recommended. Numerous reviewed studies have addressed economic analysis (Di Marcoberardino et al., 2018; Mori et al., 2021; Zhang et al., 2021). Integrating public acceptance and social effect assessments into the research and development process could be critical for the future deployment of hydrogen-based power generation. Several LCIA approaches for quantifying products' social and sociological impact have been developed in recent years. This LCIA method is known as the social LCA (S-LCA) method. Its typical impact categories represent the five main stakeholder groups of the product supply chain—workers, consumers, the local community, society, and value chain actors (United Nations Environment, 2020). However, no studies that addressed social LCA were encountered during this systematic review.
Conclusion and outlook
Hydrogen-based electricity generation LCAs were synthesized in this systematic review. Although the number of LCA studies has grown over the last 4 years, an inherent limitation of this systematic review is the small number of available studies and scenarios. The findings of the reviewed LCAs could help us evaluate the life-cycle environmental impact of hydrogen-based electricity systems, identify hotspots, direct future research, set performance goals, and provide a baseline for large-scale applications.
To address the first objective of this review, a qualitative analysis of the technological aspects of hydrogen-based electricity was performed. Electrolysis from renewable energy resources was the most widely considered hydrogen production method in the LCAs. In addition, storing hydrogen in compressed gaseous form was the most often used storage option. Fuel cell technology was the most common conversion equipment used in hydrogen-based electricity LCAs. Only a few studies have reported gas turbine and combined cycle with pure hydrogen fuel or hydrogen mixed with natural gas fuel. Many scenarios also focused on using hydrogen for energy storage and co-generation purposes. We identify some significant knowledge gaps and technology difficulties. Performing a LCA studies requires sufficient data and information about material and energy inputs and outputs, as well as the cause-effect relationships throughout the entire supply-chain of a technology which is generally obtainable in mature technology. The lack of data availability as well as uncertainty in the data and findings of LCA studies are common, especially for low Technology Readiness Level (TRL) technologies. Such is the case for many hydrogen-based power systems. On the other hand, early technology assessment offers a great chance to improve design and environmental profile. Hence, considering both level of technology maturity and the level of maturity of the market into which the technology will be implemented, are significant elements in emerging technology evaluations.
To address the secondary objective of this systematic review, a qualitative analysis of the methodological choices made in the hydrogen-based electricity LCAs was conducted. Based on the results, the methodological choices differed between studies, including functional units, allocation, system boundaries, and LCIA methods. Based on the observed methodological trends of reviewed studies and its accordance with FC-HyGuide, the quality of data and limitation of data availability should be reported. The selection of impact categories and impact assessment method should be done in accordance with FC-HyGuide. When conducting LCA with multi-functional concerns, ISO recommends using the multi-functionality hierarchy with system expansion or allocation (ISO, 2006). In the case of allocation of hydrogen-based power should be avoided, there is a possible way either by adopting exergy as functional unit or applying system expansion.
Due to uncertainty of endpoint indicators and single scores, FC-HyGuide suggest using midpoint indicators in the assessment. Therefore, the following ISO recommendations would be the appropriate approach: providing results with midpoint indicators (mandatory) and using endpoint indicators and particularly, single scores (optionally) only when comparing declaration are not to be disclosed to the public. LCA should not be restricted to climate change impacts only. Instead, a wide range of environmental impacts should be examined to prevent shifting the burden to other impact categories, such as reducing climate change impact yet raising human toxicity. These tradeoffs should be thoroughly examined.
To address the third aim, a quantitative analysis of environmental impact results was conducted. Based on GWP, the most extensively discussed impact indicator, hydrogen-based power generation systems, had a median of 289 g CO2 eq. per kWh of electricity produced and ranged from 17.29 g CO2 eq. per kWh to 4,040 g CO2 eq. per kWh. These extreme values were likely caused by various technical and methodological choices in the preparation of the LCA. For this reason, a reliable comparison of the LCA results of multiple studies with varying methodological and technical options is not possible without first creating a harmonization procedure.
A lack of full traceability of results was noted from the reviewed studies. Only a few studies which identify the critical issues by quantifying which process/ flows are major contributors to the total impact. For future LCA studies, along with FC-HyGuide we recommend showing the contribution of significant processes to the total impact in stacked columns or pie-charts for identifying hotpots.
Lastly, it is essential to note that LCA only presents the environmental profile of a prospective product. However, other aspects, such as socioeconomic issues, are just as important. Economic considerations have been examined in a few of the reviewed studies. However, no studies that addressed social impact were encountered during this systematic review. Applying the social LCA (S-LCA) methodology to the research and development process may be beneficial in understanding the future impact of the hydrogen-based power generation more holistically.
Data availability statement
The raw data supporting the conclusions of this article will be made available by the authors, without undue reservation.
Author contributions
DIR carried out the review with support from AK and ST. DIR and AK wrote the manuscript. SM helped supervise the project. All authors contributed to the article and approved the submitted version.
Funding
This research was supported by the New Energy and Industrial Technology Development Organization under a Grant (number P14026).
Acknowledgments
DIR would like to thank Diponegoro University, The Ministry of Education and Culture of the Republic of Indonesia for the full support through the Doctoral Degree Scholarship Program (Grant No. 8205/UN7.P2/KS/2019).
Conflicts of interest
The authors declare that the research was conducted in the absence of any commercial or financial relationships that could be construed as a potential conflict of interest.
Publisher's note
All claims expressed in this article are solely those of the authors and do not necessarily represent those of their affiliated organizations, or those of the publisher, the editors and the reviewers. Any product that may be evaluated in this article, or claim that may be made by its manufacturer, is not guaranteed or endorsed by the publisher.
Author disclaimer
Any opinions, findings, and conclusions expressed in this paper are those of the authors and do not necessarily reflect the views of the funding agency.
Supplementary material
The Supplementary Material for this article can be found online at: https://www.frontiersin.org/articles/10.3389/frsus.2022.920876/full#supplementary-material
References
Balat, M. (2008). Potential importance of hydrogen as a future solution to environmental and transportation problems. Int. J. Hydrog. 33, 4013–4029. doi: 10.1016/j.ijhydene.2008.05.047
Bare, J. C., Hofstetter, P., Pennington, D. W., and Udo De Haes, H. A. (2000). State-of-the-art, LCIA midpoints versus endpoints, The sacrifices and benefits. Int. J. Life Cycle Assess. 5, 319–326. doi: 10.1007/BF02978665
Becheikh, N., Landry, R., and Amara, N. (2006). Lessons from innovation empirical studies in the manufacturing sector, a systematic review of the literature from 1993-2003. Technovation. 26, 644–664. doi: 10.1016/j.technovation.2005.06.016
Bhandari, R., Trudewind, C. A., and Zapp, P. (2014). Life cycle assessment of hydrogen production via electrolysis - a review. J. Clean. Prod. 85:151–163. doi: 10.1016/j.jclepro.2013.07.048
Bicer, Y., and Khalid, F. (2020). Life cycle environmental impact comparison of solid oxide fuel cells fueled by natural gas, hydrogen, ammonia and methanol for combined heat and power generation. Int. J. Hydrog. 45, 3670–3685. doi: 10.1016/j.ijhydene.2018.11.122
Bocklisch, T. (2016). Hybrid energy storage approach for renewable energy applications. J. Energy Storage. 8, 311–319. doi: 10.1016/j.est.2016.01.004
Breeze, P. (2019). Power Generation Technologies (Third edition). Oxford: Elsevier Ltd. doi: 10.1016/B978-0-08-102631-1.00014-6
Clean Hydrogen JU. (2022). Clean Hydrogen Joint Undertaking (Clean Hydrogen JU) Work Programme 2022. Available online at: https://www.clean-hydrogen.europa.eu/system/files/2022-02/Clean%20Hydrogen%20JU%20AWP%202022_0.pdf (accessed June 12, 2022)
Cottrell, C. A., Grasman, S. E., Thomas, M., Martin, K. B., and Sheffield, J. W. (2011). Strategies for stationary and portable fuel cell markets. Int. J. Hydrog. 36, 7969–7975. doi: 10.1016/j.ijhydene.2011.01.056
Cucurachi, S., Van Der Giesen, C., and Guinée, J. (2018). Ex-ante LCA of emerging technologies. Procedia CIRP. 69, 463–468. doi: 10.1016/j.procir.2017.11.005
Di Florio, G., Macchi, E. G., Mongibello, L., Baratto, M. C., Basosi, R., Busi, E., et al. (2021). Comparative life cycle assessment of two different SOFC-based cogeneration systems with thermal energy storage integrated into a single-family house nanogrid. Appl. Energy. 285, 116378. doi: 10.1016/j.apenergy.2020.116378
Di Marcoberardino, G., Manzolini, G., Guignard, C., and Magaud, V. (2018). Optimization of a micro-CHP system based on polymer electrolyte membrane fuel cell and membrane reactor from economic and life cycle assessment point of view. Chem. Eng. Process. 131, 70–83. doi: 10.1016/j.cep.2018.06.003
Dincer, I., and Acar, C. (2014). Review and evaluation of hydrogen production methods for better sustainability. Int. J. Hydrog. 40, 11094–11111. doi: 10.1016/j.ijhydene.2014.12.035
Dong, Y. H., and Ng, S. T. (2014). Comparing the midpoint and endpoint approaches based on ReCiPe - a study of commercial buildings in Hong Kong. Int. J. Life Cycle Assess. 19, 1409–1423. doi: 10.1007/s11367-014-0743-0
Durbin, D. J., and Malardier-Jugroot, C. (2013). Review of hydrogen storage techniques for on board vehicle applications. Int. J. Hydrog. 38, 14595–14617. doi: 10.1016/j.ijhydene.2013.07.058
European Commission - Joint Research Centre. (2011). International Reference Life Cycle Data System (ILCD) Handbook- Recommendations for Life Cycle Impact Assessment in the European context. First edition. Luxemburg: Publication Office of the European Union. Available online at: https://www.eplca.jrc.ec.europa.eu/ilcd.html
Friedlingstein, P., Jones, M. W., Sullivan, M. O., Andrew, R. M., Andrew, R. M., Bakker, D. C. E., et al. (2022). Global carbon budget 2021. Earth System Science Data. 14, 1917–2005, doi: 10.5194/essd-14-1917-2022
Guinée, J. B. (2002). Handbook on Life Cycle Assessment. Operational Guide to the ISO Standards. Kluwer Academic Publishers. doi: 10.1007/BF02978897
Hwang, J. J., and Chang, W. R. (2010). Life-cycle analysis of greenhouse gas emission and energy efficiency of hydrogen fuel cell scooters. Int. J. Hydrog. 35, 11947–11956. doi: 10.1016/j.ijhydene.2010.07.148
IEA. (2017). Energy Technology Perspectives 2017. Paris: Catalysing Energy Technology Transformations. Available online at: https://www.iea.org/reports/energy-technology-perspectives-2017 (accessed March 31, 2022)
IEA. (2019a). Global Energy and CO2 Status Report 2019. Paris. Available online at: https://www.iea.org/reports/global-energy-co2-status-report-2019 (accessed March 14, 2022)
IEA. (2019b). The Future of Hydrogen. Paris. Available online at: https://www.iea.org/reports/the-future-of-hydrogen (accessed March 10, 2022)
IEA. (2021a). Global Energy Review 2021. Paris. Available online at: https://www.iea.org/reports/global-energy-review-2021 (accessed June 9, 2022)
IEA. (2021b). Global Hydrogen Review 2021. Paris. Available online at: https://www.iea.org/reports/global-hydrogen-review-2021 (accessed June 9, 2022)
IEA. (2021c). Net Zero by 2050, A Roadmap for the Global Energy Sector. Paris. Available online at: https://www.iea.org/reports/net-zero-by-2050 (accessed June 9, 2022)
ISO. (2006). ISO 14044 Environmental management - Life cycle assessment - Requirements and guidelines. The International organization for standardization. (2006).
Khan, F. I., Hawboldt, K., and Iqbal, M. T. (2005). Life cycle analysis of wind-fuel cell integrated system. Renew. Energy. 30, 157–177. doi: 10.1016/j.renene.2004.05.009
Koj, J. C., Wulf, C., and Zapp, P. (2019). Environmental impacts of power-to-X systems - A review of technological and methodological choices in Life Cycle Assessments. Renew. Sust. Energ. Rev. 112, 865–879. doi: 10.1016/j.rser.2019.06.029
Lozanovski, A., Schuller, O., and Faltenbacher, M. (2011). Guidance Document for Performing LCA on Hydrogen Production Systems. Guidance Document for Performing LCAs on Fuel Cells and H2 Technologies. 139. Available online at: https://www.hytechcycling.eu/wp-content/uploads/HY-Guidance-Document.pdf (accessed March, 26 2022)
Lunghi, P., and Bove, R. (2003). Life cycle assessment of a molten carbonate fuel cell stack. Fuel Cells. 3, 224–230. doi: 10.1002/fuce.200330124
Lunghi, P., Bove, R., and Desideri, U. (2004). Life-cycle-assessment of fuel-cells-based landfill-gas energy conversion technologies. J. Power Sources. 131, 120–126. doi: 10.1016/j.jpowsour.2004.01.006
Masoni, P., and Zamagni, A. (2011). Guidance Document for performing LCAs on Fuel Cells and Hydrogen Technologies (Hyguide). Available online at: https://www.hytechcycling.eu/wp-content/uploads/FC-Guidance-Document.pdf
Mazloomi, K., and Gomes, C. (2012). Hydrogen as an energy carrier, Prospects and challenges. Renew. Sust. Energ. Rev. 16, 3024–3033. doi: 10.1016/j.rser.2012.02.028
Mekhilef, S., Saidur, R., and Safari, A. (2012). Comparative study of different fuel cell technologies. Renew. Sust. Energ. Rev. 16, 981–989. doi: 10.1016/j.rser.2011.09.020
Melamu, R., and von Blottnitz, H. (2009). A comparison of environmental benefits of transport and electricity applications of carbohydrate derived ethanol and hydrogen. Int. J. Hydrog. 34, 1126–1134. doi: 10.1016/j.ijhydene.2008.11.020
Mori, M., Gutiérrez, M., and Casero, P. (2021). Micro-grid design and life-cycle assessment of a mountain hut's stand-alone energy system with hydrogen used for seasonal storage. Int. J. Hydrog. 46. doi: 10.1016/j.ijhydene.2020.11.155
Mori, M., Jensterle, M., MrŽljak, T., and Drobnič, B. (2014). Life-cycle assessment of a hydrogen-based uninterruptible power supply system using renewable energy. Int. J. Life Cycle Assess. 19, 1810–1822. doi: 10.1007/s11367-014-0790-6
Muench, S., and Guenther, E. (2013). A systematic review of bioenergy life cycle assessments. Appl. Energy. 112, 257–273. doi: 10.1016/j.apenergy.2013.06.001
Oliveira, L., Messagie, M., Mertens, J., Laget, H., Coosemans, T., and Van Mierlo, J. (2015). Environmental performance of electricity storage systems for grid applications, a life cycle approach. Energy Convers. Manag. 101, 326–335. doi: 10.1016/j.enconman.2015.05.063
Ozawa, A., Kudoh, Y., Kitagawa, N., and Muramatsu, R. (2019). Life cycle CO2 emissions from power generation using hydrogen energy carriers. Int. J. Hydrog. 44, 11219–11232. doi: 10.1016/j.ijhydene.2019.02.230
Parra, D., Valverde, L., Pino, F. J., and Patel, M. K. (2019). A review on the role, cost and value of hydrogen energy systems for deep decarbonisation. Renew. Sust. Energ. Rev. 101(October 2018), 279–294. doi: 10.1016/j.rser.2018.11.010
Peppas, A., Kollias, K., Politis, A., Karalis, L., Taxiarchou, M., and Paspaliaris, I. (2021). Performance evaluation and life cycle analysis of RES-hydrogen hybrid energy system for office building. Int. J. Hydrog. 46, 6286–6298. doi: 10.1016/j.ijhydene.2020.11.173
Reuß, M., Grube, T., Robinius, M., Preuster, P., Wasserscheid, P., and Stolten, D. (2017). Seasonal storage and alternative carriers, a flexible hydrogen supply chain model. Appl. Energy. 200, 290–302. doi: 10.1016/j.apenergy.2017.05.050
Rinawati, D. I., Keeley, A. R., Takeda, S., and Managi, S. (2022). A systematic review of life cycle assessment of hydrogen for road transport use. Prog. Energy. 4, 012001. doi: 10.1088/2516-1083/ac34e9
Rossi, F., Parisi, M. L., Maranghi, S., Basosi, R., and Sinicropi, A. (2020). Environmental analysis of a nano-grid, a life cycle assessment. Sci. Total Environ. 700, 134814. doi: 10.1016/j.scitotenv.2019.134814
Sevencan, S., and Çiftcioglu, G. A. (2013). Life cycle assessment of power generation alternatives for a stand-alone mobile house. Int. J. Hydrog. 38, 14369–14379. doi: 10.1016/j.ijhydene.2013.09.029
SH2E. (2022). From FCH - LCA guidelines, through FCH-LCC and SLCA guidelines, to robust FCH-LCSA guidelines and tools. Available online at: https://sh2e.eu/
Shimizu, T., Hasegawa, K., Ihara, M., and Kikuchi, Y. (2020). A region-specific environmental analysis of technology implementation of hydrogen energy in Japan based on life cycle assessment. J. Industrial Ecol. 24, 217–233. doi: 10.1111/jiec.12973
Spitzley, D. V., Keoleian, G. A., and Baron, S. G. (2007). Life cycle energy and environmental analysis of a microgrid power pavilion. Int. J. Energy Res. 31:1–13. doi: 10.1002/er.1223
Strazza, C., Del Borghi, A., Costamagna, P., Traverso, A., and Santin, M. (2010). Comparative LCA of methanol-fuelled SOFCs as auxiliary power systems on-board ships. Appl. Energy. 87, 1670–1678. doi: 10.1016/j.apenergy.2009.10.012
Stropnik, R., Sekavčnik, M., Ferriz, A. M., and Mori, M. (2018). Reducing environmental impacts of the UPS system based on PEM fuel cell with circular economy. Energy. 165, 824–835. doi: 10.1016/j.energy.2018.09.201
Suwanmanee, U., Saebea, D., Hacker, V., Assabumrungrat, S., Arpornwichanop, A., and Authayanun, S. (2018). Conceptual design and life cycle assessment of decentralized power generation by HT-PEMFC system with sorption enhanced water gas shift loop. Energy Convers. Manag. 171, 20–30. doi: 10.1016/j.enconman.2018.05.068
Tashie-Lewis, B. C., and Nnabuife, S. G. (2021). Hydrogen production, distribution, storage and power conversion in a hydrogen economy - a technology review. Adv. Chem. Eng. 8, 100172. doi: 10.1016/j.ceja.2021.100172
Tranfield, D., Denyer, D., and Smart, P. (2003). Towards a methodology for developing evidence-informed management knowledge by means of systematic review. Br. J. Management. 14, 207–222. doi: 10.1111/1467-8551.00375
United Nations Environment P.rogramme. (2020). Guidelines for Social Life Cycle Assessment of Products 2020. Available online at: https://www.unep.fr/shared/publications/pdf/DTIx1164xPA-guidelines_sLCA.pdf
Valente, A., Iribarren, D., and Dufour, J. (2016). Life cycle assessment of hydrogen energy systems, a review of methodological choices. Int. J. Life Cycle Assess. 22, 346–363. doi: 10.1007/s11367-016-1156-z
Valente, A., Iribarren, D., Dufour, J., and Spazzafumo, G. (2015). Life-cycle performance of hydrogen as an energy management solution in hydropower plants, a case study in Central Italy. Int. J. Hydrog. 40, 16660–16672. doi: 10.1016/j.ijhydene.2015.09.104
Varun Bhat, I. K., and Prakash, R. (2009). LCA of renewable energy for electricity generation systems-a review. Renew. Sust. Energ. Rev. 13, 1067–1073. doi: 10.1016/j.rser.2008.08.004
Walker, S. B., Lanen, D., Van, M., ukherjee, U., and Fowler, M. (2017). Greenhouse gas emissions reductions from applications of Power-to-Gas in power generation. Sustain. Energy Technol. Assess. 20, 25–32. doi: 10.1016/j.seta.2017.02.003
Work Energy Council. (2022). World Energy Insights, Working Paper Regional Insights into Low Carbon Hydrogen. London. Available online at: https://www.worldenergy.org/publications/entry/regional-insights-low-carbon-hydrogen-scale-up-world-energy-council
Yue, M., Lambert, H., Pahon, E., Roche, R., Jemei, S., and Hissel, D. (2021). Hydrogen energy systems, A critical review of technologies, applications, trends and challenges. Renew. Sust. Energ. Rev. 146, 111180. doi: 10.1016/j.rser.2021.111180
Zhang, J., Meerman, H., Benders, R., and Faaij, A. (2021). Techno-economic and life cycle greenhouse gas emissions assessment of liquefied natural gas supply chain in China. Energy. 224. doi: 10.1016/j.energy.2021.120049
Keywords: life-cycle assessment, hydrogen, power generation, environmental impact, electricity
Citation: Rinawati DI, Keeley AR, Takeda S and Managi S (2022) Life-cycle assessment of hydrogen utilization in power generation: A systematic review of technological and methodological choices. Front. Sustain. 3:920876. doi: 10.3389/frsus.2022.920876
Received: 15 April 2022; Accepted: 30 June 2022;
Published: 28 July 2022.
Edited by:
Roberta Salomone, University of Messina, ItalyReviewed by:
Teresa Maria Gulotta, University of Messina, ItalyChristina Wulf, Helmholtz Association of German Research Centres (HZ), Germany
Copyright © 2022 Rinawati, Keeley, Takeda and Managi. This is an open-access article distributed under the terms of the Creative Commons Attribution License (CC BY). The use, distribution or reproduction in other forums is permitted, provided the original author(s) and the copyright owner(s) are credited and that the original publication in this journal is cited, in accordance with accepted academic practice. No use, distribution or reproduction is permitted which does not comply with these terms.
*Correspondence: Dyah Ika Rinawati, ZHlhaC5yaW5hd2F0aS4yNzJAcy5reXVzaHUtdS5hYy5qcA==