- 1Sustainability and Design Lab, Centre for Product Design and Manufacturing, Indian Institute of Science, Bangalore, India
- 2Sustainability and Design Lab, Centre for Sustainable Technologies and Centre for Product Design and Manufacturing, Indian Institute of Science, Bangalore, India
Manufacturing organizations continuously improve their energy, environmental, and economic performance at different manufacturing levels (products, processes, enterprise, etc.) using various assessment methodologies for visibility and a competitive market edge. Sustainability assessment has become the focus of the manufacturing performance measurement in the last decade and has triggered numerous methodological developments and adoption in practice. The assessment focus has broadened from process to enterprise-level, single to multiple parameters, fragmented to a holistic point of view, and local businesses to global sustainability and circularity. Increasing global environmental burden, resource scarcity, and human health challenges urge a shift toward effective assessment practices. This article critically reviews sustainability assessment practices in manufacturing from a methodological efficiency-effectiveness perspective. A clear distinction between efficiency and effectiveness practices has been discussed. The requirements and research challenges for effectiveness in the sustainability assessment practice in theory (academia) and practice (industry) is presented.
Introduction
Sustainability is a crucial global concern underlying all aspects of human activities today, broadly dealing with the system's ability in its entity (earth) to support and sustain a healthy life (Mani et al., 2005). Tracing modern civilization's history alongside environmental vitality, industrialization bolstered consumerism (economic growth) have seeded the gruesome manifestations of unsustainability we are witnessing today, e.g., ocean plastics, biodiversity loss, soil infertility, greenhouse gas (GHG) emissions, forever chemicals, and pandemics. While native cultures always emphasized caution for centuries against rapid modernization (Mani et al., 2005), modern researchers (US Government Printing Office, 1980; Carson et al., 2002) had also forewarned of imminent unsustainable consequences based on trends prevalent nearly half a century back. Among the foremost to postulate a sustainable development model was the Gandhian Economist J.C. Kumarappa way back in the 1940s. He discussed an approach to development that does no irreparable damage to the environment (Kumarappa, 1946). Despite early caution on unsustainable consequences, the world finally heeded to the need for sustainable development at the World Commission on Environment and Development in 1984, accepting Brundtland's definition of sustainable development. Brundtland's report “Our Common Future” finds one of the earliest associations of the term “sustainability” with “industry” and thus manufacturing (Brundtland, 1987). The sustainability perspective was originally efficiency-based which led to economic benefits to industries. Later, the perspective changed to product performance-based, which dealt with operational environmental concerns. The increase in population, consumerism, and enhancing lifestyles has resulted in unprecedented resource consumption, driving manufacturing-dominated growth globally. The transdisciplinary domain of sustainability science can be characterized by dynamic interactions between society (including economy) and nature and fundamentally requires a systems perspective (Mani et al., 2005; Sala et al., 2013a; Kumar and Mani, 2019). Sustainability and manufacturing are diverse yet intricately connected areas of research.
Manufacturing is a critical sub-component of a larger product life cycle involving design, material extraction, production, assembly, transport, use (service/maintenance), and post-use phases (recycling and disposal). It is economically defined as the process of transforming materials into usable products using one or more manufacturing processes, finishing, and assembly operations. Manufacturing can be viewed as operating at different levels from a production layout point of view viz. unit manufacturing process/single machine, work cell/manufacturing line/multi-machine, facility/factory, and multi factory/production network (Radnor and Barnes, 2007; Cao and Folan, 2012). The term “manufacturing” is generally used interchangeably but is conceptually different from “production,” which is limited to production planning, material processing, and quality assurance. Manufacturing has a broader scope covering all functions or activities starting from product ideation and its disposal along the value chain (Herausgeber, 2004). It consists of humans, production machines and systems, control and automation systems, storage and material handling systems, supply chain networks, and global business operations with various technical and planning characteristics (Esmaeilian et al., 2016), networked over diverse geographies (Chandran et al., 2016). Figure 1 presents an overview of different levels of manufacturing, associated entities, decision-makers, and factors affecting its operation. Manufacturing generally involves a globally distributed network that utilizes local and global (material and energy) resources to produce products used and disposed of in different geographical locations (Duflou et al., 2012; Chakrabati, 2015). It is one of the most resource-intensive (e.g., material, energy, and water) sectors with economies of scale, lower labor costs, and higher employment potential. Organizations understand that sustainability is critical to modern manufacturing, as it provides a market competitive edge and visibility. It has led to the evolution of various paradigms such as lean manufacturing, cleaner production, sustainable manufacturing (SM), industrial sustainability, sustainable production, industrial ecology, and circular economy.
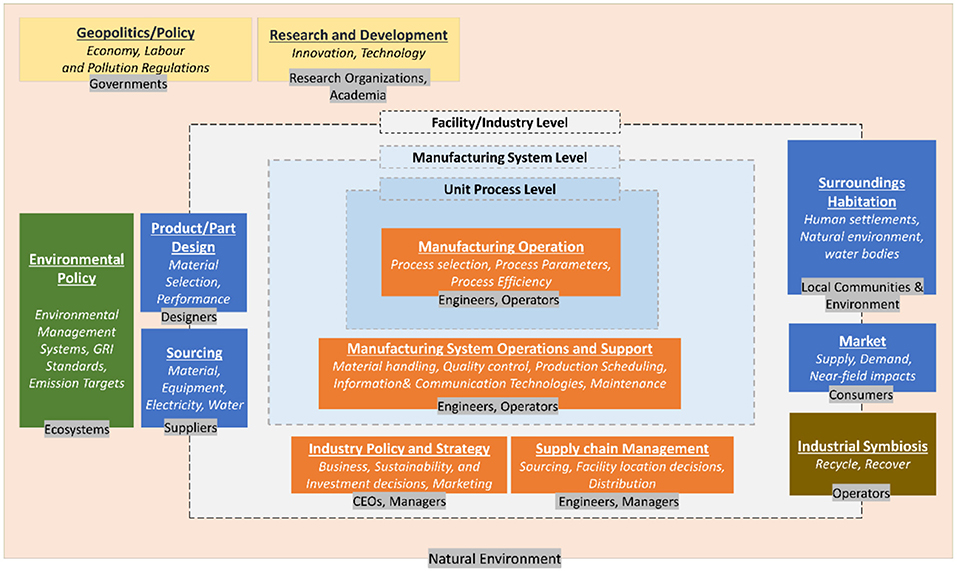
Figure 1. Levels of manufacturing, decision-makers (gray shaded) involved and their roles, and surrounding entities affecting manufacturing operations (derived from Kumar and Mani, 2021b).
Developing economies perceive manufacturing as a source of growth as it boosts employment opportunities and economic growth, although it comes with an environmental cost. It causes various regional and global impacts (embodied and active) on the natural environment and human health by emitting various pollutants to air, water, and soil. Globally, the industry sector was the second largest contributor (24%; 14 GtCO2eq) to the direct GHG emissions in 2018, primarily driven by the manufacturing of pulp and paper, food and tobacco, glass and ceramics, refrigerants, solvents etc. (Lamb et al., 2021). Manufacturing is the third-largest contributor to the CO2 emissions in the United States after transportation and electricity production (US EPA, 2021). Recent trends (from 2001 to 2010) show a strong correlation between the world's energy consumption, emissions, material requirements, and GDP (Gutowski et al., 2013). A historic shift of manufacturing location toward developing nations like China and India can be observed in the late 20th century (Gutowski et al., 2013), primarily due to cheap labor, conducive (not so stringent) regulations, and vast sales market. In 2018, the Chinese manufacturing sector contributed 28.4% of the global manufacturing output (Ritcher, 2020). In 2015, manufacturing (excluding agriculture, mining, and construction) accounted for 23% of total energy consumption for the International Energy Agency member countries, which is the second highest among all the sectors, followed by transportation (36%) (International Energy Agency, 2017). Indian manufacturing sectors accounted for 44% of total electricity consumption in 2015 (NITI Aayog, 2017). The manufacturing output shows a high correlation with the CO2 emissions (Gutowski et al., 2013), e.g., increased air and water pollution in China (Vennemo et al., 2009). A study on soil contamination in Europe found that industrial and commercial activities are the second highest contributor (~33.3%) to soil contamination; metal industries are reported to be the most frequent source (Panagos et al., 2013). Sites surrounding Chinese steel industries have been identified as heavy metals contamination hotspots compared to non-industrialized sites (Qing et al., 2015). Industrial activities cause environmental burdens and adverse effects on the health of local communities (WHO, 2009; Mudu et al., 2014). The detrimental impacts of environmental pollution on human health are well documented (Revel et al., 2018; Gupta et al., 2019; Nobile et al., 2020). Global environmental assessment reports have reiterated the requirement for an urgent focus on planetary wellness (UN Environment, 2019; Singh et al., 2021).
Besides, various kinds of impacts on social groups (consumers, workers, and society) have been recorded in history (Sutherland et al., 2016), e.g., cases of work stress leading to several suicides among Chinese workers (Ngai and Chan, 2012). Apart from negative impacts directly associated with the manufacturing activities and the supply chain, numerous indirect impacts are associated with the product life cycle stages on the endless spatial and temporal scales traceable to design and manufacturing decisions. BPA used in the production of various consumer products (Vandenberg et al., 2007) is found in plastic consumer products (use and post-use phase), e.g., in the food storage cans, baby bottles, individuals in developed nations, aquatic animals, and the environment. Further, chronic exposure risks of aluminum leading to Alzheimer's disease are well-documented (Wang et al., 2016). BPA used as a color developer in various paper products has significant exposure to the human population (Liao and Kannan, 2011; Porras et al., 2014). Estimates of 5.25 trillion plastic particles weighing 268,940 tons floating in the sea have been reported (Eriksen et al., 2014). More shreds of evidence of microplastics in the human placenta (Ragusa et al., 2021) and endocrine-disrupting chemicals in a wide variety of products (Wong and Durrani, 2017) are alarming. Such impacts are not directly addressed in the conventional efficiency-based corporate sustainability reporting and life cycle assessment (LCA)-based methods. The sustainability of the manufacturing enterprises is majorly addressed with the help of sustainability standards, such as environmental management and LCA standards (ISO 14001, 14040, and 14044), ISO 50001 energy management standard, global reporting standards (GRI), and sustainability accounting standards board (SASB) standards.
Existing sustainability assessment (SA) literature predominantly focuses on efficiency improvements without considering absolute (local and global) pollution limits and rebound effects (Hauschild, 2015; Gutowski, 2018; Fantke and Illner, 2019). An analysis of 83 case studies revealed the gaps in the implementation of sustainability measures in manufacturing (Despeisse et al., 2012). Environmental impact and economic benefits are more frequently reported than social aspects. SA methods operating at the factory level lack a generic and holistic approach (Chen et al., 2013). Various SA methods for discrete manufacturing process (gate-to-gate) level observed a shift toward life cycle sustainability analysis (LCSA) (Gbededo et al., 2018). Most studies focus on environmental dimensions, whereas social dimensions are overlooked in the SA of the automotive sector (Pallaro et al., 2015). The need for integrating the three sustainability dimensions (planet, people, profit) for a holistic assessment has been consistently reiterated in literature.
Impacts occurring in a product life cycle can be categorized as embodied and active (Kumar and Mani, 2019) (Table 1). Impacts occurring in the production of a product can be defined as embodied impacts and primarily addressed using an efficiency perspective, e.g., energy and resource efficiency. In contrast, active impacts are ongoing impacts that are likely to occur during a product's use and post-use phases, e.g., human toxicity impacts due to product use and toxic materials emissions to the environment due to product use (Kumar and Mani, 2019). Embodied impacts are reasonably straightforward to identify and deal with. They mainly involve efficiency improvements and are primarily associated with design and manufacturing phases. System boundaries for embodied impacts are well-known and easy to define and regulate. Assessing embodied impacts generally leads to economic benefits to the company. Efficiency-based approaches counterintuitively lead to rebound effects, resulting in a higher resource and energy net consumption. Effectiveness-based active impacts involve inherent uncertainty and are difficult to estimate.
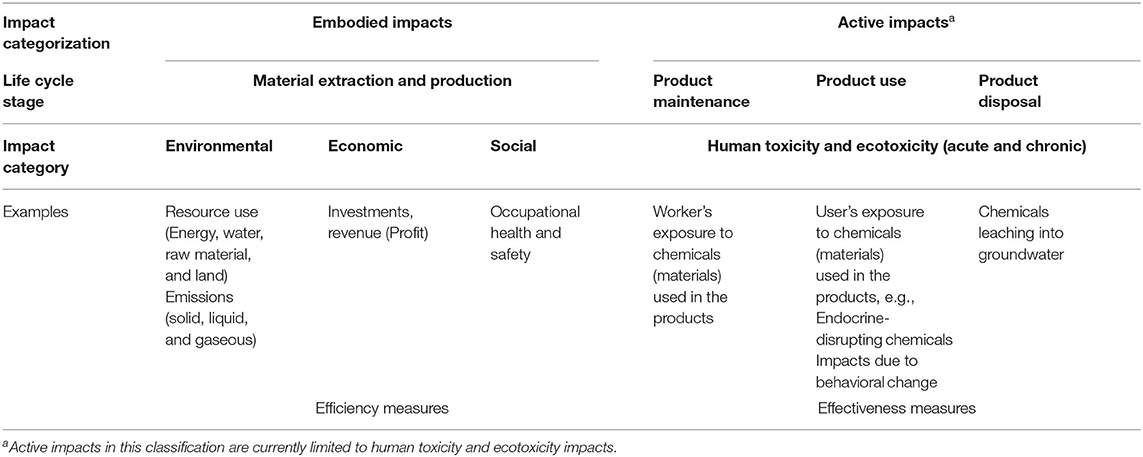
Table 1. Classification of product life cycle impacts, abridged from Kumar and Mani (2019).
On the other hand, active impacts are not straightforward and can be notorious to identify and deal with. Use-phase exposure to organic and inorganic substances and material disintegration is omitted in the existing SA practices in methodological considerations, impact assessment, and systemic consequences. Assessing (effectiveness-based) use phase impacts is considered challenging in contrast to those related to (efficiency-based) production and disposal phases, as product characteristics, user's behavior, and circumstances of use both vary (Schmidt I. et al., 2004). A manufactured product's performance also depends on the use phase in the living environment, e.g., a fuel-efficient car is just as polluting as any other car when driven in a heavy-traffic scenario. This distinction is crucial for the SM community to gain clarity on effective measures that could aid the identification of appropriate indicators and product life cycles that genuinely contribute to sustainability.
The need for the transition from efficiency to effectiveness in industrial sustainability has recently been reviewed in the context of product design, manufacturing process planning, and CE (Haapala et al., 2013). A comprehensive evaluation of SA practices in manufacturing from an efficiency and effectiveness perspective has not been conducted so far. In this direction, we have discussed the distinction between efficiency and effectiveness assessment methods and presented the way forward to develop effectiveness-based approaches. This article presents challenges and opportunities for effectiveness-based SA in manufacturing.
Efficiency vs. Effectiveness
Efficiency and effectiveness have been debated in different contexts in the literature of sustainability and manufacturing. Efficiency focuses on minimizing resources to achieve the desired output level, while effectiveness emphasizes ascertaining and making the right choices for utilizing resources (Duflou et al., 2012). International policy and industry sustainability initiatives focus on energy efficiency, GHG emissions reduction, carbon neutrality, etc. Corporate sustainability reports commonly practice relative/efficiency improvements with a minimal mention (~5% of all corporate reporting) of planetary boundaries and ecological limits (Bjørn et al., 2017a). Efficiency may not assure or yield effective results; effective results may not involve an efficient approach as a necessary condition. A good example could be derived from military operations, where effectively achieving results is the only performance driver and not how efficiently an operation is performed. The same principle operates in the design/manufacturing of vehicles for operation in adverse conditions, where reliability or fail-safe operation is the fundamental consideration, and not so much fuel efficiency.
In the context of manufacturing industries, efficiency-based performance measures address customer satisfaction by the firm/manufacturer (internally), keeping in mind the firm's economic viability; In contrast, effectiveness measures focus on fulfilling customer requirements (Neely et al., 1995). Energy effectiveness is a far more complex and broader concept as compared to energy efficiency and involves value judgment and ethical considerations about the best utilization of resources (Shade and Sutherland, 2018).
Effective sustainability principles include an adequately defined scope of the assessment, transparent and effective communications, and stakeholder participation (Mani et al., 2005; Pintér et al., 2012). Eco-effectiveness implies proactively looking at positive contributions through absolute improvements (“doing good than just doing less bad”) for positive recoupling between economy and ecology (Hauschild, 2015; Herrmann et al., 2015). Figure 2 (adapted from Kumar and Mani, 2021a) illustrates the distinction between efficiency and effectiveness in SA. The ability to foresee likely impacts of human activities is crucial to guide assessment methods toward sustainability (Gibson, 2006), requiring an effectiveness-based systems approach. An effective sustainable design approach simultaneously addresses market success (economy), environmental and social challenges, and undesirable consequences (such as rebound effects) that can overshadow economic, environmental, and social benefits (Hauschild, 2015). The importance of transdisciplinary studies to cope with complex sustainability challenges has been reiterated in literature (Bond and Morrison-Saunders, 2013). Stakeholder engagement and communication throughout the assessment practice are vital for ensuring SA effectiveness (Gutowski et al., 2013; Chandran et al., 2016). The effectiveness in SA requires improved assessment methods and enhanced implementation and decision-making for sustainability.
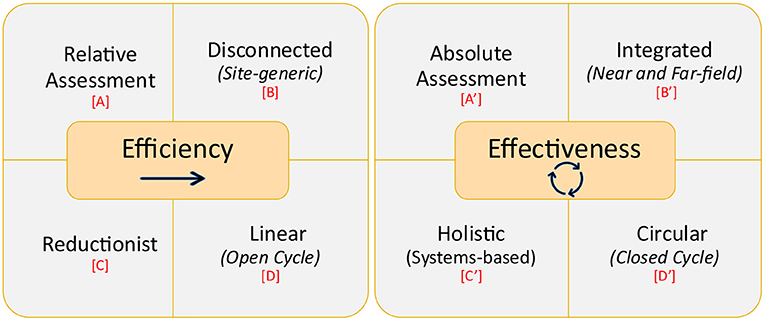
Figure 2. Contextualizing efficiency and effectiveness for sustainability assessment, adapted from (Kumar and Mani, 2021a).
Various aspects (see Figure 2) of effectiveness assessment are discussed briefly:
• Relative vs. absolute assessment: SA has been promoted as efficiency improvement for a long time, leading to various concepts focused on efficiencies such as energy efficiency, material efficiency, and resource efficiency. Even though these concepts are well-studied in the literature and implemented on a broader scale, they are criticized from time to time for their ineffectiveness. Lack of integrated assessment, including human behavior (Gutowski, 2018), rebound effects (Gillingham et al., 2013; Azevedo, 2014; Wei and Liu, 2017) and feedback systems, efficiency improvements have yielded counterintuitive results. Absolute assessments integrate the concept of planetary boundaries (Rockström et al., 2009) in the SA methods. Multiple studies have recently explored the application of the planetary boundaries framework at different levels of manufacturing (Bjørn et al., 2020a).
• Disconnected (Site-generic) vs. Integrated (Near-field and Far-field) Assessment: Widely followed impacts assessment methods adopt a site-generic approach to report impacts for a general population, primarily for the ease of communication and calculations. For effective assessment, impacts should be characterized and assessed as per their geographical relevance. Barriers to following such a process include lack of data and appropriate assessment techniques, which are still nebulous and evolving. Given the fact that humans spend 80–90% of their time indoors (Morrison, 2008; González-Martín et al., 2021), near-field impacts are crucial to understand and integrate with SA. Near-field impacts are quite relevant for the product use phase, which are earlier also defined as active impacts (Kumar and Mani, 2019). Various assessment methods have been developed for assessing near-field impacts. A widespread focus has been on the near-field impacts due to chemical exposure (Huang et al., 2017). A few products/product categories have been studied, e.g., buildings (Huang et al., 2019, 2021), children's toys (Aurisano et al., 2021), cosmetics (Ernstoff et al., 2016), etc. Recent studies on chemical exposure, indoor pollution, and human toxicity suggest an urgent need to focus on active impacts in near-field environment, for example, health concerns due to prolonged exposure to LED light (Nash et al., 2019), indoor pollution (Ham, 2019), and underestimated effects of BPA on human health (Gerona et al., 2019), etc.
• Reductionist vs. holistic assessment: A reductionist SA approach focuses on individual aspects of sustainability for simplified assessment and user-friendliness and can be classified into three categories: monetary, biophysical, and sustainability indicators/indices (Gasparatos et al., 2008). In contrast, a holistic sustainability approach considers the complex interdependencies and interconnectedness between the sustainability dimensions, acknowledging the uncertainties and undesirable consequences and associated stakeholders of an activity (Sala et al., 2013b, 2015). System-based approaches capture the interactions between the sustainability dimensions and trace cause-effect chains from an activity to an impact. Such an approach is crucial to understand the direct and indirect impacts associated with an activity in a product life cycle. Systems-based approaches prevent sub-optimization by following a holistic approach considering the trade-offs within the connected systems. A holistic approach to integrating (traditional) efficiency-based and eco-effectiveness approaches is needed for sustainable industrial development (Gaziulusoy and Boyle, 2013).
• Linear vs. circular life cycle: Circularity is increasingly seen as a precursor to sustainability (Geissdoerfer et al., 2017) and provides the horizontal facilitator traversing the three pillars (verticals) of sustainability. Resource scarcity has been a crucial concern for sustainability research, widely practiced with resource efficiency measures. The linear take-make-dispose model has failed to sustain in the developing society with endless resources demands (biotic and abiotic). Despite the peak of efficiency improvements and recycling rates, the insatiable demand for resources has led to resource scarcity (Allwood et al., 2011; Gutowski et al., 2013; Graedel et al., 2015). Global circularity metrics have reported that the world is only 8–9% circular (Circle Economy, 2022). Circular Economy is an alternate economic concept that emphasizes on restorative and regenerative use of resources by designing out waste, keeping materials and products in use, and transitioning to renewable energy sources (The Ellen MacArthur Foundation, 2017). Product design and life cycle management have a crucial role to play in circularity scenarios. Conserving resources is viewed as a potential solution for SM (Rashid et al., 2013) and is also imperative for circularity. The criticality of materials has also received the attention of sustainability research (Schrijvers et al., 2020). Integrating material criticality assessment with LCA is crucial to ensuring material supply (Sonnemann et al., 2015).
Sustainability Assessment: Overview of Existing Approaches
SA has its roots in the environment and pollution domain. It has evolved as the extended version of existing environmental assessment methods such as environmental impact assessment (EIA) and strategic environmental assessment (SEA) by integrating social and economic dimensions to address the triple bottom line (TBL) concept (Pope et al., 2004). SA comprises two dimensions: the concept of sustainability (definition) and the decision-making context (purpose of assessment) (Pope et al., 2017). Several SA frameworks, methodologies, tools, and indicators have been reviewed (Ness et al., 2007; Singh et al., 2012). Table 2 presents various SA methodologies developed for product/processes in chronological order.
SA is a predictive decision-making tool for sustainability (Pope et al., 2017) that should follow a systems approach and involve stakeholders throughout the assessment process (NRC, 2013). SA are comprehensive context-dependent assessments to identify emergent properties and involve multiple field-specific methods depending on the application (Sala et al., 2015). The terminology in SA literature is characterized as the framework (e.g., LCSA), methodologies (e.g., LCA, S-LCA, LCC), methods or tools (e.g., LCIA methods such as Eco-Indicator99, CML, and ReCiPe; software (GaBi, SimaPro, and OpenLCA); and indicators (DALY, acidification potential, and ozone depletion potential, etc.) (Sala et al., 2013b). SA methods are categorized into monetary, biophysical, and indicator-based methods following anthropocentric, eco-centric, and mixed valuation perspectives (Gasparatos and Scolobig, 2012). Another broad categorization of SA methods is product-based (which follows a life cycle perspective), indicators-based, and integrated assessment methods (focused on policy implementation) (Ness et al., 2007). Integrated assessment methods are prospective on a temporal scale, whereas indicator-based methods are retrospective (Ness et al., 2007). Most assessment methods are reductionist except indicator-based multi-criteria methods (Gasparatos and Scolobig, 2012). Forecasting methods are more favorable for SA to help decision-makers identify impacts, benefits, risks, and vulnerabilities of action(s) of integrated natural and societal systems in short and long-time scenarios at local and global scales (Ness et al., 2007). Recent studies have integrated future studies into SA to assess long-term sustainability perspectives. Scenario-oriented SA methods have been classified into five categories: procedural tools, analytical tools, tools for aggregating impacts, combined scenario assessment methods, and strategic environmental assessment (Fauré et al., 2017).
Further, various methods for conducting SA (broadly categorized as life cycle and indicator-based approaches) and recent developments to integrate and address effectiveness in the sustainability practice have been discussed.
Life Cycle Approaches (LCA, S-LCA, LCC, and LCSA)
LCA, also known as Environmental-LCA (E-LCA), has been extensively used as a decision support tool by many industries to make strategic and planning decisions related to sustainability. LCA has evolved from a mere focus on energy and resource efficiency to linking material flows to the Areas of Protection (AoP) currently limited to human health, ecosystem quality, and natural resources. The UNEP life cycle initiative has recently proposed new AoPs: Socio-Economic Assets, Cultural Heritage, and Natural Heritage (Verones et al., 2017), and are yet to be further developed for implementation. Further, E-LCA has emerged into new methodologies such as Consequential-LCA (C-LCA) (Earles and Halog, 2011), Dynamic LCA (Levasseur et al., 2010), Working Environment LCA (WE-LCA) (Poulsen and Jensen, 2004) (Schmidt A. et al., 2004), Economic Input-Output LCA, Social and Socio-economic LCA (Benoît et al., 2009) and Prospective LCA (Arvidsson et al., 2017). WE-LCA is restricted to impacts within the working environment applicable at different manufacturing levels (Poulsen and Jensen, 2004). The LCA framework can be further expanded by including the Ecosystem Services (ES) impact category to consider the ecosystems' capacity to impacts caused by human activities (Dreyer et al., 2006). Also, few advancements can be seen in human toxicity and exposure impact categories (Jolliet et al., 2015) with the development of LCIA methods such as USEtox. Intake fraction (iF), widely followed for outdoor (far-field) exposure assessment, has been modified to product intake fraction (PiF) for near-field/indoor emissions to study consumer exposures in the product use phase (Jolliet et al., 2015). An increasing interest in integrating planetary boundaries to develop absolute SA methods can be observed in the literature (Bjørn et al., 2016; Chandrakumar and McLaren, 2018). Issues related to data aggregation make LCA an unreliable method for accurate assessments. Efforts have been made by CO2PE!-initiative (Cooperative Effort on Process Emissions in Manufacturing) for providing reliable LCI data by generating unit process-specific LCI datasets (Kellens et al., 2012). Multinational companies could engage various actors along the value chain to enable an integrated LCI data collection process (Miah et al., 2018). Simulation models, data-driven approaches, and blockchain integrated supply chains are a way forward to fill the data gaps pertaining to LCA studies of manufacturing processes (Stock and Seliger, 2016; Leng et al., 2020; Bernstein et al., 2021).
Social-LCA (S-LCA) provides complementary information to E-LCA, with a more detailed picture of impacts focusing on the social dimension. It aims to estimate positive and negative social and socio-economic impacts and incorporate a large amount of qualitative data wherever applicable, whereas E-LCA relies only on quantitative data. In S-LCA studies, manufacturing is the most explored area (followed by agriculture, energy, buildings, and photovoltaics) (Schmidt I. et al., 2004). Social impacts (e.g., human dignity, wellbeing, human rights, labor practices, and product responsibility) of products depend on the conduct of the companies (business decisions) involved in the value chain, contrary to environmental impact calculations that rely on material and energy flow processes (Dreyer et al., 2006, 2010). The relationship between social impacts and manufacturing is unclear due to a lack of data tracing social impacts to manufacturing decisions (Sutherland et al., 2016). Few studies have attempted to establish this relationship, e.g., mortality risk from lung cancer and labor rights violations associated with the welding process and Nickel production (Hutchins et al., 2013). A broader application of S-LCA at the organization level using Social Organizational-LCA (SO-LCA) is presented in the latest S-LCA guidelines by UNEP ( UNEP,2020).
Life cycle costing (LCC) originated in the 1950s and is a predecessor to LCA and accounts for all product life cycle costs beyond manufacturing boundaries and is considered relevant for SA (Klöpffer and Ciroth, 2011). It subsequently led to the development of ISO standards (BS 15868-5), vastly applied in the building and construction industry. The total cost of ownership (TCO) is also used in the industry to estimate the purchasing cost of a product/service. Environmental life cycle costing (environmental LCC or eLCC) calculates life cycle environmental aspects related to financial costs (Guinée et al., 2011; Swarr et al., 2011). Life cycle cost analysis (LCCA) determines the economic feasibility based on LCC calculations (Asiedu and Gu, 1998).
A conceptual formula “LCSA = LCA + LCC + S-LCA” has been proposed to move from conventional LCA to SA (Kloepffer, 2008). LCSA is a holistic representation of analytical and descriptive approaches to SA. It aims to expand LCA to a comprehensive method by broadening of impacts (including environmental, economic, and social dimensions under one framework), broadening the level of analysis, and deepening the assessment (considering other relationships such as physical and behavioral relationships) (Guinée et al., 2011; Guinée, 2016). It has been interpreted as the “third wave of LCA” or “decade of consolidation” that focuses on reducing existing practical challenges and integrating sustainability dimensions (Zimek et al., 2019). An LCSA framework to address the interaction between various systems using technical, physical, micro, and macro-economic, cultural, ethical, and societal models have been proposed (Heijungs et al., 2010). Few studies have applied LCSA approaches for SA in manufacturing in practice. A step-by-step guide for applying LCSA at the manufacturing facility (factory) level has been presented (Onat et al., 2016; Hannouf and Assefa, 2017). The study included six areas of protection: economic stability, ecosystem quality, human health, human-made environment, social justice, and resource availability (Hannouf and Assefa, 2017). Most of the current applications of LCSA in practice focus on the environmental science discipline with a meager contribution to social sciences and economics and misses out on the interrelationships within the system, feedback mechanisms, and rebound effect (Onat et al., 2017). The focus of methodologies varies from reactive to proactive nature, utilizing both quantitative and qualitative data. Most ex-post assessments follow multi-criteria decision models, whereas the ex-ante (proactive) assessments utilize mathematical programming for SA (Walker et al., 2021).
Many challenges exist in developing a robust methodology for LCSA as the underlying approaches are under development and have methodological and practical challenges (Sala et al., 2013a). The challenges include requirements of future scenario-oriented life cycle approaches, integrated with the quantitative and practical indicators to address inadvertent uncertainties such as rebound effects (Guinée, 2016). Dynamic interactions between various sustainability indicators, feedback mechanics, and systems surroundings can be captured using a systems dynamic model for a holistic LCSA. Capturing the case-specific interlinkages among sustainability dimensions remains a significant challenge for LCSA studies (Hannouf and Assefa, 2017).
Indicators-Based Sustainability Assessment
Indicators provide the means to measure sustainable development to provide feedback to the decision-makers (Mani et al., 2005). Sustainability indicators must be appropriate, measurable, representative of the concerned phenomenon, reliable, technically feasible, communicable, and aid effective action/decision to support sustainability (Hák et al., 2012). Various organizations and authors have proposed numerous sustainability indicators for the sustainability categories (environmental, economic, social, institutional, and circularity). A common criticism about the sustainability indicators approach is that they are reductionist, i.e., they tend to isolate the problem by oversimplifying it and lack a systems approach (Bell and Morse, 2012). Indicators do not necessarily establish a cause-effect relationship between an activity and the impact but can effectively contribute to sustainable development by involving the quantified analysis and meaningful interpretation (Mair et al., 2018). Organizational sustainability indicators can be classified as lagging (assessing impacts that occurred in the past) and leading indicators (for future performance and impacts) (Tanzil and Beloff, 2006). SeeBalance® is an indicators-based method to estimate the social impacts of products and processes in various phases (material sourcing, production, use, and disposal) by considering indicators associated with employees, suppliers, customers, society, future generations, and international society (Schmidt I. et al., 2004). Case and geography-specific indicators must be developed, considering the decision-making context and stakeholders' interests for effective implementation (Lyytimäki et al., 2013).
Sustainability Assessment in Manufacturing: Overview and Challenges
SM is conventionally defined as the creation of manufactured goods that minimize negative impacts while conserving energy and resources in a most economical way (US Dept of Commerce, 2007). Methodologies developed under a particular paradigm imbibe relevant philosophical assumptions of the paradigm (Sudit, 1995). The definition of SM plays a crucial role in SA. Sustainability in the context of manufacturing has been defined and applied in different ways by various studies and organizations. There is no standard definition to date, though the definition proposed by the U.S. Department of Commerce “Sustainable manufacturing includes things such as making products using less energy and materials, producing less waste, and using fewer hazardous materials as well as products that have greener attributes such as recyclability or lower energy use,” is frequently cited (Moldavska and Welo, 2017) Table 3 lists salient SM definitions and contributions. Definitions proposed by Lowell Centre for Sustainable Production, Alting and Jøgensen (1993), Garetti and Taisch (2012), and Nahkala (2013) link SM to products and services, whereas other definitions focus on the policy aspect of SM (OECD, 2011). Other definitions emphasize social dimensions and the circular economy perspective (Nahkala, 2013; Moldavska and Martinsen, 2018). A detailed review of 89 definitions of SM has been presented, focusing on various factors (e.g., manufacturing levels and associated stakeholders) that influence the sustainability performance (Moldavska and Welo, 2017). The majority of definitions do not consider the interconnectedness among the sustainability dimensions and across product life cycle stages, which is crucial for effectiveness (Kumar and Mani, 2021b).
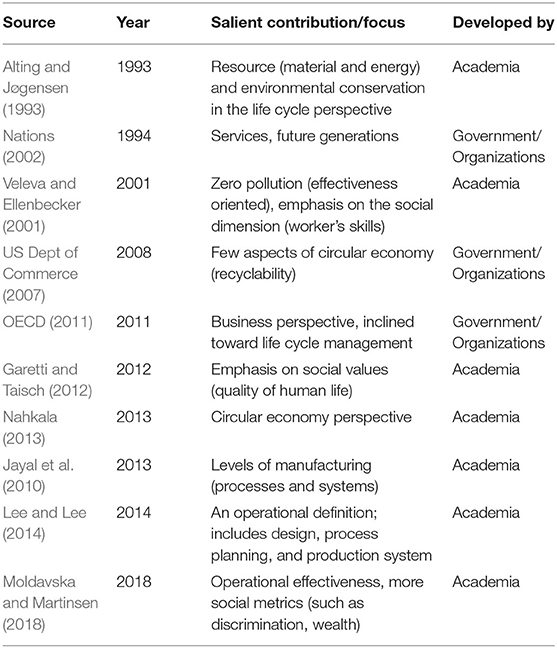
Table 3. Analyzing definitions of Sustainable Manufacturing, extended from Kumar and Mani (2021b).
Theory (Academic) Perspective
SA in manufacturing is an emerging area of concern among the manufacturing research community in both industry and academic research. SA practice in manufacturing can be classified as indicators-based approach (development of sustainability indicators and indices), product-based assessment (e.g., LCA, material flow analysis), and integrated assessment (e.g., system dynamics, multi-criteria decision models etc.) (Ness et al., 2007). Numerous frameworks, methodologies, and tools have been developed for SA in manufacturing. The assessment methods for SA in manufacturing range from process, multi-facility, and global supply chains level. SA in manufacturing can be classified into two assessment categories: sustainable product development (segmented and integrated) and sustainable performance measurement (segmented and integrated) (Gbededo et al., 2018). Current SA practices in manufacturing focus on the environmental dimension throughout the supply chain, and the social dimension has received less attention (Despeisse et al., 2012). Existing practices in manufacturing are not generic, lack consistency, are company-specific, and focus on external reporting (Moldavska and Welo, 2015). Figure 3 shows the mapping of widely used SA methods in manufacturing on operational (product) to application (policy) scale at various manufacturing levels (product to multi-facility and global level). Few methods operate at the product level, whereas the strategic (policy) zone is populated with several methodologies.
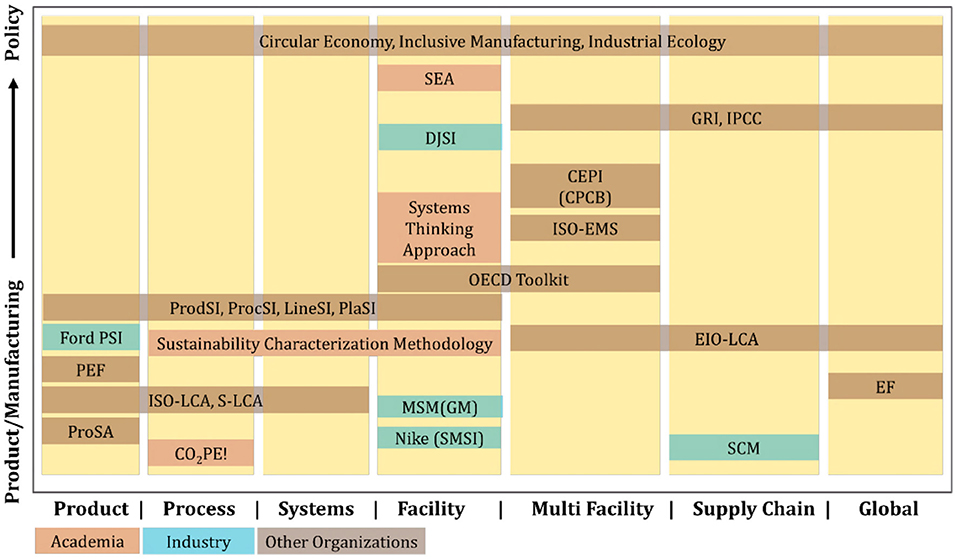
Figure 3. SA measures in manufacturing at various levels, extended from Feng et al. (2010). CEPl (CPCB), Comprehensive Environmental Pollution Index (Central for Pollution Control Board, India); CO2PE!, Cooperative Effort on Process Emissions in Manufacturing; DJSI, Dow Jones Sustainability Index; EF, Ecological Footprint; EIO-LCA, Economic Input Output Life Cycle Assessment; GRI, Global Reporting Index; Ford PSI, Ford Product Sustainability Index; IPCC, International Panel on Climate Change; ISO·LCA, ISO-Life Cycle Assessment MSM (Genera l Motors): Metrics for Sustainable Manufacturing; OECD, Organisation for Economic Co-operation and Development; PEF, Product Environment Footprint; ProdSI, Product Sustainability Index; ProcSI, Process SI; Line SI, Line SI; PlaSI, Plant SI; ProSA, Product Sustainability Assessment; SCM, Supply Chain Management; S-LCA, Social LCA; SMSI (Nike), Sustainable Manufacturing Sourcing Index; Systems Thinking Approach (Moldavska and Welo, 2016); Sustainability Characterization Methodology, Mani et al. (2014).
Most of the SA literature in manufacturing follows an indicators-based approach and extensively employs LCA for assessment in conjunction with various decision-making tools (e.g., MCDM, DES, and DEA). A combination of qualitative and quantitative metrics has been used for assessment. SM research has studied three types of indicators: conventional (efficiency, cost, quality, etc.), operation (energy and water use, pollutant emissions, etc.), and prevention (potential for recycle, reuse, and disassembly, etc.) (Singh et al., 2012). A comprehensive set of classification of indicators has been presented based on NIST's Sustainable Manufacturing Indicator Repository (SMIR), adding technological advancement and performance management to the conventional sustainability dimensions/TBL concept (Feng et al., 2010; OECD, 2011). Other SA indices (e.g., eco-points, eco-compass, and eco-indicator99) for manufacturing industries have been presented (Singh et al., 2012). Human toxicity and water footprint indicators have received lesser attention than extensively studied environmental indicators (e.g., resource-use and emission reduction) (Esmaeilian et al., 2016). Stakeholders' focus varies with the manufacturing level, e.g., designers and engineers for the product, process, and systems-level; decision-makers (manufacturers) for facility level. A holistic SA framework to capture value across the manufacturing networks has been developed (Bocken et al., 2013). Further, a control engineering-based decision-support systems framework to improve the sustainability of manufacturing industries has also been developed (Moradi-Aliabadi and Huang, 2018). A strong sustainability-oriented framework for manufacturing has been proposed (Pinto et al., 2020). This work's noticeable contribution is industry stakeholders' involvement in agreeing on the weighting of sustainability dimensions for strong sustainability. A novel approach to assess sustainability and circular economy performance of industrial sectors has been proposed (Arbolino et al., 2022).
The use and post-use phases have received less attention than the manufacturing phase, probably due to well-defined boundaries and easily available data. The use stage impacts such as consumers' exposure due to indoor (near-field) emissions during the use stage have not been considered so far. Near-field active impacts are also crucial to address in a manufacturing environment for effective SA. An inclination toward social and technical dimensions can be observed in recent publications. Indicators such as workers' and consumers' health and safety, employment, community impact, risk and hazards, and toxic releases have been studied generally under the social dimension. The assessment methods are not geographically relevant except few studies, e.g., ISO (2006) and Kamyotra and Central Pollution Control Board (2009). Strong sustainability has been overlooked in the past, but with the current pandemic and climate change is being accepted, though with operational reluctance.
Process-Level SA
Unit manufacturing process (UMP) level SA in manufacturing employs multiple combinations of approaches for assessment (e.g., LCA, analytical/mathematical modeling, material information models) and decision making (e.g., multi-criteria decision making, analytical network process) (Pineda-Henson and Culaba, 2004; Roy and Li, 2014; Bork et al., 2016; Doran et al., 2016). The assessment focuses on either the manufactured part/product or the production process under study. SA has been conducted for generic and specific subtractive and additive production processes (Lu et al., 2012; Priarone and Ingarao, 2017; Hegab et al., 2018; Saad et al., 2019). A wide range of general and case-specific, qualitative, and quantitative indicators assess the environmental, social, economic, and technical impacts. A recent study evaluated the effect of behavioral and cognitive aspects of the manufacturing environment on workers' performance (Peruzzini and Pellicciari, 2018).
Systems and Facility Level SA
Systems and facility-level SA utilize assessment methods from manufacturing performance literature, e.g., systems integration model (Mani et al., 2013), value stream mapping and material resource planning (Faulkner and Badurdeen, 2014; Huang and Badurdeen, 2017), enterprise resource planning (Li et al., 2016). Further, many methods employ methods from disciplines other than manufacturing performance assessment, such as economic input-output (Egilmez et al., 2013), Fuzzy inference system (Singh et al., 2014), Graph theory (Jayakrishna et al., 2016), and systems thinking (Moldavska and Welo, 2016), and multi-criteria decision models (Stoycheva et al., 2018). A SA framework integrating traditional manufacturing parameters (quality, flexibility, and time) with sustainability metrics has been proposed to compare different process plans (Saxena et al., 2020). The indicators span across multiple sustainability dimensions with a sufficient focus on social dimensions and workers' health and wellbeing. A questionnaire-based study included ecosystem health indicators such as biodiversity and natural hazard (Chen et al., 2014) and impacts on species, aquatic life, and land use (Stoycheva et al., 2018). A few studies consider the impacts across product life cycle stages (Yang et al., 2014; Huang and Badurdeen, 2017; Stoycheva et al., 2018). Another framework studied geography-specific environmental impacts due to manufacturing clusters (Kamyotra and Central Pollution Control Board, 2009). A system thinking and complexity-theory-based approach for facility-level SA also has been proposed (Moldavska and Welo, 2019). A simulation tool named Sustainable Manufacturing Planning and Simulation (SMPS) has been developed to predict environmental impacts occurring during various product development (design and manufacturing) stages (Mani et al., 2013). It was developed based on the earlier model Systems Integration for Manufacturing Applications (SIMA) by integrating sustainability aspects in the product life cycle. Further, a simulation-based theoretical framework has been proposed for sustainability impact analysis to integrate traditional product design processes with sustainability strategies (Moldavska and Welo, 2016). The systems dynamics approach has been demonstrated to model SM problems (Zhang et al., 2013). Systems thinking can potentially shift the focus of SA from a reductionist to a holistic approach. Further, the framework has been tested for manufacturing process-level decision-making regarding absolute environmental sustainability (Bjørn et al., 2020b).
Operationalizing SA practices in the manufacturing industry by implementing the frameworks and tools developed by academia have been the priority. In this direction, few attempts have been made to fill the gap between industry and academia by involving industry stakeholders in the assessment process. The information-gathering regarding various challenges to implementing SA methods in the industry has been carried out using semi-structured interviews of the industry focus groups (Smullin et al., 2016; Trianni et al., 2017). A study has analyzed a comprehensive database of about 45,000 reports to understand the reporting pattern and challenges in using LCA in corporate sustainability reporting (Stewart et al., 2018). Standardized metrics supporting the decision-making responsive to local geographies involved in the product life cycle, relatable to the industry's core business practices, are required to support the industry SA practice (Smullin et al., 2016). Reactive assessment focusing on energy and material efficiency is predominantly practiced by most companies, primarily driven by economic benefits and regulatory pressure (Alayón et al., 2017). Simple metrics related to core business practices, supported by transparent data, standardized process information models, and incentives are required to accelerate the adoption of SA practices in the manufacturing industry (Nahkala, 2013). More recently, a detailed analysis of corporate reporting from 2000 to 2014 revealed that a small percentage of industries incorporate ecological limits (planetary boundaries) in the sustainability reporting process (Bjørn et al., 2017b). Integrating circularity in the SA in the industry requires an indispensable contribution by all stakeholders involved in the value chain (Lieder and Rashid, 2016). Industry 4.0 and digitization is anticipated as a potential enabler of circularity (Nascimento et al., 2019; Rajput and Singh, 2019). To further explain the state of industrial SA practice, we present a brief overview of four sustainability reports and sustainability standards followed in the industries for assessing and reporting sustainability.
Industry Perspective
Various standards are followed (ISO, GRI, GHG protocol, etc.) for conducting and reporting the sustainability of the industry. GRI is one of the most followed standards in larger organizations and has launched a digital reporting tool (https://digital.globalreporting.org/) containing multiple organizations' databases and sustainability reports. Organizations attempt to look at all three pillars of sustainability with varying degrees of focus. The size of the firm/manufacturing facility is one of the deciding factors for the type of assessment practice being followed, as large firms in India tend to follow environmental assessments compared to small and medium level firms (Singh et al., 2016). Chemicals and pharmaceuticals, electronics and computers, oil and gas, automotive, and metals and manufacturing industries are the top sustainability reporting sectors among the panel of Fortune Global 250 firms. The sustainability reporting methods adopted by industry can be broadly categorized into three categories:
• reporting frameworks (e.g., GRI),
• standards (e.g., ISO EMS. ISO 26000, OECD), and
• ratings/indices (e.g., LEED green building rating) (Olanipekun et al., 2021).
LCA tools are commonly used for sustainability reporting in Europe and North America regions (Stewart et al., 2018), primarily because the regional databases are easily available. Companies often confuse sustainability performance (assessment) with sustainability-oriented practices because of unclear definitions of SM. The sustainability challenges would be more dominant as more industries focus on economic sustainability over environmental sustainability in a post-COVID-19 world (Zhang et al., 2020).
Industry Claim of Practice
This section reviews sustainability reports of four leading apparel, electronics, and automobiles manufacturing industries (Nike Inc., Apple Inc., Dell, and BMW) based on the effectiveness criteria discussed in the paper. The selection was primarily made based on the quality of reporting, level of details provided, and information disclosed. Table 4 summarizes the review results from the four reports. The scope definition interprets the industry's vision of sustainability and SM. It is observed that the definition follows a similar trend as SM definitions proposed in academic research (Table 3). The focus for assessment across various life cycle phases and geographical locations has been analyzed and presented in the reports. Significant efficiency-based improvements in material and energy usage can be noticed (Table 5). The improvements are benchmarked against earlier financial year's reporting data, e.g., improvements such as environmental concerns (including ethical sourcing) have been discussed for the company's economic benefits. The benchmarking must be done against the absolute pollution boundaries for effectiveness, requiring theoretical developments. Notably, most industries have not addressed geographical challenges (see Table 5). The importance and difficulty in tracing impacts over the geographies have been indicated in the reports. Also, an inclination toward the circular economy and safer chemicals can be observed.
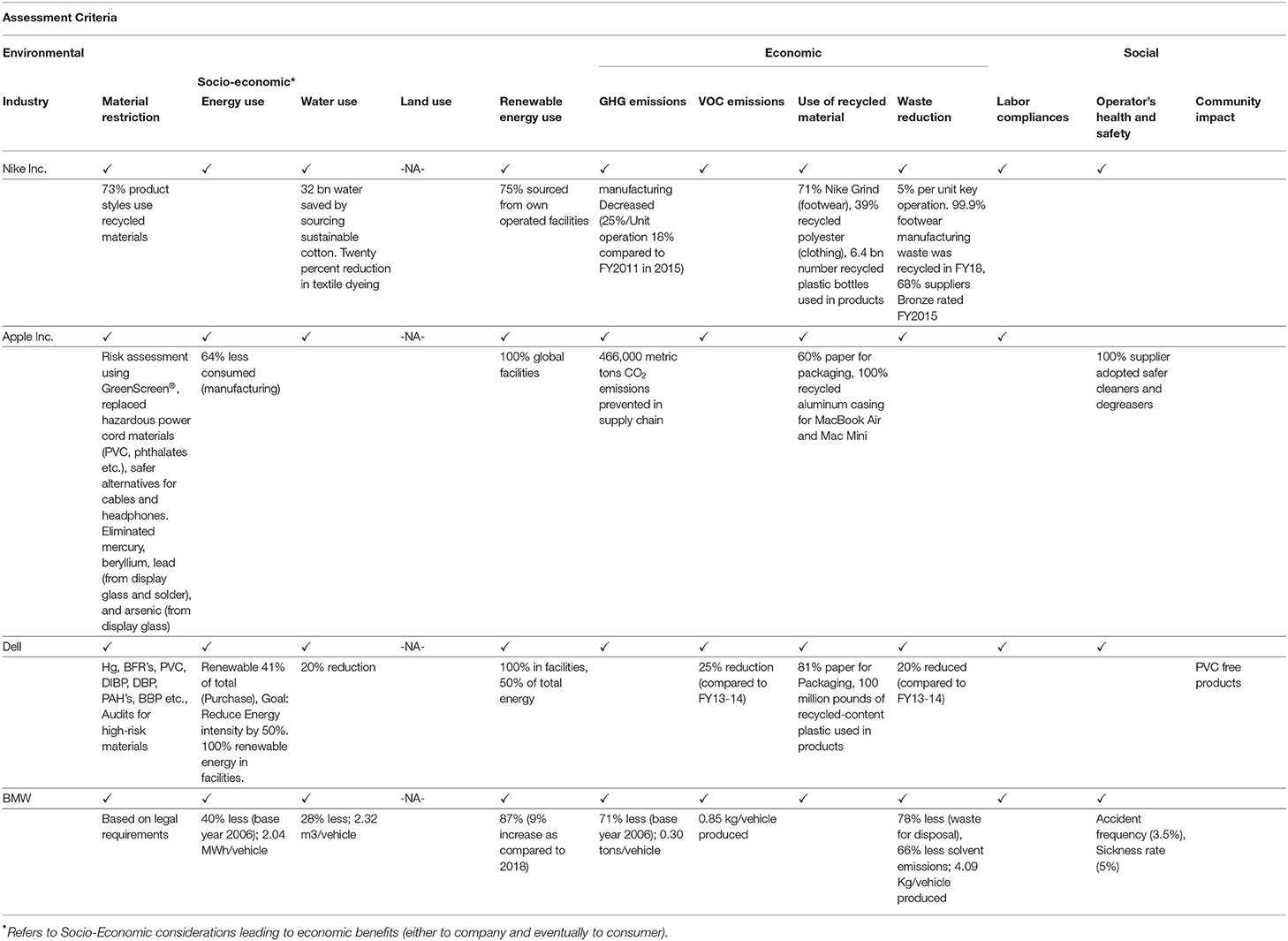
Table 5. Sustainability assessment in industry; compared against effectiveness metrics (partially adapted from Joung et al., 2013).
Contrary to literature reviewed in SA in academia (theory), the corporate reporting focuses on individual sustainability pillars and misses systemic linkages. Industry adoption of theoretical assessment methods is at a nascent stage due to lack of industry-specific metrics, incomplete understanding of corporate sustainability, resource, and time-intensive assessment. Few industries have developed their own SA tools, e.g., Ford PSI and Nike SMSI. The selected reports have been evaluated against the environmental, economic, socio-economic, and social parameters in Table 5. Reduction in various parameters (per financial year) has been reported (see Table 5), reflecting efficiency-based assessment.
Sustainable Manufacturing for Effectiveness: Challenges and Opportunities
The main challenges/opportunities in achieving effectiveness in SM can be broadly categorized into three categories:
(a) methodological challenges requiring paradigm shift from efficiency-based assessment to effectiveness,
(b) data requirements pertaining to the effectiveness-based methodologies, and
(c) Implementation challenges enabling the adoption of SA practices in the industry.
Effectiveness in SM implies products and processes that do not pollute, as against efficient operations that pollute, but less. Effectiveness is most pertinent today, as pursuits of efficiency in energy/resource use have only aggravated net energy/resource consumption. Integrated systems approach involving multiple stakeholders and assessment tools, effectiveness indicators, knowledge from multiple disciplines (e.g., SA, manufacturing performance, systems thinking, and CE), benchmarked against local and global pollution limits, would be a way forward toward effectiveness Effectively assessing sustainability performance for a globally distributed manufacturing/life cycle network is a challenging task. Policy (top-down) and process-level (bottom-up) interventions are required to integrate (a) the hierarchal disconnect in manufacturing decision-makers and (b) different stakeholders' concerns in the value chain on a single platform for effective decision-making. A potential future challenge for SM is to establish the causality between product life cycle activities and various impacts and trace impacts to design and manufacturing decisions. It can be effectively achieved by considering life cycle stages beyond manufacturing, incorporating verifiable metrics, simulation-based design, manufacturing impact analysis, and resolving trade-offs among sustainability dimensions and conflicts such as rebound effects. Integrating design and manufacturing simulation tools with the SA frameworks is crucial for effective SA. Utilizing strategies such as waste utilization (avoid downcycling), closed-loop material and energy flows (circularity), and material substitution (safer chemicals) could be helpful in this direction. It is crucial to concurrently consider the horizontal dimension of the product life cycle (i.e., life cycle stages and circular economy scenarios) and vertical structure. i.e., manufacturing levels (process, systems, facility, and supply chain level) for a holistic assessment. New manufacturing processes and business models (e.g., product-service systems and circular economy), predictive LCA tools with reliable datasets for assessing various impacts on local and global communities are required for improved SM (Moldavska and Welo, 2015). The manufacturing industry needs to expand the focus of SA beyond efficiency measures (material efficiency, resource efficiency, emissions reduction per year) and practice resource accounting with respect to the ecosystem capacity, and include social dimensions.
An integrated top-down (policy) and bottom-up (industry decision-making) approach considering environmental impacts, waste avoidance, and economic benefits simultaneously could aid the effective implementation of circularity strategies in the manufacturing industry (de Pascale et al., 2020; Kristensen and Mosgaard, 2020). The circular economy framework has been proposed to decouple environmental impacts from economic growth by adapting various circularity principles such as designing out waste, keeping materials in use by extending life cycles, and regenerating natural systems (The Ellen MacArthur Foundation, 2017). Circularity is essential for handling resource scarcity and metal criticality challenges (Tercero Espinoza et al., 2020) and is also continuously perceived as a prerequisite for SM (Skerlos, 2015). Circular Manufacturing strategies are adopted in the industry at various manufacturing levels (i.e., process, systems, factory-level/policy level or micro, meso, and macro-level, respectively (Kirchherr et al., 2017). Various circularity assessment metrics and indicators have been developed at different levels of manufacturing, e.g., product level (Cayzer et al., 2017), company level (Vinante et al., 2021), and supply chain level (Calzolari et al., 2021). Integrating circularity indicators in SA practices in manufacturing is a way forward to address resource effectiveness challenges such as metal criticality in addition to implementing circularity practice. A few studies have integrated LCA and circularity assessment (Rigamonti and Mancini, 2021). It is also crucial to consider the exposure limits of chemical and various material-chemical interactions while evaluating CE scenarios (Fantke and Illner, 2019).
Further, integrating environmental footprint methodologies with the local/regional and global planetary boundaries (PB) is vital to assess human activities' performance relative to environmental thresholds (Fang et al., 2015). An increased interest in the absolute environmental sustainability assessment (AESA) for positive decoupling of environmental impacts and economic growth can be observed in the literature (Bjørn et al., 2020a). Previous work on absolute SA includes determining planetary for industries in a particular region (Zhang et al., 2022), individual industries (Kara et al., 2018; Moshrefi et al., 2019), industry networks (Samaroo et al., 2020), and socio-ecological systems (Hossain et al., 2017). These studies are a good starting point to develop SA methods for different geographies and industrial sectors integrating local and global pollution limits.
The challenges associated with the data requirements for the pathways for effectiveness discussed above are crucial to overcome. The challenges involve data variability, user behavior, and interconnections among sustainability dimensions (Brundage et al., 2018). Big data analytics and deep learning could play a crucial role in guiding decision-making for SM in the era of industry 4.0 (Lee et al., 2019).
Aggregated metrics from multiple manufacturing levels and dedicated tools for each level must be collectively employed for a comprehensive and effective SA (Herrmann et al., 2014, 2015). “Positive impact factories” aim to effectively contribute to positive social and environmental impacts instead of doing “less bad” (efficiency-based approach) by incorporating interdependencies in the elements in the industry environment (Herrmann et al., 2015). Industry 4.0 has a huge potential for contributing to SM through data collection, improved working conditions and operator's health, energy efficiency, and enabling circular economy by systems integration and use of additive manufacturing technology (Sartal et al., 2020). Blockchains technology could enhance the traceability of products by operationalizing product service systems (PSS) and CE. In addition, material substitution strategies such as chemical alternatives assessment (Lavoie et al., 2010; Fantke et al., 2020) could aid performance assessment for alternative materials for effective decision-making and results.
From an implementation/operational point of view, multiple barriers limit the adoption of SA practice in the manufacturing industry. Adopting a SA framework depends on the time and resources (data, skills, and financial) required for conducting the assessment. The barriers/challenges vary across the size of manufacturing enterprises due to their financial constraints. The development of sustainability standards could be a viable option for improved implementation of SA methods in small and medium manufacturing enterprises (Escoto et al., 2022).
Conclusion
This paper reviews several SA methods and tools supporting manufacturing enterprises' sustainability decision-making. Despite the advances in the development of SA measures in manufacturing, the practical applicability is lacking, leading to an inadequate positive (intended) impact on the global scale. Environmental and economic dimensions are more commonly considered than the social dimension, currently limited to the working environment (worker's health and safety). Assessment methods are comparatively less focused on the near field/indoor exposures and human toxicity impacts during product use. Industry adoption of existing SA methods in manufacturing is also not been appropriately documented. Assessment measures in the industry (practice) are primarily focused on organizations' sustainability compared to absolute environmental improvements. Reporting trends largely follow a reductionist (efficiency-based) approach, where the impact of resource and energy per product is reported. The industry reports have hardly accounted for the active impacts over the use phase. Sustainability reporting analysis shows the gap between industry and academic practices in SA in manufacturing. Including more reports in the study might reveal detailed information about the trends in industrial practice. Stakeholders significantly influence such impacts, and therefore, behavioral aspects are also essential to be considered in the assessment. Potential future work includes conducting a comprehensive questionnaire industry survey that might help reveal detailed information about challenges in adopting SA measures. Future studies on SA in manufacturing involve studying the impacts and benefits of new manufacturing paradigms such as Industry 4.0 on sustainability. Bridging the industry-academia gap can address climate change and global pollution challenges due to emerging pollutants such as microplastics and endocrine-disrupting chemicals. The effectiveness of SA would eventually guide toward absolute sustainability improvements and planetary wellness.
Author Contributions
Both authors listed have made a substantial, direct, and intellectual contribution to the work and approved it for publication.
Conflict of Interest
The authors declare that the research was conducted in the absence of any commercial or financial relationships that could be construed as a potential conflict of interest.
Publisher's Note
All claims expressed in this article are solely those of the authors and do not necessarily represent those of their affiliated organizations, or those of the publisher, the editors and the reviewers. Any product that may be evaluated in this article, or claim that may be made by its manufacturer, is not guaranteed or endorsed by the publisher.
Abbreviations
GHG, Greenhouse Gas; LCA, Life Cycle Assessment; LCSA, Life Cycle Sustainability Assessment; SA, Sustainability Assessment; SM, Sustainable Manufacturing.
References
Alayón, C., Säfsten, K., and Johansson, G. (2017). Conceptual sustainable production principles in practice: do they reflect what companies do? J. Clean. Prod. 141, 693–701. doi: 10.1016/j.jclepro.2016.09.079
Allwood, J. M., Ashby, M. F., Gutowski, T. G., and Worrell, E. (2011). Material efficiency: a white paper. Resour. Conserv. Recycl. 55, 362–381. doi: 10.1016/j.resconrec.2010.11.002
Alting, D. L., and Jøgensen, D. J. (1993). The life cycle concept as a basis for sustainable industrial production. CIRP Ann. Manufact. Technol. 42, 163–167. doi: 10.1016/S0007-8506(07)62417-2
Apple Inc. (2019). Environmental responsibility report. 87. Available online atat: https://www.apple.com/environment/pdf/Apple_Environmental_Responsibility_Report_2019.pdf (accessed June 18, 2021).
Arbolino, R., Boffardi, R., Ioppolo, G., Lantz, T. L., and Rosa, P. (2022). Evaluating industrial sustainability in OECD countries: a cross-country comparison. J. Clean. Prod. 331, 129773. doi: 10.1016/j.jclepro.2021.129773
Arvidsson, R., Tillman, A.-M., Sandén, B. A., Janssen, M., Nordelöf, A., Kushnir, D., et al. (2017). Environmental assessment of emerging technologies: recommendations for prospective LCA. J. Industr. Ecol. 22, 1286–1294. doi: 10.1111/jiec.12690
Asiedu, Y., and Gu, P. (1998). Product life cycle cost analysis: state of the art review. Int. J. Prod. Res. 36, 883–908. doi: 10.1080/002075498193444
Aurisano, N., Fantke, P., Huang, L., and Jolliet, O. (2021). Estimating mouthing exposure to chemicals in children's products. J. Expos. Sci. Environ. Epidemiol. 32, 94–102. doi: 10.1038/s41370-021-00354-0
Azevedo, I. M. L. (2014). Consumer end-use energy efficiency and rebound effects. Ann. Rev. Environ. Resourc. 39, 393–418. doi: 10.1146/annurev-environ-021913-153558
Baxter, T., Bebbington, J., Cutteridge, D., and Harvey, G. (2003). “The sustainability assessment model (SAM): measuring sustainable development performance,” in SPE Offshore Europe Oil and Gas Exhibition and Conference (Aberdeen). doi: 10.2118/83986-MS
Bell, S., and Morse, S. (2012). Sustainability Indicators, 2nd Edn. London: Routledge. doi: 10.4324/9781849772723
Benoît, C., Mazjin, B., Andrews, E. S., Barthel, L.-P., Ciroth, A., Cucuzzella, C., et al. (2009). Guidelines for Social Life Cycle Assessment of Products. Paris: UNEP.
Bernstein, W. Z., Boettjer, T., and Ramanujan, D. (2021). Quantifying life cycle inventories for machining processes at detailed design. Proc. CIRP 98, 370–375. doi: 10.1016/j.procir.2021.01.119
Bjørn, A., Bey, N., Georg, S., Røpke, I., and Hauschild, M. Z. (2017a). Is Earth recognized as a finite system in corporate responsibility reporting? J. Clean. Prod. 163, 106–117. doi: 10.1016/j.jclepro.2015.12.095
Bjørn, A., Bey, N., Georg, S., Røpke, I., and Hauschild, M. Z. (2017b). Is Earth recognized as a finite system in corporate responsibility reporting? J. Clean. Prod. 163, 106–117. doi: 10.1016/j.jclepro.2015.12.05
Bjørn, A., Chandrakumar, C., Boulay, A.-M., Doka, G., Fang, K., Gondran, N., et al. (2020a). Review of life-cycle based methods for absolute environmental sustainability assessment and their applications. Environ. Res. Lett. 15, 083001. doi: 10.1088/1748-9326/ab89d7
Bjørn, A., Margni, M., Roy, P.-O., Bulle, C., and Hauschild, M. Z. (2016). A proposal to measure absolute environmental sustainability in life cycle assessment. Ecol. Indicat. 63, 1–13. doi: 10.1016/j.ecolind.2015.11.046
Bjørn, A., Sim, S., King, H., Patouillard, L., Margni, M., Hauschild, M. Z., et al. (2020b). Life cycle assessment applying planetary and regional boundaries to the process level: a model case study. Int. J. Life Cycle Assess. 25, 2241–2254. doi: 10.1007/s11367-020-01823-8
Blok, K., Huijbregts, M., Patel, M. K., Hertwich, E., Hauschild, M., Sellke, P., et al. (2013). Handbook on a Novel Methodology for the Sustainability Impact of New Technologies. Utrecht: Utrecht University.
BMW Group. (2019). Sustainable Value Report 2019. Available online at: https://www.bmwgroup.com/content/dam/grpw/websites/bmwgroup_com/responsibility/downloads/en/2020/2020-BMW-Group-SVR-2019-Englisch.pdf (accessed May 27, 2021).
Bocken, N., Short, S., Rana, P., and Evans, S. (2013). A value mapping tool for sustainable business modelling. Corp. Govern. Int. J. Business Soc. 13, 482–497. doi: 10.1108/CG-06-2013-0078
Bond, A., and Morrison-Saunders, A. (2013). “Challenges in determining the effectiveness of sustainability assessment,” in Sustainability Assessment: Pluralism, Practice and Progress, eds A. Bond, A. Morrison-saunders, and R. Howitt (London: Routledge), 37–50.
Bork, C. A. S., de Souza, J. F., de Oliveira Gomes, J., Canhete, V. V. P., and de Barba, D. J. (2016). Methodological tools for assessing the sustainability index (SI) of industrial production processes. Int. J. Adv. Manufact. Technol. 87, 1313–1325. doi: 10.1007/s00170-014-6684-8
Brundage, M. P., Bernstein, W. Z., Hoffenson, S., Chang, Q., Nishi, H., Kliks, T., et al. (2018). Analyzing environmental sustainability methods for use earlier in the product lifecycle. J. Clean. Prod. 187, 877–892. doi: 10.1016/j.jclepro.2018.03.187
Brundtland, G. H. (1987). Report of the World Commission on Environment and Development: Our Common Future. United Nations General Assembly, 300.
Calzolari, T., Genovese, A., and Brint, A. (2021). Circular economy indicators for supply chains: a systematic literature review. Environ. Sust. Indic. 13, 100160. doi: 10.1016/j.indic.2021.100160
Cao, H., and Folan, P. (2012). Product life cycle: the evolution of a paradigm and literature review from 1950–2009. Prod. Plann. Control 23, 641–662. doi: 10.1080/09537287.2011.577460
Carson, R., Carson, R., Lear, L., Lear, L., Wilson, E. O., and Wilson, E. O. (2002). Silent Spring. Boston, MA; New York, NY: Houghton Mifflin Harcourt Learning Technology.
Cayzer, S., Griffiths, P., and Beghetto, V. (2017). Design of indicators for measuring product performance in the circular economy. Int. J. Sust. Eng. 10, 289–298. doi: 10.1080/19397038.2017.1333543
Chakrabati, A. (2015). “A spatio-temporal network representation for manufacturing.” in ICoRD'15-Research into Design Across Boundaries, Vol. 2 (New Delhi: Springer), 459–470. doi: 10.1007/978-81-322-2229-3
Chandrakumar, C., and McLaren, S. J. (2018). Towards a comprehensive absolute sustainability assessment method for effective Earth system governance: defining key environmental indicators using an enhanced-DPSIR framework. Ecol. Indicat. 90, 577–583. doi: 10.1016/j.ecolind.2018.03.063
Chandran, K. M., Chakrabarti, A., and Mani, M. (2016). “A spatio-temporal product lifecycle network representation,” in Product Lifecycle Management for Digital Transformation of Industries: 13th IFIP WG 5.1 International Conference, PLM 2016, Columbia, SC, USA, July 11-13, 2016, Revised Selected Papers, eds. R. Harik, L. Rivest, A. Bernard, B. Eynard, and A. Bouras (Cham: Springer International Publishing), 606–617. doi: 10.1007/978-3-319-54660-5_54
Chen, D., Schudeleit, T., Posselt, G., and Thiede, S. (2013). A state-of-the-art review and evaluation of tools for factory sustainability assessment. Proc. CIRP 9, 85–90. doi: 10.1016/j.procir.2013.06.173
Chen, D., Thiede, S., Schudeleit, T., and Herrmann, C. (2014). A holistic and rapid sustainability assessment tool for manufacturing SMEs. CIRP Ann. Manufact. Technol. 63, 437–440. doi: 10.1016/j.cirp.2014.03.113
Circle Economy. (2022). The Circularity Gap Report 2022. Amsterdam. Available online at: https://www.circularity-gap.world/2022#Download-the-report (accessed February 14, 2022).
de Pascale, A., Arbolino, R., Szopik-Depczyńska, K., Limosani, M., and Ioppolo, G. (2020). A systematic review for measuring circular economy: the 61 indicators. J. Clean. Prod. 281, 124942. doi: 10.1016/j.jclepro.2020.124942
Dell Corporation. (2017). An annual update on our 2020 legacy of good plan FY2016 corporate social responsibility report, 1–47. Available online at: http://i.dell.com/sites/doccontent/corporate/corp-comm/en/Documents/fy17-cr-report.pdf (accessed June 21, 2021).
Despeisse, M., Mbaye, F., Ball, P. D., and Levers, A. (2012). The emergence of sustainable manufacturing practices. Prod. Plann. Control 23, 354–376. doi: 10.1080/09537287.2011.555425
Doran, M. P., Smullin, M. M., and Haapala, K. R. (2016). “An approach to compare sustainability performance of additive and subtractive manufacturing during process planning, Vol. 4: 21st design for manufacturing and the life cycle conference,” in 10th International Conference on Micro- and Nanosystems (Charlotte, NC), V004T05A047-V004T05A047. doi: 10.1115/DETC2016-60209
Dreyer, L., Hauschild, M., and Schierbeck, J. (2006). A framework for social life cycle impact assessment (10 pp). Int. J. Life Cycle Assess. 11, 88–97. doi: 10.1065/lca2005.08.223
Dreyer, L. C., Hauschild, M. Z., and Schierbeck, J. (2010). Characterisation of social impacts in LCA: part 1: development of indicators for labour rights. Int. J. Life Cycle Assess. 15, 247–259. doi: 10.1007/s11367-009-0148-7
Duflou, J. R., Sutherland, J. W., Dornfeld, D., Herrmann, C., Jeswiet, J., Kara, S., et al. (2012). Towards energy and resource efficient manufacturing: a processes and systems approach. CIRP Ann. Manufact. Technol. 61, 587–609. doi: 10.1016/j.cirp.2012.05.002
Earles, J. M., and Halog, A. (2011). Consequential life cycle assessment: a review. Int. J. Life Cycle Assessment.16, 445–453. doi: 10.1007/s11367-011-0275-9
Egilmez, G., Kucukvar, M., and Tatari, O. (2013). Sustainability assessment of US manufacturing sectors: an economic input output-based frontier approach. J. Clean. Prod. 53, 91–102. doi: 10.1016/j.jclepro.2013.03.037
Eriksen, M., Lebreton, L. C. M., Carson, H. S., Thiel, M., Moore, C. J., Borerro, J. C., et al. (2014). Plastic pollution in the world's oceans: more than 5 trillion plastic pieces weighing over 250,000 tons Afloat at Sea. PLoS ONE 9, e111913. doi: 10.1371/journal.pone.0111913
Ernstoff, A. S., Fantke, P., Csiszar, S. A., Henderson, A. D., Chung, S., and Jolliet, O. (2016). Multi-pathway exposure modeling of chemicals in cosmetics with application to shampoo. Environ. Int. 92, 87–96. doi: 10.1016/j.envint.2016.03.014
Escoto, X., Gebrehewot, D., and Morris, K. C. (2022). Refocusing the barriers to sustainability for small and medium-sized manufacturers. J. Clean. Prod. 338, 130589. doi: 10.1016/j.jclepro.2022.130589
Esmaeilian, B., Behdad, S., and Wang, B. (2016). The evolution and future of manufacturing: a review. J. Manufact. Syst. 39, 79–100. doi: 10.1016/j.jmsy.2016.03.001
European Commission. (2017). Review report of the Environmental Footprint Pilot phase, 1–48. Available online at: http://ec.europa.eu/environment/eussd/smgp/pdf/2017_peer_rev_finrep.pdf (accessed May 20, 2021).
Fang, K., Heijungs, R., and de Snoo, G. R. (2015). Understanding the complementary linkages between environmental footprints and planetary boundaries in a footprint–boundary environmental sustainability assessment framework. Ecol. Econ. 114, 218–226. doi: 10.1016/j.ecolecon.2015.04.008
Fantke, P., Huang, L., Overcash, M., Griffing, E., and Jolliet, O. (2020). Life cycle based alternatives assessment (LCAA) for chemical substitution. Green Chem. 22, 6008–6024. doi: 10.1039/D0GC01544J
Fantke, P., and Illner, N. (2019). Goods that are good enough: introducing an absolute sustainability perspective for managing chemicals in consumer products. Curr. Opin. Green Sust. Chem. 15, 91–97. doi: 10.1016/j.cogsc.2018.12.001
Faulkner, W., and Badurdeen, F. (2014). Sustainable Value Stream Mapping (Sus-VSM): methodology to visualize and assess manufacturing sustainability performance. J. Clean. Prod. 85, 8–18. doi: 10.1016/j.jclepro.2014.05.042
Fauré, E., Arushanyan, Y., Ekener, E., Miliutenko, S., and Finnveden, G. (2017). Methods for assessing future scenarios from a sustainability perspective. Eur. J. Fut. Res. 5, 17. doi: 10.1007/s40309-017-0121-9
Feng, S., Joung, C., and Li, G. (2010). “Development overview of sustainable manufacturing metrics,” in 17th CIRP International Conference on Life Cycle Engineering, Vol. 6 (Hefei), 6–12.
Garetti, M., and Taisch, M. (2012). Sustainable manufacturing: trends and research challenges. Prod. Plann. Control 23, 83–104. doi: 10.1080/09537287.2011.591619
Gasparatos, A., El-Haram, M., and Horner, M. (2008). A critical review of reductionist approaches for assessing the progress towards sustainability. Environ. Impact Assess. Rev. 28, 286–311. doi: 10.1016/j.eiar.2007.09.002
Gasparatos, A., and Scolobig, A. (2012). Choosing the most appropriate sustainability assessment tool. Ecol. Econ. 80, 1–7. doi: 10.1016/j.ecolecon.2012.05.005
Gaziulusoy, A. I., and Boyle, C. (2013). Proposing a heuristic reflective tool for reviewing literature in transdisciplinary research for sustainability. J. Clean. Prod. 48, 139–147. doi: 10.1016/j.jclepro.2012.04.013
Gbededo, M. A., Liyanage, K., and Garza-Reyes, J. A. (2018). towards a life cycle sustainability analysis: a systematic review of approaches to sustainable manufacturing. J. Clean. Prod. 184, 1002–1015. doi: 10.1016/j.jclepro.2018.02.310
Geissdoerfer, M., Savaget, P., Bocken, N. M. P., and Hultink, E. J. (2017). The circular economy – a new sustainability paradigm? J. Clean. Prod. 143, 757–768. doi: 10.1016/j.jclepro.2016.12.048
Gerona, R., vom Saal, F. S., and Hunt, P. A. (2019). BPA: have flawed analytical techniques compromised risk assessments? Lancet Diabetes Endocrinol. 8, 2019–2021. doi: 10.1016/S2213-8587(19)30381-X
Gibson, R. B. (2006). Beyond the pillars: sustainability assessment as a framework for effective integration of social, economic and ecological considerations in significant decision-making. J. Environ. Assess. Policy Manag. 8, 259–280. doi: 10.1142/S1464333206002517
Gillingham, K., Kotchen, M. J., Rapson, D. S., and Wagner, G. (2013). The rebound effect is overplayed. Nature 493, 475–476. doi: 10.1038/493475a
Global Reporting Initiative. (2015). Global Reporting Initiative. Available online at: https://www.globalreporting.org/information/about-gri/Pages/default.aspx (accessed October 10, 2018).
González-Martín, J., Kraakman, N. J. R., Pérez, C., Lebrero, R., and Muñoz, R. (2021). A state–of–the-art review on indoor air pollution and strategies for indoor air pollution control. Chemosphere 262, 128376. doi: 10.1016/j.chemosphere.2020.128376
Graedel, T. E., Harper, E. M., Nassar, N. T., Nuss, P., Reck, B. K., and Turner, B. L. (2015). Criticality of metals and metalloids. Proc. Natl. Acad. Sci. U. S. Am. 112, 4257–4262. doi: 10.1073/pnas.1500415112
Grießhammer, R., Buchert, M., Gensch, C.-O., Hochfeld, C., Reisch, L., and Rüdenauer, I. (2007). PROSA – product sustainability assessment guideline. Integr. Vlsi J. 23, 477–483.doi: 10.1002/lary.23646
Guinée, J. (2016). “Life cycle sustainability assessment: what is it and what are its challenges?” in BT - Taking Stock of Industrial Ecology, eds. R. Clift and A. Druckman (Cham: Springer International Publishing), 45–68. doi: 10.1007/978-3-319-20571-7_3
Guinée, J. B., Heijungs, R., Huppes, G., Zamagni, A., Masoni, P., Buonamici, R., et al. (2011). Life cycle assessment: past, present, and future. Environ. Sci. Technol. 45, 90–96. doi: 10.1021/es101316v
Gupta, J., Hurley, F., Grobicki, A., Keating, T., Stoett, P., Baker, E., et al. (2019). Communicating the health of the planet and its links to human health. Lancet Planet. Health 5196, 2018–2020. doi: 10.1016/S2542-5196(19)30040-3
Gutowski, T. G. (2018). A critique of life cycle assessment; where are the people? Proc. CIRP 69, 11–15. doi: 10.1016/j.procir.2018.01.002
Gutowski, T. G., Allwood, J. M., Herrmann, C., and Sahni, S. (2013). A global assessment of manufacturing: economic development, energy use, carbon emissions, and the potential for energy efficiency and materials recycling. Annu. Rev. Environ. Resour. 38, 81–106. doi: 10.1146/annurev-environ-041112-110510
Haapala, K. R., Camelio, J., Sutherland, J. W., Skerlos, S. J., Dornfeld, D. A., Zhao, F., et al. (2013). A review of engineering research in sustainable manufacturing. J. Manuf. Sci. Eng. 135, 041013-1-041013-041016. doi: 10.1115/1.4024040
Hák, T., Moldan, B., and Dahl, A. L. (2012). “Sustainability indicators: A scientific assessment,” in Island Press, Vol. 67 (Washington, DC: Island Press).
Ham, B. (2019). Indoor chemical pollution impacts often remain invisible. Science 366, 1084–1085. doi: 10.1126/science.366.6469.1084
Hannouf, M., and Assefa, G. (2017). Life cycle sustainability assessment for sustainability improvements: a case study of high-density polyethylene production in Alberta, Canada. Sustainability 9, 2332. doi: 10.3390/su9122332
Hauschild, M. Z. (2015). Better – But is it Good Enough? On the need to consider both eco-efficiency and eco-effectiveness to gauge industrial sustainability. Proc. CIRP 29, 1–7. doi: 10.1016/j.procir.2015.02.126
Hegab, H. A., Darras, B., and Kishawy, H. A. (2018). Towards sustainability assessment of machining processes. J. Clean. Prod. 170, 694–703. doi: 10.1016/j.jclepro.2017.09.197
Heijungs, R., Huppes, G., and Guinée, J. B. (2010). Life cycle assessment and sustainability analysis of products, materials and technologies. Toward a scientific framework for sustainability life cycle analysis. Polymer Degrad. Stability 95, 422–428. doi: 10.1016/j.polymdegradstab.2009.11.010
Herrmann, C., Blume, S., Kurle, D., Schmidt, C., and Thiede, S. (2015). The positive impact factory - transition from eco-efficiency to eco-effectiveness strategies in manufacturing. Proc. CIRP 29, 19–27. doi: 10.1016/j.procir.2015.02.066
Herrmann, C., Schmidt, C., Kurle, D., Blume, S., and Thiede, S. (2014). Sustainability in manufacturing and factories of the future. Int. J. Precis. Eng. Manufact. Green Technol. 1, 283–292. doi: 10.1007/s40684-014-0034-z
Hossain, M. S., Dearing, J. A., Eigenbrod, F., and Johnson, F. A. (2017). Operationalizing safe operating space for regional social-ecological systems. Sci. Total Environ. 584–585, 673–682. doi: 10.1016/j.scitotenv.2017.01.095
Huang, A., and Badurdeen, F. (2017). Sustainable manufacturing performance evaluation at the enterprise level: index- And value-based methods. Smart Sust. Manufact. Syst. 1, 178–203. doi: 10.1520/SSMS20170004
Huang, L., Anastas, N., Egeghy, P., Vallero, D. A., Jolliet, O., and Bare, J. (2019). Integrating exposure to chemicals in building materials during use stage. Int. J. Life Cycle Assess. 24, 1009–1026. doi: 10.1007/s11367-018-1551-8
Huang, L., Ernstoff, A., Fantke, P., Csiszar, S. A., and Jolliet, O. (2017). A review of models for near-field exposure pathways of chemicals in consumer products. Sci. Total Environ. 574, 1182–1208. doi: 10.1016/j.scitotenv.2016.06.118
Huang, L., Fantke, P., Ritscher, A., and Jolliet, O. (2021). Chemicals of concern in building materials: a high-throughput screening. J. Hazard. Mater. 424, 127574. doi: 10.1016/j.jhazmat.2021.127574
Hutchins, M. J., Robinson, S. L., and Dornfeld, D. (2013). Understanding life cycle social impacts in manufacturing: a processed-based approach. J. Manufact. Syst. 32, 536–542. doi: 10.1016/j.jmsy.2013.05.008
International Energy Agency. (2017). Energy Efficiency 2017. Paris: OECD. doi: 10.1787/9789264284234-en
ISO. (2006). “ISO 14040-environmental management - life cycle assessment - principles and framework,” in International Organization for Standardization, Vol. 3 (Geneva). Available online at: https://www.iso.org/standard/37456.html
Jayakrishna, K., Vinodh, S., and Anish, S. (2016). A Graph Theory approach to measure the performance of sustainability enablers in a manufacturing organization. Int. J. Sust. Eng. 9, 47–58. doi: 10.1080/19397038.2015.1050970
Jayal, A. D., Badurdeen, F., Dillon, O. W., and Jawahir, I. S. (2010). Sustainable manufacturing: modeling and optimization challenges at the product, process and system levels. CIRP J. Manufact. Sci. Technol. 2, 144–152. doi: 10.1016/j.cirpj.2010.03.006
Jolliet, O., Ernstoff, A. S., Csiszar, S. A., and Fantke, P. (2015). Defining product intake fraction to quantify and compare exposure to consumer products. Environ. Sci. Technol. 49, 8924–8931. doi: 10.1021/acs.est.5b01083
Joung, C. B., Carrell, J., Sarkar, P., and Feng, S. C. (2013). Categorization of indicators for sustainable manufacturing. Ecol. Indicat. 24, 148–157. doi: 10.1016/j.ecolind.2012.05.030
Kamyotra, J. S., and Central Pollution Control Board, D. (2009). Criteria for Comprehensive Environmental Assessment of Industrial Clusters. New Delhi: Central Pollution Control Board Ministry of Environment and Forests. Available online at: https://cpcb.nic.in/comprehensive-environmental-pollution-index-cepi/ (accessed May 18, 2021).
Kara, S., Hauschild, M., and Herrmann, C. (2018). Target-driven life cycle engineering: staying within the planetary boundaries. Proc. CIRP 69, 3–10. doi: 10.1016/j.procir.2017.11.142
Kellens, K., Dewulf, W., Overcash, M., Hauschild, M. Z., and Duflou, J. R. (2012). Methodology for systematic analysis and improvement of manufacturing unit process life cycle inventory (UPLCI) CO2PE! initiative (cooperative effort on process emissions in manufacturing). Part 2: Case studies. Int. J. Life Cycle Assess. 17, 242–251. doi: 10.1007/s11367-011-0340-4
Kirchherr, J., Reike, D., and Hekkert, M. (2017). Conceptualizing the circular economy: an analysis of 114 definitions. Resour. Conserv. Recycl. 127, 221–232. doi: 10.1016/j.resconrec.2017.09.005
Kloepffer, W. (2008). Life cycle sustainability assessment of products. Int. J. Life Cycle Assess. 13, 89–94. doi: 10.1065/lca2008.02.376
Klöpffer, W., and Ciroth, A. (2011). Is LCC relevant in a sustainability assessment? Int. J. Life Cycle Assess. 16, 99–101. doi: 10.1007/s11367-011-0249-y
Knoepfel, I. (2001). Dow Jones sustainability group index: a global benchmark for corporate sustainability. Corp. Environ. Strat. 8, 6–15. doi: 10.1016/S1066-7938(00)00089-0
Kristensen, H. S., and Mosgaard, M. A. (2020). A review of micro level indicators for a circular economy – moving away from the three dimensions of sustainability? J. Clean. Prod. 243, 118531. doi: 10.1016/j.jclepro.2019.118531
Kumar, M., and Mani, M. (2019). A systems-based sustainability assessment framework to capture active impacts in product life cycle/manufacturing. Proc. Manufact. 33, 647–654. doi: 10.1016/j.promfg.2019.04.081
Kumar, M., and Mani, M. (2021a). Towards an interdisciplinary framework for effective sustainability assessment in manufacturing. Proc. CIRP 98, 79–84. doi: 10.1016/j.procir.2021.01.009
Kumar, M., and Mani, M. (2021b). “Sustainability assessment in manufacturing: perspectives, challenges, and solutions,” in Sustainable Manufacturing, eds K. Gupta and K. Salonitis (Chennai: Elsevier), 287–311. doi: 10.1016/B978-0-12-818115-7.00013-4
Lamb, W. F., Wiedmann, T., Pongratz, J., Andrew, R., Crippa, M., Olivier, J. G. J., et al. (2021). A review of trends and drivers of greenhouse gas emissions by sector from 1990 to 2018. Environ. Res. Lett. 16, 073005. doi: 10.1088/1748-9326/abee4e
Lavoie, E. T., Heine, L. G., Holder, H., Rossi, M. S., Lee, R. E., Connor, E. A., et al. (2010). Chemical alternatives assessment: enabling substitution to safer chemicals. Environ. Sci. Technol. 44, 9244–9249. doi: 10.1021/es1015789
Lee, H. T., Song, J. H., Min, S. H., Lee, H. S., Song, K. Y., Chu, C. N., et al. (2019). Research trends in sustainable manufacturing: a review and future perspective based on research databases. Int. J. Prec. Eng. Manufact. Green Technol. 6, 809–819. doi: 10.1007/s40684-019-00113-5
Lee, J. Y., and Lee, Y. T. (2014). A framework for a research inventory of sustainability assessment in manufacturing. J. Clean. Prod. 79, 207–218. doi: 10.1016/j.jclepro.2014.05.004
Leng, J., Ruan, G., Jiang, P., Xu, K., Liu, Q., Zhou, X., et al. (2020). Blockchain-empowered sustainable manufacturing and product lifecycle management in industry 4.0: a survey. Renew. Sust. Energy Rev. 132, 110112. doi: 10.1016/j.rser.2020.110112
Levasseur, A., Lesage, P., Margni, M., Deschěnes, L., and Samson, R. (2010). Considering time in LCA: dynamic LCA and its application to global warming impact assessments. Environ. Sci. Technol. 44, 3169–3174. doi: 10.1021/es9030003
Li, W., Alvandi, S., Kara, S., Thiede, S., and Herrmann, C. (2016). CIRP annals - manufacturing technology sustainability cockpit : an integrated tool for continuous assessment and improvement of sustainability in manufacturing. CIRP Ann. Manufact. Technol. 65, 5–8. doi: 10.1016/j.cirp.2016.04.029
Liao, C., and Kannan, K. (2011). Widespread occurrence of bisphenol a in paper and paper products: implications for human exposure. Environ. Sci. Technol. 45, 9372–9379. doi: 10.1021/es202507f
Lieder, M., and Rashid, A. (2016). Towards circular economy implementation: a comprehensive review in context of manufacturing industry. J. Clean. Prod. 115, 36–51. doi: 10.1016/j.jclepro.2015.12.042
Lowell Centre for Sustainable Production. (2020). Principles of Sustainable Production. https://www.uml.edu/Research/Lowell-Center/About/Sustainable-Production-Defined.aspx (accessed October 20, 2021).
Lu, T., Rotella, G., Feng, S. C., Badurdeen, F., Dillon, O. W., Rouch, K., and Jawahir, I. S. (2012). “Metrics-based sustainability assessment of a drilling process,” in Sustainable Manufacturing, ed G. Seliger (Berlin; Heidelberg: Springer), 59–64. doi: 10.1007/978-3-642-27290-5_8
Lyytimäki, J., Tapio, P., Varho, V., and Söderman, T. (2013). The use, non-use and misuse of indicators in sustainability assessment and communication. Int. J. Sust. Dev. World Ecol. 20, 385–393. doi: 10.1080/13504509.2013.834524
Mair, S., Jones, A., Ward, J., Christie, I., Druckman, A., and Lyon, F. (2018). “A critical review of the role of indicators in implementing the sustainable development goals BT - handbook of sustainability science and research,” in Handbook of Sustainability Science and Research, ed. W. Leal Filho (Cham: Springer International Publishing), 41–56. doi: 10.1007/978-3-319-63007-6_3
Mani, M., Ganesh, L. S., and Varghese, K. (2005). Sustainability and Human Settlements: Fundamental Issues, Modeling and Simulations. New Delhi: Sage.
Mani, M., Johansson, B., Lyons, K. W., Sriram, R. D., and Ameta, G. (2013). Simulation and analysis for sustainable product development. Int. J. Life Cycle Assess. 18, 1129–1136. doi: 10.1007/s11367-012-0538-0
Mani, M., Madan, J., Lee, J. H., Lyons, K. W., and Gupta, S. K. (2014). Sustainability characterisation for manufacturing processes. Int. J. Prod. Res. 52, 5895–5912. doi: 10.1080/00207543.2014.886788
Miah, J. H., Griffiths, A., McNeill, R., Halvorson, S., Schenker, U., Espinoza-Orias, N., et al. (2018). A framework for increasing the availability of life cycle inventory data based on the role of multinational companies. Int. J. Life Cycle Assess. 23, 1744–1760. doi: 10.1007/s11367-017-1391-y
Moldavska, A., and Martinsen, K. (2018). defining sustainable manufacturing using a concept of attractor as a metaphor. Proc. CIRP 67, 93–97. doi: 10.1016/j.procir.2017.12.182
Moldavska, A., and Welo, T. (2015). On the applicability of sustainability assessment tools in manufacturing. Proc. CIRP 29, 621–626. doi: 10.1016/j.procir.2015.02.203
Moldavska, A., and Welo, T. (2016). Development of manufacturing sustainability assessment using systems thinking. Sustainability 8, 5. doi: 10.3390/su8010005
Moldavska, A., and Welo, T. (2017). The concept of sustainable manufacturing and its definitions: a content-analysis based literature review. J. Clean. Prod. 166, 744–755. doi: 10.1016/j.jclepro.2017.08.006
Moldavska, A., and Welo, T. (2019). A holistic approach to corporate sustainability assessment: incorporating sustainable development goals into sustainable manufacturing performance evaluation. J. Manufact. Syst. 50, 53–68. doi: 10.1016/j.jmsy.2018.11.004
Moradi-Aliabadi, M., and Huang, Y. (2018). decision support for enhancement of manufacturing sustainability: a hierarchical control approach. ACS Sust. Chem. Eng. 6, 4809–4820. doi: 10.1021/acssuschemeng.7b04090
Morrison, G. (2008). Interfacial chemistry in indoor environments. Environ Sci Technol. 42, 3494–3499. doi: 10.1021/es087114b
Moshrefi, S., Kara, S., and Hauschild, M. (2019). A framework for estimating regional footprint of companies towards absolute sustainability. Proc. CIRP 80, 446–451. doi: 10.1016/j.procir.2019.01.050
Mudu, P., Terracini, B., and Martuzzi, M. (2014). Human Health in Areas with Industrial Contamination. Denmark: WHO Regional Office for Europe.
Nahkala, S. (2013). “Aligning product design methods and tools for sustainability,” in Re-engineering Manufacturing for Sustainability (Springer), 53–58. doi: 10.1007/978-981-4451-48-2_9
Nascimento, D. L. M., Alencastro, V., Quelhas, O. L. G., Caiado, R. G. G., Garza-Reyes, J. A., Rocha-Lona, L., et al. (2019). Exploring Industry 4.0 technologies to enable circular economy practices in a manufacturing context. J. Manufact. Technol. Manag. 30, 607–627. doi: 10.1108/JMTM-03-2018-0071
Nash, T. R., Chow, E. S., Law, A. D., Fu, S. D., Fuszara, E., Bilska, A., et al. (2019). Daily blue-light exposure shortens lifespan and causes brain neurodegeneration in Drosophila. npj Aging Mech. Dis. 5, 8. doi: 10.1038/s41514-019-0038-6
Nations, U. (2002). Sustainable Consumption and Production, 11–14. Available at: https://sustainabledevelopment.un.org/topics/sustainableconsumptionandproduction (accessed June 19, 2018).
Neely, A., Gregory, M., and Platts, K. (1995). Performance measurement system design: a literature review and research agenda. Int. J. Operat. Prod. Manag. 15, 80–116. doi: 10.1108/01443579510083622
Ness, B., Urbel-piirsalu, E., Anderberg, S., and Olsson, L. (2007). Categorising tools for sustainability assessment. Ecol. Econ. 60, 498–508. doi: 10.1016/j.ecolecon.2006.07.023
Ngai, P., and Chan, J. (2012). Global capital, the state, and Chinese workers: the Foxconn experience. Mod. China 38, 383–410. doi: 10.1177/0097700412447164
NIKE Inc. (2018). FY18 Impact Report. Available online at: https://purpose-cms-production01.s3.amazonaws.com/wp-content/uploads/2019/05/20194957/FY18_Nike_Impact-Report_Final.pdf (accessed May 25, 2021).
NITI Aayog. (2017). India Energy Dashboards. Available online at: https://niti.gov.in/edm/#elecConsumption (accessed October 10, 2018).
Nobile, M., Arioli, F., Pavlovic, R., Ceriani, F., Lin, S.-K., Panseri, S., et al. (2020). Presence of emerging contaminants in baby food. Food Addit. Contaminants Part A 37, 131–142. doi: 10.1080/19440049.2019.1682686
OECD. (2011). OECD Sustainable Manufacturing Tookit - Seven Steps to Environmental Excellence (Paris), 54.
Olanipekun, A. O., Omotayo, T., and Saka, N. (2021). Review of the use of corporate social responsibility (CSR) tools. Sust. Prod. Consump. 27, 425–435. doi: 10.1016/j.spc.2020.11.012
Onat, N. C., Kucukvar, M., Halog, A., and Cloutier, S. (2017). Systems thinking for life cycle sustainability assessment: a review of recent developments, applications, and future perspectives. Sustainability 9, 1–25. doi: 10.3390/su9050706
Onat, N. C., Kucukvar, M., Tatari, O., and Egilmez, G. (2016). Integration of system dynamics approach toward deepening and broadening the life cycle sustainability assessment framework: a case for electric vehicles. Int. J. Life Cycle Assess. 21, 1009–1034. doi: 10.1007/s11367-016-1070-4
Pallaro, E., Subramanian, N., Abdulrahman, M. D., and Liu, C. (2015). Sustainable production and consumption in the automotive sector: integrated review framework and research directions. Sust. Prod. Consump. 4, 47–61. doi: 10.1016/j.spc.2015.07.002
Panagos, P., Liederkerke, M., van Yigini, Y., and Montanarella, L. (2013). Contaminated sites in europe: review of the current situation based on data collected through a European network. J. Environ. Public Health 2013, 11. doi: 10.1155/2013/158764
Peruzzini, M., and Pellicciari, M. (2018). User experience evaluation model for sustainable manufacturing. Int. J. Comput. Integr. Manuf. 31, 494–512. doi: 10.1080/0951192X.2017.1305502
Pineda-Henson, R., and Culaba, A. B. (2004). A diagnostic model for green productivity assessment of manufacturing processes. Int. J. Life Cycle Assess. 9, 379–386. doi: 10.1007/BF02979081
Pintér, L., Hardi, P., Martinuzzi, A., and Hall, J. (2012). Bellagio STAMP: principles for sustainability assessment and measurement. Ecol. Indicat. 17, 20–28. doi: 10.1016/j.ecolind.2011.07.001
Pinto, L. F. R., Venturini, G., de, F. P., Digiesi, S., Facchini, F., and Neto, G. C. O. (2020). Sustainability assessment in manufacturing under a strong sustainability perspective—an ecological neutrality initiative. Sustainability 12, 1–40. doi: 10.3390/su12219232
Pope, J., Annandale, D., and Morrison-Saunders, A. (2004). Conceptualising sustainability assessment. Environ. Impact Assess. Rev. 24, 595–616. doi: 10.1016/j.eiar.2004.03.001
Pope, J., Bond, A., Hugé, J., and Morrison-saunders, A. (2017). Reconceptualising sustainability assessment. Environ. Impact Assess. Rev. 62, 205–215. doi: 10.1016/j.eiar.2016.11.002
Porras, S. P., Heinälä, M., and Santonen, T. (2014). Bisphenol A exposure via thermal paper receipts. Toxicol. Lett. 230, 413–420. doi: 10.1016/j.toxlet.2014.08.020
Poulsen, P. B., and Jensen, A. A. (2004). Working Environment in Life-Cycle Assessment. Pensacola, FL: Society of Environmental Toxicology and Chemistry (SETAC).
Priarone, P. C., and Ingarao, G. (2017). Towards criteria for sustainable process selection: on the modelling of pure subtractive versus additive/subtractive integrated manufacturing approaches. J. Clean. Prod. 144, 57–68. doi: 10.1016/j.jclepro.2016.12.165
Qing, X., Yutong, Z., and Shenggao, L. (2015). Assessment of heavy metal pollution and human health risk in urban soils of steel industrial city (Anshan), Liaoning, Northeast China. Ecotoxicol. Environ. Saf. 120, 377–385. doi: 10.1016/j.ecoenv.2015.06.019
Radnor, Z., and Barnes, D. (2007). Historical analysis of performance measurement and management in operations management. Int. J. Prod. Perform. Manag. 56, 384–396. doi: 10.1108/17410400710757105
Ragusa, A., Svelato, A., Santacroce, C., Catalano, P., Notarstefano, V., Carnevali, O., et al. (2021). Plasticenta: first evidence of microplastics in human placenta. Environ. Int. 146, 106274. doi: 10.1016/j.envint.2020.106274
Rajput, S., and Singh, S. P. (2019). Connecting circular economy and industry 4.0. Int. J. Inform. Manag. 49, 98–113. doi: 10.1016/j.ijinfomgt.2019.03.002
Rashid, A., Asif, F. M. A., Krajnik, P., and Nicolescu, C. M. (2013). Resource Conservative Manufacturing: an essential change in business and technology paradigm for sustainable manufacturing. J. Clean. Prod. 57, 166–177. doi: 10.1016/j.jclepro.2013.06.012
Revel, M., Châtel, A., and Mouneyrac, C. (2018). Micro(nano)plastics: a threat to human health? Curr. Opin. Environ. Sci. Health 1, 17–23. doi: 10.1016/j.coesh.2017.10.003
Rigamonti, L., and Mancini, E. (2021). Life cycle assessment and circularity indicators. Int. J. Life Cycle Assess. 26, 1937–1942. doi: 10.1007/s11367-021-01966-2
Ritcher, F. (2020). Chart: China Is the World's Manufacturing Superpower | Statista. Statista. Available online at: https://www.statista.com/chart/20858/top-10-countries-by-share-of-global-manufacturing-output/ (accessed January 12 2021).
Rockström, J., Steffen, W., Noone, K., Persson, Å., Chapin, I. I. I. F. S., et al. (2009). Planetary boundaries: exploring the safe operating space for humanity. Ecol. Soc. 14, 32. doi: 10.5751/ES-03180-140232
Roy, U., and Li, Y. (2014). “Sustainability assessment of the injection molding process and the effects of material selection, Vol. 4,” in 19th Design for Manufacturing and the Life Cycle Conference and 8th International Conference on Micro- and Nanosystems (Buffalo, NY: American Society of Mechanical Engineers), 1–10. doi: 10.1115/DETC2014-35205
Saad, M. H., Nazzal, M. A., and Darras, B. M. (2019). A general framework for sustainability assessment of manufacturing processes. Ecol. Indicat. 97, 211–224. doi: 10.1016/j.ecolind.2018.09.062
Saisana, M. (2014). “Environmental sustainability index,” in Encyclopedia of Quality of Life and Well-Being Research (Dordrecht: Springer Netherlands), 1926–1931. doi: 10.1007/978-94-007-0753-5_899
Sala, S., Ciuffo, B., and Nijkamp, P. (2015). A systemic framework for sustainability assessment. Ecol. Econ. 119, 314–325. doi: 10.1016/j.ecolecon.2015.09.015
Sala, S., Farioli, F., and Zamagni, A. (2013a). Life cycle sustainability assessment in the context of sustainability science progress (part 2). Int. J. Life Cycle Assess. 18, 1686–1697. doi: 10.1007/s11367-012-0509-5
Sala, S., Farioli, F., and Zamagni, A. (2013b). Progress in sustainability science: lessons learnt from current methodologies for sustainability assessment: part 1. Int. J. Life Cycle Assess. 18, 1653–1672. doi: 10.1007/s11367-012-0508-6
Samaroo, N., Koylass, N., Guo, M., and Ward, K. (2020). Achieving absolute sustainability across integrated industrial networks – a case study on the ammonia process. Green Chem. 22, 6547–6559. doi: 10.1039/D0GC02520H
Sartal, A., Bellas, R., Mejías, A. M., and García-Collado, A. (2020). The sustainable manufacturing concept, evolution and opportunities within Industry 4.0: A literature review. Adv. Mech. Eng. 12, 1687814020925232. doi: 10.1177/1687814020925232
Saxena, P., Stavropoulos, P., Kechagias, J., and Salonitis, K. (2020). Sustainability assessment for manufacturing operations. Energies 13, 2730. doi: 10.3390/en13112730
Schmidt, A., Poulsen, P. B., Andreasen, J., Floe, T., and Poulsen, K. E. (2004). “The working environment in LCA: A new approach,” in Guidelines from the Danish Environmental Agency, Vol. 72 (Denmark: Danish Environmental Protection Agency).
Schmidt, I., Meurer, M., Saling, P., Kicherer, A., Reuter, W., and Gensch, C. O. (2004). SEEbalance: managing sustainability of products and processes with the socioeco- efficiency analysis by BASF. Greener Manag. Int. 79–94. doi: 10.9774/GLEAF.3062.2004.sp.00007
Schrijvers, D., Hool, A., Blengini, G. A., Chen, W.-Q., Dewulf, J., Eggert, R., et al. (2020). A review of methods and data to determine raw material criticality. Resour. Conserv. Recycl. 155, 104617. doi: 10.1016/j.resconrec.2019.104617
Shade, S. A., and Sutherland, J. W. (2018). “Energy efficient or energy effective manufacturing?,” in Energy Efficient Manufacturing, eds J. W. Sutherland, D. A. Dornfeld, and B. S. Linke (Hoboken, NJ: John Wiley and Sons), 421–444. doi: 10.1002/9781119519904.ch16
Singh, N., Jain, S., and Sharma, P. (2016). Environmental benchmarking practices in Indian industries. Benchmarking Int. J. 23, 1132–1146. doi: 10.1108/BIJ-08-2014-0079
Singh, R., Joshi, S., and Mani, M. (2021). Transforming India's Built Environment: A 2050 Vision for Wellness and Resilience. Lawrence Berkeley National Laboratory. Available online at: https://escholarship.org/uc/item/81g7049b
Singh, R. K., Murty, H. R., Gupta, S. K., and Dikshit, A. K. (2012). An overview of sustainability assessment methodologies. Ecol. Indicat. 15, 281–299. doi: 10.1016/j.ecolind.2011.01.007
Singh, S., Olugu, E. U., and Fallahpour, A. (2014). Fuzzy-based sustainable manufacturing assessment model for SMEs. Clean Technol. Environ. Policy 16, 847–860. doi: 10.1007/s10098-013-0676-5
Skerlos, S. J. (2015). Promoting effectiveness in sustainable design. Proc. CIRP 29, 13–18. doi: 10.1016/j.procir.2015.02.080
Smullin, M. M., Haapala, K. R., Mani, M., and Morris, K. C. (2016). “Using industry focus groups and literature review to identify challenges in sustainable assessment theory and practice,” in ASME 2016 International Design Engineering Technical Conferences and Computers and Information in Engineering Conference (Charlotte, NC: American Society of Mechanical Engineers), V004T05A048. doi: 10.1115/DETC2016-60216
Sonnemann, G., Gemechu, E. D., Adibi, N., de Bruille, V., and Bulle, C. (2015). From a critical review to a conceptual framework for integrating the criticality of resources into Life Cycle Sustainability Assessment. J. Clean. Prod. 94, 20–34. doi: 10.1016/j.jclepro.2015.01.082
Stewart, R., Fantke, P., Bjørn, A., Owsianiak, M., Molin, C., Hauschild, M. Z., et al. (2018). Life cycle assessment in corporate sustainability reporting: Global, regional, sectoral, and company-level trends. Business Strat. Environ. 27, 1751–1764. doi: 10.1002/bse.2241
Stock, T., and Seliger, G. (2016). Opportunities of sustainable manufacturing in industry 4.0. Proc. CIRP 40, 536–541. doi: 10.1016/j.procir.2016.01.129
Stoycheva, S., Marchese, D., Paul, C., Padoan, S., Juhmani, A., and Linkov, I. (2018). Multi-criteria decision analysis framework for sustainable manufacturing in automotive industry. J. Clean. Prod. 187, 257–272. doi: 10.1016/j.jclepro.2018.03.133
Sudit, E. F. (1995). Productivity measurement in industrial operations. Eur. J. Operat. Res. 85, 435–453. doi: 10.1016/0377-2217(94)00312-Z
Sutherland, J. W., Richter, J. S., Hutchins, M. J., Dornfeld, D., Dzombak, R., Mangold, J., et al. (2016). The role of manufacturing in affecting the social dimension of sustainability. CIRP Ann. Manufactur. Technol. 65, 689–712. doi: 10.1016/j.cirp.2016.05.003
Swarr, T. E., Hunkeler, D., Klöpffer, W., Pesonen, H.-L., Ciroth, A., Brent, A. C., et al. (2011). Environmental life-cycle costing: a code of practice. Int. J. Life Cycle Assess. 16, 389–391. doi: 10.1007/s11367-011-0287-5
Tanzil, D., and Beloff, B. R. (2006). Assessing impacts: Overview on sustainability indicators and metrics. Environ. Qual. Manag. 15, 41–56. doi: 10.1002/tqem.20101
Tercero Espinoza, L., Schrijvers, D., Chen, W.-Q., Dewulf, J., Eggert, R., Goddin, J., et al. (2020). Greater circularity leads to lower criticality, and other links between criticality and the circular economy. Resour. Conserv. Recycl. 159, 104718. doi: 10.1016/j.resconrec.2020.104718
The Ellen MacArthur Foundation (2017). What Is a Circular Economy? Available online at: https://www.ellenmacarthurfoundation.org/circular-economy/concept (accessed Nov 02, 2021).
Trianni, A., Cagno, E., and Neri, A. (2017). Modelling barriers to the adoption of industrial sustainability measures. J. Clean. Prod. 168, 1482–1504. doi: 10.1016/j.jclepro,0.2017.07.244
UN Environment (2019). Global Environment Outlook – GEO-6: Healthy Planet, Healthy People. Nairobi: UN Environment Cambridge University Press. doi: 10.1017/9781108627146
UNEP. (2020). Guidelines for Social Life Cycle Assessment of Products and Organizations 2020, eds C. Benoît Norris, M. Traverso, S. Neugebauer, E. Ekener, T. Schaubroeck, S. Russo Garrido, M. Berger, S. Valdivia, A. Lehmann, M. Finkbeiner, and G. Arcese. Paris: United Nations Environment Programme (UNEP). Available online at: https://www.lifecycleinitiative.org/wp-content/uploads/2021/01/Guidelines-for-Social-Life-Cycle-Assessment-of-Products-and-Organizations-2020-22.1.21sml.pdf
US Dept of Commerce (2007). US Deptt. of Commerce. Available online at: https://legacy.trade.gov/green/sm-101-module.asp (accessed January 5, 2018).
US EPA (2021). Sources of Greenhouse Gas Emissions. Available online at: https://www.epa.gov/ghgemissions/sources-greenhouse-gas-emissions (accessed January 31, 2022).
US Government Printing Office (1980). The Global 2000 Report to the President: Entering the Twenty-First Century.
Vandenberg, L. N., Hauser, R., Marcus, M., Olea, N., and Welshons, W v. (2007). Human exposure to bisphenol A (BPA). Reprod. Toxicol. 24, 139–177. doi: 10.1016/j.reprotox.2007.07.010
Veleva, V., and Ellenbecker, M. (2001). Indicators of sustainable production: framework and methodology. J. Clean. Prod. 9, 519–549. doi: 10.1016/S0959-6526(01)00010-5
Vennemo, H., Aunan, K., Lindhjem, H., and Seip, M. (2009). Environmental Pollution in China: status and Trends. Rev. Environ. Econ. Policy 3, 209–230. doi: 10.1093/reep/rep009
Verones, F., Bare, J., Bulle, C., Frischknecht, R., Hauschild, M., Hellweg, S., et al. (2017). LCIA framework and cross-cutting issues guidance within the UNEP-SETAC life cycle initiative. J. Clean. Prod. 161, 957–967. doi: 10.1016/j.jclepro.2017.05.206
Vinante, C., Sacco, P., Orzes, G., and Borgianni, Y. (2021). Circular economy metrics: literature review and company-level classification framework. J. Clean. Prod. 288, 125090. doi: 10.1016/j.jclepro.2020.125090
Wackernagel, M., and Rees, W. (1998). Our Ecological Footprint: Reducing Human Impact on the Earth. Gabriola Island: New Society Publishers.
Walker, A. M., Vermeulen, W. J., v Simboli, A., and Raggi, A. (2021). Sustainability assessment in circular inter-firm networks: an integrated framework of industrial ecology and circular supply chain management approaches. J. Clean. Prod. 286, 125457. doi: 10.1016/j.jclepro.2020.125457
Wang, Z., Wei, X., Yang, J., Suo, J., Chen, J., Liu, X., et al. (2016). Chronic exposure to aluminum and risk of Alzheimer's disease: a meta-analysis. Neurosci. Lett. 610, 200–206. doi: 10.1016/j.neulet.2015.11.014
Wei, T., and Liu, Y. (2017). Estimation of global rebound effect caused by energy efficiency improvement. Energy Econ. 66, 27–34. doi: 10.1016/j.eneco.2017.05.030
Wong, K. H., and Durrani, T. S. (2017). Exposures to endocrine disrupting chemicals in consumer products—a guide for pediatricians. Curr. Probl. Pediatr. Adolesc. Health Care 47, 107–118. doi: 10.1016/j.cppeds.2017.04.002
Yang, M., Vladimirova, D., Rana, P., and Evans, S. (2014). Sustainable value analysis tool for value creation. Asian J. Manag. Sci. Applic. 1, 312–332. doi: 10.1504/AJMSA.2014.070649
Zhang, D., Hao, M., and Morse, S. (2020). Is environmental sustainability taking a backseat in china after COVID-19? The perspective of business managers. Sustainability 12, 10369. doi: 10.3390/su122410369
Zhang, H., Calvo-Amodio, J., and Haapala, K. R. (2013). A conceptual model for assisting sustainable manufacturing through system dynamics. J. Manufact. Syst. 32, 543–549. doi: 10.1016/j.jmsy.2013.05.007
Zhang, Q., Wiedmann, T., Fang, K., Song, J., He, J., and Chen, X. (2022). Bridging planetary boundaries and spatial heterogeneity in a hybrid approach: a focus on Chinese provinces and industries. Sci. Total Environ. 804, 150179. doi: 10.1016/j.scitotenv.2021.150179
Keywords: effectiveness & efficiency (E&E), sustainability assessment (SA), circularity, manufacturing, design decisions, systems approach
Citation: Kumar M and Mani M (2022) Sustainability Assessment in Manufacturing for Effectiveness: Challenges and Opportunities. Front. Sustain. 3:837016. doi: 10.3389/frsus.2022.837016
Received: 16 December 2021; Accepted: 25 February 2022;
Published: 29 March 2022.
Edited by:
Gilberto Santos, Instituto Politécnico do Cávado e do Ave (IPCA), PortugalReviewed by:
Maria Angela Butturi, University of Modena and Reggio Emilia, ItalyJolita Kruopienė, Kaunas University of Technology, Lithuania
Copyright © 2022 Kumar and Mani. This is an open-access article distributed under the terms of the Creative Commons Attribution License (CC BY). The use, distribution or reproduction in other forums is permitted, provided the original author(s) and the copyright owner(s) are credited and that the original publication in this journal is cited, in accordance with accepted academic practice. No use, distribution or reproduction is permitted which does not comply with these terms.
*Correspondence: Manish Kumar, bWFuaXNoayYjeDAwMDQwO2lpc2MuYWMuaW4=