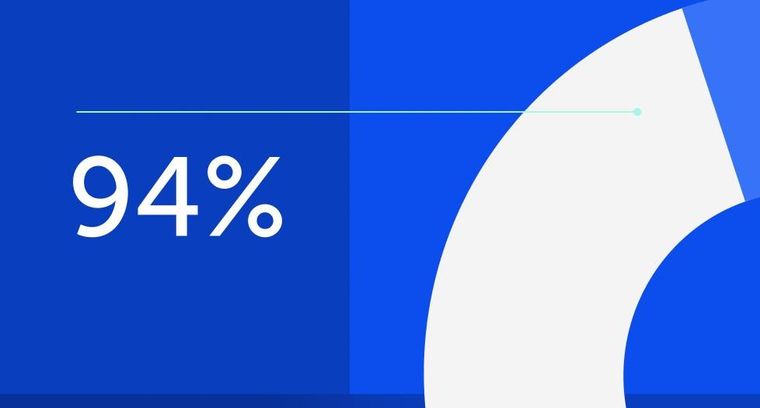
94% of researchers rate our articles as excellent or good
Learn more about the work of our research integrity team to safeguard the quality of each article we publish.
Find out more
BRIEF RESEARCH REPORT article
Front. Sustain., 05 December 2022
Sec. Quantitative Sustainability Assessment
Volume 3 - 2022 | https://doi.org/10.3389/frsus.2022.1060130
Introduction: Going a step further than quantifying environmental impacts, establishing the environmental and energy payback times of a wind turbine can significantly impact the planning of a wind farm. This study applies the Life Cycle Assessment methodology to a wind turbine and verifies its environmental and energy payback times.
Methods: The Life Cycle Assessment was developed with the SimaPro software, using the Ecoinvent database and the IPCC 2013 GWP 100y and Cumulative Energy Demand environmental impact assessment methods. The Life Cycle Assessment considered the extraction of raw material, production of parts and pieces, transportation, assembly, use, and decommissioning. Besides the material composition of the wind turbine, meteorological data was also utilized to calculate wind electricity production in Northeast Brazil. The environmental analysis and data on energy production were used to calculate the time required to recoup the energy and emissions due to wind electricity compared to the emissions of the electricity grid.
Results: The emission factor of wind electricity was 0.0083 kg CO2-eq/kWh, and the emissions associated with consumption of electricity from the Brazilian Electricity mix was 0.227 kg CO2-eq/kWh. Consideration of the energy consumed for the manufacture of the wind turbine yielded an energy payback of 0.494 years, and greenhouse gas accountancy led to a payback of 0.755 years.
Discussion: The results demonstrate that the payback periods are much lower than the lifetime of the wind turbine, highlighting the important role in addressing climate change and energy savings. The combination of Life Cycle Assessment and energy and environmental paybacks can be used to measure sustainability and deploy wind energy projects in locations with the shorter payback times.
The generation of electricity to supply human demands has always been one of the priorities of modern society. However, utilizing natural resources to meet these energy demands has not been carried out sustainably throughout the years (Pereira et al., 2022). As new energy conversion and supply technologies are developed, better use of resources and higher efficiency in electricity generation can be achieved. The efficient use of natural resources is aligned with the sustainability concept, aiming at resource consumption that considers the interests of future generations (Uysal et al., 2022).
In Brazil, annual National Energy Balances are published with data referring to the consumption and generation of electricity from different sources. According to the 2020 report (Energy Research Company, 2020), there was an increase of 1.4% in the share of electricity compared to the previous year, with a significant contribution of renewable sources. The contribution of renewable energy sources increased by 2.8%, which results in a 46.1% share of renewables within the Brazilian national electricity matrix. Wind energy production presented a 15.5% increase when comparing 2019 and 2020 (Energy Research Company, 2020), which benefits the environment, as it can displace the production of electricity from other sources with higher environmental loads.
Wind energy can be employed in low-carbon schemes and to decarbonize the electricity supply, and should be a leading technology in this transformation context due to its high performance, high availability, and fast decreasing costs (Simón-Martín et al., 2019). Even though wind turbines do not generate pollutants during operation, there are significant impacts on the environment during the production phase (manufacture of parts), construction, and decommissioning (Schreiber et al., 2019). In addition, wind turbines could influence the local microclimate (Stergiannis et al., 2021).
Reducing greenhouse gas (GHG) emissions is an essential factor for a country that wants to generate energy with less pollution. Brazil is one of the countries that have a significant percentage of low-carbon (“clean”) power in its energy matrix (Energy Research Company, 2020), which contributes to the achievement of goals established in international agreements, such as the Paris Agreement signed in 2015. In addition, Brazil presents national plans and public policies to reduce GHG emissions and mitigate climate change - following the premises established in the Paris Agreement.
One of the ways to reduce these environmental impacts is knowing the components required for the manufacture and construction of wind turbines and formulating an action plan for final disposal once the lifetime is over. This knowledge can assist in selecting materials and methods that decrease overall impacts. In this context, the Life Cycle Assessment (LCA) methodology can be employed, quantifying the environmental impacts and liabilities of a product, process, or activity (Ciacci and Passarini, 2020).
Although there have been studies developed to verify the environmental impacts caused by wind turbines in different locations (of which the most recent are Wang and Teah, 2017; Schreiber et al., 2019; Li et al., 2021; Teffera et al., 2021; Garcia-Teruel et al., 2022), different turbine configurations and different results were obtained. The general conclusion was that there are significant environmental impacts embedded in the construction (and manufacturing) phase of the parts and decommissioning.
Kaldellis and Apostolou (2017) reviewed studies published since 2000 and verified that most present energy payback time (EPBT, time to produce the amount of energy required for production and installation) under 1 year for onshore and offshore wind farms. Although annual mean energy production values are obtained, it is not clear whether all studies reviewed employed mean annual wind speed data. Overall, offshore facilities usually presented lower EPBTs, mainly due to higher wind speeds that enable higher generation (kWh/year).
Marimuthu and Kirubakaran (2013) calculated the environmental and energy paybacks of photovoltaic and wind systems in India, considering annual wind speed values. For a 1.65 MW turbine, EPBT was 1.12 years and GHG payback time (GPBT, period during which the system must operate to offset the emissions embedded in its production) was 50 days. Haapala and Prempreeda (2014) analyzed two 2 MW wind turbines using LCA and EPBT, and obtained that environmental impacts were concentrated in the manufacturing stage, accounting for 78% of impacts. The EPBT were 5.2 and 6.4 months, in which annual energy production was obtained via an estimated annual capacity factor. Dammeier et al. (2019) used hourly wind speed data between 1979 and 2013 and showed that the GPBT of wind turbines in northwestern Europe varied between 1.8 and 22.5 months.
Estimates for the GBPT of onshore wind projects employed annual wind speed averages, and ranged from 6 months to 2 years, but construction on forested peatlands could approach 6 years, which is still lower than the average lifetime of wind systems (Thomson and Harrison, 2015a). However, when expected decreases in grid emissions are considered, as mentioned by Thomson and Harrison (2015a,b), longer payback times are expected but should be achieved within the wind farm lifetime. It is expected that wind farms constructed on the forested peatlands of Scotland after 2022 might not achieve payback, and more research is required to verify how to minimize GHG emissions associated with construction at these locations.
The avoided emissions achieved by the operation of wind energy systems are usually obtained by comparison with fossil fuel-generated power plants (which could result in an overestimation) or with the electricity mix supplied by the national electric grid. However, wind actually displaces only the generators operating on the margin. Thomson et al. (2017) presented a methodology to isolate the marginal emissions displacement of wind power from historical empirical data, taking into account the impact on the operating efficiency of the conventional power plants operating in the UK. It was verified that wind power was almost as technically effective as demand-side reductions at decreasing GHG emissions from power generation.
LCA was emphasized by Stavridou et al. (2020) as crucial when assessing the actual contribution of wind energy systems to environmental protection, with the most important parameters being EPBT and GPBT. Two onshore wind turbine towers, a lattice tower and a tubular tower, were investigated and presented EPBTs of 4 and 5 months using the same capacity factors as Haapala and Prempreeda (2014). GHG emissions could be further reduced by saving material from the foundation and tower, which are the most energy-consuming components of the wind structure.
Although the aforementioned studies have considered EPBT and GPBT, few have considered both parameters simultaneously. Most studies have employed annual average wind speeds in their calculations, and none have been carried out in Brazil to date with the combination LCA + electricity forecast with daily wind speed data + EPBT + GPBT.
Daily wind speed data has been employed in recent studies, such as in wind power forecasting in Korea (Kim et al., 2022), Spain (Collados-Lara et al., 2022), and Brazil (Andrade et al., 2021). The study presented herein uses daily wind speed data to forecast wind electricity production and goes a step further in a different direction by combining the results with LCA, to provide environmental paybacks for a specific location.
This is the first study to employ daily wind speeds instead of annual values to calculate the environmental payback after a LCA is developed. Besides GHG accounting, the cumulative energy demand (CED) is employed as an additional environmental impact assessment method within LCA, to quantify the energy embedded in the wind turbine.
The objective of the study presented herein is to quantify the energy and GHG payback times for a wind turbine installed in Northeast Brazil. With these indicators, it is possible to quantify the potential benefits associated with the installation of renewable technologies, such as wind, in reaching specific environmental goals. It must be highlighted that although renewable energy is considered low-carbon, meeting specific emission targets depends on the local electricity mix that will be displaced. Moreover, the energy production is also affected by climate parameters and this could help decide on the most strategic location for the installation of a specific energy project, as achieving maximum or optimal energy production means a lower environmental payback time and higher environmental benefits. To this end, an LCA is developed considering the GHG emissions and cumulative energy demand associated with a wind turbine. Historical meteorological data and technical parameters are used to calculate its energy production. GHG emissions and CED are environmental indicators that evaluate the sustainability and environmental performance of renewable energy systems but have not been considered simultaneously in an LCA.
LCA is a consolidated, validated methodology for quantifying potential environmental impacts associated with a product (process, service, or activity) throughout its life cycle (Hauschild et al., 2018). An LCA can encompass the extraction of raw materials, manufacture, processing, distribution, transportation, use, maintenance, and final disposal. LCA is standardized by the International Organization for Standardization (ISO 14040 - International Organization for Standardization, 2006; ISO 14044 - International Organization for Standardization., 2006) and consists of four main phases: definition of the objective and scope (definition of the functional unit – to which all inputs and outputs are related to –, its life cycle, and system boundaries), inventory analysis (list of material and energy flows associated with the functional unit), impact assessment (selection of environmental impact assessment method to quantify environmental loads), and interpretation. Industries often use LCA to improve processes, increase efficiency and reduce costs.
The LCA is developed herein with the SimaPro software 9.1.1.1 (SimaPro, 2020), with the Ecoinvent (2019) database version 3.6. Two environmental impact assessment methods are employed: i) IPCC 2013 GWP 100y (IPCC-Intergovernmental Panel on Climate Change, 2014) that groups GHG emissions in a common metric, CO2-eq, throughout a horizon of 100 years, and ii) cumulative energy demand (CED) version 1.11 (also called “primary energy consumption”), which has been one of the critical indicators being addressed in LCAs (Frischknecht et al., 2015). The CED covers the total amount of primary energy necessary to produce, use and dispose of a product, based on higher heating values (HHV) and encompasses non-renewable (fossil, nuclear, and biomass) and renewable (biomass, wind, solar, geothermal, and hydro) energy. Table 1 shows the material composition of the wind turbine.
The wind turbine analyzed herein is a Gamesa onshore wind turbine, G8X model, with 2-MW rated power, 80 m rotor blade, and 5027 m2 sweep area (Gamesa, 2011). The city of Patos, Northeast Brazil, is the installation site. The environmental analysis encompassed the production of each component, transportation to the installation site, installation, start-up, maintenance, and final decommissioning, with waste disposal.
Regarding transportation, for the foundation, no transportation was considered. For the rotor, the tower, and the nacelle, mean distances were considered for road transportation: 700 km for the rotor, 810 km for the tower, and 2,860 km for the nacelle.
For the final disposal of the wind turbine, it is considered that all iron and steel are recycled with 10% losses, copper is recycled with 5% losses, while fiberglass, PVC, other plastics, and rubber are landfilled. For metals recycling, the mean distance in road transport was 20 km, while landfilled materials were transported for 82.5 km.
Removal of the foundation from the site would result in using heavy equipment and machinery, which could cause even more soil contamination and GHG emissions. Therefore, it was considered that the foundation was left behind, covered with 30 centimeters of organic soil.
Daily wind speed data for the Patos location were obtained from its climatological station (1975-2020) (National Institute of Meteorology - NIMET, 2020). Wind speed data were acquired at a reference height of 10 meters, as established by Brazilian standards NBR 6123 (Brazilian Association of Technical Standards, 1990). The wind speed must be recalculated considering the height of the hub, according to Equation 1 (Stiebler, 2008).
v2 is the wind speed at height z2, which is the height of the hub; v1 is the average wind speed for a specific location; z1 is the reference height, at which speed v1 was measured; and z0 is the length of the roughness, characteristic of each site. Herein z2 = 80 m, z0 = 0.02 m (CRESESB, 2017), and z1 = 10 m.
The power produced by the turbine is calculated with Equation 2 (Stiebler, 2008).
A is the sweep area (5,027 m2), ρ is the specific mass of air (1.2 kg/m3), and cp is the power coefficient (0.5). The method presented by Andrade et al. (2021) was employed to calculate the energy production from daily wind speed data.
The energy payback (EPBT) is the time that a system has to operate to generate the same amount of energy that was spent in its manufacture, assembly, installation, and final disposal. The time required to reset this energy balance is calculated by Equation 3.
Eused is the energy used to manufacture the system, and Esaved is the energy generated over a year.
In addition to EPBT, it is possible to calculate the time required for the system to mitigate the GHG emissions from the wind turbine production, the GHG payback time (GPBT), given by Equation 4.
EmissionsLCA is the total GHG emissions for the manufacture, installation, operation and disposal of the wind turbine (obtained via LCA), and AnnualEmissionsavoided is the annual avoided value of GHG emissions, calculated as the difference between the emissions that would be generated by consuming this electricity from the grid and the GHG emissions from the turbine. Turbineproduction is the amount of energy produced in kWh. EFee refers to the emission factor of the electricity consumed in the country (kg CO2-eq/kWh), and EFwind refers to the emission factor of the electricity generated by the wind turbine (kg CO2-eq/kWh). AnnualEmissionsLCA is the value of EmissionsLCA divided by the system's lifetime, considered 20 years (Wang and Teah, 2017).
The methodology presented by Carvalho (Carvalho and Delgado, 2017) was followed to calculate the GHG emissions associated with the consumption of 1 kWh of electricity from the electricity grid in Brazil (EF). Data from the National Electric System Operator (2020) was obtained. The first, fifteenth, and thirtieth days of the month were used to calculate monthly mean values, leading to the mean annual composition of the electricity mix in 2020: hydroelectric 66.67%, natural gas 9.28%, wind 9.15%, sugarcane bagasse 8.25%, nuclear 2.79%, coal 1.62%, fuel oil 1.55% and solar 0.69%.
Table 2 shows the GHG emissions associated with each component of the wind turbine system, using the information from Table 1 and the disposal process considered for each element. The energy consumed in each phase is also shown in Table 2.
Regarding manufacture, the sub-components with the highest GHG emissions are the three blades, the footing, and the three sections of the tower.
The footing is constituted of 700 t of concrete and 25 t of iron. According to Gomes et al. (2019), concrete is a composition of mineral and chemical additives, water, and cement and is responsible for a considerable amount of GHG emissions. Approximately 4% of global GHG emissions can be attributed to cement, according to Andrew (2018). For the three sections of the tower, also constituted of steel, there are significant embedded emissions, as reported by the Brazil Steel Institute (2010): ~95% of the energy employed in its production originates from solid fuels (mainly coal).
Although the nacelle presented the longest distance for transportation, this component did not present the highest GHG emissions.
Regarding decommissioning, the positive values (blades, nose, and cover) shown in Table 2 refer to the landfill process. The negative values refer to the recycling processes of sub-components, which avoids producing new material despite consuming energy.
The CED column in Table 2 is better represented in Figure 1. The rotor presents the highest share of embedded energy. This result is similar to Martínez et al. (2009), where the authors explain that the rotor has the most significant impact due to the manufacture of the blades. Alsaleh (2017) reported that the tower had the highest energy share while the rotor had the lowest, but this can be explained by the separation adopted by the authors for the main parts of the wind turbine, which was different from the separation adopted herein.
After recalculating the corrected values for daily wind speed for 80 m height using Equation 1, and calculating the daily power produced by the wind turbine with Equation 2, the monthly and annual energy produced by the wind turbine are obtained. Figure 2 shows the monthly mean wind turbine power for an operational year.
The annual production of the wind turbine is 2,576.81 MWh. Consideration of the energy consumed for the manufacture of the wind turbine (Table 2, 1,272.17 MWh) and Equation 3 yields that EPBT = 0.494 years, which is equivalent to ~180 days, or 2.47% of the wind turbine's lifetime.
Regarding the environmental payback, Annual EmissionsLCA = 21,284 kg CO2-eq/year. Thus, EFwind = 0.0083 kg CO2-eq/kWh. Following the methodology of Carvalho and Delgado (2017), the emission factor of electricity consumed from the Brazilian grid is 0.227 kg CO2-eq/kWh. Thus, Equation 5 provides Annual Emissionsavoided = 563,653 kg CO2-eq over 1 year of operation. Finally, GPT = 0.755 years, which is equivalent to 276 days, approximately.
Compared with existing studies, the order of magnitude of the paybacks is similar. Rankine et al. (2006) evaluated a type of wind turbine called SWIFT (1.5 kW and hub height 10 m). These authors assessed different values of energy generated (1-2-3-4 MW) and obtained EPBT = 4.20-2.10-1.40-1.05 years and GPBT = 3.28-1.64-1.09-0.82 years. These times are longer than those obtained herein. Still, the authors argue that microgeneration can achieve lower carbon emissions by replacing electricity from the grid with that produced by small generators in a home (fewer emissions associated with the construction and maintenance of a national electricity grid).
Comparing the EPBT and GPBT results obtained herein with those presented in scientific literature, most are under 1 year, and all studies considered a 20-year lifetime. Differences in values could be due to different wind speeds, the electricity matrix of the country where the turbine is installed, methods used in the analysis, and individual characteristics of the turbines. One point that must be emphasized is that there are other positive effects associated with the existence and continued use of wind electricity, and often overlooked. The primary benefit is that wind electricity (as other renewable electricity) steadily reduce reliance on mainstream power generation (fossil-fuel based).
When placing the results obtained herein within a broader context, Figure 3 shows the installed capacity (MW) of wind electricity generation, in Brazil and Northeast Brazil, for 2018 and 2019. It is observed that Northeast Brazil holds the majority of installed capacity, with increasing penetration, demonstrating that the country is moving toward compliance with the Paris Agreement and to the diversification of the electricity matrix, consequently reducing the levels of GHG emissions.
Figure 3. Installed capacity (MW) of wind power generation in Brazil and the Northeast region. Source: National Electric System Operator (2020).
The environmental benefits of introducing renewable energy generation in the electricity mix has already been shown for Brazil regarding solar photovoltaic electricity (Carvalho and Delgado, 2017; Schultz and Carvalho, 2022) – and wind electricity has the potential to realize further benefits. During the UN Climate Change Convention in 2015, Brazil voluntarily committed to reducing carbon emissions by 2020 (Brazilian Ministry of the Environment (ME)., 2022). The National Energy Balance (Energy Research Company, 2020) shows that the installed capacity for wind generation in 2020 expanded by 6.9% compared to 2019. The implementation of renewable energy schemes has a strong influence on this decrease in emissions. This can be demonstrated by comparing the carbon emissions required to produce 1 MWh in 2015–2020 (according to the reports presented by the Energy Research Company): there was a 34% reduction in emissions, from 137 kg CO2/MWh to 90 kg CO2/MWh. This represents an increase from 12,210 GWh to 55,986 GWh of energy generated by wind sources. Furthermore, there was also a reduction in the share of non-renewable sources from 60.6 to 53.9% for the same period.
According to the Brazilian Business Council for Sustainable Development (BBCSD, 2020), the 2015 Paris Agreement established that the signatory countries were committed to reducing global warming, which is accomplished directly through the actions implemented by each government. Of the 38 participating companies, 21 are Brazilian or have information on the levels of emissions in Brazil (BBCSD, 2020) – this evidences a clear understanding that the improvement of services and actions have a direct impact on the environment, and electricity generation is one of the main areas that have a significant influence on this factor.
Regarding the Paris Agreement, Brazil established the National Determined Contribution (NDC) in 2016, a national commitment that sets targets for reducing GHG emissions by 37% by 2025, compared to the levels emitted in 2005 (AdaptaCLIMA, 2017). In addition, the reduction could reach 50% by 2030, also compared to the levels emitted in 2005, which was officially assumed as an objective by the Brazilian Ministry of Foreign Affairs (MFA) (2021) and the official document sent to the public registry of NDC communications of the signatory countries of the Paris Agreement (National Determined Contribution, 2016, 2022). Brazil also set the “objective of reaching climate neutrality in 2050” (Gomes and Rodrigues, 2020; National Determined Contribution, 2022).
Also, in the official National Determined Contribution (2016) published, Brazil intends to increase the share of renewable sources in the energy matrix up to 45% by 2030, including the expansion of renewable sources (other than hydropower) from 28 to 30% for 2030. The domestic use of energy from non-fossil sources is also expected to increase by at least 23%, employing wind, biomass, and solar.
In 2009, the country also presented the National Policy on Climate Change, with plans and actions to mitigate or adapt to climate change in accordance with the 2015 Convention. Some of the measures include deforestation control, energy expansion, emission reduction in the steel industry, low emissions in agriculture and the manufacturing industry, mitigation of GHG in the transport and mobility sector, and mitigation of GHG in the health sector (Brazilian Ministry of Science, 2016).
With all these plans and agreements signed by Brazil, the country is moving toward better energy management in reducing emissions and implementing public policies to encourage using renewable sources to achieve these goals.
Regarding the GHG emissions associated with thermal generators on standby for backup, it was considered herein that all energy generation technologies (e.g., coal, gas, nuclear, wind, solar) must be supported by other generators with similar backup requirements. Therefore, the associated GHG emissions are minimal (National Renewable Energy Laboratory – NREL, 2013; Broeer et al., 2018).
Wind electricity, characterized by a lower amount of embedded energy and higher conversion efficiency, is becoming increasingly popular. At the same time, different options of energy conversion and supply systems are being developed, motivating further research on the energy payback and environmental performances of each technology. Environmental assessments of this kind are typically developed after an in-depth analysis of wind resource potential, which should investigate the short-term penetration of regional wind power (such as in Nedaei et al., 2018). Green tags can further motivate the reduction of GHG emissions. These incentives are in the spotlight because they enhance the viability of renewable energy projects, being excellent alternatives for investments in wind energy projects, which can be sold, bartered, or traded (Nedaei et al., 2020).
Finally, although it is well-known that wind electricity is a low-carbon option, considerations on the installation of wind power plants should include the production of electricity throughout the lifetime of the project. EPBT and GPBT can be affected by technology type, efficiency, wind speed, and the electricity mix (or the portion of materials used in electricity production).Given the importance of climate change, A case point is made herein to adopt GPBT to complement viability assessments, emphasizing the time required to recoup the emissions due to wind electricity compared to the emissions of the electricity grid.
Although traditional energy policies have employed economic paybacks to assist in the decision-making process, environmental paybacks such as EPBT and GPBT can provide a life-cycle approach and are valuable to promote discussions on the improvement of policies. Based on the results herein presented, different locations can be tested for the installation of a wind farm and ranked on the basis of GPBT. The ranking results suggest the locations where (new, improved) policies could be used to provide environmental benefits. The selection of locations with shorter EPBT and GPBT will realize more environmental benefits compared to consuming electricity directly from the grid.
The environmental performance of wind electricity is usually determined by a Life Cycle Assessment (LCA). The environmental impact depends on the amount and type of materials and energy employed to build and maintain the wind turbine, the electricity produced over its lifetime, and also on the local electricity that is being displaced. This is the first study to report energy payback time (EPBT) and GHG payback time (GPBT) for a Northeast Brazil location, combining LCA and electricity forecast with daily wind speed data.
Consideration of the energy consumed for the manufacture of the wind turbine yielded EPBT = 0.494 years (~180 days, or 2.47% of the wind turbine's lifetime). The emission factor of wind electricity was 0.0083 kg CO2-eq/kWh, and the emissions associated with consumption of electricity from the Brazilian Electricity mix was 0.227 kg CO2-eq/kWh. The GPBT was 0.755 years (equivalent to 276 days). The results demonstrate that the payback periods are much lower than the lifetime of the wind turbine, the important role of EPBT and GPBT in addressing climate change and energy savings.
The combination of LCA, EPBT and GPBT can be used to measure sustainability and deploy wind energy projects in locations with the shorter EPBT and GPBT. Despite the increasing research on energy and environmental aspects associated with wind power in Brazil, there is a lack of studies on EPBT and GPBT.
It is vital to extend studies on wind energy, such as expanding data collection for more cities in northeastern Brazil. The combination of LCA, EPBT and GPBT can provide location-specific predictions of the environmental impacts for wind turbines. In this way, it would be possible to analyze and actually quantify the potential to mitigate GHG emissions and its wind electricity generation capacity. Further refining of wind speed data, employing hourly speeds, could be tested to verify the potential improvement in the precision of annual energy production values, leading to more accurate paybacks.
The original contributions presented in the study are included in the article, further inquiries can be directed to the corresponding author.
All authors listed have made a substantial, direct, and intellectual contribution to the work and approved it for publication.
The authors wish to acknowledge the support of the National Council for Scientific and Technological Development (Brazil), Productivity Grant No. 309452/2021-0, and the Scientific Initiation Scholarship (PIBIC) within the Federal University of Paraíba (UFPB).
The authors declare that the research was conducted in the absence of any commercial or financial relationships that could be construed as a potential conflict of interest.
All claims expressed in this article are solely those of the authors and do not necessarily represent those of their affiliated organizations, or those of the publisher, the editors and the reviewers. Any product that may be evaluated in this article, or claim that may be made by its manufacturer, is not guaranteed or endorsed by the publisher.
AdaptaCLIMA. (2017). International Agreements and Public Policies. Available online at: http://adaptaclima.mma.gov.br/acordos-internacionais-e-politicas-publicas (accessed November 10, 2022).
Alsaleh, A. (2017). Life cycle assessment of greenhouse gas emissions, traditional air pollutants, water depletion, and cumulative energy demand from 2-MW wind turbines in Texas. (PhD thesis). University of Texas, Arlington, Tx, United States.
Andrade, A. R., Melo, V. F. M. B., Lucena, D. B., and Abrahão, R. (2021). Wind speed trends and the potential of electricity generation at new wind power plants in Northeast Brazil. J. Braz. Soc. Mech. Sci. Eng. 43, 1–11. doi: 10.1007/s40430-021-02911-y
Andrew, R. M. (2018). Global CO2 emissions from cement production. Earth Syst. Sci. Data 10, 195–217. doi: 10.5194/essd-10-195-2018
BBCSD. (2020). The Paris Agreement and Sustainable Development. Available online at: https://cebds.org/o-acordo-de-paris-e-o-desenvolvimento-sustentavel/#.XrmUmmhKhPZ (accessed November 10, 2022).
Brazil Steel Institute. (2010). Emissions of Greenhouse Gases in Industrial Processes – Production of Metals: Iron and Steel. 2nd Brazilian Inventory of Anthropogenic Emissions and Removals of Greenhouse Gases. Brasilia: MCTI (2010). p. 42. Available online at: https://cetesb.sp.gov.br/inventario-gee-sp/wp-content/uploads/sites/34/2014/04/brasil_mcti_ferro_aco.pdf (accessed November 24, 2022).
Brazilian Association of Technical Standards. (1990). Wind Forces in Buildings. Rio de Janeiro: BATS. Available online at: https://www.abntcatalogo.com.br/grd.aspx (accessed November 24, 2022).
Brazilian Ministry of Foreign Affairs (MFA) (2021). Presentation of Brazil's Nationally Determined Contribution under the Paris Agreement. Available online at: https://www.gov.br/mre/pt-br/canais_atendimento/imprensa/notas-a-imprensa/2020/apresentacao-da-contribuicao-nacionalmente-determinada-do-brasil-perante-o-acordo-de-paris (accessed November 10, 2022).
Brazilian Ministry of Science Technology Innovation (MCTI).. (2016). 3rd National Communication of Brazil to the Convention - United Nations Framework on Climate Change. Executive Summary. Available online at: http://www.ccst.inpe.br/publicacao/terceira-comunicacao-nacional-do-brasil-a-convencao-quadro-das-nacoes-unidas-sobre-mudanca-do-clima-portugues/ (accessed November 10, 2022).
Brazilian Ministry of the Environment (ME). (2022). Brazil Close to meeting CO2 Reduction Target in 2020. Available online at: https://www.gov.br/mma/pt-br/noticias/brasil-perto-de-cumprir-meta-de-reducao-de-co2-em-2020 (accessed November 10, 2022).
Broeer, T., Tuffner, F. K., Franca, A., and Djilali, N. (2018). A demand response system for wind power integration: greenhouse gas mitigation and reduction of generator cycling. CSEE J. Power Energy Syst. 4, 121–129. doi: 10.17775/CSEEJPES.2018.00500
Carvalho, M., and Delgado, D. (2017). Potential of photovoltaic solar energy to reduce the carbon footprint of the Brazilian electricity matrix. LALCA 1, 64–85. doi: 10.18225/lalca.v1i1.3779
Ciacci, L., and Passarini, F. (2020). Life cycle assessment (LCA) of environmental and energy systems. Energies 13, 5892. doi: 10.3390/en13225892
Collados-Lara, A. J., Baena-Ruiz, L., Pulido-Velazquez, D., and Pardo-Igúzquiza, E. (2022). Data-driven mapping of hourly wind speed and its potential energy resources: a sensitivity analysis. Renew. Energy 199, 87–102. doi: 10.1016/j.renene.2022.08.109
CRESESB. (2017). Wind Atlas of the State of Paraíba: 2017. Available online at: http://www.cresesb.cepel.br/index.php?link=/atlas_eolico_brasil/atlas.htm (accessed November 10, 2022).
Dammeier, L. C., Loriaux, J. M., Steinmann, Z. J., Smits, D. A., Wijnant, I. L., van den Hurk, B., et al. (2019). Space, time, and size dependencies of greenhouse gas payback times of wind turbines in Northwestern Europe. Environ. Sci. Technol. 53, 9289–9297. doi: 10.1021/acs.est.9b01030
Ecoinvent. (2019). The Ecoinvent Database 3.5. Available online at: http://www.ecoinvent.org/ (accessed November 10, 2022).
Energy Research Company. (2020). National Energy Balance 2020: Summary Report/Base Year 2019. Available online at: http://www.epe.gov.br/pt/publicacoes-dados-abertos/publicacoes/balanco-energetico-nacional-2020 (accessed November 10, 2022).
Frischknecht, R., Wyss, F., Knöpfel, S. B., Lützkendorf, T., and Balouktsi, M. (2015). Cumulative energy demand in LCA: the energy harvested approach. Int. J. Life Cycle Assess. 20, 957–969. doi: 10.1007/s11367-015-0897-4
Gamesa. (2011). GAMESA G9X-2.0MW - Technological Evolution. Available online at: https://www.ledsjovind.se/fjaras/catalogo-g9x-20-mw-eng.pdf (accessed November, 10 2022).
Garcia-Teruel, A., Rinaldi, G., Thies, P. R., Johanning, L., and Jeffrey, H. (2022). Life cycle assessment of floating offshore wind farms: an evaluation of operation and maintenance. Appl. Energy 307, 118067. doi: 10.1016/j.apenergy.2021.118067
Gomes, K. C., Carvalho, M., Diniz, D. D. P., Abrantes, R. D. C. C., Branco, M. A., and Carvalho Junior, P. R. O. D. (2019). Carbon emissions associated with two types of foundations: CP-II Portland cement-based composite vs. geopolymer concrete. Matéria (Rio de Janeiro) 24, e-12525. doi: 10.1590/s1517-707620190004.0850
Gomes, P. H., and Rodrigues, M. (2020). Paris Agreement: Brazil predicts 'zero emissions' by 2060 and Wants US$ 10 Billion Annually From Rich Countries to Bring Forward Date. Available online at: https://g1.globo.com/politica/noticia/2020/12/08/acordo-de-paris-brasil-preve-neutralidade-de-emissoes-ate-2060-e-quer-us-10-bilhoes-anuais-para-antecipar-data.ghtml (accessed November 10, 2022).
Haapala, K. R., and Prempreeda, P. (2014). Comparative life cycle assessment of 2.0 MW wind turbines. Int. J. Sustain. Manuf. 3, 170–185. doi: 10.1504/IJSM.2014.062496
IPCC-Intergovernmental Panel on Climate Change. (2014). Revised Supplementary Methods and Good Practice Guidance Arising from the Kyoto Protocol. Available online at: http://www.ipcc-nggip.iges.or.jp/public/kpsg/ (accessed November 10, 2022).
ISO 14040 - International Organization for Standardization. (2006). Environmental Management - Life Cycle Assessment - Principles and Framework. Geneva: ISO. Available online at: https://www.iso.org/standard/37456.html (accessed November 24, 2022).
ISO 14044 - International Organization for Standardization. (2006). Environmental Management - Life Cycle Assessment - Requirements and Guidelines. Geneva: ISO. Available online at: https://www.iso.org/standard/38498.html (accessed November 24, 2022).
Kaldellis, J. K., and Apostolou, D. (2017). Life cycle energy and carbon footprint of offshore wind energy. Comparison with onshore counterpart. Renew. Energy 108, 72–84. doi: 10.1016/j.renene.2017.02.039
Kim, J., Afzal, A., Kim, H. G., Dinh, C. T., and Park, S. G. (2022). Wind power forecasting based on hourly wind speed data in South Korea using machine learning algorithms. J. Mech. Sci. Technol. 36, 1–7. doi: 10.1007/s12206-022-1125-3
Li, Q., Duan, H., Xie, M., Kang, P., Ma, Y., Zhong, R., et al. (2021). Life cycle assessment and life cycle cost analysis of a 40 MW wind farm with consideration of the infrastructure. Renew. Sustain. Energy Rev. 138, 110499. doi: 10.1016/j.rser.2020.110499
Marimuthu, C., and Kirubakaran, V. (2013). Carbon payback period for solar and wind energy project installed in India: a critical review. Renew. Sustain. Energy Rev. 23, 80–90. doi: 10.1016/j.rser.2013.02.045
Martínez, E., Sanz, F., Pellegrini, S., Jiménez, E., and Blanco, J. (2009). Life-cycle assessment of a 2-MW rated power wind turbine: CML method. Int. J. Life Cycle Assess. 14, 52–63. doi: 10.1007/s11367-008-0033-9
National Determined Contribution. (2016). Federative Republic of Brazil. Towards achieving the objective of the United Nations framework convention on climate change. Available online at: https://unfccc.int/sites/default/files/BRAZIL%20iNDC%20english%20FINAL.pdf (accessed November 10, 2022).
National Determined Contribution. (2022). Federative Republic of Brazil. Paris Agreement. Brazil's Nationally Determined Contribution (NDC). Available online at: https://unfccc.int/sites/default/files/NDC/2022-06/Updated%20-%20First%20NDC%20-%20%20FINAL%20-%20PDF.pdf (accessed November 10, 2022).
National Electric System Operator. (2020). DPOR - Daily Preliminary Operation Report. Available online at: http://www.ons.org.br/paginas/conhecimento/acervo-digital/documentos-e-publicacoes?categoria=IPDO (accessed November 10, 2022).
National Institute of Meteorology - NIMET. (2020). NIMET Meteorological Database. Available online at: https://bdmep.inmet.gov.br/# (accessed November 10, 2022).
National Renewable Energy Laboratory – NREL. (2013). The Western Wind and Solar Integration Study Phase 2. Golden, CO: NREL. Available online at: https://www.nrel.gov/docs/fy13osti/55588.pdf (accessed November 24, 2022).
Nedaei, M., Assareh, E., and Walsh, P. R. (2018). A comprehensive evaluation of the wind resource characteristics to investigate the short term penetration of regional wind power based on different probability statistical methods. Renew. Energy 128, 362–374. doi: 10.1016/j.renene.2018.05.077
Nedaei, M., Walsh, P., and Assareh, E. (2020). Sustainable energy planning of a wind power plant by coordinating clean development strategies. Int. J. Energy Clean Environ. 21, 59–89. doi: 10.1615/InterJEnerCleanEnv.2020033676
Pereira, M. T. R. M., Carvalho, M., and Mady, C. E. K. (2022). Addressing energy demand and climate change through the second law of thermodynamics and LCA towards a Rational Use of Energy in Brazilian Households. Entropy. 24, 1524. doi: 10.3390/e24111524
Rankine, R. K., Chick, J. P., and Harrison, G. P. (2006). Energy and carbon audit of a rooftop wind turbine. Proc. Inst. Mech. Eng. A 220, 643–654. doi: 10.1243/09576509JPE306
Schreiber, A., Marx, J., and Zapp, P. (2019). Comparative life cycle assessment of electricity generation by different wind turbine types. J. Clean. Prod. 233, 561–572. doi: 10.1016/j.jclepro.2019.06.058
Schultz, H. S., and Carvalho, M. (2022). Design, greenhouse emissions, and environmental payback of a photovoltaic solar energy system. Energies 15, 6098. doi: 10.3390/en15166098
SimaPro. (2020). New release: SimaPro 9.1.1 is Here! Available online at: https://simapro.com/2020/whats-new-in-simapro-9-1-1/ (accessed November 10, 2022).
Simón-Martín, M., Puente-Gil, Á., Borge-Diez, D., Ciria-Garcés, T., and González-Martínez, A. (2019). Wind energy planning for a sustainable transition to a decarbonized generation scenario based on the opportunity cost of the wind energy: Spanish Iberian Peninsula as case study. Energy Procedia 157, 1144–1163. doi: 10.1016/j.egypro.2018.11.282
Stavridou, N., Koltsakis, E., and Baniotopoulos, C. C. (2020). A comparative life-cycle analysis of tall onshore steel wind-turbine towers. Clean Energy 4, 48–57. doi: 10.1093/ce/zkz028
Stergiannis, N., Caralis, G., van Beeck, J., and Runacres, M. C. (2021). The effect of wind energy on microclimate: lessons learnt from a CFD modelling approach in the case study of Chios Island. Appl. Sci. 11, 5873. doi: 10.3390/app11135873
Teffera, B., Assefa, B., Björklund, A., and Assefa, G. (2021). Life cycle assessment of wind farms in Ethiopia. Int. J. Life Cycle Assess. 26, 76–96. doi: 10.1007/s11367-020-01834-5
Thomson, R. C., and Harrison, G. P. (2015a). Life Cycle Costs and Carbon Emissions of Offshore Wind Power. High School Yards; Edinburgh: Edinburgh Climate Change Institute. Available online at: https://www.climatexchange.org.uk/media/1461/main_report_-_life_cycle_costs_and_carbon_emissions_of_offshore_wind_power.pdf (accessed November 24, 2022).
Thomson, R. C., and Harrison, G. P. (2015b). Life Cycle Costs and Carbon Emissions of Wind Power. High School Yards; Edinburgh: Edinburgh Climate Change Institute. Available online at: https://www.climatexchange.org.uk/media/1459/life_cycle_wind_-_executive_summary_.pdf (accessed November 24, 2022).
Thomson, R. C., Harrison, G. P., and Chick, J. P. (2017). Marginal greenhouse gas emissions displacement of wind power in Great Britain. Energy Policy 101, 201–210. doi: 10.1016/j.enpol.2016.11.012
Uysal, C., Agbulut, Ü., Elibol, E., Demirci, T., Karagoz, M., and Saridemir, S. (2022). Exergetic, exergoeconomic, and sustainability analyses of diesel–biodiesel fuel blends including synthesized graphene oxide nanoparticles. Fuel 327, 125167. doi: 10.1016/j.fuel.2022.125167
Keywords: wind energy, environmental impacts, greenhouse gas emissions, carbon footprint, Life Cycle Assessment, SDG 12
Citation: Fonseca LFS and Carvalho M (2022) Greenhouse gas and energy payback times for a wind turbine installed in the Brazilian Northeast. Front. Sustain. 3:1060130. doi: 10.3389/frsus.2022.1060130
Received: 02 October 2022; Accepted: 21 November 2022;
Published: 05 December 2022.
Edited by:
Antonino Marvuglia, Luxembourg Institute of Science and Technology (LIST), LuxembourgReviewed by:
Cuneyt Uysal, Karabük University, TurkeyCopyright © 2022 Fonseca and Carvalho. This is an open-access article distributed under the terms of the Creative Commons Attribution License (CC BY). The use, distribution or reproduction in other forums is permitted, provided the original author(s) and the copyright owner(s) are credited and that the original publication in this journal is cited, in accordance with accepted academic practice. No use, distribution or reproduction is permitted which does not comply with these terms.
*Correspondence: Monica Carvalho, bW9uaWNhQGNlYXIudWZwYi5icg==
Disclaimer: All claims expressed in this article are solely those of the authors and do not necessarily represent those of their affiliated organizations, or those of the publisher, the editors and the reviewers. Any product that may be evaluated in this article or claim that may be made by its manufacturer is not guaranteed or endorsed by the publisher.
Research integrity at Frontiers
Learn more about the work of our research integrity team to safeguard the quality of each article we publish.