- 1National Engineering Laboratory for Industrial Wastewater Treatment, East China University of Science and Technology (ECUST), Shanghai, China
- 2State Key Laboratory of Chemical Engineering, East China University of Science and Technology, Shanghai, China
The recovery of nutrients from unconventional water such as domestic sewage is considered as a sustainable solution to environmental sanitation, personal hygiene, water, and food safety issues. Source separation of urine and the sequenced treatment techniques are the promising approaches to recover the resources from this unconventional water. However, in the storage of urine, urea is hydrolyzed under the action of urease, resulting in odor, precipitation and the loss of ammonia, which is a challenge to be overcome in the process of urine recycling. This review collates research related to urine stabilization, and aims to summarize the characteristics and applications of existing urine stabilization methods, such as acidification, alkalization, electrochemistry, inhibitors and etc. Overall, acidification and alkalization have higher dosage requirements and have an attentional impact on the environment; electrochemical technology is suitable for decentralized sanitation facilities, but the inhibition duration is short; inhibitors are the least effective and are usually used to regulate urease activities in soil environment rather than for urine stabilization. Choosing the appropriate approaches of urine stabilization should focus on the overall perspective of urine resource utilization, consider the separation method and recovery form, and combine it with the concentration technology.
Introduction
Water is a natural resource that living things depend on. It is not only directly related to human health, but also affects people's lives in terms of energy and food. However, the current situation of global water supply and demand is not optimistic. Since the 1980s, due to population growth, socio-economic development and changes in consumption patterns, global water consumption has increased by 1% every year. Unfortunately, this situation is expected to continue until at least 2050, when water consumption will increase by 20–30% over the current period. For more than a month each year, about 4 billion people suffer from severe water scarcity, and 22 countries will face serious water shortage risks (United Nations World Water Assessment Programme (WWAP), 2017, 2019). As the demand for water continues to increase and the impact of climate change becomes more intuitive, they will affect the sustainable use of water resources and increase potential risk in conflicts between the users.
In contrast to the scarcity of water resources, 56.41% of the annual fresh water intake flows into the nature in the form of urban wastewater, industrial wastewater and agricultural drainage (United Nations World Water Assessment Programme (WWAP), 2017). In low-income and middle-income countries, such as India, South Africa, Nigeria, etc., only a low percentage of wastewater is discharged after treatment (Akpan et al., 2020; Montwedi et al., 2021; Vij et al., 2021). Less treated wastewater is used for irrigation and agriculture, and most countries currently recycle <10% of the total wastewater flow, which not only pollutes the environment, but also wastes a lot of available resources (Mayer et al., 2016). The collection, treatment and safe use of wastewater are the basis for the formation of a circular economy, balanced economic development and sustainable use of resources. After treatment, wastewater will become a “precious” resource that can meet the growing demand for clean water and other raw materials.
As the concept of sustainable development has been accepted by most countries, the idea of recycling resources from wastewater has been vigorously promoted by governments and increasingly supported by people. Domestic sewage in wastewater is a typical unconventional water due to its rich nutrition, it has become an attractive choice for unconventional water reuse. Urine is an important part of domestic sewage, which contains ~50% of the phosphorus and 70–85% of the nitrogen (in the form of urea) in the toilet wastewater (Sundberg, 1995). As a nitrogen-containing organic matter, urea plays an important role in the global nutrient cycle and is a key molecule in the water-energy-food nexus (Figure 1). In 2020, 132 million people would suffer from undernourishment due to the impact of the COVID pandemic (FAO, 2020). The essential way to solve this problem is to continuously increase the productivity under the premise of sustainable consumption of natural resources, ensure the integrity and optimization of all links in the food supply chain, and increase the purchasing power of the disadvantaged groups. Recycling urea from urine wastewater is undoubtedly a sustainable way to turn waste into treasure, effectively reducing the dependence on the Haber-Bosch method for fertilizer production, saving costs, and reducing environmental impact, especially in developing countries (Akpan-Idiok et al., 2012; Andersson, 2015; Araújo et al., 2019). Compared with mineral fertilizers, urine fertilizers reduce the need for energy and the impact on the environment (Medeiros et al., 2020). It may soon become an important part of ecological wastewater management.
The use of urine and feces as natural fertilizers can be traced back to ancient times, but there are environmental and health risks (Angelakis and Rose, 2014). If a large amount of nutrient-rich urine is discharged without any treatment, it easily leads to the eutrophication of water and soil, and the microorganisms in the excrement may also cause disease transmission. The Sustainable Target 6.2 proposed by the United Nations is: by 2030, achieve access to adequate and equitable sanitation and hygiene for all and end open defecation, paying special attention to the needs of women and girls and those in vulnerable situations. However, only 2.9 billion people (39%) worldwide had access to safely managed sanitation services by 2015 (WHO, 2018). The “World Water Development Report 2019” released by the United Nations emphasized the need to implement the “2030 Agenda for Sustainable Development” and recognize the human rights of safe drinking water and sanitation, both of which are essential for eradicating poverty and building a prosperous society (Andersson, 2015; Araújo et al., 2019; United Nations World Water Assessment Programme (WWAP), 2019).
Since the last century, people have begun to scientifically evaluate the methods and effects of urine resource utilization. Nowadays, most countries adopt traditional toilet drainage mode. A large amount of clean water is used to dilute human feces, urine and other excrement, mixed and transported to the sewage treatment plant for centralized treatment through the municipal pipe network. This treatment mode not only consumes a lot of water resources, but also completely blocks the ecological path of nutrients in feces and urine returning to the land. With the increasing municipal development and human population, sewage discharge, and pollution load exceed the self-purification and bearing capacity of natural water bodies. In order to meet the sanitation requirements and environmental protection requirements, it is necessary to carry out the reconstruction and expansion of the sewage treatment system, the discharge system and the continuous improvement of the sewage treatment level, which is accompanied by a large increase in investment as well as operation and maintenance costs. On the other hand, in many countries and regions, due to economic and technical reasons, this traditional drainage system cannot be constructed and operated.
To solve this problem, Sweden first proposed the urine source separation system as a sustainable alternative to centralized wastewater treatment systems in 1980. Source separation system means that urine is collected separately from feces and other domestic water through the transformation of sanitation facilities, and treated separately according to their characteristics. Since 1997, a series of source separation drainage practices have been promulgated, led by European countries. In 1997, the Swiss Federal Institute of Water Science and Technology (EAWAG) introduced NoMix toilets in several small pilot projects in the NOVAQUATIS project (Hellström and Johansson, 1999; Lienert and Larsen, 2006). In the same year, the Danish government invested 42 million US dollars in the implementation of the “Ecological Activity Plan.” The German government launched an eco-sanitation system plan in 2001, with the main goal of establishing an eco-sanitation system demonstration project in densely populated cities. Around the world, major international organizations such as Stockholm Environmental Research Institute (SEI), German Technical Cooperation Corporation (GIZ), Swiss Federal Institute of Water Quality (EAWAG), International Water Association Eco-Sanitation Technical Expert Committee, UNICEF, World Health Organization (WHO), Bill & Melinda Gates Foundation (BMGF), etc. have all actively promoted source separation drainage technology (Gajurel et al., 2003; Wang and Bao, 2007; Beal et al., 2008; Bodík and Ridderstolpe, 2008).
The source-separated urine system is used to achieve sanitation goals, which is beneficial to the effective recovery of nutrients, simple control of micro-pollutants, and reduction of health hazards from fecal pathogens (Larsen and Gujer, 1996; Ganesapillai et al., 2016). However, there are still many problems during the storage, transport and usage of source-separated urine. The excreted urine generally has an initial pH of 4.8–7.5, and about 75–90% of nitrogen exists in the form of urea, which only produces a slightly peculiar smell, but through storage, part of the urea is rapidly hydrolyzed, raising the pH to 9.0–9.3, accompanied by precipitation and ammonia gas with pungent odor (Diem, 1970; Stenström et al., 2000). The calcium and magnesium ions in the urine spontaneously precipitate as calcium phosphate, struvite, and calcite due to the increase in pH, especially in undiluted urine. It is easy to scale and clog the toilet pipe, which is common in engineering cases of source separation, causing great difficulties to the operation and maintenance of the system. This undesirable conversion will lose a large amount of nitrogen and reduce the recovery efficiency, and hinders the implementation and promotion of urine source separation technology (Udert et al., 2003b, 2006). Therefore, it should be avoided through appropriate measures in the project, that is, urine needs to be stabilized first in pretreatment before recovery. Based on this problem, a method to artificially promote the complete hydrolysis of urea and then recovering struvite precipitation is adopted to reuse the nutrients in the urine (Udert et al., 2003a; Fumasoli et al., 2015; Olofsson, 2016). However, compared with the capture of urea, this method is cumbersome and would adversely affect the environment (Xu, 2008). According to the life cycle analysis of using urine as fertilizer, controlling ammonia volatilization is the key to reducing acidification and eutrophication impacts (Medeiros et al., 2020).
Urea Hydrolysis and Urine Stablization
In 1824, Wöhler synthesized ammonium cyanate by mixing cyanic acid with ammonia and then evaporating the solution, but accidentally obtained urea in the product (Figure 2), which strongly proved that organic matter can be synthesized from inorganic matter, overturned the vitalistic theory that hindered the development of chemistry at that time. In 1895, Walker and Hambley first noticed the reverse reaction of Wöhler's urea synthesis (Walker and Hambly, 1895). Subsequently, the urea decomposition reaction in the presence of acid and base was discussed (Fawsitt, 1902; Werner, 1918; Warner, 1942). When heated in an acid or alkali-containing aqueous solution, the decomposition ability of urea generally depends on its ability to decompose into ammonia and cyanic acid, and the hydrolysis of cyanic acid and basic cyanate ester is a representative step of urea hydrolysis.
Urea is a stable compound with a half-life of 3.6 years when it is chemically hydrolyzed without enzymes at 38°C, while urine contaminated with feces usually shows positive hydrolysis properties, of which the hydrolysis rate even reaches 104 times that of chemical hydrolysis (Andrews et al., 1984; Amtul et al., 2002). Hotta and Funamizu found the growth, decline and inhibition process of ammonification potential during the storage process of urine with fecal contamination (Hotta and Funamizu, 2008b). The reason for this phenomenon is that the stool contains a variety of urease-containing bacteria, which hydrolyzes urea when mixed with urine (Suzuki et al., 1979). In a source separation system, this reaction usually happens because of the cross-contamination of residue feces in the toilet bowl and sewer pipe, or biofilm formed on the pipe surface. Airborne bacteria can also be an alternative source of infection. Urease, a nickel-containing oligomerase, which is widely present in bacteria, fungi, plants, algae and invertebrates in nature, is dominating the hydrolysis of urea (Karine et al., 2018). Its structure is shown in Figure 3. Since urease has bi-nickel active sites, when interacting with urea, one binds and activates the substrate urea, and the other binds and activates water molecules (Ciurli et al., 1999; Amtul et al., 2002). It is generally believed that the reaction of urea hydrolysis by urease is composed of four steps. The overall reaction equation (1) and stepwise equations (2)–(5) are as follows (Mobley and Hausinger, 1989).
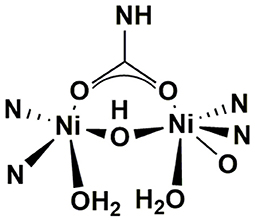
Figure 3. Structure of Urease (Mazzei et al., 2019).
Urease converts urea and water into ammonia and carbamate, which then spontaneously hydroxylates to ammonia and carbonic acid. In the state-of-art source separation system, urine is completely hydrolyzed into a mixture of inorganic salts, subsequently followed by recovering phosphorus through magnesium ammonium phosphate precipitation, and nitrogen sequestration through either ion exchange, electrochemical technology, biological treatment, or stripping (Udert et al., 2003a; Xu, 2008; Olofsson, 2016; Tun et al., 2016; Simha et al., 2018; Ray et al., 2019; Xu et al., 2019). However, the hydrolysis of urine means that it may cause the aforementioned problems such as pipe blockage, odor caused by ammonia volatilization, air pollution, and waste of resources. Considering this situation, inhibiting urea hydrolysis, that is, urine stabilization, not only avoid these problems, but also provide necessary conditions for the direct recovery of urea in urine.
The formation of ammonia in the hydrolysis of urea causes an increase in pH. There is a direct connection between the increased ammonia concentration and the electrical conductivity, indicating that the hydrolysis of urea in the storage container can be tracked by the changes in urea concentration, ammonia concentration, conductivity and pH over time, as shown in Figures 4, 5 (Hellström et al., 1999; Zhang et al., 2013a; Ray et al., 2018). Source separation of pathogens in urine and feces may cause up to 22–37% of cross-infection, leading to the spread of pathogenic microorganisms and endangering human health (Schönning et al., 2002; Bischel et al., 2015). A model based on a source separation system that can predict changes in ammonia concentration over time using urine output, remaining urine volume from previous urination events, and experimental time was established since urea hydrolysis has been demonstrated to follow a first-order response (Saetta et al., 2019). Hotta and Funamizu used the initial ammoniation rate (IAR) to describe the hydrolysis of urea contaminated by feces, and studied the influence of three factors: pH, free ammonia nitrogen (FAN) and salinity on the inhibitory effect of urea hydrolysis. At 30°C, urea hydrolysis is most active when the pH is 5–8, while FAN and salinity have a significant negative effect on urea hydrolysis (Hotta and Funamizu, 2008a).
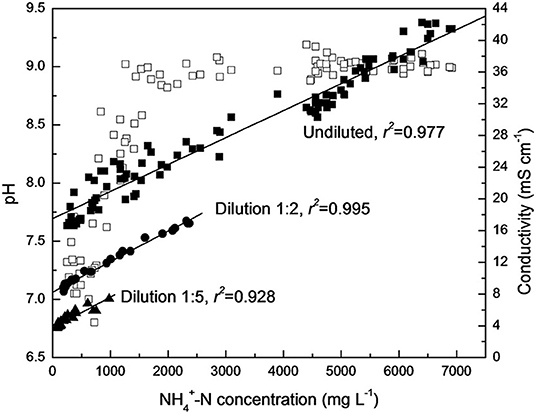
Figure 4. Correlation of pH value (□), conductivity (▴•■), and corresponding -N concentration during urine hydrolysis process. Reprinted with permission from Zhang et al. (2013a). Copyright ©2013 Taylor & Francis.
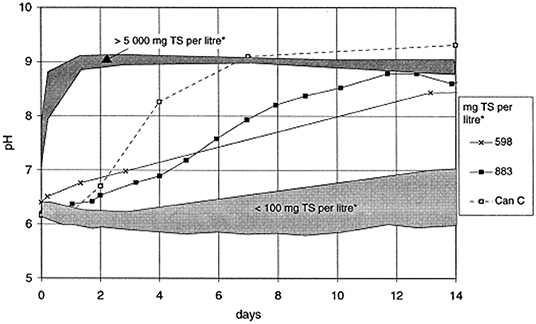
Figure 5. Variations of pH during storage of urine with different concentrations of feces. Reprinted with permission from Hellström et al. (1999). Copyright ©1999 Elsevier Science B.V. All rights reserved.
The enzymatic hydrolysis of urea is a necessary process during fertilization recommendation. As the most common organic nitrogen fertilizer, urea is hydrolyzed into ammonium carbonate or ammonium bicarbonate by the action of urease in the soil, which can be absorbed and utilized by crops. In order to make the absorption sufficient, urea is usually applied 4–8 days before the fertilizer demand period of the crops, and the early hydrolysis will volatilize the ammonia and cause the waste of nitrogen. Therefore, it is necessary to grasp the timing of urea hydrolysis to avoid hydrolysis as much as possible before it becomes fertilizer to ensure the high efficiency of nitrogen cycle.
Currently, acidification, alkalization, electrochemical treatment, adding inhibitors, etc. are all feasible methods to decelerated the enzymatic activity of urease. Different methods have different mechanisms for inhibiting urease activity. The pH, temperature, salinity, free ammonia concentration and some other factors can be controlled to affect the stability of urine. Stabilization of urine also aims to inactivate pathogens. The effect of various stabilization methods on pathogen inactivation should be evaluated. The following will classify and sort the previous studies according to different methods, which will help researchers to intuitively understand the current status of urine stabilization, and put forward their own views on its advantages and disadvantages and future development directions. The representative studies of different methods of urine stabilization are listed in Table 1.
Acidification
One of the methods to inhibit the hydrolysis of urea is to add acid to maintain the pH at a low level. In 1999, Daniel Hellström studied the inhibitory effect of acidification on urea decomposition during urine storage. The results show that, at the initial stage of storage, a one-time dose of 60 meq of sulfuric acid or acetic acid per liter of undiluted urine can inhibit the decomposition of urea in multiple doses of urine tanks for more than 100 days (Hellström et al., 1999). In 2012, in order to eliminate excess sulfate ions in pretreated urine, Dean Muirhead replaced the sulfuric acid (H2SO4) in the urine stabilizer solution with three different mineral acids (phosphoric acid, hydrochloric acid, and nitric acid), and control the dose to stabilize the urine and minimize the risk of mineral precipitation during the distillation process, obtained the results that phosphoric acid is the safest alternative acid of the three tested to replace sulfuric acid (Muirhead and Carrier, 2012).
In 2017, Saetta et al. added 2.5 ml of 2,500 meq/L acetic acid to an anhydrous urinal after each urination to reduce the pH to inhibit the hydrolysis of urea in artificial urine and real urine (Saetta and Boyer, 2017). Hannah Ray designed an experiment to study the inhibitory effect of three types of urease inhibitors, metal, fluoride and acid on urea hydrolysis. The results were citric acid> acetic acid> vinegar> sulfuric acid> ionic silver> ionic zinc> sodium fluoride. Acetic acid, citric acid and vinegar can effectively inhibit urea hydrolysis when the concentration is between 3.2 × 101 and 1.6 × 102 meq L−1 (Ray et al., 2018). It is worth noting that the order of addition of urease and acid will affect the hydrolysis of urea. The pre-adjusted acidic environment can more inhibit the activity of urease. Since the addition of acid is a reversible pH-dependent inhibition strategy, adding acid before going to the toilet for the first time in the morning and feeding regularly to maintain a low pH is an effective way to stabilize urine. Batch chemical addition experiments also proved this method.
In addition, acidification has a positive effect on hygiene. In the case of low pH, the number of microorganisms and bacteria in the urine is greatly reduced, and it is difficult for pathogens to survive, which to a certain extent curbs the spread of diseases. Therefore, acidification is a good choice for under-developed areas.
Alkalization
Urease is inhibited when the pH is higher than 10 or the temperature is higher than 80°C (Hotta and Funamizu, 2008a; Geinzer, 2017). This inhibition may be due to the inactivation of urease-producing microorganisms in urine at high pH. In the condition of pH higher than 12, although it can inhibit the enzymatic hydrolysis of urea, it promotes the non-enzymatic hydrolysis of urea, that is, the elimination reaction catalyzed by OH− for degradation. However, this uncatalyzed hydrolysis is 1,010 times slower than enzyme-catalyzed hydrolysis (Senecal and Vinnerås, 2017). Therefore, the addition of strong alkali to fresh urine combines the prevention of hydrolysis and increases the availability of plant nutrients, is an effective way to stabilize urea.
The combination of alkali stabilization (calcium hydroxide) and volume reduction (reverse osmosis) is regarded as the most promising treatment method to achieve maximum resource recovery, as shown in Figure 6 (Chipako and Randall, 2020). Randall used calcium hydroxide to increase the pH to stabilize fresh urine, evaluated the influence of temperature and pH on inhibiting hydrolysis, gave a recommended working temperature range of 14°-40°C and the upper limit of enzymatic urea hydrolysis pH is 11, in which condition urine can be stored for one month without urea hydrolysis (Randall et al., 2016). Compared with the traditional phosphorus precipitation agent MgCl2, calcium hydroxide is cost-effective, and the additional cost is only 0.8 cents/L urine (specifically 10 g Ca(OH)2/L urine). In 2017, Jenna Senecal alkalized fresh human urine before concentrating, added wood ash or biochar at 35° and 65°C to inhibit urease, and finally obtained a fertilizer containing 7.8% nitrogen, 2.5% phosphorus and 10.9% potassium (Senecal, 2017). During this process, microorganisms (Enterococcus faecalis, MS2 phage and Φx174 phage) and pathogens (Ascaris suis and Salmonella enteritidis) in the urine were effectively removed. This method is applied to Blue Diversion Autarky Toilet (BDAT) in Switzerland (Sutherland et al., 2021).
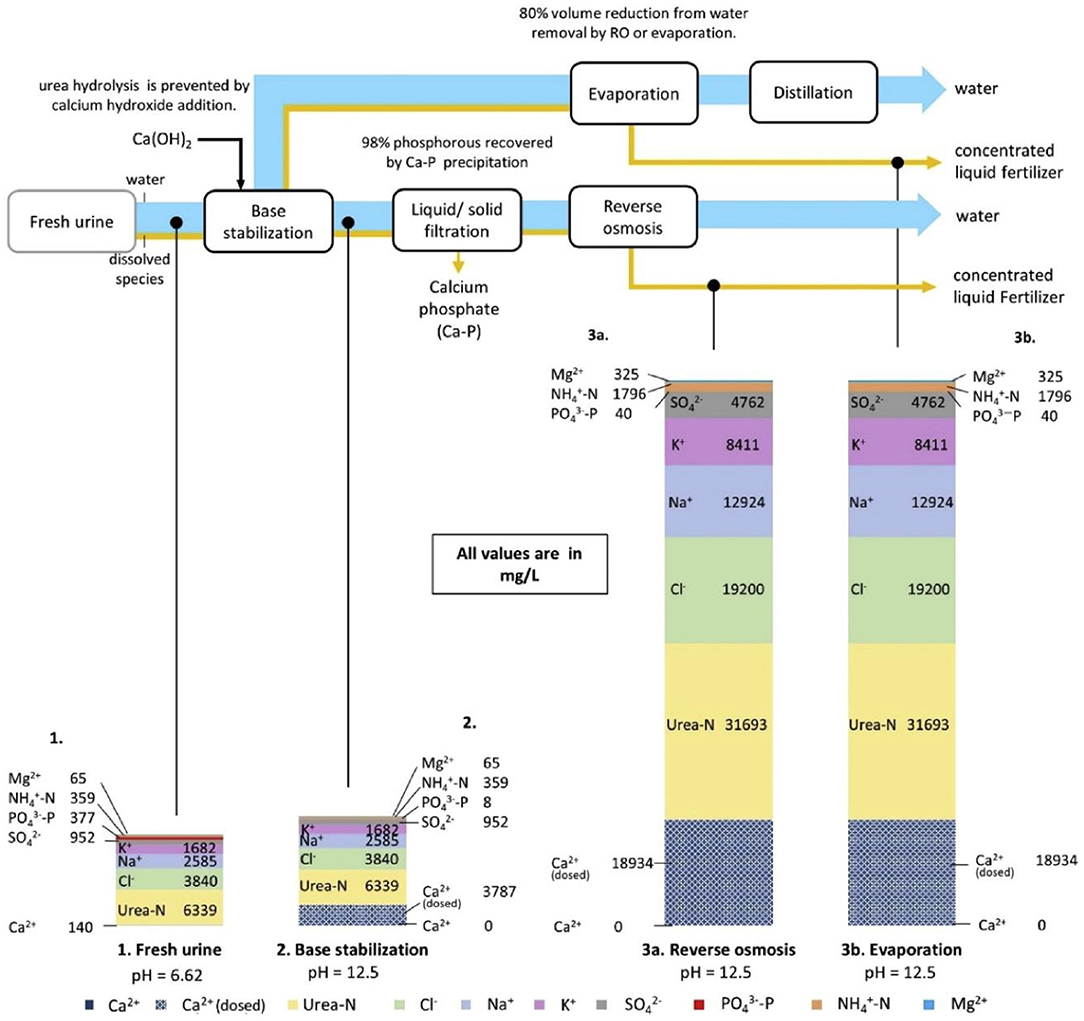
Figure 6. The process of stabilizing urine with alkalization. The size of the arrows represents a mass flow of dissolved species in urine and water. Reprinted with permission from Chipako and Randall (2020). Copyright ©2019 Elsevier Ltd.
The literature indicates that adding alkali to urine can effectively inhibit the hydrolysis of urea and inactivate pathogens, but the precipitation of calcium and magnesium ions under alkaline conditions should be considered and effective measures should be taken to avoid clogging the pipeline. In addition, the pH should be maintained at 8–11 to prevent chemical hydrolysis of urea.
Electrochemistry
The essence of the electrolysis method mentioned so far is that the product after electrolysis stabilizes the urine. Electrolysis was originally used for the sterilization and disinfection of source separated urine, which was attributed to the strong oxidizing nature of the chlorine species produced. Sanyo's research found that the production of active chlorine at high potentials can inhibit urease activity, which is believed to be the inactivation of urease caused by the unfolding of urease. Through the PtIr/Pt/PtIr electrode system, they can produce chlorine in situ to avoid urea hydrolysis at a potential above 240 mV. The treated urine can also be used as toilet flushing water, and the electricity cost is lower than the daily water cost (Ikematsu et al., 2007).
Depape et al. used the electrochemical induced precipitation method to circulate urine between the sedimentation tank and the cathode chamber of the electrochemical cell, as shown in Figure 7 (De Paepe et al., 2020). The concept developed by Clauwaert et al. for softening water provides inspiration (Clauwaert et al., 2020). The hydroxide ions produced by the cathodic hydrogen evolution reaction during electrolysis are used as an alternative source of hydroxide ions in the alkalization method to inhibit urine hydrolysis, which represents less environmental pollution and a more sustainable operation mode. In the recent work of Depape et al. electrochemically stabilized urine is fed to a nitrification reactor, and the pH is controlled by adjusting the current to promote the complete nitrification reaction. This provides a solution for stabilizing urine in situ and extracting and concentrating nutrients.
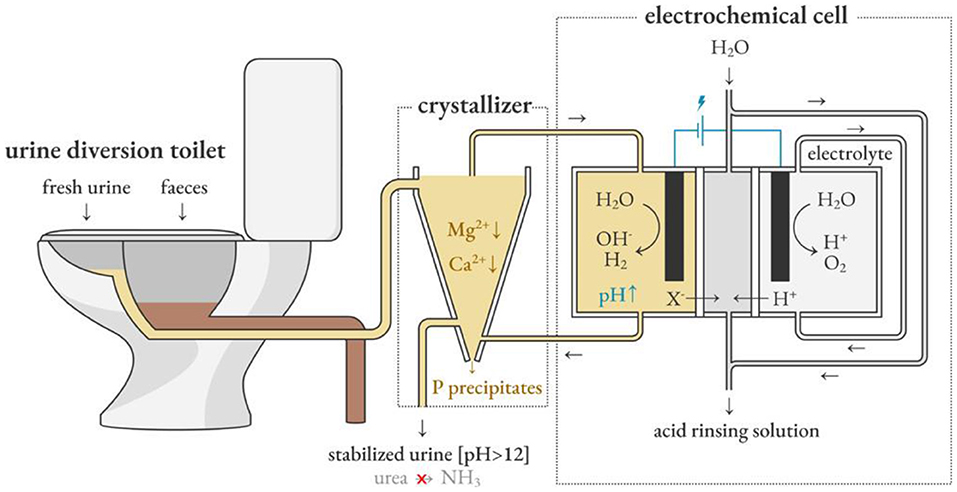
Figure 7. Electrochemically induced precipitation stabilizes fresh urine and promotes source separation. Reprinted with permission from De Paepe et al. (2020). Copyright ©2020 American Chemical Society.
Electrochemical method can inhibit the hydrolysis of urea in a short period of time. It is a promising and stable technology that can be used in decentralized sanitation facilities. With the large-scale application of renewable electricity, the cost of electricity will gradually decrease, which makes the electrochemical method both safe and sustainable. While the electrochemical method is applied to urine stabilization, the device should be installed in the toilet or as close as possible, and the batch feed mode should be adopted to increase the pH and then send it to the storage tank for centralized processing to minimize the time before stabilization. Similar to the principle of alkalization, the optimal pH value for electrochemical stability and precipitation should be around 11.
Chemical Inhibitor and Oxidizer
Inhibitors are usually used to inhibit the hydrolysis of urea in the soil rather than the stabilization of urine, but their research has always been an important part of the molecular field. The existing urease inhibitors can be roughly divided into several categories: hydroxamic acid and its derivatives, substituted bisphosphoric acid amides, heterocyclic compounds, thiolic compounds, nickel ligands and chelating agents, such as metal ions, etc.
The inhibitory effect of heavy metal ions on urease is usually attributed to the reaction of ions with thiolase, which leads to the formation of thiols (Rezaei Behbehani et al., 2011). Among metal ions, silver and mercury have better inhibitory properties, followed by copper, nickel, cadmium, zinc, and cobalt plasma. Metals inhibit hydrolysis by binding to functional groups necessary for enzyme catalytic function. The fluoride is directly combined with active nickel centers (Ray et al., 2018). These metals have stronger affinities for various sulfhydryl compounds, and are more active than urea. They can bind to the necessary sulfhydryl group in the center of the enzyme and inhibit the activity of urease.
Some urease inhibitors are found in natural plants, and some require artificial synthesis. Zofia Olech determined that garlic (garlic, onion, leeks) and brassica (cabbage, cabbage) plant juices have an inhibitory effect on kidney bean urease activity, which is due to the thiosulfite contained therein (Olech et al., 2014). Wang et al. explored the effects of urease inhibitors hydroquinone (HQ), phenyl diphosphate diamide (PPDA) and N-(n-butyl) thiophosphoric triamide (NBPT) in delaying urea hydrolysis, mineral nitrogen formation and passing volume, NBPT is the most effective urease inhibitor under aerobic conditions. PPDA has a better inhibitory effect under waterlogged conditions, Hydroquinone has lower inhibitory properties but has the advantage of low cost and easy synthesis (Zhengping et al., 1991).
In 2015, Mnaza Noreen used the indoxyl method to determine its inhibitory effect on the urease activity. The results showed that N-((5-(4-chlorophenyl)thiophen-2-yl)sulfonyl) acetamide at a concentration of 15 μg/mL, IC50 17.1 μg/mL has a good inhibitory effect on urease, and the inhibition rate is ~46.23 ± 0.11% (Mnaza et al., 2015). Disulfiram and other compounds containing thiol reactive groups can be used to redesign better and more specific urease inhibitors (Díaz-Sánchez et al., 2016). In 2018, Liu et al. found that biochar has an inhibitory effect on urease activity, which may be the result of the oxidation reaction of biochar surface with free radicals or the oxidation reaction with free radical-promoted active oxygen (Liu et al., 2018).
These urease inhibitors have certain biological toxicity and should be used with caution. Some inhibitors such as glutaraldehyde can be used as disinfectants and deodorants. However, people can reuse new pollutants such as chemicals in the environment and their metabolites to achieve environmental friendliness. Although this characteristic represents a risk to the soil system, it can be further studied for application in the field of urea stabilization.
Heat Solar and Others
According to Pahore's research, a high concentration of salt, urea and creatinine mixture (up to 227–334 g L−1) can effectively control the hydrolysis of urea in urine contaminated by feces (Pahore et al., 2012). The addition of NaCl at a concentration of 150 g/L can reduce the initial ammoniation rate of stored urine contaminated with feces by 50% (Hotta and Funamizu, 2008a). For urine with a low level of fecal pollution, this is a feasible stabilization method, but it has the disadvantages of high raw material consumption and low efficiency.
Directly adding an oxidant to inhibit hydrolysis of urea is also an effective method. In water treatment, peroxides play an important role because of their oxidizing properties. Hydrogen peroxide can directly combine or coordinate peroxy (—O—O—) and hydroperoxy (H—O—O—) to reactant molecules to form peroxyacids and peroxy complexes. Various hydrogen peroxide derivatives are also formed through molecular addition. Hydrogen peroxide and its inorganic derivatives show great chemical activity in many reactions, and have the ability to decompose into functional groups. These properties enable hydrogen peroxide to be used in sewage treatment and have a detoxification effect on cyanide-containing wastewater and nitrite-containing wastewater. Sodium peroxide can be used as a material for softening drinking water and wastewater treatment, which can demineralize the water and prevent the formation of algae and aquatic plants. It can disinfect sewage with the participation of catalyst. It can also be used as a deodorizer to remove the odor of human waste or wastewater. In terms of urine stabilization, the oxidizing property of hydrogen peroxide has the ability to inactivate cells, so it can inactivate bacteria and oxidize urease. Compared with ozone, hydrogen peroxide has a more significant inhibitory effect on the hydrolysis of urea, which is also effective in urine contaminated by feces. Studies shows that adding 0.03 mol/L of hydrogen peroxide can inhibit the hydrolysis of urea in fresh urine (Zhang et al., 2013b). Furthermore, in urine contaminated by feces, the amount of hydrogen peroxide required increases with the degree of pollution.
Due to the strong toxicity of oxidants and strong acids, Niklas Adam et al. explored less toxic stable technologies, such as food-grade and commercial health preservatives (Adams et al., 2012). The inactivation of urease caused by high temperature is also one of the ideas to inhibit urea hydrolysis. Compared with room temperature storage, urea hydrolysis is inhibited under 60° and 70°C storage conditions (Hagenkamp-Korth et al., 2015; Zhou et al., 2017). Therefore, human urine can be stored at 70°C for 7 days to meet the purpose of hygiene and stability.
Summary and Conclusion
Urine stabilization is not an isolated method, but in the cycle of source separation and recycling of urine. Taking into account the dynamic relationship between the separation, stabilization, concentration and recycling of urine, the selection of a suitable stabilization method is related to the urine collection method, application, and expected recovery form. In addition, economic costs and environmental impact also occupy an important position. This article collates the previous work in the field of urine stabilization and evaluates the advantages, disadvantages and applicability of various stabilization methods.
Among the various urine stabilization methods, acidification, and alkalization have the advantages of simple operation, but there are potential safety hazards and need to be carefully applied in public health facilities. The use of large amounts of acid and alkali may also cause a burden on the environment. Electrochemical method is a promising and stable technology that can be used in decentralized sanitation facilities to inhibit urea hydrolysis in a short period of time. It is foreseeable that if the cost can be reduced, it will be widely used. Urease inhibitors are rarely used in industry because of their low efficiency, but their research is constantly advancing. A molecular inhibitor with excellent performance may appear in the future and be treated for urine stabilization.
Current research usually evaluates the influence of factors such as agents and temperature on the experiment revolves around one method only, without comparing various stabilization techniques. In the future, the inhibitory effect, economic cost and environmental impact of different urine stabilization technologies can be evaluated through life cycle assessment and other tools to provide a basis for the selection of stabilization technologies.
Author Contributions
WY was responsible for the collection and sorting of documents related to urine stabilization. JL and XY provided assistance in the selection and summary of the documents. All authors edited and revised the document throughout.
Funding
The authors acknowledge the was supported financially by National Key Basic Research Program of China (2019YFA0705800, 2019YFC1906700), the National Natural Science Foundation of China (Nos. 22006039, 21876049 and 91834301), the Strategic Priority Research Program of the Chinese Academy of Sciences (XDA23010400) and the China Postdoctoral Science Foundation(2019M661412).
Conflict of Interest
The authors declare that the research was conducted in the absence of any commercial or financial relationships that could be construed as a potential conflict of interest.
Publisher's Note
All claims expressed in this article are solely those of the authors and do not necessarily represent those of their affiliated organizations, or those of the publisher, the editors and the reviewers. Any product that may be evaluated in this article, or claim that may be made by its manufacturer, is not guaranteed or endorsed by the publisher.
References
Adams, N., Mitchell, J., and Pickering, K. (2012). “Development of low-toxicity urine stabilization for spacecraft water recovery systems,” in International Conference on Environmental Systems (ICES) (Bellevue, WA), 14–18.
Akpan, V. E., Omole, D. O., and Bassey, D. E. (2020). Assessing the public perceptions of treated wastewater reuse: opportunities and implications for urban communities in developing countries. Heliyon 6:e05246. doi: 10.1016/j.heliyon.2020.e05246
Akpan-Idiok, A. U., Udo, I. A., and Braide, E. I. (2012). The use of human urine as an organic fertilizer in the production of okra (Abelmoschus esculentus) in South Eastern Nigeria. Resour. Conserv. Recycling 62, 14–20. doi: 10.1016/j.resconrec.2012.02.003
Amtul, Z., Atta ur, R., Siddiqui, R. A., and Choudhary, M. I. (2002). Chemistry and mechanism of urease inhibition. Curr. Med. Chem. 9, 1323–1348. doi: 10.2174/0929867023369853
Andersson, E. (2015). Turning waste into value: using human urine to enrich soils for sustainable food production in Uganda. J. Clean. Prod. 96, 290–298. doi: 10.1016/j.jclepro.2014.01.070
Andrews, R. K., Blakeley, R. L., and Zerner, B. (1984). Urea and urease. Adv. Inorg. Biochem. 6, 245–283.
Angelakis, A. N., and Rose, J. B. (2014). Evolution of Sanitation and Wastewater Technologies Through the Centuries. London: IWA Publishing.
Araújo, N. C., Lima, V. L. A., Lima, G. S., Andrade, E. M. G., Ramos, J. G., and Oliveira, S. J. C. (2019). Nutrient contents and growth of corn fertigated with human urine and cassava wastewater. Revista Bras. Engenharia Agrícola e Ambiental. 23, 681–686. doi: 10.1590/1807-1929/agriambi.v23n9p681-686
Beal, C., Gardner, T., Ahmed, W., Walton, C., and Harris, D. (2008). Urine-seperation and reuse trial. Water 35, 66–69.
Bischel, H. N., Özel Duygan, B. D., Strande, L., McArdell, C. S., Udert, K. M., and Kohn, T. (2015). Pathogens and pharmaceuticals in source-separated urine in eThekwini, South Africa. Water Res. 85, 57–65. doi: 10.1016/j.watres.2015.08.022
Bodík, I., and Ridderstolpe, P. (2008). Sustainable Sanitation in Central and Eastern Europe - Addressing the Needs of Small and Medium-Size Settlements. Published by Global Water Partnership Central and Eastern Europe.
Chipako, T. L., and Randall, D. G. (2020). Urine treatment technologies and the importance of pH. J. Environ. Chem. Eng. 8:11. doi: 10.1016/j.jece.2019.103622
Ciurli, S., Benini, S., Rypniewski, W. R., Wilson, K. S., and Mangani, S. (1999). Structure-based mechanism for urease: why urea hydrolysis costs two nickels. J. Inorg. Biochem. 74, 18–18.
Clauwaert, P., De Paepe, J., Jiang, F., Alonso-Fariñas, B., Vaiopoulou, E., Verliefde, A., et al. (2020). Electrochemical tap water softening: a zero chemical input approach. Water Res. 169:115263. doi: 10.1016/j.watres.2019.115263
De Paepe, J., De Pryck, L., Verliefde, A. R. D., Rabaey, K., and Clauwaert, P. (2020). Electrochemically induced precipitation enables fresh urine stabilization and facilitates source separation. Environ. Sci. Tech. 54, 3618–3627. doi: 10.1021/acs.est.9b06804
Díaz-Sánchez, Á., G., Alvarez-Parrilla, E., Martínez-Martínez, A., Aguirre-Reyes, L., Orozpe-Olvera, J. A., et al. (2016). Inhibition of urease by Disulfiram, an FDA-approved thiol reagent used in humans. Molecules 21:1628. doi: 10.3390/molecules21121628
FAO, UNICEF, WFP, and WHO. (2020). The State of Food Security and Nutrition in the World 2020. Rome: FAO.
Fawsitt, C. E. (1902). Die Zersetzung des Harnstoffs. Z. Phys. Chem. 41U, 601–629. doi: 10.1515/zpch-1902-4143
Fumasoli, A., Etter, B., Sterkele, B., Morgenroth, E., and Udert, K. M. (2015). Operating a pilot-scale nitrification/distillation plant for complete nutrient recovery from urine. Water Sci. Tech. 73, 215–222. doi: 10.2166/wst.2015.485
Gajurel, D., Li, Z., and Otterpohl, R. (2003). Investigation of the effectiveness of source control sanitation concepts including pre-treatment with Rottebehaelter. Water Sci. Tech. 48, 111–118. doi: 10.2166/wst.2003.0029
Ganesapillai, M., Simha, P., Gupta, K., and Jayan, M. (2016). Nutrient recovery and recycling from human urine: a circular perspective on sanitation and food security. Proc. Eng. 148, 346–353. doi: 10.1016/j.proeng.2016.06.461
Geinzer, M. (2017). Inactivation of the urease enzyme by heat and alkaline pH treatment Retaining urea nitrogen in urine for fertilizer use (Master's thesis). SLU, Swedish University of Agricultural Sciences, Uppsala, Sweden.
Hagenkamp-Korth, F., Haeussermann, A., and Hartung, E. (2015). Effect of urease inhibitor application on urease activity in three different cubicle housing systems under practical conditions. Agric. Ecosyst. Environ. 202, 168–177. doi: 10.1016/j.agee.2015.01.010
Hanæus, A., Hellström, D., and Johansson, E. (1996). Conversion of urea during storage of humane urine. Vatten 52, 263–270.
Hanæus, J., Hellström, D., and Johansson, E. (1997). A study of a urine separation system in an ecological village in Northern Sweden. Water Sci. Technol. 35, 153–160. doi: 10.1016/S0273-1223(97)00193-5
Hellström, D., and Johansson, E. (1999). Swedish experiences with urine separating systems. Wasser Boden 51, 26–29.
Hellström, D., Johansson, E., and Grennberg, K. (1999). Storage of human urine: acidification as a method to inhibit decomposition of urea. Ecol. Eng. 12, 253–269. doi: 10.1016/S0925-8574(98)00074-3
Hotta, S., and Funamizu, N. (2008a). Inhibition factor of ammonification in stored urine with fecal contamination. Water Sci. Technol. 58, 1187–1192. doi: 10.2166/wst.2008.349
Hotta, S., and Funamizu, N. (2008b). Evolution of ammonification potential in storage process of urine with fecal contamination. Bioresour. Technol. 99, 13–17. doi: 10.1016/j.biortech.2006.12.001
Ikematsu, M., Kaneda, K., Iseki, M., and Yasuda, M. (2007). Electrochemical treatment of human urine for its storage and reuse as flush water. Sci. Total Environ. 382, 159–164. doi: 10.1016/j.scitotenv.2007.03.028
Karine, K., Angela, R. P., Celia, R. C., and Rodrigo, L. B. (2018). Ureases: historical aspects, catalytic, and non-catalytic properties-a review. J. Adv. Res. 3–17. doi: 10.1016/j.jare.2018.05.010
Larsen, T. A., and Gujer, W. (1996). Separate management of anthropogenic nutrient solutions (human urine). Water Sci. Technol. 34, 87–94. doi: 10.2166/wst.1996.0420
Lienert, J., and Larsen, T. (2006). Considering user attitude in early development of environmentally friendly technology: a case study of NoMix toilets. Environ. Sci. Technol. 40:4838. doi: 10.1021/es060075o
Liu, Y., Dai, Q., Jin, X., Dong, X., Peng, J., Wu, M., et al. (2018). Negative impacts of biochars on urease activity: high pH, heavy metals, polycyclic aromatic hydrocarbons, or free radicals? Environ. Sci. Technol. 52, 12740–12747. doi: 10.1021/acs.est.8b00672
Mayer, B. K., Baker, L. A., Boyer, T. H., Drechsel, P., Gifford, M., Hanjra, M. A., et al. (2016). Total value of phosphorus recovery. Environ. Sci. Tech. 50, 6606–6620. doi: 10.1021/acs.est.6b01239
Mazzei, L., Cianci, M., Benini, S., and Ciurli, S. (2019). The structure of the elusive urease–urea complex unveils the mechanism of a paradigmatic nickel-dependent enzyme. Angew. Chem. Int. Edn. 58, 7415–7419. doi: 10.1002/anie.201903565
Medeiros, D. L., Queiroz, L. M., Cohim, E., de Almeida-Neto, J. A., and Kiperstok, A. (2020). Human urine fertiliser in the Brazilian semi-arid: environmental assessment and water-energy-nutrient nexus. Sci. Total Environ. 713:13. doi: 10.1016/j.scitotenv.2019.136145
Mnaza, N., Nasir, R., Yasmeen, G., Muhammad, Z., Tariq, M., Khurshid, A., et al. (2015). Synthesis, Density Functional Theory (DFT), urease inhibition and antimicrobial activities of 5-Aryl thiophenes bearing sulphonylacetamide moieties. Molecules 20, 19914–19928. doi: 10.3390/molecules201119661
Mobley, H. L. T., and Hausinger, R. (1989). Microbial ureases: significance, regulation, and molecular characterization. Microbiol. Rev. 53, 85–108. doi: 10.1128/mr.53.1.85-108.1989
Montwedi, M., Munyaradzi, M., Pinoy, L., Dutta, A., Ikumi, D. S., Motoasca, E., et al. (2021). Resource recovery from and management of wastewater in rural South Africa: possibilities and practices. J. Water Process Eng. 40. doi: 10.1016/j.jwpe.2021.101978
Muirhead, D., and Carrier, C. (2012). “Comparison of four strong acids on the precipitation potential of gypsum in brines during distillation of pretreated, augmented urine: preliminary results,” in 42nd International Conference on Environmental Systems (AIAA) (San Diego, CA).
Nordin, A., Niwagaba, C., Jönsson, H., and Vinner?s, B. (2013). Pathogen and indicator inactivation in source separated human urine heated by the sun. J. Water Sanitat. Hyg. Dev. 3, 181–188. doi: 10.2166/washdev.2013.174
Olech, Z., Zaborska, W., and Kot, M. (2014). Jack bean urease inhibition by crude juices of Allium and Brassica plants. Determination Thiosulfinates. Food Chem. 145, 154–160. doi: 10.1016/j.foodchem.2013.08.044
Olofsson, M. (2016). Stabilization of Urine by Nitrification in a Moving Bed Biofilm Reactor (Master's thesis). Lund University, Stora. Available online at: http://lup.lub.lu.se/student-papers/record/8883081
Pahore, M. M., Ushijima, K., Ito, R., and Funamizu, N. (2012). Fate of nitrogen during volume reduction of human urine using an on-site volume reduction system. Environ. Technol. 33, 229–235. doi: 10.1080/09593330.2011.560192
Randall, D. G., Krähenbühl, M., Köpping, I., Larsen, T. A., and Udert, K. M. (2016). A novel approach for stabilizing fresh urine by calcium hydroxide addition. Water Res. 95, 361–369. doi: 10.1016/j.watres.2016.03.007
Ray, H., Perreault, F., and Boyer, T. H. (2019). Urea recovery from fresh human urine by forward osmosis and membrane distillation (FO–MD). Environ. Sci. 5, 1993–2003. doi: 10.1039/C9EW00720B
Ray, H., Saetta, D., and Boyer, T.H. (2018). Characterization of urea hydrolysis in fresh human urine and inhibition by chemical addition. Environ. Sci. Water Res. Technol. 4, 87–98. doi: 10.1039/c7ew00271h
Rezaei Behbehani, G., Saboury, A. A., Taherkhani, A., Barzegar, L., and Mollaagazade, A. (2011). A thermodynamic study on the binding of mercury and silver ions to urease. J. Therm. Anal. Calorim. 105, 1081–1086. doi: 10.1007/s10973-011-1729-9
Saetta, D., and Boyer, T. H. (2017). Mimicking and inhibiting urea hydrolysis in nonwater urinals. Environ. Ence Technol. 51, 13850–13858. doi: 10.1021/acs.est.7b03571
Saetta, D., Padda, A., Li, X., Leyva, C., Mirchandani, P. B., Boscovic, D., et al. (2019). Real-time monitoring and control of urea hydrolysis in cyber-enabled nonwater urinal system. Environ. Sci. Tech. 53, 3187–3197. doi: 10.1021/acs.est.8b06126
Schönning, C., Leeming, R., and Stenström, T. A. (2002). Faecal contamination of source-separated human urine based on the content of faecal sterols. Water Res. 36, 1965–1972. doi: 10.1016/S0043-1354(01)00427-4
Senecal, J. (2017) Urea stabilisation and dehydration for urine diverting toilets: system and hygiene evaluation (Master's thesis). SLU, Swedish University of Agricultural Sciences, Uppsala, Sweden.
Senecal, J., and Vinnerås, B. (2017). Urea stabilisation and concentration for urine-diverting dry toilets: urine dehydration in ash. Sci. Total Environ. 586, 137–144. doi: 10.1016/j.scitotenv.2017.02.038
Simha, P., Senecal, J., Nordin, A., Lalander, C., and Vinneras, B. (2018). Alkaline dehydration of anion-exchanged human urine: volume reduction, nutrient recovery and process optimisation. Water Res. 142, 325–336. doi: 10.1016/j.watres.2018.06.001
Stenström, T., Vinnerås, B., Jonsson, H., and Hoglund, C. G. (2000). Recycling Source Separated Human Urine. Stockholm: VAV AB.
Sutherland, C., Reynaert, E., Dhlamini, S., Magwaza, F., Lienert, J., Riechmann, M. E., et al. (2021). Socio-technical analysis of a sanitation innovation in a peri-urban household in Durban, South Africa. Sci. Total Environ. 755:143284. doi: 10.1016/j.scitotenv.2020.143284
Suzuki, S., Benno, K., Mitsuoka, Y., Takebe, T., Kobashi, S., Hase, K. (1979). Urease-producing species of intestinal anaerobes and their activities. Appl. Environ. Microbiol. 3, 379–382. doi: 10.1128/aem.37.3.379-382.1979
Tun, L. L., Jeong, D., Jeong, S., Cho, K., Lee, S., and Bae, H. (2016). Dewatering of source-separated human urine for nitrogen recovery by membrane distillation. J. Memb. Sci. 512, 13–20. doi: 10.1016/j.memsci.2016.04.004
Udert, K. M., Fux, C., Münster, M., Larsen, T. A., Siegrist, H., and Gujer, W. (2003a). Nitrification and autotrophic denitrification of source-separated urine. Water Sci. Technol. 48, 119–130. doi: 10.2166/wst.2003.0031
Udert, K. M., Larsen, T. A., Biebow, M., and Gujer, W. (2003b). Urea hydrolysis and precipitation dynamics in a urine-collecting system. Water Res. 37, 2571–2582. doi: 10.1016/S00431354(03)00065-4
Udert, K. M., Larsen, T. A., and Gujer, W. (2006). Fate of major compounds in source-separated urine. Water Sci. Tech. 54, 413–420. doi: 10.2166/wst.2006.921
Vij, S., Moors, E., Kujawa-Roeleveld, K., Lindeboom, R. E. F., Singh, T., and de Kreuk, M. K. (2021). From pea soup to water factories: wastewater paradigms in India and the Netherlands. Environ. Sci. Policy 115, 16–25. doi: 10.1016/j.envsci.2020.09.015
Vinnerås, B., Nordin, A., Niwagaba, C., and Nyberg, K. (2008). Inactivation of bacteria and viruses in human urine depending on temperature and dilution rate. Water Res. 42, 4067–4074. doi: 10.1016/j.watres.2008.06.014
Walker, J., and Hambly, F. J. (1895). LXXVII.—Transformation of ammonium cyanate into urea. J. Chemical Soc. Transact. 67, 746–767.
Wang, C., and Bao, W. (2007). Case study of vacuum urine-diverting sewerage system of SIEEB Tsinghua University. gewässerschutz wasser abwasser 206, 22.
Warner, R. C. (1942). The kinetics of the hydrolysis of urea and of Arginine. J. Biol. Chem. 142, 705–723. doi: 10.1016/S0021-9258(18)45072-7
Werner, E. A. (1918). XIII. - The constitution of carbamides. Part V. The mechanism of the decomposition of urea when heated in solution with alkalis and with acids respectively. The hydrolysis of metallic cyanates. J. Chem. Soc. Transac. 113, 84–99.
WHO (2018). World Health Statistics 2018: Monitoring Health for the SDGs Sustainable Development Goals. World Health Organization.
WWAP (UNESCO World Water Assessment Programme) (2019). The United Nations World Water Development Report 2019: Leaving No One Behind. Paris: UNESCO.
WWAP (United Nations World Water Assessment Programme) (2017). The United Nations World Water Development Report 2017: Wastewater, The Untapped Resource. Paris: UNESCO.
Xu, K. N., Qu, D., Zheng, M., Guo, X. H., and Wang, C. W. (2019). Water reduction and nutrient reconcentration of hydrolyzed urine via direct-contact membrane distillation: ammonia loss and its control. J. Environ. Eng. 145:8. doi: 10.1061/(ASCE)EE.1943-7870.0001496
Xu, W. S. (2008). Nitrification of human urine for its stabilization and nutrient recycling. Bioresour. Technol. 99, 6299–6304. doi: 10.1016/j.biortech.2007.12.007
Zhang, J., Giannis, A., Chang, V. W. C., Ng, B. J. H., and Wang, J.-Y. (2013a). Adaptation of urine source separation in tropical cities: process optimization and odor mitigation. J. Air Waste Manage. Assoc. 63, 472–481. doi: 10.1080/10962247.2013.763306
Zhang, Y., Li, Z., Zhao, Y., Chen, S., and Mahmood, I. B. (2013b). Stabilization of source-separated human urine by chemical oxidation. Water Sci. Tech. 67, 1901–1907. doi: 10.2166/wst.2013.055
Zhengping, W., Cleemput, O., Demeyer, P., and Baert, L. (1991). Effect of urease inhibitors on urea hydrolysis and ammonia volatilization. Biol. Fertil. Soils 11, 43–47. doi: 10.1007/BF00335833
Keywords: urea stabilization, urease inhibition, acidification, alkalization, electrochemistry
Citation: Yang W, Li J and Yang X (2021) Features and Applications of Urine Stabilization Methods: A Review. Front. Sustain. 2:710739. doi: 10.3389/frsus.2021.710739
Received: 17 May 2021; Accepted: 13 August 2021;
Published: 10 September 2021.
Edited by:
Wafaa Mohamed Moustafa Salem, Egyptian Atomic Energy Authority, EgyptReviewed by:
Lock Hei Ngu, Swinburne University of Technology Sarawak Campus, MalaysiaAnnalisa Paolone, Istituto dei Sistemi Complessi, Italy
Copyright © 2021 Yang, Li and Yang. This is an open-access article distributed under the terms of the Creative Commons Attribution License (CC BY). The use, distribution or reproduction in other forums is permitted, provided the original author(s) and the copyright owner(s) are credited and that the original publication in this journal is cited, in accordance with accepted academic practice. No use, distribution or reproduction is permitted which does not comply with these terms.
*Correspondence: Xuejing Yang, eGoueWFuZyYjeDAwMDQwO2VjdXN0LmVkdS5jbg==