- 1The Joint Graduate School of Energy and Environment, King Mongkut's University of Technology Thonburi, Bangkok, Thailand
- 2Center of Excellence on Energy Technology and Environment, Postgraduate Education and Research Development Office (PERDO), Ministry of Higher Education, Science, Research and Innovation, Bangkok, Thailand
- 3Faculty of Science and Technology, Thammasat University, Bangkok, Thailand
- 4Department of Environmental Engineering, Faculty of Engineering, King Mongkut's University of Technology Thonburi, Bangkok, Thailand
Municipal solid waste (MSW) generation has been escalated at a global scale and poses drastic impacts on the environment along with many socio-economic problems. Waste to energy (WtE) technologies have been recognized to convert MSW into useful energy and minimize the problems related to it. This study reviewed different WtE technologies according to the conversion pathways, end-products, and their applications, and assessed statistical values of these technologies based on six different factors, viz., environmental performance, suitable waste fractions, capital and operational cost, efficiency, and complexity of the technology, the skillset of the labor, and favorable geographical location for the plant. The results of this review showed that biochemical and physicochemical WtE technologies are more favorable to convert organic waste, while thermochemical WtE technologies are suitable to process combustible fractions of organic and inorganic MSW. Based on the statistical review of considered factors from the literature, the statistical profiles of concerned WtE technologies were observed. Finally, a general framework in the form of a systematic scheme was proposed for the selection of the most suitable WtE technologies for a sustainable MSW management system. The recommended indicators, methods, and models in the proposed framework were selected after a detailed review of the literature published in well-known scientific journals, and reports of leading international organizations such as the World Bank, International Energy Agency (IEA), and International Labour Organization (ILO). Moreover, the databases to extract the data for the estimation of various recommended indicators have also been presented.
Introduction
Municipal solid waste (MSW) generation is continuously rising on a global scale. According to the World Bank, the global annual MSW generation was 2.01 billion tons in 2018, up from 1.3 billion tons in 2012. It is expected that the global annual MSW generation rate will increase up to 2.59 billion tons by 2030 and 3.40 billion tons by 2050 (Kaza et al., 2018; Mayer et al., 2019). This large increase in MSW generation is identified as a repercussion of different factors including economic growth, population expansion, industrial development, urbanization, and rural to urban migration, etc. (Moya et al., 2017b; Kaza et al., 2018; Mayer et al., 2019). Along with the bulking up of waste volumes, the composition of MSW is also becoming more heterogeneous and complex because of the development of modern economies that are highly consumer-based lifestyle centric (Moya et al., 2017b; Yadav and Samadder, 2017; Tsui and Wong, 2019).
The heterogeneity and complexity of MSW composition are causing great difficulty in sustainable disposal of this gigantic amount of waste that also causes many economic losses and poses drastic impacts on the environment and human health (Menikpura et al., 2012; Noya et al., 2018; D'Adamo et al., 2020). It has been recognized that different income level groups generate different waste compositions and quantities (Agamuthu et al., 2007; Kaza et al., 2018). Yadav and Samadder (2017) have estimated the annual MSW generation growth rate for both low-income countries and high-income countries as 2–3 and 3.2–4.5%, respectively. The difference between the MSW generation growth rate of different income groups showed that the group with greater purchasing power generates more waste is because developing countries are rapidly shifting toward industrialization (Mayer et al., 2019). With regards to the waste composition, high-income countries generate more dry waste including paper, plastic, cardboard, glass, metal, etc. that is comparatively easy to recycle, whereas more than 50% of the total MSW generation in low-income countries is organic waste which is much more difficult to manage (Kaza et al., 2018). Alongside the proliferated dilemma of MSW generation and its sustainable management, global energy demand has also increased.
The power generation sector and transportation sector are the top two most energy-intensive sectors around the globe. Most of the energy needs in these sectors are fulfilled by expensive fossil fuels (Farooq et al., 2020). The combustion of fossil fuels poses a direct negative impact on the environment that causes harm to our ecosystem (Nieuwlaar, 2013). Moreover, these conventional energy resources are rapidly depleting and threatening energy security at a global scale (Gumisiriza et al., 2017; Soleymani and Rosentrater, 2017). On the other hand, the utilization of renewable energy resources for heat, power, and different types of biofuels production has become a high priority both in national and global energy policies (Cucchiella et al., 2014). MSW has been highly considered as a renewable energy source (Mutz et al., 2017). The utilization of MSW as an energy resource can reduce the drastic environmental impacts of improper waste management practices and fossil-based electric power generation (Ayodele et al., 2017). Waste to energy (WtE) plants can convert this cheap and readily available renewable energy resource into useful energy. Therefore, WtE can be a potential solution to empower global energy security by offsetting the fossil fuels dominance in the world's energy sector (Khan and Kabir, 2020). The term WtE refers to the treatment of waste for energy recovery in the form of heat and electricity or other alternative fuels in gaseous, liquid, and solid forms. A vast range of WtE technologies is available to produce such a diverse stream of end-products from the complexly composed feedstock, i.e., MSW (Mutz et al., 2017; Beyene et al., 2018).
MSW management is a multidisciplinary and interdisciplinary field of research. Researchers from fields of economics, engineering, chemistry, physics, sociology, geography, psychology, and law, etc., have been showing a huge interest to explore the facts related to MSW management studies. Many studies have been done to propose a selection criterion for WtE technologies. Yap and Nixon (2015) conducted a study in which they used the analytical hierarchical process integrated with benefits, opportunities, costs and risks analysis approach to identify the most favorable WtE technology(s) by evaluating the tradeoffs between benefits and opportunities, and costs and risks among the considered technologies (mass burn incineration, refused derived fuel incineration, gasification, anaerobic digestion, and landfill gas recovery). The scope of their study was limited to set out the best WtE choices for India and United Kingdom. Moreover, the decision was made only on the basis of benefits and opportunities of a particular WtE technology over the other considered technologies, while the influence of socio-economic and technological situation of the given countries (India and United Kingdom) on their WtE industries was not ratiocinated. Soltani et al. (2016) also proposed a decision framework by incorporating environmental, economic, and social aspects only. They used life cycle assessment (LCA) and life cycle cost analysis for environmental and economic evaluation, respectively, and developed a weighting scheme with the help of the MCDM analysis approach to aggregate the outcomes of environmental and economic evaluation. After that they used game theory to involve the multiple stakeholders in the decision making and execution process to avoid the issue of free riders in the WtE industry. The study focused on the waste management system of Vancouver, Canada that represented only the developed world. A study performed by Qazi et al. (2018a) assessed the prioritization of different WtE technologies by using the multi criteria decision making (MCDM) analysis approach for the selection of the most optimum options. However, their study was limited to the most suitable WtE options only for the Sultanate of Oman. Recently, another study by Kurbatova and Abu-Qdais (2020) reviewed the status of MSW management and energy sector of Russian capital city Moscow and its suburbs for the selection of best WtE options for the Moscow region. Analytical hierarchical process method was used to develop a decision model by incorporating three criteria viz., environmental, technical, and socio-economic. The scope of their study was only limited to a specific region (Moscow region). However, the study did not include the territorial aspect. Recent studies proposing the selection criteria for most appropriate WtE technologies did not include the socio-economic and technological indicators which depict the actual status of any country or region's economy. Inclusion of socio-economic and technological indicators in the selected framework may help the policy makers to take reality-based decision regarding sustainable MSW management in any given region. Further, all the reviewed studies were focused on selecting the most feasible WtE options only for a specific area, and did not develop a general framework or selection criteria that can aid policy makers to take decisions regarding selection of WtE technologies for sustainable MSW management in any given country or region (Shahnazari et al., 2020). Furthermore, no previous study incorporated the territorial aspects along with the environmental, socio-economic, and technological factors associated with WtE technologies to choose the best suitable option(s). A famous French geologist Jean Gouhier introduced a new subfield of geology termed rudology (the systematic study of waste management regarding territorial implications). He also created a rudology institute at the University of Maine. However, the territorial aspect of waste management has been neglected because this field is comparatively new and less explored (Mihai, 2012). Therefore, the main goal of this study was to develop a general framework for the selection of the most suitable WtE technologies which will incorporate environmental, socio-economic, technological, and territorial aspects associated with WtE technologies. To achieve that goal, the well-established WtE technologies available in the literature were reviewed. Secondly, the statistical values of different socio-economic and technological factors, as well as the environmental performance and territorial preference of different WtE technologies, were also reviewed from the literature. Finally, various environmental, socio-economic, and technological indicators coupled with well-acknowledged methods and models available in the literature were reviewed to introduce a general framework that may help the policy makers to select the most suitable WtE technology(s) for a sustainable MSW management system in any given region.
The adopted methodological approach to achieve the aims and objectives of the study is explained in the next section. In section Review of WtE Technologies, the well-established WtE technologies are reviewed regarding conversion pathways, end products, application of the end products and merits/demerits of the WtE technologies. In section Assessment of Socio-Economic, Technological, and Environmental Factors of WtE Technologies, the concerned WtE technologies are assessed based on six different factors to observe the environmental, socio-economic, technological, and territorial aspects. Moreover, a review of statistical values of observed factors is also presented. After that well-established socio-economic and technological indicators, and academically acclaimed methods and models are identified and explained in section Indicators for Selection of Suitable WtE Technologies. Section Schematic of the Proposed Framework presents the systematic scheme of newly proposed general selection framework; the policy implications and limitations being covered in section Policy Implications and Limitations of the Proposed Framework. Finally, the conclusions and recommendations of the study are presented in section Conclusion and Recommendations.
Methodology
There are many different WtE technologies available in the market. Each WtE technology uses a different fraction regarding composition of MSW as a feedstock, following different conversion pathways, and producing different kinds of end-products. The method adopted for this study has been divided into three steps as follows:
• In the first part, different WtE technologies were reviewed, according to the conversion pathways, end-products, and their applications. Moreover, the merits and demerits of each concerned WtE technology were also identified.
• Secondly, the reviewed WtE technologies were assessed based on six different factors to observe their performance regarding environmental, socio-economic, technological, and territorial aspects. These factors included the environmental performance, the origin and type of waste, capital and operational cost, WtE conversion efficiency and complexity of the technology, the skillset of the labor, and the favorable geographical location for the plant. Further, a review of statistical values of the observed factors was also performed.
• Finally, various environmental, socio-economic, and technological indicators coupled with well-acknowledged methods and models available in the literature were reviewed to develop a general framework that may help the policy makers to select the most suitable WtE technology(s) for a sustainable MSW management system in any given region. All the reviewed indicators, methods and models are discussed in detail in section Indicators for Selection of Suitable WtE Technologies. After that a general selection criterion was developed by arranging the identified indicators, methods and models in a systematic scheme that can easily guide the policy makers and stakeholders to identify the most suitable WtE technologies for sustainable MSW management.
The framework of the whole adapted methodology has been summarized in Figure 1.
Review of WtE Technologies
There are three main WtE conversion pathways, viz., thermochemical, biochemical, and physicochemical (Ouda et al., 2016; Tozlu et al., 2016; Moya et al., 2017a). However, many different types of WtE process technologies are available, and each process technology follows one of the three conversion pathways mentioned above as shown in Figure 2 (DOE, 2019; Shahnazari et al., 2020). In the following section, the description of the technology, types of end-products, the application of these end-products falling under all three conversion pathways have been explained briefly, followed by the merits and demerits of each WtE technology which have been explained in Table 1.
Thermochemical Technologies
Thermochemical conversion needs thermal energy to break down the molecular structure of MSW components and convert larger molecules into smaller molecules. After that additional oxygen is provided which combines with the hydrogen and carbon atoms released from the decomposed larger waste molecules and produces more energy than it provided to break the complex molecular structure of MSW components (Qazi et al., 2018b). The technologies which adapt thermochemical conversion pathways, use very high temperature to convert different fractions of MSW into heat, electricity, and other value-added products (DOE, 2019; Shahnazari et al., 2020). These technologies include incineration, pyrolysis, and gasification. In the following section, these thermochemical WtE technologies are briefly explained.
Incineration is a well-established and the most commonly used WtE technology globally. This technology is proficient in completely combusting the organic components present in MSW to reduce its volume and convert it into heat and power. Moreover, regarding energy, economic and environmental (3Es) impacts of WtE technologies, incineration is the best solution when combined heat and power (CHP) generation is concerned (Cucchiella et al., 2017). An incinerator plant operates at a very high temperature, about 850–1,100°C. The main components of an incineration plant are the feeding system, incinerator (combustion chamber), exhaust gas system, and residual disposal system. The most commonly known incineration plants are industrial incinerators and mass burn, etc. (Tan et al., 2015; Ouda et al., 2016; Cucchiella et al., 2017; Qazi et al., 2018b; Shahnazari et al., 2020). The main outputs of an MSW incineration plant are heat and hot flue gas which consist of carbon dioxide (CO2), nitrogen N2, oxygen O2, water vapor (H2O), etc. Moreover, ash (both bottom ash and fly ash) is also considered a significant end-product, because the total ash weighs around 20–25% of the total feedstock (Atwadkar et al., 2015; Tan et al., 2015; Qazi et al., 2018b). The heat and hot flue gases produced in the incineration process are used to produce high-pressure steam which is used in a steam turbine to generate electricity (Tan et al., 2015). After that, the flue gases are treated in a flue gas treatment plant to reduce toxicity for controlling environmental pollution (Jurczyk et al., 2016). The ash produced during the incineration process may end up in two ways. It can be used as secondary raw material in the cement industry or can be disposed of in landfills (Qazi et al., 2018b).
Pyrolysis is a thermal process that is performed in the absence of oxygen. The pyrolysis process can recover up to 80% of the energy from the carbonaceous fraction of MSW (Ouda et al., 2016). There are three different types of pyrolysis reactions depending on temperature, heating rate, particle size, and residence time. These reactions are slow, fast, and flash pyrolysis (Qazi et al., 2018b). The operating parameters for all three pyrolysis reactions are also different. These parameters for slow, fast and flash pyrolysis are temperature 500–950, 850–1,250, 1,050–1,300°C; residence time 450–550 s, 0.5–10 s, < 0.5 s; particle size 5–50 mm, < 1 mm, < 0.2 mm; and heating rate 0.1–1, 10–200, 200–1,000°C/s, respectively. The most commonly used technology for pyrolysis reaction is fluidized bed reactors (Balat et al., 2009; Jahirul et al., 2012; Shahnazari et al., 2020). This technology converts the carbonaceous fractions of MSW into gaseous, liquid, and solid fuels (char). The yield of each type of fuel is different for all three (slow, fast, and flash) pyrolysis reactions due to different operating parameters. The percentage share of liquid, solid, and gaseous fuels production for slow pyrolysis is 30, 35, 35%; for fast pyrolysis is 50, 20, 30%; and for flash pyrolysis is 75, 12, 13%, respectively (Balat et al., 2009; Jahirul et al., 2012; Qazi et al., 2018b). All three types of fuels (gaseous, liquid, and solid) produced in the pyrolysis process have properties very similar to fossil-based fuels. Pyrolysis gas is very rich in ethylene that can be used for alcohol/gasoline production. All three pyrolysis fuels can be used for heat and power generation purposes directly (Balat et al., 2009; Marshall et al., 2014; Qazi et al., 2018b).
Gasification is a modified pyrolysis process where the reaction happens in the presence of a limited amount of oxygen or steam (Tozlu et al., 2016; Shahnazari et al., 2020). It is indirect combustion, as during the gasification process an exothermic reaction occurs and thermal energy is produced as a result of carbon and oxygen reaction (Ouda et al., 2016). The whole process takes place at a very high temperature of around 800°C. Normally, the yield of gasification products is very high (up to 85%). However, several parameters are involved in determining the gasification efficiency, viz., temperature, feedstock particle size, moisture content, and gasification agent. The temperature in the gasifier should be between 500 and 1,000°C, the feedstock particles should be very small and uniform in size, the moisture content should be below 15% (Qazi et al., 2018b). There are eight different reactors available that can be used for gasification reactions. These reactors are rotary kiln, updraft fixed bed reactor, downdraft fixed bed reactor, bubbling fluidized bed reactor, entrained flow bed reactor, plasma reactor, vertical shaft, and moving grate furnace (Arena, 2012; Moya et al., 2017b). This technology converts plastics and combustible organic fractions of MSW into clean and very useful syngas or synthesis gas. The major components of syngas are carbon monoxide (CO) and Hydrogen (H2). Along with CO and H2, smaller amounts of carbon dioxide (CO2), water vapor (H2O), nitrogen (N2), and methane (CH4) are also found (Lombardi et al., 2012; Ouda et al., 2016; Qazi et al., 2018b). The clean syngas produced in the gasification process can be used directly in a gas turbine to produce combined heat and power (CHP) or can be used as valuable transportation fuels. Moreover, it can also be used as secondary raw material in fertilizers and chemical industries (Ouda et al., 2016; Moya et al., 2017b; Qazi et al., 2018b).
Biochemical Technologies
Biochemical conversion of MSW to energy involves biological agents or micro-organisms such as yeast, to convert the organic fraction of the waste into gaseous or liquid biofuels. Anaerobic digestion and fermentation are the WtE technologies that follow the biochemical conversion pathway (Ouda et al., 2016; Qazi et al., 2018b).
Anaerobic digestion is the process that decomposes the organic fraction of MSW with the help of micro-organisms in the absence of free oxygen. The microbes involved in different stages (acidogenesis, acetogenesis, and methanogenesis) of the anaerobic digestion process are very sensitive to pH level and need specific conditions to grow and boost the yield of end products. Therefore, this process takes place in special reactors that operate at specific conditions that include well-maintained temperature and pH level (Weedermann et al., 2013; Chiu et al., 2016; Bajpai, 2017; Qazi et al., 2018b). The pH level should be maintained between 6.7 and 7 according to the microbes used in the corresponding stage of the process while there are three different ranges for temperature, viz., <25°C (psychrophilic), 35–48°C (mesophilic), and >50°C (thermophilic) conditions. Mostly, mesophilic or thermophilic conditions are preferred as these conditions are economically viable (Weedermann et al., 2013; Mutz et al., 2017). The organic feedstock is mixed well and kept in the digester for 5–10 days and during this time the anaerobic digestion process takes place in four different phases, viz., hydrolysis, acidogenesis, acetogenesis, and methanogenesis. An additional phase called sulfur reduction is also included. It is introduced to reduce the sulfur content in the final product for quality control purposes. However, this step also reduces the final yield of the end product (Tan et al., 2015; Qazi et al., 2018b). There are different types of reactors for different types of feedstock. For food waste, continuously stirred tank reactors are preferred, while for other types of organic waste, plug-flow and batch reactors are used (Mutz et al., 2017). There are three different end products generated from the anaerobic digestion process. The main product is biogas which contains 50–80% methane (CH4), 20–50% carbon dioxide (CO2), and small traces of sulfide and ammonia. The other two products that are generated along with biogas, are fiber and liquid digestate (Vindis et al., 2009; Sitorus et al., 2013; Chiu et al., 2016; Mutz et al., 2017; Qazi et al., 2018b). The biogas produced in the anaerobic digestion process can be used to replace natural gas in CHP generation. However, the efficiency of heat and power production from biogas is around 5.5–7.5 kWh/m3 which is less as compared to natural gas. This is because the calorific value of biogas is about two-third that of natural gas (Mutz et al., 2017; Qazi et al., 2018b). The liquid digestate and fiber produced in the anaerobic digestion process can be used as secondary raw material in the fertilizers industry (Qazi et al., 2018b).
The fermentation process also uses microbes for the decomposition of organic materials in an oxygen-free environment (Qazi et al., 2018b). It is a metabolic process that is carried out in non-sterilized conditions by using yeast or bacteria to convert sugar into alcohol (Moukamnerd et al., 2013; Kumar and Samadder, 2017). The fermentation process also includes almost all the phases similar to anaerobic digestion, except the methanogenesis stage. Therefore, the end product of fermentation is liquid biofuel rather than biogas. The fermentation process ends at acetogenesis where diluted alcohol is formed which is separated from the fermentation digestate by performing an additional step known as distillation (Qazi et al., 2018b). There two different ways to perform the fermentation process, viz., continuous or batch process. In the continuous process, fermentation is carried out by keeping a balance between feedstock load and discharge rate which means that the same amount of feedstock is loaded into the reactor, as the quantity discharged. Therefore, the initial investment and capital and operation costs for continuous fermentation process are relatively lower as compared to batch process because it needs smaller reactor volumes to use larger amounts of feedstock (Abreu-Cavalheiro and Monteiro, 2013). On the other hand, the batch process yields a larger quantity of end product as compared to the continuous process. Moreover, the payback period is also shorter for a batch fermentation reactor (normally 1 year) as compared to continuous fermentation (Lopes et al., 2016). The main output from the fermentation process is ethanol (Qazi et al., 2018b). After performing the distillation process by using advanced technology such as a molecular sieve, 99.99% pure fuel-grade biofuel (ethanol) can be obtained (Farooq et al., 2020). Other than ethanol, CO2, distilled-dried grains (DDGs), and stillage (wastewater) are also obtained as by-products from the fermentation process (Sorapipatana and Yoosin, 2011). The ethanol produced by the fermentation process can be used to replace gasoline as a transportation fuel. CO2 produced during the fermentation process can be liquefied and sold as dry ice to confectionaries or processed food industries to generate extra revenue (Farooq et al., 2020). DDGs can be used as a raw material in the cattle feed industry while stillage can be processed further in an anaerobic digestion plant to produce biogas (Sorapipatana and Yoosin, 2011).
Physicochemical Technologies
Transesterification is the most famous WtE technology that follows the physicochemical conversion pathway and converts food waste, specifically used cooking oils (UCO) and animal fats into liquid fuels (Shahnazari et al., 2020). Transesterification is the process that converts the fat fractions of food waste in the MSW stream into biodiesel. The fat fractions include UCO from restaurants and households, and also the inedible parts of animals (blood, fat, and internal organs) from slaughterhouses (Li et al., 2012; Shahzad et al., 2017). To perform the transesterification process, UCO is collected and then undergoes screening and dehydration to remove impurities and moisture. On the other hand, slaughterhouse waste is also processed in a rendering facility where the inedible animal parts are converted into fat with one more value-added product as meat and bone meal. The processed fat then undergoes transesterification reaction with monoalkyl alcohols, viz., ethyl, or methyl alcohol in the presence of acid or base catalyst to produce biodiesel. Potassium hydroxide (KOH) or carbonic acid (H2CO3) is the commonly used catalysts in transesterification reaction (Gardy et al., 2014; Nizami et al., 2016). The selection of catalysts depends on the amount of free fatty acids (FFA) in the feedstock. Normally, FFA content in rendered animal fat is up to 20% while it is just 15% in the UCO. The reaction time for alkane-catalyzed reaction is faster (half an hour) as compared to acid-catalyzed (1–8 h) (Sharma et al., 2008; Thamsiriroj and Murphy, 2010). However, the yield of end product from the acid-catalyzed transesterification process is higher as compared to alkane-catalyzed but the reactor has to stay under a corrosive environment for a longer period that can end up in higher maintenance cost. The solution to this problem is that the transesterification process can be performed in two steps to handle feedstock with higher FFA content. In the first step, an acid catalyst can be applied to an esterification reaction to convert the FFA into biodiesel. After that alkane catalyst can be applied through transesterification reaction to convert remaining triglycerides into biodiesel (Gerpen, 2005). Biodiesel is produced as the main end product from the transesterification process while glycerol is also produced as a by-product. The quality and quantity of produced biodiesel depend on the amount of unsaturated fatty acids present in the feedstock. The higher the amount of unsaturated fatty acids in the feedstock, the higher is the quality and quantity of produced biodiesel. While in the case of higher concentration of saturated fatty acids in feedstock, both the quality and yield of biodiesel are low (Shahzad et al., 2017). The biodiesel produced from UCO and animal fat can replace petrochemical diesel and can be used as vehicle fuel (Li et al., 2012). Moreover, both biodiesel and the glycerol that is produced as a by-product can be used for electricity production because their higher heating values (HHV) are very high at 40.17 MJ/kg and 19 MJ/kg, respectively. The meat and bones meal obtained from the rendering process can be used as a raw material in the livestock feed industry or can be burnt for energy recovery as well (Shahzad et al., 2017).
Assessment of Socio-Economic, Technological, and Environmental Factors of WtE Technologies
In this section, the above reviewed WtE technologies have been assessed based on six different factors including (1) environmental performance, (2) suitable waste fractions, (3) capital and operational cost, (4) WtE conversion efficiency and complexity of the technology, (5) skillset of the labor, and (6) suitable geographical location for the assessment of socio-economic, technological, and environmental statistics of reviewed WtE technologies.
Environmental Performance of WtE Technologies
Environmental assessment tools are gaining more importance due to the increasing concern of including environmental impacts of different products and production systems for the sake of sustainable development in industry. LCA is the one out of many tools that can be considered as the broadest environmental assessment tool (Shahbazi et al., 2019). It is a valuable tool to document the environmental considerations of life cycle of a product, service, commodity, or a project that need to be a part of decision making toward sustainability. LCA assesses the significant environmental aspects and their impacts from extraction to production, use and end of life stage, hence consider the whole life cycle—from cradle to grave (from raw material acquisition through production, use, and disposal). LCA helps to avoid the problem shifting across the different stages of a product system by taking into account the upstream and downstream processes and extends the focus beyond the physical boundaries. It also quantifies all relevant emissions, consumed and depleted resources, and include multiple impact categories associated to every stage of product life cycle. This feature of LCA helps to identify the weak points and hotspots stages which can potentially be improved. The outcomes of LCA highly complement the techno-economic measurements and aid policy makers to take decision to achieve high level of sustainability (Chaya and Gheewala, 2007; Pandyaswargo et al., 2012; Tong et al., 2018; Shahbazi et al., 2019). In a recent couple of decades, LCA has been broadly used for the environmental assessment of the WtE technologies both as stand-alone technologies or as a part of an integrated MSW management system (Astrup et al., 2015). In the literature, several studies have adopted LCA to compare the environmental profiles of different WtE technologies. Some of these studies have been reviewed in Table 2. The selected WtE technologies in the reviewed studies were chosen due the environmental competitiveness after performing a detailed LCA in all the cases. Menikpura et al. (2016) performed both LCA and life cycle cost analysis to select the technologies which were superior regarding both environmental and economic performance. Life cycle cost analysis is a life cycle-based economic assessment tool, used to determine the aggregate cost incurred during the whole expected life cycle of a good, service, asset, system, or a facility. In the recent years, life cycle cost analysis has become a well-recognized method for cost management and financial planning due to the rising demand for accountability, cost-effectiveness, perceptible return on investments and firm reasons for the acquisition of a commodity in the society (Luo et al., 2009; Hui and Mohammed, 2015).
The outcomes of the reviewed studies have been used to identify the WtE technology(s) which are relatively competent regarding environmental performance. It can be seen from Table 2, that not a single reviewed study covered all the considered WtE technologies for comparing the environmental competency of these techniques over one another because of possible variations in the waste composition, geographical location, technical aspects, and framework conditions. Therefore, it is not easy to select a particular WtE technology over another regarding environmental performance. However, some general observations can be made such as the studies which considered anaerobic digestion and/or gasification alongside other WtE technologies in an MSW management system; these two technologies were found to be the best choice among the concerned technologies (Zaman, 2009, 2010; Astrup et al., 2015; Dong et al., 2018a,b; Mayer et al., 2019). Moreover, when anaerobic digestion is considered to be integrated with thermochemical technologies then the preference order of integrated systems was found as anaerobic digestion integrated with gasification and anaerobic digestion integrated with incineration, respectively (Ayodele et al., 2017; Tong et al., 2018). From these observations, it can be concluded that anaerobic digestion and gasification are comparatively more competent WtE technologies regarding environmental performance.
Suitable Waste Fractions for WtE Technologies
According to the 2019 refinement to the 2006 IPCC guidelines for national greenhouse gas inventories, the composition of MSW is divided into 11 different components. These components include food waste, garden waste, paper/cardboard, wood, textiles, absorbent hygiene products (diapers, nappies, and sanitary napkins, etc.), rubber/leather, plastic, metal, glass, and others (soil, dust, bricks, etc.) (IPCC, 2019). These MSW fractions are generally divided into two main waste streams, viz., biogenic or biodegradable, and non-biogenic or inorganic fractions. The biodegradable waste fraction includes food waste, garden waste, wood, and paper/cardboard components in MSW streams. On the other hand, plastic and rubber waste are considered combustible organic fractions in the MSW waste stream (Horttanainen et al., 2013). The non-combustible inorganic waste fractions (such as metals, glass, dust, soil, and bricks, etc.) are not accounted to process under any WtE technology because these fractions result in the formation of solid slag or bottom ash. This solid slag needs to be removed from the bottom of the combustion chamber before carrying on the combustion process, by using an electromagnetic belt that requires additional energy input. Therefore, the non-combustible components are separated from the MSW streams before energy recovery with the help of mechanical and biological treatment (MBT) for resource recovery and recycling purposes (Qazi et al., 2018b). Some of the waste components contain both biogenic and non-biogenic (inorganic) fractions. These components include textile, leather, and nappies (Horttanainen et al., 2013). However, a significant proportion of MSW consists of more than 75 percent moisture. This property of MSW is unfavorable for the WtE technologies which depend on the application of heat to convert the waste into energy because a huge amount of energy is lost for removing the moisture content present in the waste feedstock (DOE, 2019). Another very important feature of waste components is the energy content or calorific value. The heating values of different waste fractions are presented in Table 3. The energy content or calorific values for different fractions of MSW is different but generally, the heating value of combustible MSW falls within the range of 7 MJ/kg to 12 MJ/kg (Moora et al., 2017). One more drawback of the diverse elements of waste composition is that it contains higher levels of nitrogen, sulfur, and ash as compared to other lignocellulosic biomass feedstocks. For example, the nitrogen and sulfur content of biodegradable or biogenic fractions (food and garden waste) in MSW waste stream can be 20 times higher than corn cobs and pine shells. Moreover, the non-biodegradable or inorganic fractions of MSW contain a very high concentration of chlorine that can produce dioxins during the combustion process (DOE, 2019). Moya et al. (2017a) estimated the energy recovery potential and power generation potential of the biodegradable and non-biodegradable fraction of MSW fractions for biochemical and thermochemical processes. They found that both biodegradable and non-biodegradable fractions of MSW can be used to recover energy and generate power via thermochemical process while for energy recovery through biochemical processes, only the biodegradable waste fraction can be used (Moya et al., 2017a). The recommended waste types for different WtE technologies are presented in Table 4. It can be seen from Table 4 that the WtE technologies that follow biochemical and physicochemical conversion pathways can only use organic waste as a feedstock and convert it into different types of liquid and gaseous fuels. On the other hand, the technologies which follow the thermochemical conversion can utilize most of the waste components in MSW waste streams, and can directly convert this waste into combined heat and power and some technologies also produce solid, liquid, or gaseous fuels as value-added products (Ouda et al., 2016; Cucchiella et al., 2017).

Table 3. Gross calorific values (GCV) of MSW waste components (Horttanainen et al., 2013; Ozbay and Durmusoglu, 2013).
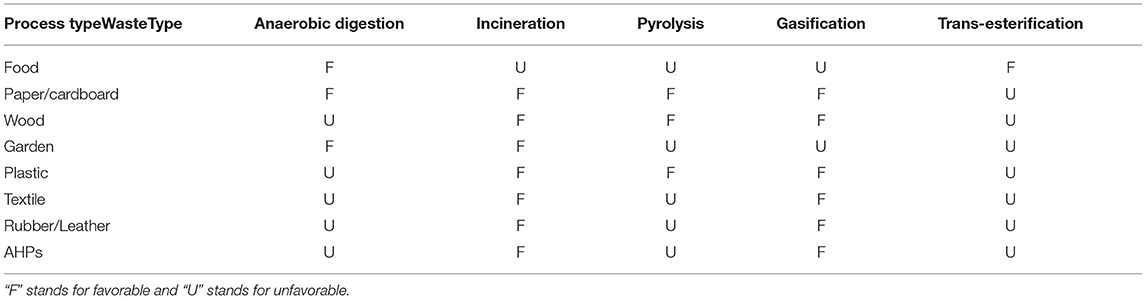
Table 4. Recommendation of waste types for different WtE Technologies (Ouda et al., 2016).
Review of Statistical Values of Socio-Economic and Technological Factors
The statistical values of socio-economic and technological factors of concerned WtE technologies have been referenced from reviewed literature. The capital and operational cost, per day power generation, efficiency, complexity level, required skill level, and suitable geographical location for each reviewed WtE technology has been presented in Table 5. From the observed historic statistical values of different socio-economic and technological factors, it can be seen that biochemical technologies are the cheapest options, while the capital and operating costs of incineration technology were found to be cheaper than the other thermochemical technologies. The capital and operating costs of the transesterification plant were found to be the highest among the concerned WtE technologies. The efficiency of converting waste into useful energy is the highest for the transesterification process followed by gasification, pyrolysis, and biochemical technologies. The conversion efficiency was the lowest for incineration. On the other hand, the level of complexity was found to be low for transesterification, biochemical technologies, and incineration, while pyrolysis and gasification were found as the most complex technologies. Similarly, a low level of labor skills is required for incineration, transesterification, and biochemical technologies while an intermediate level of skills is required for pyrolysis and gasification. Moreover, the pyrolysis and gasification technologies are suitable for the urban areas which are more industrially developed, whereas incineration and transesterification technologies are preferable for normal urban locations. Only biochemical technologies were found as the best choice for the less developed (rural) areas. It is clear from the above discussion and review of statistical values that all these WtE technologies use different fractions of waste as input, use different conversion pathways, produce different end products having diverse applications. Moreover, all these WtE technologies have many advantages and some limitations as well. Therefore, the selection of suitable WtE technology(s) is highly challenging for any country, and it depends upon the composition of MSW, economic, technological, and urban development, and national waste management policy. Therefore, there is a need to assess the above-mentioned socio-economic, technological, and environmental factors by using country-specific socio-economic and environmental indicators, and well-established methods and models for the selection of the most suitable WtE technologies to support the MSW management policy in any given country. In the following section, a set of different socio-economic indicators and academically acclaimed methods and models is proposed as a general selection framework.
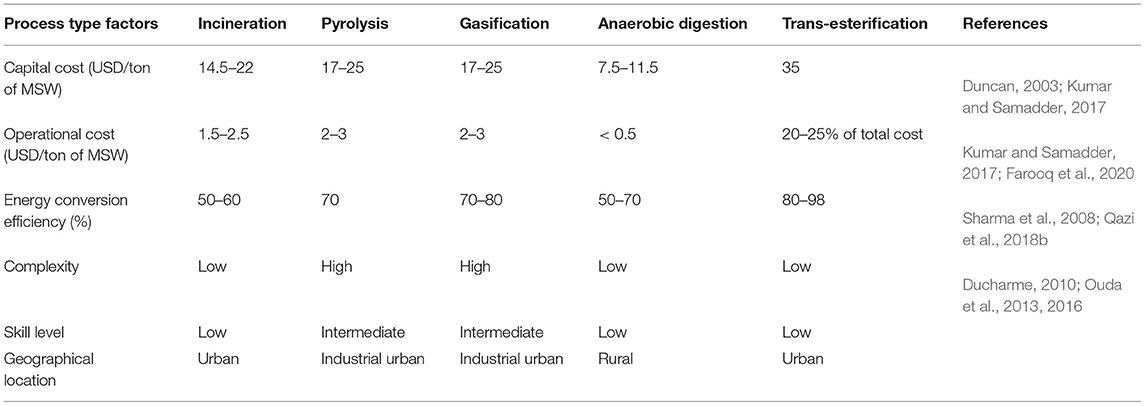
Table 5. Comparison of WtE technologies based on different socio-economic and technological factors.
Indicators for Selection of Suitable WtE Technologies
In the previous section, all the reviewed WtE technologies have been assessed regarding environmental performance, suitable waste fractions as feedstock, level of labor skillset required, and favorable geographical location for each technology. Moreover, the level of complexity, efficiency, and capital and operating costs of each technology were also defined. In this section, different socio-economic and environmental indicators, and methods are introduced for the assessment of concerning factors according to the country-specific socio-economic situation.
Estimation of Environmental Performance
Conventional WtE technologies have been highly criticized due to increased environmental concerns by both policymakers and the public. Therefore, there is a need to explore more advanced WtE technologies that pose minimum impacts on the environment and human health. LCA is the most commonly used environmental assessment tool for evaluating the environmental performance of any system or process technology. In the recent past, there was a huge interest in the application of LCA for the evaluation of environmental impacts of different WtE technologies (Liamsanguan and Gheewala, 2008; Mubeen and Buekens, 2019). However, the LCA of WtE technologies in an MSW management system is not an easy task. To perform a holistic environmental evaluation of an MSW management system, all the life cycle stages of the system should be considered, including the energy and material recovery from the WtE technologies that can offset the environmental burden of energy and material production from virgin resources (Liamsanguan and Gheewala, 2008). To facilitate a holistic environmental assessment of WtE technologies, Menikpura et al. (2012) introduced a detailed framework for the sustainability assessment of MSW management systems that can be used to assess the environmental sustainability performance of different WtE technologies in a single MSW management system. The framework proposed by Menikpura et al. (2012) was based on life cycle thinking. They developed a clear methodology via life cycle thinking and also developed a set of composite indicators that deal with all three themes (environment, society, and economics) of environmental sustainability of MSW management system. To deal with the environmental sustainability they developed two indicators, viz., damage to ecosystem and damage to abiotic resources. For economic sustainability assessment of WtE technologies they developed a indicator based on life cycle costing which includes all the costs associated to the design and construction of plant, collection and transportation of waste, processing, operation, maintenance, and final disposal. MSW management poses both negative and positive impacts on society. Therefore, it is necessary to involve both positive and negative aspects for the assessment of social sustainability of MSW management. Negative social impacts were characterized by the indicator, damage to human health whereas positive ones, by community well-being (income based measure). For more details see Menikpura et al. (2012).
Estimation of Waste Composition
The composition of MSW varies all around the globe according to the consumption habits, geographical locations, and variation in seasonality and climatic conditions (Ayodele et al., 2017; Moya et al., 2017b; Maisarah et al., 2018). The effective approach to identify local MSW composition is to collect waste samples from various local MSW sources which is quite a challenging and resource-intensive process as well. Therefore, the data regarding waste composition should be collected from the designated local authorities for waste management. If the local authorities do not have satisfactory data regarding waste composition, then the IPCC's country or regional default values [https://www.ipccnggip.iges.or.jp/public/2019rf/pdf/5_Volume5/19R_V5_2_Ch02_Waste_Data.pdf] for MSW composition can be used.
Estimation of Urban Development
Urban development is a global phenomenon. It depends upon many socio-economic factors including an increase in urban population, employment, infrastructure development, the increased living standard of the people, and social public services. All these socio-economic factors refer to economic development. Gross domestic product (GDP) per capita is the most commonly used indicator to measure the level of economic development. Chen et al. (2014) performed a study to examine the global pattern of urban development and economic growth by using GDP per capita and level of urbanization as economic indicators through cross-sectional, panel estimation. The outcomes of the study showed that GDP per capita and level of urbanization are highly correlated. Moreover, many researchers have recognized these two indicators as the most appropriate to measure urban development (Chen et al., 2014). Therefore, these two indicators are recommended to measure the level of urban development of any country. The level of urban development can be compared with the countries which are economically more developed. Income level is the measure to compare the level of economic development between countries. According to the World Bank, there are four threshold income levels as presented in Table 6. The GDP per capita of a given country can be compared with the higher income countries to estimate the level of urban development to identify the most suitable WtE technology regarding favorable geographical location presented in Table 5. The data for these indicators can be used from the World Bank's online database [http://data.worldbank.org/].
Estimation of Technological Development
Since the beginning of the global financial crisis in 2008, the world economy has tried to move from initial recovery to more sustained expansion. Governments all around the world have applied looser fiscal and monetary policies, which initiated a period of recovery. However, fiscal, or monetary policies are not sufficient enough for the economies to enter into a more lasting expansionary period. Policymakers have recognized that technological progress is needed for the sustainable economic development of any country. From the 1950s to the 1990s, more than one-third of the global economic development was due to technological progress (Cavdar and Aydin, 2015). One of the most important reasons why China could realize such great achievements in terms of economic growth is scientific progress and innovation (Yang, 2012). The level of complexity of WtE technologies is also associated with the technological development of any country. Higher the level of technological development, the higher the tendency of a country to deal with WtE technologies with a high level of complexity. Therefore, the level of complexity of WtE technology is indirectly related to the country's economic development. According to International Energy Agency (IEA), the total public energy research development and demonstration (RD&D) budget per unit of GDP is a key indicator to measure the level of technological development in the energy sector of a country (IEA, 2020). The statistical data for total public energy RD&D budget is available on [https://www.iea.org/subscribe-to-data-services/energy-technology-rdd], and the data for current total GDP can be used from the World Bank's online database [http://data.worldbank.org/]. The total public energy RD&D budget per unit of GDP can be compared with the higher income countries to estimate the level of technological development in the energy sector of the given country, and the most suitable WtE technology can be selected according to the complexity level of the technology expressed in Table 5.
Indicators to Measure the Skill Level of Labor
Labor skill is an attribute of the supply side of the labor market. The development of labor skills is very important to enhance the sustainable productivity of a market or industry. It is also essential to improve the employability of labor. This factor is the most difficult to measure because there is no consensus on any well-developed methodology to measure the labor skill level. However, the International Labor Organization (ILO) categorizes labor skills into three different types, viz., basic skills, technical skills, and core skills (ILO, 2018). ILO proposed a set of academically attested indicators to measure the skill level of the labor which is applicable at a global scale. The list of these indicators is presented in Table 7.
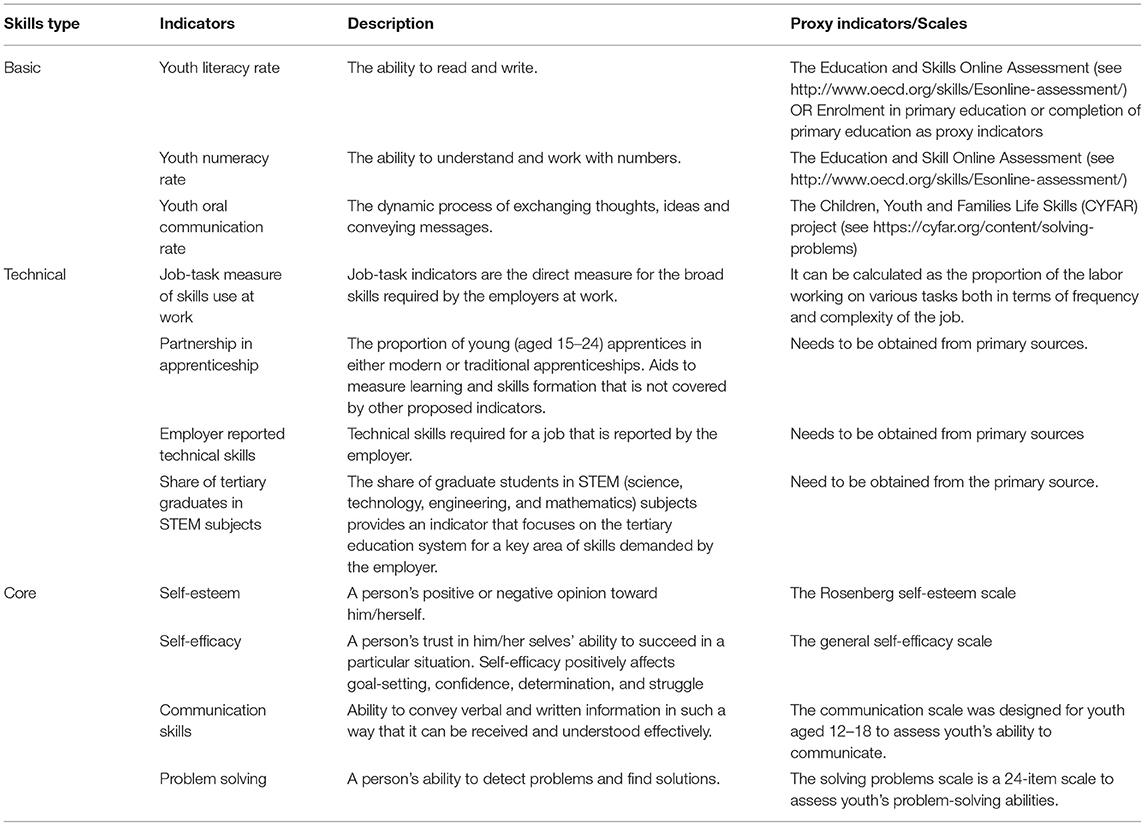
Table 7. Indicators to measure basic, technical, and core skills of labor (ILO, 2018).
Capital and Operational Cost of WtE Technologies
The decision of the most suitable WtE technology is highly dependent on the initial capital investment cost and operating cost of the plant (Mubeen and Buekens, 2019). The capital cost depends on the plant type, plant size, geographical location, site implementation (construction, etc.), and land requirement. On the other hand cost of operations is the aggregated cost incurred to operate the plant that includes the cost of labor, energy, maintenance as well as working capital (Mubeen and Buekens, 2019; Farooq et al., 2020). For the best approach, both capital and operating cost should be estimated by obtaining the data from primary sources. In that case, the cost of plant machinery and equipment should be estimated directly from the manufacturer's quotations and the cost of operations should be estimated according to the cost data from a representative operational WtE plant (Sorapipatana and Yoosin, 2011; Farooq et al., 2020). If the data cannot be obtained from the primary sources, then historic prices can be used from the literature after adjusting to the present prices. The capital and operating costs per ton of waste processed for concerned WtE technologies from the referenced literature are presented in Table 5. The capital and operational costs presented in Table 5 are the estimated costs that were taken from the referenced literature. However, the actual capital and operational costs may vary due to various factors viz., governmental incentives, plant capacity, availability of raw material (MSW), and availability of skilled labor (Kumar and Samadder, 2017).
Schematic of the Proposed Framework
In the previous section, many socio-economic, technological, and environmental indicators and methods were introduced to propose a framework for the selection of suitable WtE technologies for sustainable MSW management. However, there is no specific order or significance level between the assessed factors. It is quite a complex task to list the concerning factors in the least to most significant order. This is where the multi criteria decision making (MCDM) analysis approach is germane. MCDM analysis methods have been extensively used in environmental decision-making and sustainable energy planning (Qazi et al., 2018a). Yap and Nixon (2015), performed a very brief but critical review of application of MCDM techniques in waste management and energy planning decisions. The results of the review showed that there is a huge potential for MCDM techniques to be applied in decision making in the field of MSW management and energy planning. The most popular MCDM techniques are multiple attribute utility theory, analytical hierarchical process, analytical network process, preference ranking organization method for enrichment evaluation, elimination at choice translating reality, and technique for order preference by similarity of ideal solution. The analytical hierarchical process method is the most popular and frequently used MCDM analysis method for the evaluation and selection of renewable energy technologies (Yap and Nixon, 2015). The reason behind the popularity of this method is its effectiveness which overshadows other ranking methods. Therefore, the analytical hierarchical process method is selected to address the ranking of concerning socio-economic, technological, and environmental factors in the least to most significant order. In this section, the concerned indicators and methods are arranged in a systematic scheme (as presented in Figure 3) to develop a framework that can guide toward an appropriate selection of the most feasible WtE technologies. According to the schematic of the framework, the first step is to select a study area (country, region, etc.). The second step refers to the ranking of the concerned socio-economic, technological and environmental factors to understand which factor(s) affects the selection of WtE technologies the most. The next step is to analyze the environmental credibility of WtE technologies by performing LCA which will include all the stages of waste management, along with the socio-economic and technological profile of concerning factors with help of proposed indicators, methods, and models. In the fourth and final step of the framework, the most suitable WtE technologies can be selected by comparing the outcomes of the analysis performed in the third step with the information presented in section Assessment of Socio-Economic, Technological, and Environmental Factors of WtE Technologies (collected from the referenced literature). For example, if the level of technological development, urban development, and labor skills are found to be high in the selected region, WtE technologies with a high level of complexity (gasification and pyrolysis) can be selected as these technologies are more suitable for the regions with a high level of urban development and need labor with higher skills to be operated. The identification of favorable WtE technologies regarding waste composition and capital and operational costs is pretty much straightforward. The structure of the waste composition of the selected region can be assessed by using information presented in Table 4 to identify the most suitable WtE technology regarding waste composition. After identifying the suitable WtE technology, the quantity of waste should also be considered to select the adequate plant capacity to be installed. On the other hand, the favorability of WtE technologies regarding capital and operational cost depends upon the concern of investors. For example, the technology with low capital and operational cost will be more favorable if cost-effectiveness is concerned. On the other hand, the WtE technologies competitive regarding environmental performance can be identified through LCA. It might happen that the WtE technologies which are better choices regarding some of the concerned factors will not be so favorable regarding others. In this case, the WtE technologies which are favorable regarding indicators found as more significant through the analytical hierarchical process method will be given priority or vice versa. Therefore, it is highly recommended to use the analytical hierarchy process method to analyze the most to least significant factors for the sake of developing a holistic selection criteria to identify the most suitable WtE technologies.
Policy Implications and Limitations of the Proposed Framework
The selection of right WtE options for sustainable MSW management is a complex task for policy makers, private public investors, and other stakeholders. In this study, a detailed review was carried out to assess environmental, socio-economic, technological, and territorial aspects of different WtE alternatives, and a general selection framework was proposed in the form of a systematic scheme. The proposed framework is an amalgamation of academically acclaimed socio-economic indicators, and well-established methods and models. The new framework is found to be useful for the prior evaluation of multiple aspects (environmental, socio-economic, technological, and territorial) related to WtE technologies in order to develop sustainable MSW management systems and aid policy makers and stakeholders to make a reality-based decision regarding sustainable MSW management in any given region. The proposed framework can be implemented to evaluate the WtE technologies both as standalone projects or as a part of a MSW management system because the framework addresses the interest of policymakers, public and private sector investors, and other stakeholders. However, there are some limitations of the proposed framework. A huge amount of data is required for the proper estimation of environmental performance and proposed socio-economic and technological indictors. Therefore, the availability and transparency of the data can highly affect the credibility of the proposed framework. Moreover, the selection of proper WtE technologies is highly dependent on the interest of stakeholders regarding the desired end products. Furthermore, governments and other legislative bodies can also influence the selection of optimum WtE technologies. If the government or legislation bodies wants to promote any particular WtE technology to achieve the policy targets or for any other reason, they subsidize the desired WtE technology even if it is a less favorable option. This kind of intervention can make it very difficult to attain sustainability in any MSW management system.
Conclusion and Recommendations
Municipal solid waste (MSW) generation is an inexorable consequence of human activities, and its management is both a complex and resource-intensive task. Governments and policymakers have been trying to design effective MSW management systems around the globe, by incorporating a combination of waste treatment methods. However, waste management policies and systems have been consistently revised due to the rapid growth in MSW generation and its heterogeneous composition. Waste to energy (WtE) technologies have been recognized as a promising solution being incorporated in modern MSW management systems in developed countries like the European Union and the USA etc., to cope with the problem of complexly composed and ever-increasing waste volumes. However, the selection of appropriate WtE technologies to design sustainable waste management systems for developing countries is still a big challenge for governments and policymakers. Therefore, this study was performed to propose a general systematic framework that can aid policymakers to identify the most suitable WtE technologies for designing sustainable MSW management systems for developing countries.
The proposed framework considered six different factors including environmental performance, the origin and type of waste, capital and operational cost, WtE conversion efficiency and complexity of the technology, the skillset of the labor, and the favorable geographical location to cover the environmental, socio-economic, technological, and territorial aspects related to WtE technologies. Different socio-economic indicators coupled with highly acclaimed methods and models are incorporated in the form of a systematic scheme that includes four steps. The recommended indicators, methods, and models in the proposed framework are selected after a detailed review of the literature published in well-known scientific journals, or reports of leading international organizations such as the World Bank, International Energy Agency (IEA), and International Labor Organization (ILO). Moreover, internationally recognized databases are also introduced to extract the secondary data for the estimation of various recommended socio-economic indicators.
The analytical hierarchical process method is proposed to rank the considered factors from least to most significant order. The ranking of these factors is a very important and crucial step because it will help the policymakers to understand which factor should be given more importance during the process of selecting favorable WtE technologies. Life cycle assessment (LCA) is proposed for the assessment of the environmental performance of different WtE technologies. For the assessment of the waste composition of MSW, the best approach is to identify the waste composition structure by collecting the data from primary sources such as local authorities or designated departments. The economic indicators proposed to assess the level of urban development are the gross domestic product (GDP) per capita and level of urbanization, and for the assessment of technological development is the total public energy research development and demonstration (RD&D) budget as a share of total GDP. The assessment of the level of labor skillset is very difficult; hence, there is not a well-defined methodology or set of indicators for its estimation. However, a total of 11 indicators (academically acclaimed) are proposed for the estimation of labor skillset level. Similar to the waste composition, the best approach for the estimation of capital and operational costs is by using primary data. If for any reason the primary data is not available for the estimation of waste composition, and capital and operational costs, then the secondary data can be used. The proposed framework is an effort toward the development of such a policy that can help to design sustainable municipal waste management systems around the globe. However, the application of the proposed framework is highly sensitive to the availability of the data and the interests of governments, policymakers, and public and private sector investors.
The proposed framework helps to address the key factors associated to the selection of suitable WtE technologies. However, the authors recommend incorporating the aspect of law and order to define the environmental and social rules and regulations to overcome the limitation of governments' and other legislative bodies' influence on the selection of most suitable WtE technologies for sustainable MSW management.
Data Availability Statement
The original contributions presented in the study are included in the article/supplementary material, further inquiries can be directed to the corresponding author/s.
Author Contributions
AF conceived the study and wrote the initial draft manuscript under the supervision of SHG. PH and TS provided inputs during the study and on the manuscript writing. All authors contributed to the article and approved the submitted version.
Conflict of Interest
The authors declare that the research was conducted in the absence of any commercial or financial relationships that could be construed as a potential conflict of interest.
Acknowledgments
The authors would like to thank Petchra Pra Jom Klao doctoral degree scholarship, the King Mongkut's University of Technology Thonburi (KMUTT), and the Joint Graduate School of Energy and Environment (JGSEE) for their financial support and peaceful academic atmosphere to complete this study. The authors would also like to acknowledge all the researchers, institutes, and organizations whose research and reports have been used and referenced to fulfill the research objectives.
References
Abreu-Cavalheiro, A., and Monteiro, G. (2013). Solving ethanol production problems with genetically modified yeast strains. Braz. J. Microbiol. 44, 665–671. doi: 10.1590/S1517-83822013000300001
Agamuthu, P., Fauziah, S. H., and Khizdir, K. M. Aiza, N. (2007). “Sustainable waste management - Asian perspectives,” in Proceedings of the International Conference on Sustainable Solid Waste Management (Chennai), 15–26.
Arena, U. (2012). Process and technological aspects of municipal solid waste gasification. A review. Waste Manag. 32, 625–639. doi: 10.1016/j.wasman.2011.09.025
Astrup, T. F., Davide, T., Roberto, T., and Alessio, B. (2015). Life cycle assessment of thermal waste-to-energy technologies: review and recommendations. Waste Manag. 37, 104–115. doi: 10.1016/j.wasman.2014.06.011
Atwadkar, R. R., Jadhav, L. D., Wagh, M. M., and Shinde, N. N. (2015). A multi criteria ranking of different technologies for the waste to energy of municipal solid waste in the city of Kolhapur. Int. J. Emerg. Technol. Adv. Eng. 4, 937–942.
Ayodele, T. R., Ogunjuyigbe, A. S. O., and Alao, M. A. (2017). Life Cycle assessment of waste-to-energy (WtE) technologies for electricity generation using municipal solid waste in Nigeria. Appl. Energy. 201, 200–218. doi: 10.1016/j.apenergy.2017.05.097
Bajpai, P. (2017). “Basics of anaerobic digestion process”, in Anaerobic Technology in Pulp and Paper Industry, ed J. Kacprzky (Singapore: Springer Nature), 7–12. doi: 10.1007/978-981-10-4130-3
Balat, M., Balat, M., Kirtay, E., and Balat, H. (2009). Main routes for the thermo-conversion of biomass into fuels and chemicals. Part 1 : pyrolysis systems. Energy Convers. Manag. 50, 3147–3157. doi: 10.1016/j.enconman.2009.08.014
Beyene, H. D., Werkneh, A. A., and Ambaye, T. G. (2018). Current updates on waste to energy (WtE) technologies: a review. Renew. Energy Focus. 24, 1–11. doi: 10.1016/j.ref.2017.11.001
Cavdar, S. C., and Aydin, A. D. (2015). An empirical analysis about technological development and innovation indicators. Proc. Soc. Behav. Sci. 195, 1486–1495. doi: 10.1016/j.sbspro.2015.06.449
Cecchi, F., and Cavinato, C. (2015). Anaerobic digestion of bio-waste: a mini-review focusing on territorial and environmental aspects. Waste Manag. Res. 33, 429–438. doi: 10.1177/0734242X14568610
Chaya, W., and Gheewala, S. H. (2007). Life cycle assessment of MSW-to-energy schemes in Thailand. J. Clean. Prod. 15, 1463–1468. doi: 10.1016/j.jclepro.2006.03.008
Chen, M., Zhang, H., Liu, W., and Zhang, W. (2014). The global pattern of urbanization and economic growth: evidence from the last three decades. PLoS ONE 9:e103799. doi: 10.1371/journal.pone.0103799
Chiu, H. Y., Pai, T. Y., Liu, M. H., Chang, C. A., Lo, F. C., Chang, T. C., et al. (2016). Electricity production from municipal solid waste using microbial fuel cells. Waste Manag. Res. 34, 619–629. doi: 10.1177/0734242X16649681
Cucchiella, F., D'Adamo, I., and Gastaldi, M. (2014). Sustainable management of waste-to-energy facilities. Renew. Sustain. Energy Rev. 33, 719–728. doi: 10.1016/j.rser.2014.02.015
Cucchiella, F., D'Adamo, I., and Gastaldi, M. (2017). Sustainable waste management: waste to energy plant as an alternative to landfill. Energy Convers. Manag. 131, 18–31. doi: 10.1016/j.enconman.2016.11.012
D'Adamo, I., Gastaldi, M., and Rosa, P. (2020). Recycling of end-of-life vehicles: assessing trends and performances in Europe. Technol. Forecast. Soc. Change. 152. doi: 10.1016/j.techfore.2019.119887
DOE (2019). Waste-to-Energy From Municipal Solids Wastes. U.S Department of Energy. Available online at: https://www.energy.gov/sites/prod/files/2019/08/f66/BETO–Waste-to-Energy-Report-August−752~2019.pdf
Dong, J., Tang, Y., Nzihou, A., Chi, Y., Weiss-Hortala, E., Ni, M., et al. (2018a). Comparison of waste-to-energy technologies of gasification and incineration using life cycle assessment: case studies in Finland, France and China. J. Clean. Prod. 203, 287–300. doi: 10.1016/j.jclepro.2018.08.139
Dong, J., Tang, Y., Nzihou, A., Chi, Y., Weiss-Hortala, E., and Ni, M. (2018b). Life cycle assessment of pyrolysis, gasification and incineration waste-to-energy technologies: theoretical analysis and case study of commercial plants. Sci. Tot. Environ. 626, 744–753. doi: 10.1016/j.scitotenv.2018.01.151
Ducharme, C. (2010). Technical and Economic Analysis of Plasma-Assisted Waste-to-Energy Processes (Dissertation/master's thesis). Columbia University. Available online at: http://www.seas.columbia.edu/earth/wtert/sofos/ducharme_thesis.pdf
Duncan, J. (2003). Costs of Biodiesel Production. Energy Efficiency and Conversion Authority New Zealand. Available online at: http://www.globalbioenergy.org/uploads/media/0305_Duncan_-_Cost-of-biodiesel-production.pdf
Farooq, A., Bangviwat, A., and Gheewala, S. H. (2020). Life cycle cost analysis of ethanol production from sugarcane molasses for gasoline substitution as transportation fuel in Pakistan. J. Sustain. Energy Environ. 11, 49–59.
Gardy, J., Hassanpour, A., Lai, X., Cunliffe, A., and Rehan, M. (2014). The influence of blending process on the quality of rapeseed oil-used cooking oil biodiesels. Int. Sci. J. Environ. Sci. 3, 1–8.
Gerpen, J. V. (2005). Biodiesel processing and production. Fuel Process. Technol. 86, 1097–1107. doi: 10.1016/j.fuproc.2004.11.005
Gumisiriza, R., Hawumba, J. F., Okure, M., and Hensel, O. (2017). Biomass waste-to-energy valorisation technologies: a review case for banana processing in Uganda. Biotechnol. Biofuels 10, 1–29. doi: 10.1186/s13068-016-0689-5
Horttanainen, M., Teirasvuo, N., Kapustina, V., Hupponen, M., and Luoranen, M. (2013). The composition, heating value and renewable share of the energy content of mixed municipal solid waste in Finland. Waste Manag. 33, 2680–2686. doi: 10.1016/j.wasman.2013.08.017
Hui, C. O., and Mohammed, H. A. (2015). The role of cost breakdown structure in life cycle cost model. J. Teknol. 2, 117–121.
ILO (2018). Concepts and Definitions of Employment Indicators Relevant to Young People. Geneva: International Labour Organization.
IPCC (2019). Waste Generation, Composition And Management. Geneva: Intergovernmental Pannel on Climate Change.
Jahirul, M. I., Rasul, M. G., Chowdhury, A. A., and Ashwath, N. (2012). Biofuels production through biomass pyrolysis- a technological review. Energies 5, 4952–5001. doi: 10.3390/en5124952
Jurczyk, M., Mikus, M., and Dziedzic, K. (2016). Flue gas cleaning in municipal waste-to-energy plants – Part I flue gas cleaning in municipal waste-to-energy. Infrastruct. Ecol. Rural Areas 1, 1179–1193. doi: 10.14597/infraeco.2016.4.1.086
Kaza, S., Yao, L., Bhad-Tata, P., and Woerden, F. V. (2018). What a Waste 2.0: A Global Snapshot of Solid Waste 2050. Washington, DC: The World Bank. doi: 10.1596/978-1-4648-1329-0
Khan, I., and Kabir, Z. (2020). Waste-to-energy generation technologies and the developing economies: a multi-criteria analysis for sustainability assessment. Renew. Energy 150, 320–333. doi: 10.1016/j.renene.2019.12.132
Kumar, A., and Samadder, S. R. (2017). A review on technological options of waste to energy for effective management of municipal solid waste. Waste Manag. 69, 407–422. doi: 10.1016/j.wasman.2017.08.046
Kurbatova, A., and Abu-Qdais, H. A. (2020). Using multi-criteria decision analysis to select waste to energy technology for a Mega City : the case of Moscow. Sustainability 12:9828. doi: 10.3390/su12239828
Li, J., Zhou, H., and Cao, Y. (2012). Transesterification of waste cooking oil to produce biodiesel using acid and alkaline catalyst. Adv. Mater. Res. 518–523, 3566–3572. doi: 10.4028/www.scientific.net/AMR.518-523.3566
Liamsanguan, C., and Gheewala, S. H. (2008). LCA: a decision support tool for environmental assessment of MSW management systems. J. Environ. Manage. 87, 132–138. doi: 10.1016/j.jenvman.2007.01.003
Lombardi, L., Carnevale, E., and Corti, A. (2012). Analysis of energy recovery potential using innovative technologies of waste gasification. Waste Manag. 32, 640–652. doi: 10.1016/j.wasman.2011.07.019
Lopes, M., Paulillo, S. C. DL., Godoy, A., Cherubin, R. A., Lorenzi, M. S., Giometti, F. H. C., et al. (2016). Ethanol production in Brazil: a bridge between science and industry. Braz. J. Microbiol. 47, 64–76. doi: 10.1016/j.bjm.2016.10.003
Luo, L., Voet, E. V. D., and Huppes, G. (2009). Life cycle assessment and life cycle costing of bioethanol from sugarcane in Brazil. 13, 1613–1619. doi: 10.1016/j.rser.2008.09.024
Maisarah, M., Bong, C. P. C., Ho, W. S., Lim, J. S., Muis, Z., Hashim, H., et al. (2018). Review on the suitability of waste for appropriate waste-to-energy technology. Chem. Eng. Trans. 63, 187–192. doi: 10.3303/CET1863032
Marshall, A. J. S., Wu, P. F., Mun, S., and Lalonde, C. (2014). “Commercial application of pyrolysis technology in agriculture,” in American Society of Agriculture and Biological Engineering (ASADE) Annual International Meeting.
Mayer, F., Bhandari, R., and Gäth, S. (2019). Critical review on life cycle assessment of conventional and innovative waste-to-energy technologies. Sci. Tot. Environ. 672, 708–721. doi: 10.1016/j.scitotenv.2019.03.449
Menikpura, S. N. M., Gheewala, S. H., and Bonnet, S. (2012). Framework for life cycle sustainability assessment of municipal solid waste management systems with an application to a case study in Thailand. Waste Manag. Res. 30, 708–719. doi: 10.1177/0734242X12444896
Menikpura, S. N. M., Sang-Arun, J., and Bengtsson, M. (2016). Assessment of environmental and economic performance of waste-to-energy facilities in Thai cities. Renew. Energy 86, 576–584. doi: 10.1016/j.renene.2015.08.054
Mihai, F. (2012). “Geography of waste as a new approach in waste management study,” in Papers of Geographic Seminar Dimitrie Cantemir, Vol. 33, 39–46. doi: 10.31235/osf.io/dkxcs
Moora, H., Roos, I., Kask, U., Kask, L., and Ounapuu, K. (2017). Determination of biomass content in combusted municipal waste and associated CO2 emissions in Estonia. Energy Proc. 128, 222–229. doi: 10.1016/j.egypro.2017.09.059
Moser, B. R. (2009). “Biodiesel production, properties, and feedstocks,” in In Vitro Cellular and Developmental Biology – Plant, eds P. Lakshmanan, D. D. Songstad (Switzerland: Springer Nature), 229–266. doi: 10.1007/s11627-009-9204-z
Moukamnerd, C., Kawahara, H., and Katakura, Y. (2013). Feasibility study of ethanol production from food wastes by consolidated continuous solid-state fermentation. J. Sustain. Bioenergy Syst. 3, 143–148. doi: 10.4236/jsbs.2013.32020
Moya, D., Aldás, C., Jaramillo, D., Játiva, E., and Kaparaju, P. (2017a). Waste-to-energy technologies: an opportunity of energy recovery from municipal solid waste, using quito - ecuador as case study. Energy Proc. 134, 327–336. doi: 10.1016/j.egypro.2017.09.537
Moya, D., Aldás, C., López, G., and Kaparaju, P. (2017b). Municipal solid waste as a valuable renewable energy resource: a worldwide opportunity of energy recovery by using waste-to-energy technologies. Energy Proc. 134, 286–295. doi: 10.1016/j.egypro.2017.09.618
Mubeen, I., and Buekens, A. (2019). “Energy from waste: future prospects toward sustainable development,” in Current Developments in Biotechnology and Bioengineering: Waste Treatment Processes for Energy Generation, eds S. Kumar, R. Kumar, and A. Pandey (Elsevier), 283–305. doi: 10.1016/B978-0-444-64083-3.00014-2
Mutz, D., Hengevoss, D., Christoph, H., and Gross, T. (2017). Waste-to-Energy Options in Municipal Solid Waste Management-A Guide for Decision Makers in Developing and Emerging Countries. Eschborn: Deutsche Gesellschaft Für Internationale Zusammenarbeit GmbH.
Nieuwlaar, E. (2013). Life cycle assessment and energy systems. Encycl. Energy 3, 647–654. doi: 10.1016/B0-12-176480-X/00233-3
Nizami, A. S., Mohanakrishna, G., Mishra, U., and Pant, D. (2016). “Trends and sustainability criteria for liquid biofuels,” in Biofuels Production and Future Perspective, eds R. S. Singh, A. Pandey, E. Gnansounou (London: Taylor and francis group), 59–88. doi: 10.1201/9781315370743-5
Noya, I., Inglezakis, V., González-García, S., Katsou, E., Feijoo, G., and Moreira, M. T. (2018). Comparative environmental assessment of alternative waste management strategies in developing regions: a case study in Kazakhstan. Waste Manag. Res. 36, 689–697. doi: 10.1177/0734242X18786388
Ouda, O. K. M., Cekirge, H. M., and Raza, S. A. R. (2013). An assessment of the potential contribution from waste-to-energy facilities to electricity demand in Saudi Arabia. Energy Convers. Manag. 75, 402–406. doi: 10.1016/j.enconman.2013.06.056
Ouda, O. K. M., Raza, S. A., Nizami, A. S., Rehan, M., Al-Waked, R., and Korres, N. E. (2016). Waste to energy potential: a case study of Saudi Arabia. Renew. Sustain. Energy Rev. 61, 328–340. doi: 10.1016/j.rser.2016.04.005
Ozbay, I., and Durmusoglu, E. (2013). Energy content of municipal solid waste bales. Waste Manag. Res. 31, 674–683. doi: 10.1177/0734242X13485866
Pandyaswargo, A. H., Onoda, H., and Nagata, K. (2012). Energy recovery potential and life cycle impact assessment of municipal solid waste management technologies in asian countries using ELP model. Int. J. Energy Environ. Eng. 3. doi: 10.1186/2251-6832-3-28
Qazi, W. A., Abushammala, M. F. M., and Azam, M. H. (2018a). Multi-criteria decision analysis of waste-to-energy technologies for municipal solid waste management in sultanate of Oman. Waste Manag. Res. 36, 594–605. doi: 10.1177/0734242X18777800
Qazi, W. A., Abushammala, M. F. M., Azam, M. H., and Younes, M. K. (2018b). Waste-to-energy technologies: a literature review. J. Solid Waste Technol. Manag. 44, 387–409. doi: 10.5276/JSWTM.2018.387
Shahbazi, S., Kurdve, M., Zackrisson, M., Jönsson, C., and Kristinsdottir, A. R. (2019). Comparison of four environmental assessment tools in Swedish manufacturing: a case study. Sustainability 11:2173. doi: 10.3390/su11072173
Shahnazari, A., Rafiee, M., Rohani, A., Nagar, B. B., Ebrahiminik, M. A., and Aghkhani, M. H. (2020). Identification of effective factors to select energy recovery technologies from municipal solid waste using multi-criteria decision making (MCDM): a review of thermochemical technologies. Sustain. Energy Technol. Assess. 40. doi: 10.1016/j.seta.2020.100737
Shahzad, K., Nizami, A. S., Sagir, M., Rehan, M., Maier, S., Khan, M. Z., et al. (2017). Biodiesel production potential from fat fraction of municipal waste in Makkah. PLoS ONE 12:e0171297. doi: 10.1371/journal.pone.0171297
Sharma, Y. C., Singh, B., and Upadhyay, S. N. (2008). Advancements in development and characterization of biodiesel: a review. Fuel 87, 2355–2373. doi: 10.1016/j.fuel.2008.01.014
Sitorus, B., and Sukandar Panjaitan, S. D. (2013). Biogas recovery from anaerobic digestion process of mixed fruit -vegetable wastes. Energy Proc. 32, 176–182. doi: 10.1016/j.egypro.2013.05.023
Soleymani, M., and Rosentrater, K. A. (2017). Techno-economic analysis of biofuel production from macroalgae (Seaweed). Bioengineering 4, 1–10. doi: 10.3390/bioengineering4040092
Soltani, A., Sadiq, R., and Hewage, K. (2016). Selecting sustainable waste-to-energy technologies for municipal solid waste treatment: a game theory approach for group decision-making. J. Clean. Prod. 113, 388–399. doi: 10.1016/j.jclepro.2015.12.041
Sorapipatana, C., and Yoosin, S. (2011). Life cycle cost of ethanol production from cassava in Thailand. Renew. Sustain. Energy Rev. 15, 1343–1349. doi: 10.1016/j.rser.2010.10.013
Tan, S. T., Ho, W. S., Hashim, H., Lee, C. T., Taib, M. R., and Ho, C. S. (2015). Energy, economic and environmental (3E) analysis of waste-to-energy (WTE) strategies for municipal solid waste (MSW) management in Malaysia. Energy Convers. Manag. 102, 111–120. doi: 10.1016/j.enconman.2015.02.010
Thamsiriroj, T., and Murphy, J. D. (2010). How much of the target for biofuels can be met by biodiesel generated from residues in Ireland? Fuel 89, 3579–3589. doi: 10.1016/j.fuel.2010.06.009
Tong, H., Shen, Y., Zhang, J., Wang, C., Ge, T. S., and Tong, Y. W. (2018). A comparative life cycle assessment on four waste-to-energy scenarios for food waste generated in eateries. Appl. Energy. 225, 1143–1157. doi: 10.1016/j.apenergy.2018.05.062
Tozlu, A., Özahi, E., and Abuşoʇlu, A (2016). Waste to energy technologies for municipal solid waste management in Gaziantep. Renew. Sustain. Energy Rev. 54, 809–815. doi: 10.1016/j.rser.2015.10.097
Tsui, T., and Wong, J. W. C. (2019). A critical review: emerging bioeconomy and waste-to-energy technologies for sustainable municipal solid waste management. Waste Disposal Sustain. Energy. 1, 151–167. doi: 10.1007/s42768-019-00013-z
Vindis, P., Mursec, B., Janjekovic, M., and Cus, F. (2009). The impact of mesophilic and thermophilic anaerobic digestion on biogas production. J. Achiev. 36, 192–198. doi: 10.2507/daaam.scibook.2009.27
Weedermann, M., Seo, G., and Wolkowicz, G. (2013). Mathematical model of anaerobic digestion in a chemostat : effects of syntrophy and inhibition. J. Biol. Dyn. 7, 59–85. doi: 10.1080/17513758.2012.755573
Yadav, P., and Samadder, S. R. (2017). A global prospective of income distribution and its effect on life cycle assessment of municipal solid waste management: a review. Environ. Sci. Pollut. Res. 24, 9123–9141. doi: 10.1007/s11356-017-8441-7
Yang, J. (2012). Empirical research of the relationship between energy consumption and economic growth in beijing. Technol. Invest. 3, 168–173. doi: 10.4236/ti.2012.33023
Yap, H. Y., and Nixon, J. D. (2015). A multi-criteria analysis of options for energy recovery from municipal solid waste in India and the UK. Waste Manag. 46, 265–277. doi: 10.1016/j.wasman.2015.08.002
Zaman, A. U. (2009). Life cycle environmental assessment of municipal solid waste to energy technologies. Glob. J. Environ. Res. 3, 155–163.
Keywords: sustainable municipal solid waste management, waste to energy technologies, conversion pathways, socio-economic indicators, selection framework
Citation: Farooq A, Haputta P, Silalertruksa T and Gheewala SH (2021) A Framework for the Selection of Suitable Waste to Energy Technologies for a Sustainable Municipal Solid Waste Management System. Front. Sustain. 2:681690. doi: 10.3389/frsus.2021.681690
Received: 17 March 2021; Accepted: 31 March 2021;
Published: 05 May 2021.
Edited by:
Davide Settembre Blundo, Rey Juan Carlos University, SpainReviewed by:
Sara Alonso-Muñoz, Rey Juan Carlos University, SpainAlexandros Maziotis, Pontificia Universidad Católica de Chile, Chile
Copyright © 2021 Farooq, Haputta, Silalertruksa and Gheewala. This is an open-access article distributed under the terms of the Creative Commons Attribution License (CC BY). The use, distribution or reproduction in other forums is permitted, provided the original author(s) and the copyright owner(s) are credited and that the original publication in this journal is cited, in accordance with accepted academic practice. No use, distribution or reproduction is permitted which does not comply with these terms.
*Correspondence: Shabbir H. Gheewala, c2hhYmJpcl9nQGpnc2VlLmttdXR0LmFjLnRo