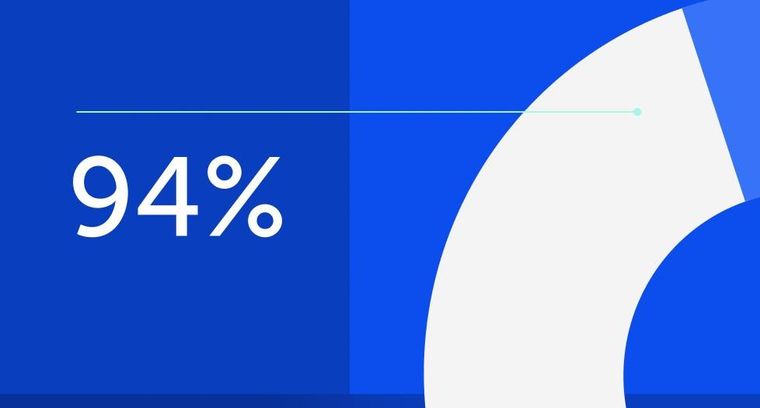
94% of researchers rate our articles as excellent or good
Learn more about the work of our research integrity team to safeguard the quality of each article we publish.
Find out more
ORIGINAL RESEARCH article
Front. Surg., 27 March 2025
Sec. Orthopedic Surgery
Volume 12 - 2025 | https://doi.org/10.3389/fsurg.2025.1520481
This article is part of the Research TopicMinimally Invasive Treatments for Lumbar Spine DisordersView all 6 articles
Objectives: Traditional cortical bone trajectory (CBT) screws in the lumbar spine offer greater holding strength and are well-suited for patients with osteoporosis. However, the screw implantation procedure is challenging and associated with significant risk. This study aimed to assess whether individualized 3D-printing navigation technology provides higher accuracy and better clinical outcomes compared to the free-hand isthmus method for lumbar CBT screw implantation.
Methods: From September 2020 to August 2023, a total of 41 patients who underwent CBT screw surgery were retrospectively collected. Among them, 23 patients underwent the free-hand isthmus method (Group A), while 18 patients underwent the individualized 3D-printing navigation technique (Group B). All imaging and clinical data for these patients were collected in a blinded manner.
Results: During the surgery, 185 CBT screws were implanted into the lumbar spines of 41 patients—78 in Group A and 107 in Group B. After the surgery, the majority of implanted screws (86.5%) were classified as grade 0, indicating satisfactory implantation. Compared to Group A, Group B had fewer screws classified as grade 1 or grade 2 (p = 0.045), indicating higher accuracy in screw implantation. Additionally, Group B also had a shorter operation duration (p = 0.02), fewer fluoroscopy exposures (p < 0.01), and less blood loss (p = 0.03). In addition, compared to Group A, individuals in Group B showed significant improvement in back pain symptoms at both 3 and 6 months (p = 0.01 and <0.01), as well as in physical activity at 3 months (p = 0.02) postoperatively. No significant difference in postoperative complications was observed between the two groups.
Conclusion: Compared to the free-hand isthmus method, lumbar CBT screw implantation with individualized 3D-printing navigation technology shows higher accuracy, shorter operative time, reduced intraoperative fluoroscopy and blood loss, and better clinical outcomes at three months post-surgery.
The Cortical Bone Trajectory (CBT) is a novel screw insertion technique introduced by Ueno and Santoni in 2009 to address the limitations of traditional pedicle screws (PS), especially in patients with osteoporosis or significant bone loss (1, 2). CBT increases the contact area between the screw and the dense bone cortex, which is largely unaffected by degeneration and osteoporosis, thereby enhancing the screw's holding strength and stability (3–9). Existing evidence shows that CBT offers 30% greater pullout resistance compared to conventional pedicle screws and provides 1.7 times more holding power, even when using shorter screws (10–12).
There are challenges in identifying the correct entry point for CBT. The classical entry point for CBT is located at the intersection of the midline of the superior articular process and 1 mm below the transverse process (1, 2, 12). However, other studies have suggested using the upper edge of the intervertebral foramen as a reference point to reduce the risk of nerve damage (13). Moreover, relying on anatomical landmarks can be challenging in older patients due to joint degeneration, especially when using CBT in individuals with osteoporosis (1–3, 13, 14). Degeneration can make it difficult to identify the entry point accurately, increasing the risk of nerve damage or operator error (15–21). In addition, using the inferior border of the transverse process as a reference increases surgical trauma and becomes particularly challenging when the transverse processes are non-horizontal, especially in patients with a history of previous surgeries. The lumbar isthmus is one of the most easily observable anatomical features during surgical procedures. Due to its proximity to the midline, the isthmus can be fully exposed in spine surgery. Therefore, Paerhati Rexiti et al. suggested a new reference system for entry points based on isthmus parameters (22). However, individual variability in assessing the tangent and distance from the entry point poses a risk of misjudgment in this reference system, which could potentially lead to screw placement errors.
3D-printing technology, an additive manufacturing technique, has been increasingly applied in orthopaedics to improve surgical accuracy (23–25). Recently, researchers have used 3D- printing to create personalized navigation templates for screw implantation, aiming to achieve greater precision (26–28). Shi et al. reported enhanced safety and accuracy when using 3D-printed templates to assist in CBT screw implantation, compared to traditional CBT screws in osteoporotic specimens (29).
However, to the best of our knowledge, there are few studies comparing the clinical outcomes of using individualized 3D-printing navigation technology vs. the free-hand isthmus method in lumbar CBT screw implantation. The aim of this study was to evaluate the intraoperative and postoperative clinical outcomes between these two surgical methods.
This single-center retrospective study evaluated the clinical outcomes of patients who underwent surgery for CBT screw implantation between September 2020 and August 2023. The inclusion criteria for this study were as follows: (1) a confirmed diagnosis of lumbar degenerative disease (LDD), osteoporosis (T-score < −2.5), and significant low back pain or neurological deficits unresponsive to conservative treatment; (2) underwent CBT screw implantation surgery using either individualized 3D-printing navigation technology or the free-hand isthums method; and (3) availability of pre- and postoperative data with a minimum follow-up period of 12 months. 56 patients met the inclusion criteria and were initially included in this study. The exclusion criteria included pedicle anomalies, lumbar spondylolysis, secondary osteoporosis, previous lumbar surgeries, and other conditions that prevented surgery or follow-up. 15 patients were excluded due to refusal or loss to follow-up (n = 10), chronic pain in other locations (n = 3), the presence of psychosis (n = 1), or an inability to complete the questionnaire (n = 1). In total, 41 patients were included in the final analysis (see Figure 1). The patients were divided into two groups based on the method used for CBT screw implantation: Group A (n = 23) utilized the freehand isthmus technique, while Group B (n = 18) employed the individualized 3D-printing navigation technique (Figure 1). All surgeries were performed by an experienced senior doctor.
In Group A surgeries, the freehand isthmus technique was used to determine the entry point at the intersection of the vertical line extending inward from the isthmus tangent point (ranging from 2.5–5.5 mm, progressively increasing from L1–L5) and the horizontal line of the distal paracentral process. The surrounding bone cortex was ground down, and the screw trajectory was prepared with a head tilt of 25° and an outward tilt of 10°. Mark's localization pin was then placed to fluoroscopically re-confirm the screw trajectory. The trajectory was tapped using a taper 0.5 mm smaller than the planned screw diameter, and a probing pin ensured the integrity of the bone wall within the channel. Once confirmed, screws of the planned length and diameter were inserted along the trajectory, and the procedure proceeded with spinal canal decompression, interbody fusion, and installation of connecting rods as appropriate.
In Group B surgeries, individualized 3D-printing navigation templates were designed using Mimics Medical software version 21.0 (Materialise Corp., Leuven, VBR, Belgium), Magics software version 24.0 (Materialise Corp., Leuven, VBR, Belgium), and 3D Studio MAX 2020 software(Autodesk Corp., San Francisco, CA, USA), and printed using polyamide fiber. Preoperative lumbar CT data were utilized to create 3D models (Figure 2A), plan cortical bone trajectory (CBT) screw paths, and produce navigation guides (Figure 2B). During surgery, the posterior soft tissues of the vertebral body were carefully stripped to expose the bony surface that aligned with the navigation template. The base of the individualized 3D-printing navigation template was securely fitted onto the cleaned bone surface in the surgical field (Figure 2C). One hand stabilized the template by pressing the curved “handle,” while the other used a power drill to slowly and steadily insert the Kirschner wire through the template guide into the bone at the designated entry point. After drilling to the predetermined depth, the navigation template and Kirschner wire were sequentially removed. The Mark positioning needle was then placed to fluoroscopically confirm the screw trajectory. The subsequent steps, consisting of depth measurement, tapping, checking the integrity of the screw trajectory, inserting the appropriately sized screw, and performing additional surgical procedures (including spinal canal decompression, intervertebral fusion, and installation of connecting rods), were conducted in the same manner as in Group A.
Figure 2. Preoperative and intraoperative steps in lumbar CBT screw implantation using individualized 3D-printing navigation templates. (A) 3D-printing navigation templates created using preoperative lumbar CT data; (B) CBT screw trajectories planned based on CT data; and (C) Intraoperative guidance for lumbar CBT screw implantation using individualized 3D-printing navigation technology.
The primary clinical outcome was the accuracy of screw implantation (Figure 3), which was evaluated by three independent specialists who were not involved in the surgeries. In instances of disagreement, a senior specialist, who was also not involved in the surgeries, acted as the final arbitrator. The CT in our study was a 64-row 128-slice spiral CT system (Siemens Shanghai Medical Equipment Co., Ltd.), and the scanning parameters of the CT machine were uniformly set as follows: tube voltage of 120 KV, tube current of 2 mAS, scanning period of 0.6 s, layer thickness of 0.6 mm, and a matrix of 512 × 512.
Figure 3. Comparison of preoperative and postoperative CT scans of patients. (A1) Preoperative CT of Group A patient: female, 70 years old, lumbar disc herniation; (A2) Postoperative CT of Group A patient, through the anterior cortex, Grade 2; (B1) Preoperative CT of Group B patient: female, 66 years old, lumbar disc herniation; (B2) Postoperative CT of Group B patient, acceptably placed screw, Grade 0.
Screw implantation accuracy was assessed using the grading system proposed by Ding et al. (30) as follows: Grade 0: The CBT screw is entirely within the pedicle, or the cortical breach on the medial or lateral side is less than half the diameter of the screw. Grade 1: The CBT screw breaches more than half the screw diameter but does not completely penetrate the medial or lateral cortical wall of the pedicle, or there is penetration of the anterior cortex, endplate, or foraminal region. Grade 2: The CBT screw fully breaches the medial or lateral cortical wall. Grade 0 was defined as acceptable screw implantation. The percentage of Grade 0 screws represented the satisfactory screw implantation rate, calculated using the following formula:
The secondary clinical outcomes included intraoperative details (the number of fluoroscopy exposures, blood loss, and operation duration), and Japanese Orthopaedic Association (JOA) back symptoms scores, which consist of 14 questions in 4 sections: subjective symptoms, clinical signs, limitations in daily activities, and bladder function. JOA score range: 0–29 (a lower score indicates a greater degree of dysfunction) (31), Oswestry Disability Index (ODI) scores range: 0–100 (a higher scores indicating more severe disability), assessed at 3, 6, and 12 months post-operation, along with monitoring for postoperative complications (32). Common postoperative complications include infection, deep vein thrombosis in the lower limbs, cerebrospinal fluid leaks, and intestinal obstruction.
The demographic characteristics of the study population were reported according to the two surgical methods: Individualized 3D-Printing Navigation Technology in Group A and the Free-Hand Isthmus Method in Group B. Categorical variables were presented as case numbers and percentages (%), while continuous variables were presented as mean ± standard deviation (SD). The independent samples t-test was used to compare continuous variables between the two groups, while the Chi-square test was utilized for categorical variables. In addition, the Wilcoxon rank-sum test was used to analyze the difference in the satisfactory rate of screw implantation (graded 0–2, %) between the two groups.
Statistical analyses were performed using SPSS software, version 27.0 (IBM Corp., Armonk, NY, USA). A two-tailed P-value of less than 0.05 was considered statistically significant.
The Local Ethical Committee Study approved this study (number: 2020-ky-45). All participants provided written informed consent prior to undergoing surgical treatment.
A total of 41 patients with an average age of 66.5 ± 6.8 years were included in this study. Of these, 23 (56.1%) underwent free-hand isthmus method (Group A), and 18 (43.9%) underwent individualized 3D-printing navigation technology (Group B) in CBT screw implantation (Table 1). There was no significant difference in age, sex, or BMI between the two patient groups (p = 0.81, 0.44 and 0.50, respectively). In addition, the preoperative T-score, JOA score, ODI score, and the number of implant screws used during surgery were also similar between the two groups (p = 0.52, 0.96, 0.93 and 0.50, respectively).
After surgery, the majority of implanted screws (86.5%) were classified as grade 0, indicating satisfactory implantation (Table 2). In Group A, 13 out of 107 screws (12.1%) were classified as grade 1, while 6 out of 107 (5.6%) were classified as grade 2. The satisfactory screw implantation rate in Group A was 82.2% (88 out of 107). In Group B, the proportion of implanted screws classified as grade 1 (5 out of 78, 6.4%) and grade 2 (1 out of 78, 1.3%) was significantly lower (P = 0.045). Meanwhile, the satisfactory screw implantation rate in Group B was also significantly higher at 92.3% (72 out of 78) (P = 0.048). Additionally, in Group A, the average number of fluoroscopy exposures, blood loss, and surgery duration were 5.3 ± 1.9 times, 241.3 ± 109.4 ml, and 143.1 ± 63.0 min, respectively. In Group B, the number of fluoroscopy exposures (3.1 ± 1.1; P < 0.01) and blood loss (172.2 ± 73.2 ml; P = 0.03) were significantly reduced. Moreover, surgery duration was also shorter in Group B (101.1 ± 47.0 min; P = 0.02).
Table 2. Comparison of screw placement accuracy and intraoperative details between the two surgical methods (n = 41).
Both groups showed significant improvements in postoperative JOA and ODI scores. In Group A, postoperative JOA scores were 15.3 ± 1.9 at 3 months and 19.5 ± 1.1 at 6 months. In contrast, Group B showed significantly higher postoperative JOA scores at both follow-up time points: 16.9 ± 1.9 at 3 months (p = 0.01) and 20.8 ± 1.3 at 6 months (p < 0.01) (Table 3). Similarly, the ODI score at the 3-month follow-up was significantly lower in Group B compared to Group A (24.4 ± 6.3 vs. 28.7 ± 5.3, p = 0.02). However, no significant difference in long-term postoperative outcomes (e.g., at 12 months) was observed between the two groups. Although no significant difference was found in postoperative complications between the two groups, Group B had a lower complication rate than Group A (p = 0.68).
Table 3. Post-operative pain intensity, disability, and complications between the two surgical methods (n = 41).
In this retrospective single-center cohort study, we found the following: (1) compared to the free-hand isthmus method, patients with 3D-printing navigation technology had a higher accuracy in screw implantation; (2) patients with 3D-printing navigation technology tended to have fewer fluoroscopy exposures, less blood loss during surgery and shorter operation duration; (3) compared to the free-hand isthmus method, patients with 3D-printing navigation technology reported lower back pain intensity at 3 and 6 months postoperatively, along with improved physical function at 3 months; (4) there was no significant difference in postoperative complications between the two groups.
Santoni (1) proposed the CBT screw technique in 2009, initially for lumbar pedicle implantation. The CBT screw offers several advantages in comparison with conventional techniques, including smaller tissue dissection, greater holding strength, fewer complications, reduced intraoperative bleeding, and lower postoperative infection rates. As a result, it has become a popular focus in spinal surgery research, particularly for patients with osteoporosis and failed pedicle screws fixation. Compared to the classical entry point for CBT, the free-hand isthmus method is symmetrically curved and easily visible during surgery, providing a reliable reference point for screw implantation. Paerhati Rexiti (22) and other researchers suggested using isthmus parameters for screw implantation to reduce tissue damage and the times for x-ray fluoroscopy during spinal surgery. However, variability in the position of the isthmus can lead to increased difficulty of surgery, potential inaccuracies, and an increased risk of failed screw implantation. To the best of our knowledge, this was the first study to explore whether the use of 3D-printing navigation technology, compared to the free-hand isthmus method, in lumbar CBT screw can improve the accuracy of screw implantation and postoperative clinical outcomes.
This study first demonstrated that an effective accuracy of CBT screws implantation (92.3%) in patients with 3D-printing navigation technology, which is relatively higher than that observed in populations with treated with the free-hand isthmus method (82.2%) and some previously reported (1, 33–35). In Group B, six Grade 1 or Grade 2 screws were identified, with four showing lateral cortical perforation and two showing medial perforation, with most perforations occurring within the first half of the study period. In Group A, among the 19 Grade 1 or Grade 2 screws, 14 showed lateral perforations, 4 demonstrated medial perforations and 1 through the anterior cortex. The concept of a “safety zone” between screw perforation and neurovascular complications is well-studied. Generally, a medial cortical perforation margin of up to 4 mm is considered safe (13), while pedicle cortical perforations under 2 mm are deemed acceptable (22, 36, 37), with recommended thresholds of less than 5 mm for medial perforations and less than 6 mm for lateral perforations (22). Anatomically, screws placed medially increase the risk of neurological complications, which leads most surgeons to favor a lateral approach to screw placement. This preference aligns with the higher incidence of lateral cortical perforations observed in this study.
In our study, the accuracy of CBT screw placement using individualized 3D-printing navigation templates exceeded that reported by Federica Penner et al. (38) for single-segment fixation (91.8%), which applied pre-operative CT scan with 3D reconstruction for planning the surgical plan, as well as the results obtained by Kaito et al. (39) and Ke Wang et al. (40) in cadaveric studies using 3D-printing navigation templates (91.4% and 91.6%, respectively). Additionally, compared to Lamartina et al. (41), who reported a 91% accuracy rate for pedicle screws using 3D-printing guides, our results demonstrated a further advantage. However, our accuracy was still lower than that reported by Yue Li et al. (42), where robotic guidance was used to achieve 93% of screws fully contained within the pedicle. However, the use of 3D-printing navigation molds has significant cost advantages over the use of robots for navigation (43).
Our intraoperative observations suggest this discrepancy may stem from inherent instability in the guided manufacturing and application process. Each step—image acquisition, segmentation, 3D modelling, base construction, surface preparation, and guide placement—requires manual intervention, making total standardization challenging. Even minor errors at any stage can create small gaps between the guide and bone surface, reducing stability and affecting screw positioning accuracy.
In our study, except for two patients in Group B, where the guide did not fit the prepared bone surface, requiring a switch to freehand placement, all other navigation templates fit securely. During drilling, the template bases remained firmly fixed, ensuring stable placement. All 185 screws were inserted in both groups successfully without trajectory overlapping.
To further optimize guide stability, surgeons should carefully remove the soft tissue from the bone surface, minimize unnecessary bone damage, and manage osteophytes to ensure a secure fit for the 3D-printing navigation templates.
Meanwhile, patients who underwent surgery with individualized 3D-printing navigation technology showed reduced surgery time, fewer intraoperative fluoroscopy instances, and decreased blood loss. The potential reasons may be attributed to both isthmus parameters and the traditional method of free-hand CBT screw implantation is subjective dependent on the surgeon's experience (44). In contrast, the use of individualized 3D-printing navigation technology allows the surgeon to comprehensively assess the patient's anatomical structure and precisely plan the optimal screw placement path in the preoperative phase (45). 3D-printing guides translate predefined screw placement trajectories directly to the surgical site using solid templates (46). The guide plate features preset apertures that direct the instruments along the correct path with greater accuracy, reducing the potential for errors in the position and angle of screw placement, and mitigating the influence of human factors on the placement trajectory. Moreover, the use of individualized 3D-printing navigation technology reduces the need for intraoperative fluoroscopy to assist with screw positioning, contributing to shorter surgery duration and reduced blood loss (47).
Individuals with lumbar degenerative disease often experienced low back pain and pain related physical disabilities (48). The ODI score, a commonly used measure to assess function in lumbar spine diseases, and the JOA score, a standardized scoring system for human dysfunction, have been widely used to assess lumbar spine function in patients undergoing lumbar fusion and internal lumbar fixation surgery, as an accurate representation of a patient's postoperative recovery (49, 50). In our study, we utilized these scores to assess patient clinical outcomes. The findings of this study indicated that both patient groups experienced significant improvements in pain intensity and pain related physical status, as reflected in their JOA and ODI scores compared to preoperative levels. Specifically, compared with the preoperative status, JOA scores in both groups showed a gradual increase at 3, 6, and 12 months postoperatively, with ODI scores demonstrating a gradual decrease over the same follow-up period, suggesting a favorable surgical outcome, with patients' functional status improving over time, and indicates that postoperative recovery is a progressive process influenced by various factors, which are in line with previous studies (51–53). However, no significant differences were observed between the two groups in ODI and JOA score improvements at all follow-ups except the third month postoperatively (P > 0.05), which suggested individualized 3D-printing navigation technology is more beneficial to the short-term outcome of patients but does not affect the long-term outcome of patients significantly. These results may be attributed to several factors: (1) reduced surgical dissection with 3D-printing navigation technology likely minimized surrounding tissues and nerves damage, facilitating better short-term functional recovery and reduced postoperative pain; (2) while precise initial placement was crucial for immediate stability, its impact on long-term recovery may diminish as healing and bone fusion progress similarly in both groups, with long-term changes in JOA and ODI scores influenced by factors including preoperative condition, decompression efficacy, disease duration, and fixation stability, which can offset the early advantages offered by 3D-printing navigation technology. Given the comparable baseline characteristics in both groups, no significant differences were observed at long-term follow-up.
CBT screws were a widely used and effective method for lumbar spine fixation. However, their successful application required a steep learning curve, as surgical outcomes were heavily dependent on the surgeon's expertise. Furthermore, anatomical variations in lumbar vertebrae can affect the accuracy of screw implantation. This study was the first to directly compare the efficacy of 3D-printing navigation technology with the traditional free-hand isthmus technique for CBT screw insertion. Our results suggested that 3D-printing navigation optimizes the surgical process by enabling the preoperative design of the screw path based on patient-specific imaging. However, this approach had some limitations, including the need for advanced planning, additional printing time, and higher costs, making it less suitable for emergency cases.
There were also some limitations in our study. First, the retrospective study design may have led to selection bias and recall bias. Second, the sample size and the fact that all surgeries were performed by the same experienced team from a single institute may limit the generalizability of our findings. Third, the patients included in the analysis had no significant comorbidities and were considered to be at low surgical risk. The study excluded patients with secondary osteoporosis, a history of previous lumbar surgery, infections, or tumors. These factors may affect the external validity of the study results. Finally, this study included only a one-year follow-up for all postoperative patients, limiting its ability to report long-term outcomes and complications. Future research should include a larger and more diverse population and incorporate blinding and well-randomized prospective studies. Once the application of individualized 3D-printing navigation templates for lumbar CBT screws becomes fully established, future studies should focus on further advancements in minimally invasive techniques and expand their use to higher-risk surgical areas, including percutaneous navigation templates and templates for the thoracic and cervical spine. These advancements aim to minimize intraoperative tissue damage, reduce surgical pain and risks, lower surgical costs, and accelerate postoperative recovery.
Our comparative analysis shows that patients receiving CBT screw implantation with individualized 3D-printing navigation technology achieve higher accuracy, improved operative efficiency, and better clinical outcomes compared to the freehand isthmus method. This new surgical technique could serve as a potential option for patients requiring CBT screw implantation.
The raw data supporting the conclusions of this article will be made available by the authors, without undue reservation.
The studies involving humans were approved by Ethics Committee of Affiliated Nanhua Hospital. The studies were conducted in accordance with the local legislation and institutional requirements. The participants provided their written informed consent to participate in this study. Written informed consent was obtained from the individual(s) for the publication of any potentially identifiable images or data included in this article.
HH: Data curation, Software, Writing – original draft, Formal analysis. ZL: Methodology, Writing – review & editing. JW: Writing – review & editing, Supervision, Funding acquisition. JO: Funding acquisition, Methodology, Writing – review & editing. XG: Data curation, Software, Writing – review & editing, Formal analysis.
The author(s) declare financial support was received for the research and/or publication of this article. The study was supported by the Health Commission of Hunan Province (grant number: 202104071421) and Clinical Medical Technology Innovation Project of Hunan Province (grant number: 2021sk51910).
This is a short text to acknowledge the contributions of specific colleagues, institutions, or agencies that aided the efforts of the authors.
The authors declare that the research was conducted in the absence of any commercial or financial relationships that could be construed as a potential conflict of interest.
The author(s) declare that no Generative AI was used in the creation of this manuscript.
All claims expressed in this article are solely those of the authors and do not necessarily represent those of their affiliated organizations, or those of the publisher, the editors and the reviewers. Any product that may be evaluated in this article, or claim that may be made by its manufacturer, is not guaranteed or endorsed by the publisher.
1. Santoni BG, Hynes RA, McGilvray KC, Rodriguez-Canessa G, Lyons AS, Henson MA, et al. Cortical bone trajectory for lumbar pedicle screws. Spine J. (2009) 9(5):366–73. doi: 10.1016/j.spinee.2008.07.008
2. Ueno M, Sakai R, Tanaka K, Inoue G, Uchida K, Imura T, et al. Should we use cortical bone screws for cortical bone trajectory? J Neurosurg Spine. (2015) 22(4):416–21. doi: 10.3171/2014.9.SPINE1484
3. Baluch DA, Patel AA, Lullo B, Havey RM, Voronov LI, Nguyen NL, et al. Effect of physiological loads on cortical and traditional pedicle screw fixation. Spine. (2014) 39(22):E1297–302. doi: 10.1097/BRS.0000000000000553
4. Seebeck J, Goldhahn J, Stadele H, Messmer P, Morlock MM, Schneider E. Effect of cortical thickness and cancellous bone density on the holding strength of internal fixator screws. J Orthop Res. (2004) 22(6):1237–42. doi: 10.1016/j.orthres.2004.04.001
5. Chao CK, Hsu CC, Wang JL, Lin J. Increasing bending strength and pullout strength in conical pedicle screws: biomechanical tests and finite element analyses. J Spinal Disord Tech. (2008) 21(2):130–8. doi: 10.1097/BSD.0b013e318073cc4b
6. Krenn MH, Piotrowski WP, Penzkofer R, Augat P. Influence of thread design on pedicle screw fixation. Laboratory investigation. J Neurosurg Spine. (2008) 9(1):90–5. doi: 10.3171/SPI/2008/9/7/090
7. Matsukawa K, Yato Y, Nemoto O, Imabayashi H, Asazuma T, Nemoto K. Morphometric measurement of cortical bone trajectory for lumbar pedicle screw insertion using computed tomography. J Spinal Disord Tech. (2013) 26(6):E248–53. doi: 10.1097/BSD.0b013e318288ac39
8. Kanno H, Onoda Y, Hashimoto K, Aizawa T, Ozawa H. Innovation of surgical techniques for screw fixation in patients with osteoporotic spine. J Clin Med. (2022) 11(9):2577. doi: 10.3390/jcm11092577
9. Liu D, Kahaer A, Wang Y, Zhang R, Maiaiti A, Maimaiti X, et al. Comparison of CT values in traditional trajectory, traditional cortical bone trajectory, and modified cortical bone trajectory. BMC Surg. (2022) 22(1):441. doi: 10.1186/s12893-022-01893-5
10. Ueno M, Imura T, Inoue G, Takaso M. Posterior corrective fusion using a double-trajectory technique (cortical bone trajectory combined with traditional trajectory) for degenerative lumbar scoliosis with osteoporosis: technical note. J Neurosurg Spine. (2013) 19(5):600–7. doi: 10.3171/2013.7.SPINE13191
11. Matsukawa K, Yato Y, Kato T, Imabayashi H, Asazuma T, Nemoto K. In vivo analysis of insertional torque during pedicle screwing using cortical bone trajectory technique. Spine. (2014) 39(4):E240–5. doi: 10.1097/BRS.0000000000000116
12. Glennie RA, Dea N, Kwon BK, Street JT. Early clinical results with cortically based pedicle screw trajectory for fusion of the degenerative lumbar spine. J Clin Neurosci. (2015) 22(6):972–5. doi: 10.1016/j.jocn.2015.01.010
13. Iwatsuki K, Yoshimine T, Ohnishi Y, Ninomiya K, Ohkawa T. Isthmus-guided cortical bone trajectory for pedicle screw insertion. Orthop Surg. (2014) 6(3):244–8. doi: 10.1111/os.12122
14. Chen W, Wang H, Jiang J, Lyu F, MA X, Xia X. Anatomic study on lumbar cortical bone trajectory of adults. Chin J Orthop. (2015) 12:1213–21. doi: 10.3760/cma.j.issn.0253-2352.2015.12.006
15. Kalichman L, Hunter DJ. Lumbar facet joint osteoarthritis: a review. Semin Arthritis Rheum. (2007) 37(2):69–80. doi: 10.1016/j.semarthrit.2007.01.007
16. Jaumard NV, Welch WC, Winkelstein BA. Spinal facet joint biomechanics and mechanotransduction in normal, injury and degenerative conditions. J Biomech Eng. (2011) 133(7):071010. doi: 10.1115/1.4004493
17. Varlotta GP, Lefkowitz TR, Schweitzer M, Errico TJ, Spivak J, Bendo JA, et al. The lumbar facet joint: a review of current knowledge: part 1: anatomy, biomechanics, and grading. Skeletal Radiol. (2011) 40(1):13–23. doi: 10.1007/s00256-010-0983-4
18. Gellhorn AC, Katz JN, Suri P. Osteoarthritis of the spine: the facet joints. Nat Rev Rheumatol. (2013) 9(4):216–24. doi: 10.1038/nrrheum.2012.199
19. Izzo R, Guarnieri G, Guglielmi G, Muto M. Biomechanics of the spine. Part I: spinal stability. Eur J Radiol. (2013) 82(1):118–26. doi: 10.1016/j.ejrad.2012.07.024
20. Manchikanti L, Kaye AD, Soin A, Albers SL, Beall D, Latchaw R, et al. Comprehensive evidence-based guidelines for facet joint interventions in the management of chronic spinal pain: American society of interventional pain physicians (ASIPP) guidelines facet joint interventions 2020 guidelines. Pain Physician. (2020) 23(3S):S1–S127. doi: 10.36076/ppj.2020/23/s1
21. Wu PH, Kim HS, Jang IT. Intervertebral disc diseases part 2: a review of the current diagnostic and treatment strategies for intervertebral disc disease. Int J Mol Sci. (2020) 21(6):2135. doi: 10.3390/ijms21062135
22. Rexiti P, Abudurexiti T, Abuduwali N, Wang S, Sheng W. Measurement of lumbar isthmus parameters for novel starting points for cortical bone trajectory screws using computed radiography. Am J Transl Res. (2018) 10(8):2413–23.30210680
23. Carletti E, Motta A, Migliaresi C. Scaffolds for tissue engineering and 3D cell culture. Methods Mol Biol. (2011) 695:17–39. doi: 10.1007/978-1-60761-984-0_2
24. Hoque ME, Chuan YL, Pashby I. Extrusion based rapid prototyping technique: an advanced platform for tissue engineering scaffold fabrication. Biopolymers. (2012) 97(2):83–93. doi: 10.1002/bip.21701
25. Fidanza A, Perinetti T, Logroscino G, Saracco M. 3D printing applications in orthopaedic surgery: clinical experience and opportunities. Appl Sci. (2022) 12(7):3245. doi: 10.3390/app12073245
26. Sugawara T, Higashiyama N, Kaneyama S, Takabatake M, Watanabe N, Uchida F, et al. Multistep pedicle screw insertion procedure with patient-specific lamina fit-and-lock templates for the thoracic spine: clinical article. J Neurosurg Spine. (2013) 19(2):185–90. doi: 10.3171/2013.4.SPINE121059
27. Chen YY, Chao LC, Fang JJ, Lee EJ. 3D-customized guiding template for posterior fixation in complex atlantoaxial instability-preliminary experiences of national cheng kung university hospital. J Neurol Surg Rep. (2020) 81(1):e20–e7. doi: 10.1055/s-0039-1695795
28. Bundoc RC, Delgado GG, Grozman SA. A novel patient-specific drill guide template for pedicle screw insertion into the subaxial cervical spine utilizing stereolithographic modelling: an in vitro study. Asian Spine J. (2017) 11(1):4–14. doi: 10.4184/asj.2017.11.1.4
29. Shi W, Aierken G, Wang S, Abuduwali N, Xia Y, Rezhake R, et al. Application study of three-dimensional printed navigation template between traditional and novel cortical bone trajectory on osteoporosis lumbar spine. J Clin Neurosci. (2021) 85:41–8. doi: 10.1016/j.jocn.2020.11.038
30. Ding H, Han B, Hai Y, Liu Y, Guan L, Pan A, et al. The feasibility of assessing the cortical bone trajectory screw placement accuracy using a traditional pedicle screw insertion evaluation system. Clin Spine Surg. (2021) 34(2):E112–E20. doi: 10.1097/BSD.0000000000001059
31. Kawakami M, Takeshita K, Inoue G, Sekiguchi M, Fujiwara Y, Hoshino M, et al. Japanese orthopaedic association (JOA) clinical practice guidelines on the management of lumbar spinal stenosis, 2021—secondary publication. J Orthop Sci. (2023) 28(1):46–91. doi: 10.1016/j.jos.2022.03.013
32. Roland M, Fairbank J. The roland-morris disability questionnaire and the oswestry disability questionnaire. Spine. (2000) 25(24):3115–24. doi: 10.1097/00007632-200012150-00006
33. Matsukawa K, Yato Y, Imabayashi H, Hosogane N, Asazuma T, Nemoto K. Biomechanical evaluation of the fixation strength of lumbar pedicle screws using cortical bone trajectory: a finite element study. J Neurosurg Spine. (2015) 23(4):471–8. doi: 10.3171/2015.1.SPINE141103
34. Lieberman IH, Togawa D, Kayanja MM, Reinhardt MK, Friedlander A, Knoller N, et al. Bone-mounted miniature robotic guidance for pedicle screw and translaminar facet screw placement: part I–technical development and a test case result. Neurosurgery. (2006) 59(3):641–50. doi: 10.1227/01.NEU.0000229055.00829.5B
35. Reid PC, Morr S, Kaiser MG. State of the union: a review of lumbar fusion indications and techniques for degenerative spine disease. J Neurosurg Spine. (2019) 31(1):1–14. doi: 10.3171/2019.4.SPINE18915
36. Matsukawa K, Yato Y, Imabayashi H, Hosogane N, Abe Y, Asazuma T, et al. Biomechanical evaluation of fixation strength among different sizes of pedicle screws using the cortical bone trajectory: what is the ideal screw size for optimal fixation? Acta Neurochir). (2016) 158(3):465–71. doi: 10.1007/s00701-016-2705-8
37. Dayani F, Chen YR, Johnson E, Deb S, Wu Y, Pham L, et al. Minimally invasive lumbar pedicle screw fixation using cortical bone trajectory—screw accuracy, complications, and learning curve in 100 screw placements. J Clin Neurosci. (2019) 61:106–11. doi: 10.1016/j.jocn.2018.10.131
38. Penner F, Marengo N, Ajello M, Petrone S, Cofano F, Santonio FV, et al. Preoperative 3D CT planning for cortical bone trajectory screws: a retrospective radiological cohort study. World Neurosurg. (2019) 126:e1468–e74. doi: 10.1016/j.wneu.2019.03.121
39. Kaito T, Matsukawa K, Abe Y, Fiechter M, Zhu X, Fantigrossi A. Cortical pedicle screw placement in lumbar spinal surgery with a patient-matched targeting guide: a cadaveric study. J Orthop Sci. (2018) 23(6):865–9. doi: 10.1016/j.jos.2018.06.005
40. Wang K, Zhang Z-J, Chen J-X, Wu A-M, Wang X-Y, Sheng S-R. Design and application of individualized, 3-dimensional-printed navigation template for placing cortical bone trajectory screws in middle-upper thoracic spine: cadaver research study. World Neurosurg. (2019) 125:e348–e52. doi: 10.1016/j.wneu.2019.01.076
41. Lamartina C, Cecchinato R, Fekete Z, Lipari A, Fiechter M, Berjano P. Pedicle screw placement accuracy in thoracic and lumbar spinal surgery with a patient-matched targeting guide: a cadaveric study. Eur Spine J. (2015) 24:937–41. doi: 10.1007/s00586-015-4261-y
42. Li Y, Chen L, Liu Y, Ding H, Lu H, Pan A, et al. Accuracy and safety of robot-assisted cortical bone trajectory screw placement: a comparison of robot-assisted technique with fluoroscopy-assisted approach. BMC Musculoskelet Disord. (2022) 23(1):328. doi: 10.1186/s12891-022-05206-y
43. Bui T, Ruiz-Cardozo MA, Dave HS, Barot K, Kann MR, Joseph K, et al. Virtual, augmented, and mixed reality applications for surgical rehearsal, operative execution, and patient education in spine surgery: a scoping review. Medicina (B Aires). (2024) 60(2):332. doi: 10.3390/medicina60020332
44. Rexiti P, Aierken A, Sadeer A, Wang S, Abuduwali N, Deng Q, et al. Anatomy and imaging studies on cortical bone screw freehand placement applying anatomical targeting technology. Orthop Surg. (2020) 12(6):1954–62. doi: 10.1111/os.12775
45. Eltes PE, Kiss L, Bartos M, Gyorgy ZM, Csakany T, Bereczki F, et al. Geometrical accuracy evaluation of an affordable 3D printing technology for spine physical models. J Clin Neurosci. (2020) 72:438–46. doi: 10.1016/j.jocn.2019.12.027
46. Lador R, Regev G, Salame K, Khashan M, Lidar Z. Use of 3-dimensional printing technology in complex spine surgeries. World Neurosurg. (2020) 133:e327–e41. doi: 10.1016/j.wneu.2019.09.002
47. Garg B, Mehta N. Current status of 3D printing in spine surgery. J Clin Orthop Trauma. (2018) 9(3):218–25. doi: 10.1016/j.jcot.2018.08.006
48. Mobbs RJ, Phan K, Malham G, Seex K, Rao PJ. Lumbar interbody fusion: techniques, indications and comparison of interbody fusion options including PLIF, TLIF, MI-TLIF, OLIF/ATP, LLIF and ALIF. J Spine Surg. (2015) 1(1):2–18. doi: 10.3978/j.issn.2414-469X.2015.10.05
49. Hu JN, Yang XF, Li CM, Li XX, Ding YZ. Comparison of cortical bone trajectory versus pedicle screw techniques in lumbar fusion surgery: a meta-analysis. Medicine. (2019) 98(33):e16751. doi: 10.1097/MD.0000000000016751
50. Qiu L, Niu F, Wu Z, Zhang W, Chen F, Tan J, et al. Comparative outcomes of cortical bone trajectory screw fixation and traditional pedicle screws in lumbar fusion: a meta-analysis. World Neurosurg. (2022) 164:e436–e45. doi: 10.1016/j.wneu.2022.04.129
51. Wu J, Lin T, Jiang H, Ma J, Zhang K, Zhao J, et al. The treatment efficacy of cortical bone trajectory (CBT) pedicle screws for lumbar degenerative disease in the Chinese Han population. Front Surg. (2022) 9:421815. doi: 10.3389/fsurg.2022.421815
52. Guo J, Xu G, Li S, Li Z, Liu J, Wang W, et al. Impact of lumbar fusion internal fixation on lumbar disc herniation in young patients: a retrospective study. Med Sci Monit. (2024) 30:e944570. doi: 10.12659/MSM.944570
53. Ding H, Hai Y, Liu Y, Guan L, Pan A, Zhang X, et al. Cortical trajectory fixation versus traditional pedicle-screw fixation in the treatment of lumbar degenerative patients with osteoporosis: a prospective randomized controlled trial. Clin Interv Aging. (2022) 17:175–84. doi: 10.2147/CIA.S349533
Keywords: cortical bone trajectory screw, 3D-printing navigation molds, free-hand isthmus method, lumbar internal fixation surgery, retrospective study
Citation: Hu H, Lu Z, Gao X, Ou J and Wang J (2025) Comparative study of individualized 3D-printing navigation technology and free-hand isthmus method in lumbar CBT screw implantation. Front. Surg. 12:1520481. doi: 10.3389/fsurg.2025.1520481
Received: 31 October 2024; Accepted: 14 March 2025;
Published: 27 March 2025.
Edited by:
Osvaldo Mazza, Bambino Gesù Children's Hospital (IRCCS), ItalyReviewed by:
Weiyang Zhong, First Affiliated Hospital of Chongqing Medical University, ChinaCopyright: © 2025 Hu, Lu, Gao, Ou and Wang. This is an open-access article distributed under the terms of the Creative Commons Attribution License (CC BY). The use, distribution or reproduction in other forums is permitted, provided the original author(s) and the copyright owner(s) are credited and that the original publication in this journal is cited, in accordance with accepted academic practice. No use, distribution or reproduction is permitted which does not comply with these terms.
*Correspondence: Jiong Wang, Mjg0MjI4MTkwQHFxLmNvbQ==; Jun Ou, MTk3NjQ0OTc4OUBxcS5jb20=; Xiaowen Gao, Nzg1OTUwMzUzQHFxLmNvbQ==
Disclaimer: All claims expressed in this article are solely those of the authors and do not necessarily represent those of their affiliated organizations, or those of the publisher, the editors and the reviewers. Any product that may be evaluated in this article or claim that may be made by its manufacturer is not guaranteed or endorsed by the publisher.
Research integrity at Frontiers
Learn more about the work of our research integrity team to safeguard the quality of each article we publish.