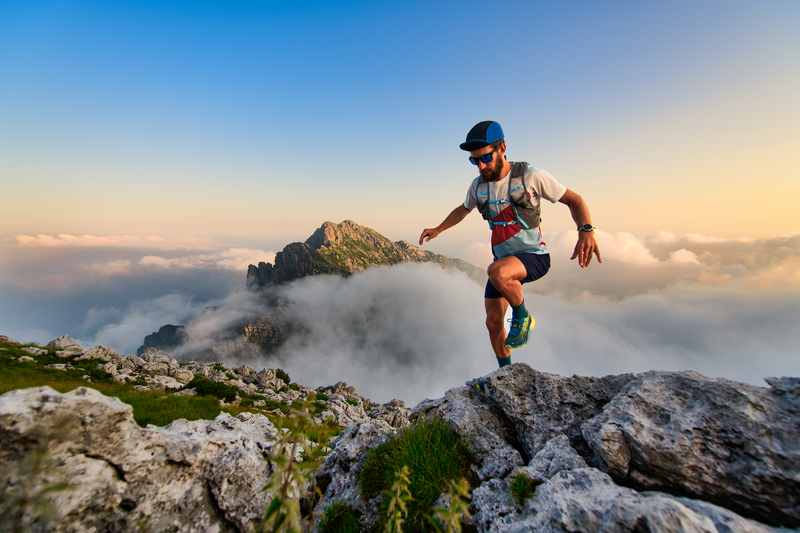
95% of researchers rate our articles as excellent or good
Learn more about the work of our research integrity team to safeguard the quality of each article we publish.
Find out more
MINI REVIEW article
Front. Surg. , 01 July 2022
Sec. Neurosurgery
Volume 9 - 2022 | https://doi.org/10.3389/fsurg.2022.929871
This article is part of the Research Topic Chronic Carotid Artery Occlusion: Surgical Techniques, Screening modalities, Evaluation Scale, Prescription, and Beyond View all 8 articles
Moyamoya disease (MMD) is a rare cerebrovascular disease characterized by progressive stenosis of large intracranial arteries and a hazy network of basal collaterals called moyamoya vessels. The etiology and pathogenesis of MMD are still obscure. The biggest obstacles in the basic research of MMD are difficulty in obtaining specimens and the lack of an animal model. It is necessary to use appropriate and rationally designed animal models for the correct evaluation. Several animal models and methods have been developed to produce an effective MMD model, such as zebrafish, mice and rats, rabbits, primates, felines, canines, and peripheral blood cells, each with advantages and disadvantages. There are three mechanisms for developing animal models, including genetic, immunological/inflammatory, and ischemic animal models. This review aims to analyze the characteristics of currently available models, providing an overview of the animal models framework and the convenience of selecting model types for MMD research. It will be a great benefit to identify strategies for future model generations.
Moyamoya disease (MMD) is a chronic cerebrovascular disease characterized by progressive occlusion of the terminal internal carotid artery (ICA) and the secondary formation of collateral vessels. Suzuki and Takaku first described this rare vascular disease (1). The term “moyamoya” refers to the unusual vascular collaterals at the base of the brain. Cerebral angiography is the gold standard investigation of MMD for diagnosis contrast to the tumors. MMD is found worldwide; China, Korea, and Japan have the highest incidence around the world (2). To date, the detailed underlying etiology and pathogenesis mechanisms are still poorly understood. Except for progressive narrowing of the ICA and circle of Willis, cortical microvascularization is a specific finding; increased microvascular density and diameter were observed in patients. However, neither the nature of moyamoya vessels, “newly formed” perforator arteries, nor the remodeling and dilatation of pre-existing vascular channels has not been determined. The histopathological changes in the distal ICA reported hyperplastic smooth muscle cells or endothelium and stenotic or occlusive lesions related to the fibrocellular thickening of the intima (3); pathological findings include fibrin deposition, elastic layer fragments, and media weakening. It is a fact that the difficulty in obtaining specimens and the lack of an animal model make it difficult to carry out the mechanism research of MMD.
Currently, much attention has been paid to the animal models for MMD. However, few articles focus on the current situation. Using appropriate and rational animal models is necessary for the correct evaluation. Numerous animal species and methods have been developed to produce an effective MMD model, each with its advantages and disadvantages. We will discuss the current state of experimental animal models for MMD in this review. As far as we know, this is the first attempt to categorize MMD models based on the animal species.
The umbrella review was based on the internationally accepted Preferred Reporting Items for Systematic Reviews and Meta-Analyses guidelines. The review protocol was not registered.
In January 2022, a literature search was performed on PubMed, Scopus, Web of Science, and Google Scholar databases. The following search terms with various combinations were used: “moyamoya,” “animal,” “model,” “zebrafish,” “rat,” “mouse,” “cat,” “rabbit,” “monkey,” “dog,” “pig,” and “cell.”
Articles were included for analysis of animal models to explicitly address MMD pathogenesis and/or to evaluate experimental treatments relevant to MMD. Articles were excluded if the animal model did not explicitly assess the cerebrovasculature.
Two reviewers screened independently, and duplicates were removed manually. Results were grouped based on species. This review’s dominating points cover data regarding animal model species, techniques, assessment methods, relevant histopathology, and outcomes (Figure 1).
Overall, 1,099 papers were identified via searching. Forty-eight articles satisfied the inclusion and exclusion criteria and were reviewed for data collection. We categorized the animal models described in each study into eight groups, which will be discussed separately (data are summarized in Supplementary Table 1), including zebrafish, mice and rats, rabbits, monkeys, cats, dogs, pigs, and peripheral blood cell models (Figure 2).
Figure 2. Eight animal models summarized by species, including zebrafish, mouse/rat, rabbit, cat, monkey, dog, miniature, and cellular models.
It is well established that around 10%–15% of patients with MMD have a family history of MMD, while most cases are sporadic (4). The ring finger protein 213 (RNF213) gene is the first identified susceptibility gene in east Asian populations (5). Recently, the RNF213 variant was also demonstrated to be related to the pathogenesis of various systemic vasculopathies within the pulmonary and cardiac vasculature (6, 7). Through the method of editing RNF213 gene, a series of experimental models were established in animal studies.
Zebrafish provides an ideal animal model for understanding the pathophysiology of human diseases (8). Zebrafish biology provides convenience for real-time imaging of developing pathologies, and researchers have ready access to all developmental stages of zebrafish. Given this, a wide range of zebrafish models has been generated for human diseases. Also, zebrafish is more economic than other vertebrate systems.
An RNF213 knockdown zebrafish model was generated by Liu et al., and the effects of RNF213 suppression on vasculature were investigated (9). Similar to the abnormal basal vasculature in MMD, knockdown zebrafish exhibited abnormal vascular development in the head but relatively normal vasculature in the trunk. The model specifically developed multiple aberrant vessels with irregular diameters originating from the inner optic circle and connecting to cranial vessels. Wen et al. (10) successfully generated RNF213a mutant zebrafish using the transcription activator-like effector nuclease (TALEN) technique. Abnormal vascular development in the cranial was observed. The in vivo model for MMD suggests that RNF213 plays a crucial, selective role in intracranial angiogenesis. These zebrafish models would contribute to understanding the role of RNF213a in angiogenesis.
Given the promising results demonstrated in zebrafish, attempts at creating a murine MMD model through RNF213 were conducted. Murine models are the most commonly used laboratory animals, with a variety of methods employed to measure the development of MMD.
Homozygous knockout mice (RNF213−/−) were generated by deleting exon 32 of RNF213 using the Cre–Lox system by Snonobe et al. (11). In contrast to the effect of RNF213 knockdown in zebrafish, radiological evaluation through MRA of RNF213−/− mice did not reveal any gross malformations in the circle of Willis development or significant difference in thickness of vascular wall compared with wild-type mice. In a corroborating study with RNF213 knock-in mice, Kanoke et al. (12) found no significant difference in the circle of Willis anatomy or MRA findings. Additional genes implicated in MMD include ACTA2 and NEO1. The former encodes α-smooth muscle actin, while the latter encodes a transmembrane receptor or coreceptor for multiple ligands. Starosolski et al. (13) identified narrowing of the circle of Willis and abnormal straightening of large vessels in ACTA2 mutant mice similar to the clinical pattern observed in MMD. NEO1-deficient mice have also recently been shown to have moyamoya-like vasculopathy, particularly the development of small, leaky, thin blood vessels within the cerebral cortex (14). The continued identification of susceptibility genes for MMD will provide novel genetic targets for the generation of animal models.
Except for gene mutations, inflammatory and infectious insults are postulated to be amplifying pathogenic mechanisms. Suzuki et al. (15) described the Wistar rats model that utilized intravenous or intrathecal injection of MDP and identified MMD-like pathological changes. Disruption of the internal elastic lamina and medial degeneration were present mainly in the intracranial ICA. However, this model failed to induce intimal thickening and stenosis. Yamada et al. (16) examined both the serum of MMD patients and the influence of inflammation in rats. In serum studies, elevated levels of several immunological factors were detected in MMD. In in vivo studies, Propionibacterium acnes was injected bilaterally around the carotid bifurcation in 7-day-old rats for 4 weeks. Histopathologically, moyamoya-like changes were demonstrated in intracranial ICAs. However, intimal thickening, medial thinning, and stenosis were not appreciated. While this model appears to recapitulate certain aspects of MMD, it fails to induce the progressive steno-occlusion and ischemia that are the hallmark consequence.
Given the underlying pathophysiological similarity between chronic vascular cognitive diseases and MMD, models of chronic hypoperfusion have been the mainstay for uncovering the brain’s response to ischemia and evaluating the effectiveness of surgical revascularization. For two-vessel occlusion of the bilateral common carotid arteries (CCAs) or ICAs, occlusion is usually achieved by ligation with 3-0 (rats) to 6-0 (mice) silk or nylon sutures of bilateral CCAs proximal to the bifurcation or distal ICAs (17–31). Single-vessel occlusion of the unilateral CCAs or ICAs is usually achieved by suture ligation of unilateral ICA (11, 12, 32–37). A series of ischemic consequences and/or treatments are described in these studies, such as growth factor application, EMS or burr hole surgery, and genetic therapies. However, the cerebral blood flow (CBF) decreases rapidly, produces an acute deficit, and is associated with high mortality up to 63% (25), especially in bilateral ligations of the CCAs. Thus, Mansour et al. developed a new modified CCA occlusion model, in which Sprague–Dawley rats underwent left CCA suture ligation and right CCA stenosis (26). A gradual CBF decline in the model results in a low mortality rate (2.3%) and cognitive impairment. With the discrepancy between the transient nature of these models and global progressive steno-occlusion MMD pathophysiology, the transient global ischemia (TGI) model was induced by occluding the CCAs for 5 min coupled with severe hypotension (38). Recently, Wang et al. (39) pioneered the implementation of a bilateral carotid artery stenotic mechanism compared to a bilateral carotid artery occlusion procedure in rats. From the microcoil perspective, Shibata et al. successfully induced CCH in C57BL/6 mice through bilateral carotid artery stenosis using 0.18-mm microcoils (40). Besides, Roberts et al. demonstrated a novel internal carotid artery stenosis (ICAS) surgical technique to simulate MMS by placing microcoils on the proximal ICA in C57BL/6 mice (41). These results showed a significant narrowing of the distal ICA and anterior cerebral artery (ACA) in the circle of Willis and successfully mimicked the moyamoya-like vasculopathy seen in Suzuki stage I MMD as observed in humans. Importantly, it provides the first animal model created through surgical interventions specific for moyamoya vasculopathy. However, it is notable that the histological and pathological vascular profiles of these models do not fit those of MMD precisely. Despite the limitations of these animal models, they play a crucial role in the understanding of MMD.
The concept that one method alone may not be sufficient to promote or induce MMD has led to a discussion of combining different approaches. The work to unite the genetic and surgical pathways may be worthwhile to examine the effect of CCH in RNF213 knockout mice. RNF213 knockout mice that underwent CCA ligation demonstrated thinner intimal and medial layers compared with the control group (P < 0.05) (11). Ito et al. (42) implemented a transient middle cerebral artery occlusion tMCAO) model in C57BL/6 RNF213 knockout mice and observed increased systemic angiogenesis because of the lack of an ideal MMD model that replicates both cerebral hypoperfusion along with angiogenesis at the base of the brain. A combined immunological–genetic model was reported by Kanoke et al., in which RNF213−/− mice were administered with adjuvants to determine whether an immunological reaction is an influential secondary insult in the development of MMD (43). However, the immunological stimulation failed to induce characteristic findings of MMD on gross inspection of the circle of Willis and by MRA of the cerebrovasculature. Morimoto et al. (44) performed bilateral CCA stenosis surgery using external microcoils on RNF213 knockout and vascular endothelial cell-specific RNF213 mutant transgenic mice; CBF restoration on day 28 and angiogenesis were significantly impaired in both groups compared with wild-type mice. Additionally, using the ICAS model pioneered by Roberts et al., the combination of such models with a transgenic mouse that expresses autoimmune disease could provide a model to understand both the disease progression and potential treatment modalities for moyamoya vasculopathy (41).
Various early research studies indicated abnormal thrombogenesis and immune complex deposition within the vasculature of affected patients. Based on histopathological evidence confirming the presence of leukocytes and proliferating smooth muscle cells within the thickened intima of stenotic intracranial arteries from patients (45), MMD has been considered a variant of the vasculitic syndrome and/or autoimmune disease (46, 47). This discovery has also promoted the development of immunologically based animal models.
In light of inflammatory and immunological theory, Ezura et al. (48) induced a rabbit model by implementing a serum sickness vasculitis model combined with intracisternal administration of antibodies or antigens. The experiment included four groups: group I received twice intravenous injections of heterologous serum; group II received intracisternal administration of antibodies or antigens combined with a second injection of serum; group III was treated with a single intravenous injection of antigens simultaneously with intracisternal administration of antibodies; and group IV was set as control. Interestingly, any rabbit exposed to intracisternal heterologous serum exhibited transient features of cerebral arteritis with significant periarterial inflammatory cell infiltrate, while rabbits that were exposed to only intravenous heterologous serum did not develop any features of cerebral arteritis. Rao et al. (49) developed a similar rabbit model by chronic inoculation of horse serum for up to 1 year. The experiment included 21 Japanese rabbits in total. Horse serum was injected intravenously in one group, and the other group was injected locally near the sympathetic ganglia.
Histological changes involved intima stenosis of the lumen or even occlusion and disconnections of the inner layer. Moreover, hyperplasic smooth muscle cells extended inward through broken portions of the internal elastic lamina, and the proliferative vascular structure was also positive for IgG and IgM. Although the rabbit model appears to be a promising approach to recapitulate MMD, the requirement for long-term maintenance limits its ability to become widely adopted.
For a cat, the carotid arterial system has extracranial and intracranial two components (50). The internal maxillary artery is the main supply vessel for the external part. The relatively large-sized intracranial ICA mainly received the blood supply from large-caliber anastomotic arteries, the cavernous sinus, and the primitive ICA. The circle of Willis differs from humans, and the anterior communicating artery was absent in cats. In the research, Kamijyo et al. described modifications in the filling pattern (50). Following unilateral short-term occlusion of the MCA with a clip, the main striate branches showed a good filling by contrast medium, as well as the circumferential branches over the hemispheric surfaces appeared. It was not designed for MMD, and no studies used the surgical method to induce the feline model.
Kamata et al. (51) attempted to build a feline model uniting an immune reaction and an arterial occlusion technique. Lactic acid–glycolic acid copolymer (LGA-50) is an immune-embolic material, and it is easy to be shaped. N-acetylmuramyl-L-alanyl-D-isoglutamine (MDP) is the main component of the bacterial cell wall, and it has the effect of inducing various biological effects or evoking an immune response (52, 53). Rod-shaped LGA-50 and MDP were injected into the unilateral CCA. Histologically, mild intimal thickening and duplication of the internal elastic lamina were observed, but these changes were minimal. Interestingly, the intimal changes of ICAs and their branches were found bilaterally, despite an injection of the immuno-embolic agent unilaterally. As more histological changes were presented in the prolonged experimental cats, the extent of the histological changes may relate to the duration and amount of immuno-embolic material administration. Moreover, the stenotic changes and development of moyamoya vessels were not expounded in the angiography of the feline model, despite a subset of the histological characteristics being replicated in the feline model.
As cerebrovascular system angiography showed, the terminal portion of the ICA and its branches of monkeys have the same morphology as the human carotid system. Terai et al. (54) also designed a new MDP method in the experimental research of monkeys. The MMD model was induced by intravascular interventional technique. Five monkeys received a repeated injection of the embolic materials in the right ICAs for all three groups. In the intravenous group, the monkeys underwent an intravenous repeated injection of MDP via a superficial vein of the forearm. In the control group, angiography was performed without any treatment. Reduplication of arteries and lamination of the internal elastic laminae were found both in embolic and intravenous groups. In the embolic group, these histological changes occurred bilaterally, and these were also observed both in the intracranial and extracranial arteries. However, neither stenosis of ICAs nor moyamoya vessels was observed.
A dog is a major model species for cerebrovascular transformation research and shares similar anatomical features of brain vascular with humans (55). Suzuki et al. (56) divided 21 dogs into two groups and conducted an experiment that lasted for 1 month. The cat serum was injected intravenously in the first group, and the second group received a silicon capsule near the cervical sympathetic ganglion. Secretion of cat serum could stimulate the ganglion continuously until 1 mL of serum was consumed. Histological results showed that ICA changes correspond to human pathologic changes. The intimal thickening and internal elastic lamina duplication were observed in both groups, and immune complexes were observed in immunohistochemical staining of the intima and internal elastic lamina. Kasai et al. (57) carried out an experiment to demonstrate an immunological etiology of MMD in mongrel dogs. Their model induced serum sickness via injection of horse or cat serum. This operation would generate circulating immune complexes that could deposit within intracranial vasculature. Although histopathology failed to demonstrate the presence of immune complexes within these vessels, pathological arterial changes were identified in the terminal portion of the ICA. However, these changes are small enough to cause arterial stenosis. While this study asserted a likely role for inflammation in MMD, failure to identify intracranial immune complexes limited its pathogenic implications.
The miniature pigs have similar brain structures to humans; they have been used in ischemic models associated with human stroke (58). For the cerebrovascular of miniature pig, it is easy to identify ICAs, ACAs, MCAs, PCAs, and anterior and posterior communicating arteries (59). Compared to the human vascular system, the MCA of miniature pig originates from the ICA in the cerebral hemisphere, one coursing laterally and another rostrally over the olfactory tract. The diameter remained constant with each other between the posterior communicating artery and ICA. It is suggested that the anterior circulation communicates well with the posterior circulation.
Nakamura et al. (37) set out a miniature pig model by internal carotid artery occlusion (ICAO). Fourteen pigs were used to investigate the chronological angiogenic changes and identify revascularization mechanisms. The results showed that a suitable environment and stimulus were required to induce angiogenesis in functional revascularization, for example, active vascular bed and ischemia. Yet, the animal model did not explicitly evaluate the cerebrovasculature, although the ICAO model of miniature pig was sufficient to discuss EMS-related revascularization.
More pieces of evidence suggest that MMD is probably a kind of hyperplastic vasculomyopathy (60), mainly an endometrial hyperplasia disease. From a cellular physiological standpoint, histopathological analysis indicates proliferation of both endothelial cells (ECs) and smooth muscle cells in the distal ICA (61–64). The first is the exploration of endothelial progenitor cells (EPCs). In 1997, EPCs were discovered in human peripheral blood for the first time (65). There is a general belief that EPCs originated from mesoderm cells during embryonic development (66); bone-marrow-derived progenitor cells could also differentiate into ECs lines (67). It is revealed that the abnormal number and function of endothelial progenitor cells (EPCs) were largely associated with the progression of MMD. Differentiation into ECs and remodeling of vascular ECM components are the ways for EPCs to play their functions after they pass through the endodermis (68, 69). For the number of EPCs, it was first found that the number of CD34+ cells in peripheral blood of MMD increased significantly in 2008 (70), and similar results were observed in other studies (71–73). However, the number of EPCs in the peripheral blood of MMD was often considered “controversial” because of two studies. One study showed a higher level of the EPCs using flow cytometry (71), while the other study revealed a lower level of EPCs employing colony-forming assays (74). First, MMD-specific induced pluripotent stem cells (iPSCs) carrying RNF213 R4810K were established by Hitomi et al. (75). The iPSCs were differentiated into ECs, and reduced angiogenic activities were detected for these ECs. It is suggested that the iPSC-derived vascular endothelial cells (iPSECs) is a promising in vitro model for MMD research. Kim et al. (76) also reported impaired angiogenic activity of EPCs in MMD, indicating that the phenotype of EPCs could be a disease-specific in vitro model. Furthermore, a study revealed disrupted mitochondrial morphology in the endothelial colony-forming cells (ECFCs) from the MMD, and the mitochondria seem to have shorter and more circular shapes and abnormal functions (77). In addition to the in vitro model described above, the only EPCs relevant in vivo experiment was conducted on rats (25). EPCs were obtained from MMD and were injected into the chronic cerebral hypoperfusion model. Nevertheless, less improvement in the cerebral perfusion, behavior, and amount of neovasculogenesis was found. Collectively, EPCs may be a potential target for in vitro model development.
The second referred to the crucial factors of smooth muscle progenitor cells (SMPCs) and smooth muscle cells (SMCs). The main histopathological finding in MMD was fibrocellular thickening of the intima, and the occlusion was essentially resulting from overproliferation of SMCs (78). Guo et al. (79) suggested that occlusive lesions and stenotic arteries of familial patients may be caused by smooth muscle tissue proliferation related to actin alpha 2 (ACTA2) mutations. The iPSC method was particularly conducive for diseases that the development of pathology has not elucidated. It is possible to explain the pathological mechanism after the establishment of the in vitro model of MMD. Kang et al. (80) purified SPCs from the peripheral blood of patients and demonstrated the development of irregularly arranged and thickened tubes in cell culture. The RNA was extracted from the cells, and differentially expressed genes (DEGs) were identified. These cells displayed specific DEGs compared with the control group. Recently, Tokairin et al. (81) characterized the VSMCs in MMD first in terms of the biological function and transcriptome profile. Both MMD and the control group displayed similar features of VSMCs, while ECs displayed distinct transcriptome profiles; the same EC features had also been validated previously (82). Therefore, SMCs may be another potential target for in vitro model development.
In addition, there was a close relationship between the EPC and SMC (83, 84). Overexpression of related factors in SMCs can help increase EPC adhesion and differentiation, migration, and proliferation (85, 86). However, according to the data in MMD research, the pathological changes are driven mainly by ECs, and VSMCs and specific environmental factors may play an important role (81). In 2017, C–C motif chemokine ligand 5 (CCL5) secreted by ECFCs was found to significantly augment the migration activities of SMCs toward ECFCs (87). Thus, EPCs contribute to neovascularization by paracrine effects. All in all, it is believed that these studies provide novel insight and cellular model for further research of MMD.
The complicated pathological features of narrow ICAs and the unknown nature of neovascularization indicate that the pathophysiology of MMD is a complex procedure. Advances made in defining pathophysiological aspects of MMD have set the foundation for the development of animal models to recapitulate the etiological mechanisms. As described previously, although a number of groups have attempted to induce MMD in zebrafish, mice or rat, rabbit, feline, canine, monkey, and miniature pig models, none were successful in replicating the full phenotype of MMD. Mice and rats were identified as the most frequently used animal models. The zebrafish model is a relatively new experimental model associated with gene mutations, given their unique external fertilization permitting direct observation of embryogenesis and their comparative ease of maintenance. Rabbit, cat, dog, and monkey models were mainly developed in the early stage of the MMD research in the last century. In the vascular anatomy aspect, the intracranial artery systems of all of the animal models are different from that of the humans except for the monkey model. An experimental model closer to the human cerebrovascular system may be further required in an investigation. As a primate, monkeys have a vascular system similar to humans. However, experimental models of primates are limited by cost, availability, and ethical considerations (88). Conversely, miniature pigs have advantages in these aspects compared with primates. Similar to the chronic ischemia of the murine models in mice or rats, the miniature pig models were mainly reported to understand the potential surgical treatment modalities for MMD at present. The preparation and procedures are relatively easier than normal pigs. In the review, three general approaches were mainly identified to develop various animal models, namely, genetic, immunological/inflammatory, and surgical approaches (78, 89) (Figure 3A).
Figure 3. (A) Three approaches for generating moyamoya disease (MMD) animal models. (B) Possible two-hit theory underlying the development of MMD.
From a genetic standpoint, genetic models were generally generated by various knockout, knockdown, or knock-in methods to study the impact of susceptibility genes on vasculogenesis, angiogenesis, and remodeling (9, 12, 42, 43, 90). A majority of genes have been described in the literature (91), such as the RNF213 gene on chromosome 17q25, ACTA2 gene on chromosome 10q23, GUCY1A3 gene on chromosome 4q32, chromosome 3p, and 8q23, and so on. RNF213 polymorphism R4810K is the strongest susceptibility gene for MMD and exists in 0.5%–2.0% of the east Asian population (5, 9). It is reported that the R4810K variant is associated with ischemic MMD, whereas non-R4810K variants are related to hemorrhagic MMD, especially A4399T (92). Notably, gene knockout and/or knock-in model of the susceptibility gene construct did not sufficiently develop MMD, indicating that additional environmental factors may play a critical role; “two-hit theory” was considered for the development of MMD (93). A study revealed that there were no obvious abnormalities both in imaging and histopathology of the vascular in RNF213−/− mice. However, significantly thinner of the intima and medial layers were observed in RNF213−/− 14 days after CCA ligation, no significant difference was observed on 7, 21, and 28 days after CCA ligation (11).
At the time of onset, “first hit” may be characterized by RNF213 deficiency. Next, the “second hit” appeared in the form of autoimmune response, infection or inflammation, radiation, mitochondrial dysfunction, oxidative stress, hemodynamic disorder, and so on. As the RNF213 deficiency vascular wall is more vulnerable to the “second hit,” progressive stenosis of ICAs and abnormality of the cerebral vascular network can develop and ultimately lead to MMD. In the future, the “multiple-hit model” appears to be widely accepted for recapitulating the complexity of MMD with further research, involving the interaction of genetic and environmental factors, crosstalk between different tissues and cells, and the influence of angiogenic factors and pro-inflammatory molecules (Figure 3B).
From an immunological standpoint, immunological models attempt to reproduce the morphological changes observed in the intracranial vasculature of patients with MMD (16, 51). The frequency of 1.7–4.7% in adults and 0.54–1.5% in infants with an inflammatory disease concurrent with MMD (94). A high prevalence of autoimmune disorders and elevated levels of autoimmune antibodies in blood revealed that the MMD could have some relationship with autoimmune diseases. The immune response caused by autoimmune thyroid disorders (95), diabetes syndrome (96), or systemic lupus erythematosus (SLE) (97) may participate in the pathological process of MMD. Kim et al. (98) reported the elevated levels of thyroid autoantibodies in MMD patients, indicating that immune disorders are involved in the progression of MMD. Furthermore, anti-inflammatory cytokines and proinflammatory cytokines are two major pathways in the inflammatory response for the development of MMD. It is revealed that the proinflammatory cytokine pathway is activating RNF213 functions distinct from the anti-inflammatory cytokine pathway (99). In addition, researchers have identified chronic inflammation aroused by immune responses as a major part of the MMD pathological process (100). However, the molecular mechanisms of the inflammatory/immune response in MMD still have not been fully clarified.
From a surgical standpoint, surgical models utilize mechanical occlusion or narrowing of extracranial and/or intracranial vasculature to replicate stenosis and accompanying cerebral ischemia (22, 28). Direct, indirect, and combined surgical revascularization is still the first choice for patients. A number of techniques have been developed to recreate chronic cerebral hypoperfusion animal models through occlusion of the CCAs and ICAs (101–104). Artery occlusion remains the most common method of simulating the ischemic microenvironment of moyamoya in mice, rats, and pigs. The clinical treatment modalities explore with the assistance of these ischemic models. The effect of indirect revascularization, especially EMS, was evaluated in detail by two-vessel occlusion (17, 18, 20, 21, 24, 28, 29), single-vessel occlusion (32, 35, 36), and microcoil (105) methods of ischemic models. Angiogenetic microRNA, gene therapy, myoblast implantation, and bone marrow stromal cells are confirmed to have the potential as a new therapeutic strategy in the chronically hypoperfused brain. Moreover, the underlying mechanism of burr hole surgery was also evaluated by two-vessel occlusion (27) and tMCAO (106, 107) methods of ischemic models. This treatment can provide feasible treatment options for MMD patients. Despite the benefits of surgical models, several limitations exist, such as a sudden reduction in challenges to achieving satisfactory reproducibility.
While these models reproduce the disparate aspects of MMD independently, no model exists that satisfactorily combines genetic predisposition, immunologically mediated vasculopathy, and chronic ischemia. Recently, EPCs and SPCs have attracted much attention in the study of the pathogenesis of MMD because of the difficulty in obtaining specimens and a lack of animal models (108). MMD is a unique disease that involves the proliferation, migration, differentiation of different vascular cells, and the maintenance of the vascular wall. Various types of cells, but not limited EPCs and SPCs, may play a complex role in the MMD process. Different cell types may be recruited during the aberrant angiogenesis process, such as SMCs (80), SMPCs (70), circulating ECs (109), and immune cells (45), eventually leading to stenosis and abnormal collateral formation. Besides, RNF213 may serve as a downstream medium of the IFN-β signaling pathway and function as a common responder of the PI3 kinase-AKT signaling pathway in ECs (7, 110). At last, the existing controversies about the EPCs in different studies were mainly caused by different classification methods and isolation/cultivation strategies. Thus, the inconsistent results lack comparability. All in all, additional research in the cellular model would complement other animal models and greatly enhance the limited understanding of the pathology underlying MMD.
To date, a variety of effective experimental models have been trailed by different animal species and approaches in an attempt to understand both the disease progression and potential treatment modalities for MMD. Animal models described in each study categorize as genetic mechanisms, immune-mediated arterial injury, and mechanical occlusion or stenosis within the cerebral vasculature based on the methods used to recapitulate MMD pathogenesis. Each animal model has its unique advantages and disadvantages. Unfortunately, none were successful in replicating the full phenotype of MMD. Mice and rat models are the most common animal models in MMD research. In the future, cellular models may provide a new perspective for understanding the etiology and pathology of MMD.
Conception and design: LC, BY, and HL. Acquisition and analysis of data: LC, YD, and KS. Table and figure: DL and HW. Drafting the article: LC. Study supervision: BY and HL. Critically revising the article: all authors. Reviewed submitted version of manuscript: all authors. Approved the final version of the manuscript on behalf of all authors: LC. All authors contributed to the article and approved the submitted version.
The Supplementary Material for this article can be found online at: https://www.frontiersin.org/articles/10.3389/fsurg.2022.929871/full#supplementary-material.
The authors declare that the research was conducted in the absence of any commercial or financial relationships that could be construed as a potential conflict of interest.
All claims expressed in this article are solely those of the authors and do not necessarily represent those of their affiliated organizations, or those of the publisher, the editors and the reviewers. Any product that may be evaluated in this article, or claim that may be made by its manufacturer, is not guaranteed or endorsed by the publisher.
1. Suzuki J, Takaku A. Cerebrovascular “moyamoya” disease. Disease showing abnormal net-like vessels in base of brain. Arch Neurol. (1969) 20(3):288–99. doi: 10.1001/archneur.1969.00480090076012
2. Goto Y, Yonekawa Y. Worldwide distribution of moyamoya disease. Neurol Med Chir (Tokyo). (1992) 32(12):883–6. doi: 10.2176/nmc.32.883
3. Bang OY, Fujimura M, Kim SK. The pathophysiology of moyamoya disease: an update. J Stroke. (2016) 18(1):12–20. doi: 10.5853/jos.2015.01760
4. Gupta A, Tyagi A, Romo M, Amoroso KC, Sonia F. Moyamoya disease: a review of current literature. Cureus. (2020) 12(8):e10141.33014640
5. Kamada F, Aoki Y, Narisawa A, Abe Y, Komatsuzaki S, Kikuchi A, et al. A genome-wide association study identifies RNF213 as the first Moyamoya disease gene. J Hum Genet. (2011) 56(1):34–40. doi: 10.1038/jhg.2010.132
6. Kobayashi H, Kabata R, Kinoshita H, Morimoto T, Ono K, Takeda M, et al. Rare variants in RNF213, a susceptibility gene for moyamoya disease, are found in patients with pulmonary hypertension and aggravate hypoxia-induced pulmonary hypertension in mice. Pulm Circ. (2018) 8(3):2045894018778155. doi: 10.1177/2045894018778155
7. Bang OY, Chung JW, Kim DH, Won HH, Yeon JY, Ki CS, et al. Moyamoya disease and spectrums of RNF213 vasculopathy. Transl Stroke Res. (2020) 11(4):580–589. doi: 10.1007/s12975-019-00743-6
8. Lieschke GJ, Currie PD. Animal models of human disease: zebrafish swim into view. Nat Rev Genet. (2007) 8(5):353–67. doi: 10.1038/nrg2091
9. Liu W, Morito D, Takashima S, Mineharu Y, Kobayashi H, Hitomi T, et al. Identification of RNF213 as a susceptibility gene for moyamoya disease and its possible role in vascular development. PLoS One. (2011) 6(7):e22542. doi: 10.1371/journal.pone.0022542
10. Wen J, Sun X, Chen H, Liu H, Lai R, Li J, et al. Mutation of rnf213a by TALEN causes abnormal angiogenesis and circulation defects in zebrafish. Brain Res. (2016) 1644:70–8. doi: 10.1016/j.brainres.2016.04.051
11. Sonobe S, Fujimura M, Niizuma K, Nishijima Y, Ito A, Shimizu H, et al. Temporal profile of the vascular anatomy evaluated by 9.4-T magnetic resonance angiography and histopathological analysis in mice lacking RNF213: a susceptibility gene for moyamoya disease. Brain Res. (2014) 1552:64–71. doi: 10.1016/j.brainres.2014.01.011
12. Kanoke A, Fujimura M, Niizuma K, Ito A, Sakata H, Sato-Maeda M, et al. Temporal profile of the vascular anatomy evaluated by 9.4-tesla magnetic resonance angiography and histological analysis in mice with the R4859K mutation of RNF213, the susceptibility gene for moyamoya disease. Brain Res. (2015) 1624:497–505. doi: 10.1016/j.brainres.2015.07.039
13. Starosolski Z, Villamizar CA, Rendon D, Paldino MJ, Milewicz DM, Ghaghada KB, et al. Ultra high-resolution in vivo computed tomography imaging of mouse cerebrovasculature using a long circulating blood pool contrast agent. Sci Rep. (2015) 5:10178. doi: 10.1038/srep10178
14. Ren X, Yao LL, Pan JX, Zhang JS, Mei L, Wang YG, et al. Linking cortical astrocytic neogenin deficiency to the development of Moyamoya disease-like vasculopathy. Neurobiol Dis. (2021) 154:105339. doi: 10.1016/j.nbd.2021.105339
15. Suzuki J, Yonemitsu T, Ezura M, Fujiwara S. Experimental study on etiology of moyamoya disease: effects of bacterial cell wall on cerebral artery. Annual report (1986) of the research commmittee on spontaneous occlusion of the circle of willis (moyamoya disease) of the ministry of health and welfare, Japan. Japan: Ministry of Health and Welfare (1987).
16. Yamada H, Deguchi K, Tanigawara T, Takenaka K, Nishimura Y, Shinoda J, et al. The relationship between moyamoya disease and bacterial infection. Clin Neurol Neurosurg. (1997) 99(Suppl 2):S221–4. doi: 10.1016/S0303-8467(97)00048-6
17. Kusaka N, Sugiu K, Tokunaga K, Katsumata A, Nishida A, Namba K, et al. Enhanced brain angiogenesis in chronic cerebral hypoperfusion after administration of plasmid human vascular endothelial growth factor in combination with indirect vasoreconstructive surgery. J Neurosurg. (2005) 103(5):882–90. doi: 10.3171/jns.2005.103.5.0882
18. Matsuda T, Abe T, Wu JL, Fujiki M, Kobayashi H. Hypoxia-inducible factor-1alpha DNA induced angiogenesis in a rat cerebral ischemia model. Neurol Res. (2005) 27(5):503–8. doi: 10.1179/016164105X25144
19. Ohtaki H, Fujimoto T, Sato T, Kishimoto K, Fujimoto M, Moriya M, et al. Progressive expression of vascular endothelial growth factor (VEGF) and angiogenesis after chronic ischemic hypoperfusion in rat. Acta Neurochir Suppl. (2006) 96:283–7. doi: 10.1007/3-211-30714-1_61
20. Kim HS, Lee HJ, Yeu IS, Yi JS, Yang JH, Lee IW. The neovascularization effect of bone marrow stromal cells in temporal muscle after encephalomyosynangiosis in chronic cerebral ischemic rats. J Korean Neurosurg Soc. (2008) 44(4):249–55. doi: 10.3340/jkns.2008.44.4.249
21. Ohmori Y, Morioka M, Kaku Y, Kawano T, Kuratsu J. Granulocyte colony-stimulating factor enhances the angiogenetic effect of indirect bypass surgery for chronic cerebral hypoperfusion in a rat model. Neurosurgery. (2011) 68(5):1372–9. discussion 1379. doi: 10.1227/NEU.0b013e31820c0289
22. Gong H, Shu L, Xu H, Chen B, Mao R, Zhang F, et al. Bilateral internal carotid arteries ligation temporary impairs brain vasculaturev in young rats. Auton Neurosci. (2013) 173(1-2):39–44. doi: 10.1016/j.autneu.2012.11.003
23. Su SH, Wu YF, Lin Q, Yu F, Hai J. Cannabinoid receptor agonist WIN55,212-2 and fatty acid amide hydrolase inhibitor URB597 suppress chronic cerebral hypoperfusion-induced neuronal apoptosis by inhibiting c-Jun N-terminal kinase signaling. Neuroscience. (2015) 301:563–75. doi: 10.1016/j.neuroscience.2015.03.021
24. Hiramatsu M, Hishikawa T, Tokunaga K, Kidoya H, Nishihiro S, Haruma J, et al. Combined gene therapy with vascular endothelial growth factor plus apelin in a chronic cerebral hypoperfusion model in rats. J Neurosurg. (2017) 127(3):679–86. doi: 10.3171/2016.8.JNS16366
25. Choi SA, Chong S, Kwak PA, Moon YJ, Jangra A, Phi JH, et al. Impaired functional recovery of endothelial colony-forming cells from moyamoya disease in a chronic cerebral hypoperfusion rat model. J Neurosurg Pediatr. (2018) 23(2):204–13. doi: 10.3171/2018.8.PEDS1883
26. Mansour A, Niizuma K, Rashad S, Sumiyoshi A, Ryoke R, Endo H, et al. A refined model of chronic cerebral hypoperfusion resulting in cognitive impairment and a low mortality rate in rats. J Neurosurg. (2018) 131(3):892–902. doi: 10.3171/2018.3.JNS172274
27. Park GH, Shin HS, Choi ES, Yoon BS, Choi MH, Lee SJ, et al. Cranial burr hole with erythropoietin administration induces reverse arteriogenesis from the enriched extracranium. Neurobiol Dis. (2019) 132:104538. doi: 10.1016/j.nbd.2019.104538
28. Chen C, Ling C, Gong J, Li C, Zhang L, Gao S, et al. Increasing the expression of microRNA-126-5p in the temporal muscle can promote angiogenesis in the chronically ischemic brains of rats subjected to two-vessel occlusion plus encephalo-myo-synangiosis. Aging (Albany NY). (2020) 12(13):13234–54. doi: 10.18632/aging.103431
29. Li W, Wei L, Wang B, Gao S, Huang T, Li Z, et al. The trend of indirect anastomosis formation in a 2-vessel occlusion plus encephalo-myo-synangiosis rat model. Ann Transl Med. (2021) 9(1):19. doi: 10.21037/atm-20-2936
30. Wang DP, Lin Q, Kang K, Wu YF, Su SH, Hai J. Preservation of spatial memory and neuroprotection by the fatty acid amide hydrolase inhibitor URB597 in a rat model of vascular dementia. Ann Transl Med. (2021) 9(3):228. doi: 10.21037/atm-20-4431
31. Rafael H. Chronic brain ischemia and revascularization. J Neurosurg. (2006) 105(2):339–40. author reply 340-1. doi: 10.3171/jns.2006.105.2.339
32. Nishihiro S, Hishikawa T, Hiramatsu M, Kidani N, Takahashi Y, Murai S, et al. High-mobility group box-1-induced angiogenesis aAfter indirect bypass surgery in a chronic cerebral hypoperfusion model. Neuromolecular Med. (2019) 21(4):391–400. doi: 10.1007/s12017-019-08541-x
33. Hecht N, Peña Tapia P, Vinci M, von Degenfeld G, Woitzik J, Vajkoczy P. Myoblast-mediated gene therapy via encephalomyosynangiosis—a novel strategy for local delivery of gene products to the brain surface. J Neurosci Methods. (2011) 201(1):61–6. doi: 10.1016/j.jneumeth.2011.07.011
34. Sonobe S, Fujimura M, Niizuma K, Fujimura T, Furudate S, Nishijima Y, et al. Increased vascular MMP-9 in mice lacking RNF213: moyamoya disease susceptibility gene. Neuroreport. (2014) 25(18):1442–6. doi: 10.1097/WNR.0000000000000289
35. Hecht N, et al. Myoblast-mediated gene therapy improves functional collateralization in chronic cerebral hypoperfusion. Stroke. (2015) 46(1):203–11. doi: 10.1161/STROKEAHA.114.006712
36. Marushima A, et al. Balanced single-vector co-delivery of VEGF/PDGF-BB improves functional collateralization in chronic cerebral ischemia. J Cereb Blood Flow Metab. (2020) 40(2):404–19. doi: 10.1177/0271678X18818298
37. Nakamura M, et al. Experimental investigation of encephalomyosynangiosis using gyrencephalic brain of the miniature pig: histopathological evaluation of dynamic reconstruction of vessels for functional anastomosis. Laboratory investigation. J Neurosurg Pediatr. (2009) 3(6):488–95. doi: 10.3171/2008.6.PEDS0834
38. Sato-Maeda M, et al. Transient global cerebral ischemia induces RNF213, a moyamoya disease susceptibility gene, in vulnerable neurons of the rat hippocampus CA1 subregion and ischemic cortex. J Stroke Cerebrovasc Dis. (2017) 26(9):1904–11. doi: 10.1016/j.jstrokecerebrovasdis.2017.06.032
39. Wang J, et al. A new rat model of chronic cerebral hypoperfusion resulting in early-stage vascular cognitive impairment. Front Aging Neurosci. (2020) 12:86. doi: 10.3389/fnagi.2020.00086
40. Shibata M, et al. White matter lesions and glial activation in a novel mouse model of chronic cerebral hypoperfusion. Stroke. (2004) 35(11):2598–603. doi: 10.1161/01.STR.0000143725.19053.60
41. Roberts JM, et al. Internal carotid artery stenosis: a novel surgical model for moyamoya syndrome. PLoS One. (2018) 13(1):e0191312. doi: 10.1371/journal.pone.0191312
42. Ito A, et al. Enhanced post-ischemic angiogenesis in mice lacking RNF213; a susceptibility gene for moyamoya disease. Brain Res. (2015) 1594:310–20. doi: 10.1016/j.brainres.2014.11.014
43. Kanoke A, et al. Temporal profile of magnetic resonance angiography and decreased ratio of regulatory T cells after immunological adjuvant administration to mice lacking RNF213, a susceptibility gene for moyamoya disease. Brain Res. (2016) 1642:1–9. doi: 10.1016/j.brainres.2016.03.009
44. Morimoto T, et al. Dysregulation of RNF213 promotes cerebral hypoperfusion. Sci Rep. (2018) 8(1):3607. doi: 10.1038/s41598-018-22064-8
45. Masuda J, Ogata J, Yutani C. Smooth muscle cell proliferation and localization of macrophages and T cells in the occlusive intracranial major arteries in moyamoya disease. Stroke. (1993) 24(12):1960–7. doi: 10.1161/01.STR.24.12.1960
46. Chen JB, Liu Y, Zhou LX, Sun H, He M, You C. Prevalence of autoimmune disease in moyamoya disease patients in Western Chinese population. J Neurol Sci. (2015) 351(1–2):184–6. doi: 10.1016/j.jns.2015.02.037
47. Chen JB, Liu Y, Zhou LX, Sun H, He M, You C. Increased prevalence of autoimmune disease in patients with unilateral compared with bilateral moyamoya disease. J Neurosurg. (2016) 124(5):1215–20. doi: 10.3171/2015.4.JNS142936
48. Ezura M, Fujiwara S, Nose M, Yoshimoto T, Kyogoku M. Attempts to induce immune-mediated cerebral arterial injury for an experimental model of moyamoya disease. Childs Nerv Syst. (1992) 8(5):263–7. doi: 10.1007/BF00300793
49. Rao M, Zhang H, Liu Q, Zhang S, Hu L, Deng F. Clinical and experimental pathology of Moyamoya disease. Chin Med J (Engl). (2003) 116(12):1845–9. PMID: 14687471
50. Kamijyo Y, Garcia JH. Carotid arterial supply of the feline brain. Applications to the study of regional cerebral ischemia. Stroke. (1975) 6(4):361–9. doi: 10.1161/01.STR.6.4.361
51. Kamata I, Terai Y, Ohmoto T. Attempt to establish an experimental animal model of moyamoya disease using immuno-embolic material—histological changes of the arterial wall resulting from immunological reaction in cats. Acta Med Okayama. (2003) 57(3):143–50. doi: 10.18926/AMO/32831
52. Nagai Y, Akiyama K, Suzuki K, Kotani S, Watanabe Y, Shimono T, et al. Minimum structural requirements for encephalitogen and for adjuvant in the induction of experimental allergic encephalomyelitis. Cell Immunol. (1978) 35(1):158–67. doi: 10.1016/0008-8749(78)90135-1
53. Kotani S, Takada H. Immunopharmacological activities of bacterial cell walls and related synthetic compounds (muramyl peptides). Yakugaku Zasshi. (1983) 103(1):1–27. doi: 10.1248/yakushi1947.103.1_1
54. Terai Y, Kamata I, Ohmoto T. Experimental study of the pathogenesis of moyamoya disease: histological changes in the arterial wall caused by immunological reactions in monkeys. Acta Med Okayama. (2003) 57(5):241–8. doi: 10.18926/AMO/32830
55. Tanaka T, Akiyoshi H, Mie K. Anatomical variations in the circle of Willis in canines. Anat Histol Embryol. (2018) 47(6):609–12. doi: 10.1111/ahe.12390
56. Suzuki J, Kodama N, Mineura K. Etiological studies of cerebrovascular Moyamoya disease. No To Shinkei. (1976) 28(5):459–70.1036065
57. Kasai N, Fujiwara S, Kodama N, Yonemitsu T, Suzuki J. The experimental study on causal genesis of moyamoya disease - correlation with immunological reaction and sympathetic nerve influence for vascular changes (author's transl). No Shinkei Geka. (1982) 10(3):251–61. PMID: 7099368
58. Tanaka Y, Imai H, Konno K, Miyagishima T, Kubota C, Puentes S, et al. Experimental model of lacunar infarction in the gyrencephalic brain of the miniature pig: neurological assessment and histological, immunohistochemical, and physiological evaluation of dynamic corticospinal tract deformation. Stroke. (2008) 39(1):205–12. doi: 10.1161/STROKEAHA.107.489906
59. Imai H, Konno K, Nakamura M, Shimizu T, Kubota C, Seki K, et al. A new model of focal cerebral ischemia in the miniature pig. J Neurosurg. (2006) 104(2 Suppl):123–32. doi: 10.3171/ped.2006.104.2.123
60. Milewicz DM, Kwartler CS, Papke CL, Regalado ES, Cao J, Reid AJ. Genetic variants promoting smooth muscle cell proliferation can result in diffuse and diverse vascular diseases: evidence for a hyperplastic vasculomyopathy. Genet Med. (2010) 12(4):196–203. doi: 10.1097/GIM.0b013e3181cdd687
61. Oka K, Yamashita M, Sadoshima S, Tanaka K. Cerebral haemorrhage in Moyamoya disease at autopsy. Virchows Arch A Pathol Anat Histol. (1981) 392(3):247–61. doi: 10.1007/BF02155663
62. Takagi Y, Kikuta K, Nozaki K, Hashimoto N. Histological features of middle cerebral arteries from patients treated for Moyamoya disease. Neurol Med Chir (Tokyo). (2007) 47(1):1–4. doi: 10.2176/nmc.47.1
63. Chmelova J, Kolar Z, Prochazka V, Curik R, Dvorackova J, Sirucek P, et al. Moyamoya disease is associated with endothelial activity detected by anti-nestin antibody. Biomed Pap Med Fac Univ Palacky Olomouc Czech Repub. (2010) 154(2):159–62. doi: 10.5507/bp.2010.024
64. Kuroda S, Houkin K. Moyamoya disease: current concepts and future perspectives. Lancet Neurol. (2008) 7(11):1056–66. doi: 10.1016/S1474-4422(08)70240-0
65. Asahara T, Murohara T, Sullivan A, Silver M, vander Zee R, Li T, et al. Isolation of putative progenitor endothelial cells for angiogenesis. Science. (1997) 275(5302):964–7. doi: 10.1126/science.275.5302.964
66. De Val S, Black BL. Transcriptional control of endothelial cell development. Dev Cell. (2009) 16(2):180–95. doi: 10.1016/j.devcel.2009.01.014
67. Laurenzana A, Fibbi G, Margheri F, Biagioni A, Luciani C, Del Rosso M, et al. Endothelial progenitor cells in sprouting angiogenesis: proteases pave the way. Curr Mol Med. (2015) 15(7):606–20. doi: 10.2174/1566524015666150831131214
68. Qin G, Ii M, Silver M, Wecker A, Bord E, Ma H, et al. Functional disruption of alpha4 integrin mobilizes bone marrow-derived endothelial progenitors and augments ischemic neovascularization. J Exp Med. (2006) 203(1):153–63. doi: 10.1084/jem.20050459
69. Hynes RO. The extracellular matrix: not just pretty fibrils. Science. (2009) 326(5957):1216–9. doi: 10.1126/science.1176009
70. Yoshihara T, Taguchi A, Matsuyama T, Shimizu Y, Kikuchi Taura A, Soma T, et al. Increase in circulating CD34-positive cells in patients with angiographic evidence of moyamoya-like vessels. J Cereb Blood Flow Metab. (2008) 28(6):1086–9. doi: 10.1038/jcbfm.2008.1
71. Rafat N, Beck GCh, Peña Tapia PG, Schmiedek P, Vajkoczy P. Increased levels of circulating endothelial progenitor cells in patients with Moyamoya disease. Stroke. (2009) 40(2):432–8. doi: 10.1161/STROKEAHA.108.529420
72. Ni G, Liu W, Huang X, Zhu S, Yue X, Chen Z, et al. Increased levels of circulating SDF-1α and CD34+ CXCR4 + cells in patients with moyamoya disease. Eur J Neurol. (2011) 18(11):1304–9. doi: 10.1111/j.1468-1331.2011.03393.x
73. Bao XY, Fan YN, Liu Y, Wang QN, Zhang Y, Zhu B, et al. Circulating endothelial progenitor cells and endothelial cells in moyamoya disease. Brain Behav. (2018) 8(9):e01035.30141248
74. Bao XY, Fan YN, Liu Y, Wang QN, Zhang Y, Zhu B, et al. Circulating endothelial progenitor cells as a pathogenetic marker of moyamoya disease. J Cereb Blood Flow Metab. (2008) 28(11):1795–803. doi: 10.1038/jcbfm.2008.67
75. Hitomi T, Habu T, Kobayashi H, Okuda H, Harada KH, Osafune K, et al. Downregulation of Securin by the variant RNF213 R4810K (rs112735431, G > A) reduces angiogenic activity of induced pluripotent stem cell-derived vascular endothelial cells from moyamoya patients. Biochem Biophys Res Commun. (2013) 438(1):13–9. doi: 10.1016/j.bbrc.2013.07.004
76. Kim JH, Jung JH, Phi JH, Kang HS, Kim JE, Chae JH, et al. Decreased level and defective function of circulating endothelial progenitor cells in children with moyamoya disease. J Neurosci Res. (2010) 88(3):510–8.19774676
77. Choi JW, Son SM, Mook Jung I, Moon YJ, Lee JY, Wang KC, et al. Mitochondrial abnormalities related to the dysfunction of circulating endothelial colony-forming cells in moyamoya disease. J Neurosurg. (2018) 129(5):1151–1159. doi: 10.3171/2017.5.JNS17147
78. Fukui M, Kono S, Sueishi K, Ikezaki K. Moyamoya disease. Neuropathology. (2000) (20 Suppl):S61–4. doi: 10.1046/j.1440-1789.2000.00300.x
79. Guo DC, Papke CL, Tran Fadulu V, Regalado ES, Avidan N, Johnson RJ, et al. Mutations in smooth muscle alpha-actin (ACTA2) cause coronary artery disease, stroke, and Moyamoya disease, along with thoracic aortic disease. Am J Hum Genet. (2009) 84(5):617–27. doi: 10.1016/j.ajhg.2009.04.007
80. Kang HS, Moon YJ, Kim YY, Park WY, Park AK, Wang KC, et al. Smooth-muscle progenitor cells isolated from patients with moyamoya disease: novel experimental cell model. J Neurosurg. (2014) 120(2):415–25. doi: 10.3171/2013.9.JNS131000
81. Tokairin K, Hamauchi S, Ito M, Kazumata K, Sugiyama T, Nakayama N, et al. Vascular smooth muscle cell derived from IPS cell of moyamoya disease - comparative characterization with endothelial cell transcriptome. J Stroke Cerebrovasc Dis. (2020) 29(12):105305. doi: 10.1016/j.jstrokecerebrovasdis.2020.105305
82. Hamauchi S, Shichinohe H, Uchino H, Yamaguchi S, Nakayama N, Kazumata K, et al. Cellular functions and gene and protein expression profiles in endothelial cells derived from moyamoya disease-Specific iPS cells. PLoS One. (2016) 11(9):e0163561. doi: 10.1371/journal.pone.0163561
83. Moonen JR, Krenning G, Brinker MG, Koerts JA, van Luyn MJ, Harmsen MC. Endothelial progenitor cells give rise to pro-angiogenic smooth muscle-like progeny. Cardiovasc Res. (2010) 86(3):506–15. doi: 10.1093/cvr/cvq012
84. Lao KH, Zeng L, Xu Q. Endothelial and smooth muscle cell transformation in atherosclerosis. Curr Opin Lipidol. (2015) 26(5):449–56. doi: 10.1097/MOL.0000000000000219
85. Zhu C, Ying D, Zhou D, Mi J, Zhang W, Chang Q, et al. Expression of TGF-beta1 in smooth muscle cells regulates endothelial progenitor cells migration and differentiation. J Surg Res. (2005) 125(2):151–6. doi: 10.1016/j.jss.2004.12.006
86. Zhu G, Huang L, Song M, Yu Z, Wu X, Zhao X, et al. Over-expression of hepatocyte growth factor in smooth muscle cells regulates endothelial progenitor cells differentiation, migration and proliferation. Int J Cardiol. (2010) 138(1):70–80. doi: 10.1016/j.ijcard.2008.10.042
87. Phi JH, Suzuki N, Moon YJ, Park AK, Wang KC, Lee JY, et al. Chemokine ligand 5 (CCL5) derived from endothelial colony-forming cells (ECFCs) mediates recruitment of smooth muscle progenitor cells (SPCs) toward critical vascular locations in moyamoya disease. PLoS One. (2017) 12(1):e0169714. doi: 10.1371/journal.pone.0169714
88. Huang J, Mocco J, Choudhri TF, Poisik A, Popilskis SJ, Emerson R, et al. A modified transorbital baboon model of reperfused stroke. Stroke. (2000) 31(12):3054–63. doi: 10.1161/01.STR.31.12.3054
89. Rallo MS, Akel O, Gurram A, Sun H. Experimental animal models for moyamoya disease and treatment: a pathogenesis-oriented scoping review. Neurosurg Focus. (2021) 51(3):E5. doi: 10.3171/2021.6.FOCUS21284
90. Kobayashi H, Matsuda Y, Hitomi T, Okuda H, Shioi H, Matsuda T, et al. Biochemical and functional characterization of RNF213 (Mysterin) R4810K, a susceptibility mutation of moyamoya disease, in angiogenesis in vitro and in vivo. J Am Heart Assoc. (2015) 4(7). doi: 10.1161/JAHA.115.002146
92. Wu Z, Jiang H, Zhang L, Xu X, Zhang X, Kang Z, et al. Molecular analysis of RNF213 gene for moyamoya disease in the Chinese Han population. PLoS One. (2012) 7(10):e48179. doi: 10.1371/journal.pone.0048179
93. Fujimura M, Sonobe S, Nishijima Y, Niizuma K, Sakata H, Kure S, et al. Genetics and biomarkers of moyamoya disease: significance of RNF213 as a susceptibility gene. J Stroke. (2014) 16(2):65–72. doi: 10.5853/jos.2014.16.2.65
94. Mejia-Munne JC, Ellis JA, Feldstein NA, Meyers PM, Connolly ES. Moyamoya and Inflammation. World Neurosurg. (2017) 100:575–578. doi: 10.1016/j.wneu.2017.01.012
95. Im SH, Oh CW, Kwon OK, Kim JE, Han DH. Moyamoya disease associated with Graves disease: special considerations regarding clinical significance and management. J Neurosurg. (2005) 102(6):1013–7. doi: 10.3171/jns.2005.102.6.1013
96. Fukuyama Y, Osawa M, Kanai N. Moyamoya disease (syndrome) and the Down syndrome. Brain Dev. (1992) 14(4):254–6. doi: 10.1016/S0387-7604(12)80242-7
97. El Ramahi KM, Al Rayes HM. Systemic lupus erythematosus associated with moyamoya syndrome. Lupus. (2000) 9(8):632–6. doi: 10.1191/096120300678828686
98. Kim SJ, Heo KG, Shin HY, Bang OY, Kim GM, Chung CS, et al. Association of thyroid autoantibodies with moyamoya-type cerebrovascular disease: a prospective study. Stroke. (2010) 41(1):173–6. doi: 10.1161/STROKEAHA.109.562264
99. Mikami T, Suzuki H, Komatsu K, Mikuni N. Influence of inflammatory disease on the pathophysiology of moyamoya disease and quasi-moyamoya disease. Neurol Med Chir (Tokyo). (2019) 59(10):361–70. doi: 10.2176/nmc.ra.2019-0059
100. Sigdel TK, Shoemaker LD, Chen R, Li L, Butte AJ, Sarwal MM, et al. Immune response profiling identifies autoantibodies specific to Moyamoya patients. Orphanet J Rare Dis. (2013) 8:45. doi: 10.1186/1750-1172-8-45
101. Ihara M, Taguchi A, Maki T, Washida K, Tomimoto H. A mouse model of chronic cerebral hypoperfusion characterizing features of vascular cognitive impairment. Methods Mol Biol. (2014) 1135:95–102. doi: 10.1007/978-1-4939-0320-7_8
102. Jing Z, Shi C, Zhu L, Xiang Y, Chen P, Xiong Z, et al. Chronic cerebral hypoperfusion induces vascular plasticity and hemodynamics but also neuronal degeneration and cognitive impairment. J Cereb Blood Flow Metab. (2015) 35(8):1249–59. doi: 10.1038/jcbfm.2015.55
103. Yang Y, Kimura Ohba S, Thompson J, Rosenberg GA. Rodent models of vascular cognitive impairment. Transl Stroke Res. (2016) 7(5):407–14. doi: 10.1007/s12975-016-0486-2
104. Washida K, Hattori Y, Ihara M. Animal models of chronic cerebral hypoperfusion: from mouse to primate. Int J Mol Sci. (2019) 20(24. doi: 10.3390/ijms20246176
105. Hayashi T, Yamamoto S, Hamashima T, Mori H, Sasahara M, Kuroda S. Critical role of platelet-derived growth factor-α in angiogenesis after indirect bypass in a murine moyamoya disease model. J Neurosurg. (2020) 134(5):1535–43. doi: 10.3171/2020.3.JNS193273
106. Sato-Maeda M, Fujimura M, Kanoke A, Morita Fujimura Y, Niizuma K, Tominaga T. Transient middle cerebral artery occlusion in mice induces neuronal expression of RNF213, a susceptibility gene for moyamoya disease. Brain Res. (2016) 1630:50–5. doi: 10.1016/j.brainres.2015.10.055
107. Nam TK, Park SW, Park YS, Kwon JT, Min BK, Hwang SN. Role of a burr hole and calvarial bone marrow-derived stem cells in the ischemic rat brain: a possible mechanism for the efficacy of multiple burr hole surgery in moyamoya disease. J Korean Neurosurg Soc. (2015) 58(3):167–74. doi: 10.3340/jkns.2015.58.3.167
108. Yu J, Du Q, Hu M, Zhang J, Chen J. Endothelial progenitor cells in moyamoya disease: current situation and controversial issues. Cell Transplant. (2020) 29:963689720913259.32193953
109. Park KW, Crouse D, Lee M, Karnik SK, Sorensen LK, Murphy KJ, et al. The axonal attractant Netrin-1 is an angiogenic factor. Proc Natl Acad Sci U S A. (2004) 101(46):16210–5. doi: 10.1073/pnas.0405984101
Keywords: moyamoya disease, animal models, genetic approach, surgical approach, immunological/inflammatory approach, review
Citation: Cao L, Dong Y, Sun K, Li D, Wang H, Li H and Yang B (2022) Experimental Animal Models for Moyamoya Disease: A Species-Oriented Scoping Review. Front. Surg. 9:929871. doi: 10.3389/fsurg.2022.929871
Received: 27 April 2022; Accepted: 31 May 2022;
Published: 1 July 2022.
Edited by:
Jincao Chen, Wuhan University, ChinaReviewed by:
Miki Fujimura, Hokkaido University, JapanCopyright © 2022 Cao, Dong, Sun, Li, Wang, Li and Yang. This is an open-access article distributed under the terms of the Creative Commons Attribution License (CC BY). The use, distribution or reproduction in other forums is permitted, provided the original author(s) and the copyright owner(s) are credited and that the original publication in this journal is cited, in accordance with accepted academic practice. No use, distribution or reproduction is permitted which does not comply with these terms.
*Correspondence: Bo Yang eWFuZ2JvOTZAMTYzLmNvbQ== Hongwei Li SG9uZ3dlaTcwNkAxMjYuY29t
Specialty section: This article was submitted to Neurosurgery, a section of the journal Frontiers in Surgery
Abbreviations: ACA, anterior cerebral artery; CCA, common carotid artery; DEGs, differentially expressed genes; ECFCs, endothelial colony-forming cells; EMS, encephalomyosynangiosis; EPCs, endothelial progenitor cells; ICA, internal carotid artery; ICAS, ICA stenosis; iPSC, induced pluripotent stem cell; LGA-50, lactic acid–glycolic acid copolymer; MCA, middle cerebral artery; MDP, muramyl dipeptide; MMD, moyamoya disease; NCSCs, neural crest stem cells; RNF213, ring finger protein 213; SMPCs, smooth muscle progenitor cells; TGI, transient global ischemia; tMCAO, transient MCA occlusion.
Disclaimer: All claims expressed in this article are solely those of the authors and do not necessarily represent those of their affiliated organizations, or those of the publisher, the editors and the reviewers. Any product that may be evaluated in this article or claim that may be made by its manufacturer is not guaranteed or endorsed by the publisher.
Research integrity at Frontiers
Learn more about the work of our research integrity team to safeguard the quality of each article we publish.