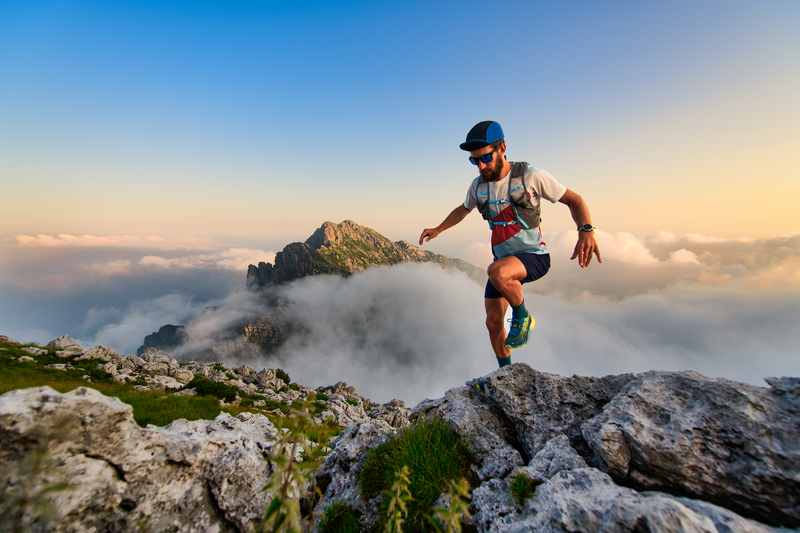
95% of researchers rate our articles as excellent or good
Learn more about the work of our research integrity team to safeguard the quality of each article we publish.
Find out more
MINI REVIEW article
Front. Surg. , 02 August 2022
Sec. Orthopedic Surgery
Volume 9 - 2022 | https://doi.org/10.3389/fsurg.2022.836367
This article is part of the Research Topic Hip Arthroscopy: Pathologies, surgical techniques and complications View all 10 articles
Exosomes are widely involved in a variety of physiological and pathological processes. These important roles are also hidden in the physiological processes related to bone. Chondrocytes, osteoblasts, synovial fibroblasts, and bone marrow mesenchymal stem cells produce and secrete exosomes, thereby affecting the biology process of target cells. Furthermore, in the primary pathogenesis of osteoarthrosis induced by steroid hormones, mainly involve glucocorticoid (GC), the exosomes have also widely participated. Therefore, exosomes may also play an important role in glucocorticoid-induced osteoarthrosis and serve as a promising treatment for early intervention of osteoarthrosis in addition to playing a regulatory role in malignant tumors. This review summarizes the previous results on this direction, systematically combs the role and therapeutic potential of exosomes in GC-induced osteoarthrosis, discusses the potential role of exosomes in the treatment and prevention of GC-induced osteoarthrosis, and reveals the current challenges we confronted.
Glucocorticoids are a class of steroid hormones that play an important role in regulating the body's development, growth, metabolism and immune function, and are also the most widely used and effective anti-inflammatory and immunosuppressive agents in clinical practice. However, long-term use of glucocorticoids can induce osteocyte apoptosis, sustained bone destruction, injury and apoptosis of bone microvascular endothelial cells (BMECs) in the femoral head, and inhibit angiogenesis accompanied by microcirculation disorders (1, 2). Therefore, long-term use of glucocorticoids can lead to Glucocorticoid-induced osteoporosis (GIOP), Osteonecrosis of the femoral head (ONFH) and other osteoarthrosis.
GIOP (Glucocorticoid osteoporosis) is one of the most common and serious adverse reactions associated with glucocorticoid use, as considered to be the most common iatrogenic cause of secondary osteoporosis, leading to early and progressive bone loss, causing osteoarthritis pain and even pathological fractures, with postmenopausal women and men over 50 years of age at high risk (3). GC mainly acts directly on osteoblasts, osteoclasts and osteocytes. GC can reduce the formation of osteoblasts, promote the apoptosis of osteoblasts and osteoblasts, and prolong the life span of osteoclasts, means inhibit bone formation and promote bone resorption. It also reduces vascular endothelial growth factor bone vessels, interstitial fluid, and bone strength (4). At present, the clinical treatment of GIOP mainly includes the combined use of calcium and vitamin D, and the treatment of anti-osteoporosis drugs, including bone resorption inhibitors: bisphosphonates, sex hormone replacement therapy and thyrocalcitonins, when necessary. And bone formation promotors: parathyroid hormone amino-terminal fragment (PTHl-34), fluorine preparations, etc. But long-term use of these drugs can also lead to adverse reactions such as gastrointestinal reactions, osteonecrosis of the jaw, musculoskeletal pain, elevated blood pressure, and kidney stones (3, 5). Therefore, we need new effective drugs to treat GIOP. ONFH (Osteonecrosis of the femoral head) is a disease of mesenchymal cells or bone cells characterized by impaired subchondral microcirculation, bone necrosis, and accumulation of microfractures (6, 7). As a disabling and progressive disease, ONFH is caused by the destruction or interruption of the blood circulation of the femoral head at the initial stage, followed by cell necrosis, which eventually leads to hip joint dysfunction (8, 9). The pathogenic factors of ONFH mainly involve traumatic (such as femoral neck fracture, hip dislocation) and non-traumatic (such as corticosteroids, alcoholism, coagulopathy) risk factors (7, 10–12). As the most common type of ONFH, steroid-induced osteonecrosis of the femoral head (SONFH) accounts for 46.03% of the 15,000–20,000 new ONFH cases in China each year (6, 12). If early intervention is not provided, about 80% of patients will develop femoral head collapse, hip joint dysfunction, and permanent disability (13). The exact mechanism of GC (glucocorticoid)-induced ONFH involves cell death, vascular damage, or insufficient bone repair (14, 15). As we all know, GC directly induces apoptosis and inhibits angiogenesis, so it plays a vital role in destroying bone tissue formation and the occurrence of ONFH (14, 16–23). ONFH is a chronic disease that seriously affects the life quality of patients. ONFH, which usually occurs in young patients, may cause the femoral head to collapse and even require the replacement of all hip joints, accompanied by systemic functional defects and serious defects (24–26). So far, a variety of surgical methods have been used for total hip replacement and autologous cell transplantation. However, no treatment can completely cure the disease (27, 28). Besides, non-surgical treatments for ONFH (such as acetaminophen and cortisone injection) are not sufficient to prevent joint damage, and traditional drugs cannot restore the normal structure and function of the damaged musculoskeletal system (29). Therefore, it is imperative to explore the pathogenesis of ONFH in depth and find a new type of treatment that contribute to delaying the progression of the disease and repairing the damage of the bone marrow microenvironment. Luckily, the discovery of exosomes may have great potential and multiple advantages in the pathogenesis, prevention, and treatment of GC-induced osteoarthrosis (30–33).
Exosomes are naturally derived 50–150 nm nanocapsules that are secreted by cells and commonly found in blood, urine, saliva, cerebrospinal fluid, pleural fluid, and milk. Exosomes play an important paracrine effector role in cell-to-cell and/or cell-to-tissue communication and cross-species communication by transferring proteins and genetic material to target cells (34, 35). Exosomes usually contain various biologically active molecules, such as protein, RNA (mRNA, microRNA, and other non-coding RNA), DNA (mitochondrial DNA [mtDNA], double-stranded DNA [dsDNA], single-stranded DNA, and viral DNA), Lipids, amino acids, and metabolites. These different components play a key role in signal transduction between cells and regulate the microenvironment of nearby or distant cells (36–38). As a new type of biological vesicles, Exosomes have multiple advantages and are considered to be suitable tools for the treatment of various diseases including cancer. First of all, most cells can secrete exosomes and retain the characteristics of parental cells. Secondly, unlike a single protein or small molecule, exosomes contain molecules with heterogeneous functions but lack the complexity of cells and organs. In addition, exosomes show many benefits in terms of biocompatibility, immunogenicity, stability, pharmacokinetics, biodistribution, and cellular uptake mechanisms. Bone-derived exosomes are believed to be essential for intercellular communication between bone cells. The exosome-mediated transfer of nucleic acid or protein cargo between bone cells can bypass the space barriers between different cells and play a vital role in the crosstalk between bone cells that regulate bone homeostasis. Since exosomes are a new biological vesicle that regulates the bone formation, we summarized the characteristics of exosomes, listed the known functions of exosomes in bone homeostasis, and discussed the clinical potential.
In this article, we will mainly through the example of GC-induced GIOP and ONFH introduce the mechanism of exosomes in GC-induced osteoarthrosis and describes the latest achievements in the treatment of GC-induced osteoarthrosis by exosomes. Then, we introduced how exosomes act on GC-induced osteoarthrosis in different aspects. Finally, we discussed the problems that must be solved in the clinical application of these methods and the future research direction of exosomes in the treatment of GC-induced osteoarthrosis.
As a new and potential substance that can be used for early intervention and treatment of GC-induced osteoarthrosis, exosomes have been found to play an important role in the pathogenesis of osteoarthrosis caused by GC. We summarized the roles of exosomes in the three main mechanisms of GC-induced osteoarthrosis (Figure 1).
Figure 1. (A) Exosome-deriving cells and application of exosome to defect sites, achieving promotion of cell proliferation, modulation of immune response, and attenuation of apoptosis. (B) Biology and functions of exosomes in Glucocorticoid-induced Osteonecrosis of the Femoral Head (ONFH). Exosomes can be regarded as vehicles for delivering antigens, proteins and RNA to modulate immune responses, gene expression, and metabolic processes. Exosomes are also involved in the transfer of lipids to recognize TLRs, thus participating in tissue repair.
Studies have shown that GC can directly act on osteoblasts, osteoclasts and osteocytes, reduce the formation of osteoblasts and promote the apoptosis of osteoblasts and osteoblasts. For osteoblasts, activation of glucocorticoid receptors up-regulates the expression level of P53 in mouse osteoblast cell line Mc3t3-e1, thereby enhancing the transcriptional activity of P53 and leading to up-regulation of pro-apoptotic genes P21, PUMA and NOXA. Finally, Mc3t3-e1 cells were induced apoptosis and cell cycle arrest (39). Deng's study found that dexamethasone can down-regulate the expression of P-PI3K and P-Akt to inhibit the activation of PI3K/AKT signaling pathway. The expression of Bax, caspase3, caspase9 and bcl-2 could be decreased and the expression of Bcl-2 could be increased to reduce dexamethasone induced osteoblast apoptosis by removing the expression of GSK3β, the downstream target of PI3K/AKT (40). In addition, GCs’ dose has different effects on bone cells. Low dose GC treatment can lead to autophagy of bone cells, while under high dose GC stress, bone cells may undergo apoptosis or necrosis (41).
Accumulated studies have shown that GC leads to the occurrence and development of ONFH through a variety of mechanisms, and GC-induced bone cell apoptosis is one of the most important ways (42, 43). Under the action of GC, a large number of bone cells undergo apoptosis, leading to loss of bone strength, and disease progression eventually leads to the collapse of the femoral head (8). The research of Hamamura and Saito et al. showed that the increase of bone cell apoptosis is related to endoplasmic reticulum (ER) stress. Specifically, the accumulation of misfolded or unfolded proteins induces phosphorylation of protein kinase-like endoplasmic reticulum kinase (PERK), activates the unfolded protein response (UPR), and helps cells adapt to ER under mild ER stress conditions Stress (44). Among the three main signal pathways of ER stress, the PERK (protein kinase RNA-like ER kinase)/CHOP (CCAATenhancer-binding protein homologous protein) pathway is considered to be closely related to apoptosis. CHOP can inhibit the expression of Bcl-2, increase the level of lytic caspase-3, and cause cell apoptosis.
More and more studies have shown that exosomes play a crucial physiological and pathological role by influencing cell apoptosis, and the same role also occurs in bone physiology (45, 46). For example, exosomes from human umbilical cord mesenchymal stem cells (HUCMSC) can reduce apoptosis of bone marrow mesenchymal stem cells (BMSC) in osteoporotic rats through Mir-1263/Mob1/Hippo signaling pathway (47). In another study, EXOs derived from adipose-derived MSCs (ADSCs-EXOs) prominently reduced H/SD (hypoxia and serum deprivation)-induced apoptosis in the osteocyte-like cell line MLO-Y4 cells by increasing the ratio of Bcl-2/Bax, reducing the production of reactive oxygen species and cytochrome c, and activating caspase-9 and caspase-3 subsequently (48). A research report from S3 shows that exosomes (PRP-EXOS) derived from PRP (Platelet-rich plasma) promote the expression of BCL-2 under ER stress through the AKT/BAD/BCL-2 signaling pathway. The ability to prevent GC-induced apoptosis in ONFH rats (49). PRP-exos significantly enhances the activation of the AKT and ERK signaling cascade, on the one hand, it promotes the angiogenesis of the bone microenvironment; on the other hand, it promotes the expression of anti-apoptotic proteins such as bcl-2 (50). Secondly, the study of S. Zhang et al. observed that exosomes were activated by CD73-mediated AKT and ERK signals to increase the expression of chondrocyte proliferation and anti-apoptotic related genes (51). Guo et al. discovered for the first time that exosomes secreted by human synovial-derived mesenchymal stem cells (SMSC-Exos) can be internalized into bone marrow-derived stromal cells (BMSCs) and enhance their proliferation and anti-apoptotic ability. In in vivo experiments, they found that infusion of SMSC-Exos can reduce GC-induced trabecular bone loss, bone marrow necrosis, and fat cell accumulation. The infusion of SMSC-Exos can effectively prevent GC-induced ONFH in the rat model. At the same time, in vitro cell experiments also found that SMSC-Exos can reverse the anti-proliferative effect induced by GC (52). According to reports, SMMSC-Exos derived from SMMSC significantly reduced glucocorticoid (GC)-induced adipocyte aggregation, bone marrow necrosis, and trabecular bone loss, and to a certain extent reversed bone cell proliferation arrest and BMSC cells apoptosis (52). Micro-CT analysis also showed that SMMSC-Exos significantly improved the trabecular bone microstructure and mineral density of ONFH (ONFH) induced by GC in rats (52).In addition, exosomes produced by MSC can prevent bone cell apoptosis in hypoxia/serum deficiency models and glucocorticoid-induced osteonecrosis models (48, 53) (Figure 2).
The numerous pieces of evidence indicate that exosomes from various sources can inhibit GC-induced apoptosis of osteoblasts and bone cells, and reverse osteonecrosis to a certain extent, which shows the great potential of exosomes in the early treatment of osteoarthrosis caused by GC.
In the pathogenesis of osteoarthrosis caused by GC, GC damage to vascular endothelial cells is another main way. A considerable number of studies have shown that: glucocorticoids can induce injury and apoptosis of bone microvascular endothelial cells (BMECs) of the femoral head, which is closely related to the development of osteonecrosis and osteoporosis, leading to a hypercoagulable state and abnormal microthrombosis in the area of ONFH, and severely reducing the blood supply of trabecular bone (22, 54–58). The work of Greenberger et al. 2010 found that: GC treatment can inhibit the expression of VEGF-a in ECS and subsequent angiogenesis (59). Vascular damage is manifested by decreased function of circulating angiogenic cells, decreased migration function, and VEGF protein secretion (60).
Therefore, inhibition of endothelial cell (EC) apoptosis is necessary to maintain the integrity of blood vessels and prevent the further development of GC-induced osteoarthrosis (61). Similarly, exosomes also play an important role in the physiological and pathological processes related to endothelial cells. The study by Hu GW et al. pointed out that exosomes secreted by mesenchymal stem cells derived from human induced pluripotent stem cells can reduce limb ischemia by promoting angiogenesis in mice (62). Anderson et al. reported that MSC-derived exosomes contained abundant angiogenesis-related proteins that promote endothelial cell proliferation and angiogenesis (63, 64). And the team also detected the promotion of angiogenesis and tissue repair (including bone formation) by exosomes in both in vivo and in vitro experiments (65).In an animal experiment with osteoporotic rats as the experimental object, eight weeks after implantation of MSC-derived exosomes, the experimental group of rats detected the strong formation of blood vessels and bone tissue that was not in the control group (62). In addition, Yokota et al. proved that exosomes can accelerate the surgical angiogenesis of vascular implantation into the necrotic bone by injecting PRP-containing exosomes (66). In addition, activated platelets can also promote the proliferation and migration of bone mesenchymal stem cells (BMSCs) and ECS, thereby promoting bone formation and capillary formation (67). Qi et al and other studies have also shown that in ovariectomized rat models, exosomes secreted by iPS-derived MSCs can promote the regeneration of bone defects by enhancing angiogenesis and bone formation (68). Zuo et al. used miR-26a transfected human CD34 + stem cell-derived exosomes and found that miR-26a-CD34+-exosome enhanced the ability of human umbilical vein endothelial cells to migrate and form blood vessels, indicating that this kind of exosomes can prevent glucocorticoid-induced necrosis of the femoral skull by promoting angiogenesis and osteogenesis (69). These findings provide a novel method for vascular remodeling and bone cell proliferation in soft tissues to enhance early tissue repair.
The pathogenesis of ONFH caused by GC is in addition to inducing osteoblast apoptosis, damage to vascular endothelial cells also involves its inhibition of bone formation (70–73). Previous research reports pointed out that GC has complex stimulating and inhibiting effects on bone metabolism. During normal bone formation, an appropriate amount of endogenous GC signal is necessary. For example, a study by Phillips JE showed that a small dose of dexamethasone (Dex) can promote the differentiation of several osteoblasts in the oval system. However, the use of high-dose GC significantly reduced the patient’s bone mass and lowered bone density, which ultimately greatly promoted the occurrence and development of ONFH (73–75). Similarly, another study also showed that GCs down-regulated the expression of osteogenic marker molecules Runt-related transcription factor 2 (RUNX2) and alkaline phosphatase, and was associated with the decrease of bone density and the rupture of trabecular bone (75). Interestingly, the findings of Ekstrom et al. found that fusion of monocyte-derived exosomes with MSC can trigger the up-regulation of two osteogenic markers: RUNX2 and BMP-2. This phenomenon and two other studies both show that exosomes can interact directly with bone cells, thereby affecting the process of bone formation (76, 77). Of course, the evidence that exosomes play an important role in osteogenesis is not limited to this limited study. As we all know, bone remodeling is a complex process that mainly involves two steps: osteoclastogenesis (used to remove damaged bone tissue) and osteogenesis (used for bone formation). Current research reports have shown that exosomes play an important role in these two steps. Published reports indicate that the transfer of exosome-specific proteins, mRNA, and miRNA is the main mechanism of exosome-mediated bone remodeling. This crosstalk establishes a new network of cell-cell interactions during bone homeostasis (78). For example, the study of Cui, Y, and her colleagues found that mature osteoblast-derived exosomes may trigger the mutation of miRNA expression profile, and then cooperatively inhibit the expression of Axin1, the core component of the Wnt signaling pathway, and finally, β-catenin is up-regulation, leading to enhanced osteogenic differentiation (79). Besides, Let-7-rich exosomes derived from osteoblasts can also enhance osteogenic effects by regulating AT-hook 2 (HMGA2) and AXIN2 (79, 80). The proliferation induced by MSC-derived exosomes has also been reported, and the MAPK pathway may be a key factor in the activity of osteoblasts mediated by exosomes (81).In addition to physiological conditions, exosomes also exhibit important functions related to osteogenesis under pathological conditions. The research results of Furuta et al. showed that during fracture healing, exosomes derived from bone marrow stem cells express MCP-1, MCP-3, SDF-1, angiogenic factors, mRNA, and miRNA, and jointly promote bone Reshape (82).At the same time, exosomes may also increase osteoblast-related proteins (RUNX-2, ALP, OCN, and OPN) and some genes (miRNA-196a, miRNA-27a, and miRNA-206) to enhance the proliferation and differentiation of osteoblasts (83). In addition to the participation of osteoblasts in bone remodeling, osteoclasts also play an important role, and the proper balance between the two is the key to complete thigh remodeling. Exosomes also play an important role in mutual signal communication between osteoblasts and osteoclasts. The inactivation of the RANK-RANKL signaling pathway in osteoblasts can release exosomes containing miR-503-3p, thereby inhibiting the formation of osteoclasts. In animal experiments, in the CD9−/− mouse femoral fracture model (in which the production of exosomes was inhibited), the formation of callus in the experimental group was significantly delayed compared with the control group. However, this delayed effect can be corrected by local injection of exosomes (82).Similar functions of exosomes in promoting fracture repair and bone remodeling have also been verified in a mouse model of osteoporosis (62). This series of research results suggest that exosomes may play an irreplaceable role in the process of bone remodeling and bone repair, and these phenomena may be occurring in the bone repair process of ONFH caused by GC. This hypothesis has also been confirmed by research by Zuo and his colleagues. Their experiments suggested that miR-26a-CD34+-exosome enhanced the osteogenic differentiation of BMSCs under the influence of GC. Finally, miR-26a-CD34+-exos increased the vascular density and small bone density of the femoral head in the GC-induced ONFH mouse model, thereby inhibiting the progression of ONFH and promoting bone repair (69). Shang-Chun Guo et al. also found that SMSC-Exos can improve bone mineral density and trabecular bone microstructure of GC-induced ONFH rats. Immunohistochemical staining for osteocalcin showed that MPS (methylprednisolone) was injected into the thigh The osteogenic response of bones is reduced, but SMSC-EXOS significantly inhibits this effect (52). Another study found that exosomes rich in miR-122-5 down-regulate SPRY2 through the RTK/Ras/mitogen-activated protein kinase (MAPK) signaling pathway, thereby delaying the development of ONFH (84).
In the past few decades, exosomes are involved in many biological processes related to bone metabolism, including angiogenesis, cell differentiation, immune regulation, metabolic balance, and development (36, 85–88). However, exosomes are not simple nucleic acid or protein molecules, but microvesicles containing a variety of substances including RNA, DNA, protein, and lipids. While there are extensive biological functions, exosomes are also highly heterogeneous, involving different sources and different contents. Therefore, since exosomes work through each of the molecules contained, understanding the mechanism of action of each content is crucial for further understanding and application of exosomes. We reviewed the roles played by different contents of exosomes derived from cells related to bone metabolism and the molecular mechanisms of their effects and summarized the possible roles of various substances in GC-induced osteoarthrosis (Table 1).
As one of the most studied contents in exosomes, miRNA released by exosomes has been shown to play an important role in multiple physiological processes of bone metabolism. For example, exosomes derived from myoblasts enter pre-osteogenic cells and promote osteoblast differentiation through miR-27a-3p-mediated β-catenin pathway activation (89). Young MSC exosomes can rejuvenate senescent HSCs through autophagy-related miR-17 and miR-34a cell-to-cell transfer, while miR-23b and miR-92a can effectively treat OA (Osteoarthritis) (90, 91). Furthermore, the exosomes of chondrocytes may promote the chondrogenesis and differentiation of BMSCs by activating the Wnt/β-catenin pathway, which is related to the inhibition of GSK-3β expression by miR-8485 in the exosomes (92).
Besides, recent studies have also highlighted the importance and significance of microRNA (miRNA) in the pathogenesis, prevention, and treatment of GC-induced osteoarthrosis (31, 32). One study showed that exosomal miRNAs promote osteoarthrosis development by influencing osteoblasts, osteoclasts and bone matrix through oxidative stress (OS) mediation. Exogenous antioxidants can help prevent or delay the development of osteoarthrosis, while the antioxidant balance in the body is disrupted (93). But Chen et al. detected the expression of Mir-425-5p in bone marrow mesenchymal stem cells (MSC) by quantitative reverse transcriptase-polymerase chain reaction (qRT-PCR) and the expression of TNF by ELISA, and the results showed that Mir-425-5p could regulate cell apoptosis, proliferation and differentiation induced by TNF. ANXA2 is a target of Mir-425-5p and is involved in TNF-induced apoptosis, proliferation and differentiation of MSC cells. It was concluded that Mir-425-5P could enhance osteoporosis in mice (94). The above studies indicate that the mechanism of miRNA action on osteoporosis still needs further study. The above study indicates that the current research on the mechanism of miRNA action on osteoporosis is limited, and it is necessary to conduct in-depth basic and clinical research.
Wu et al. verified three up-regulated miRNAs (miR-210-3p, miR-320e, and let-7c) by comparing the expression of miRNA in non-traumatic ONFH and femoral neck fractures (95).In previous research evidence, Let-7 in osteoblast-derived exosomes has been shown to enhance osteogenesis by regulating AT-hook 2 (HMGA2) and AXIN2 (79, 80). This indicates that there are still a large number of miRNA that may have a potentially important role in bone repair and bone remodeling in ONFH, waiting to be discovered and explained. ONFH caused by overuse of glucocorticoids accounts for the majority of non-traumatic ONFH. The decrease in the proliferation of mesenchymal stem cells is related to the pathogenesis of glucocorticoid-induced ONFH, and this mutual connection may be involved in the exosomes released by mesenchymal stem cells. Bian et al. compared the expression of miRNA in human mesenchymal stem cells treated with and without dexamethasone. The study found that 11 up-regulated (miR-16-5p, miR-103a-3p, miR-107, miR-196a/b-5p, miR-378d, miR-1268a/b/f/g, miR-4289) and 6 down-regulated (miR-24-3p, miR-378a/h/I, miR-4448, miR-4634) miRNA were found between the two different concentrations of dexamethasone treatment groups. For further analysis, they injected methylprednisolone (21 mg/kg) subcutaneously into C57BL/6J mice and found that miR-21-3p and miR-652-5p were up-regulated and miR-34b-3p, miR-34c-5p, miR-148a-3p, miR-196a-5p, and miR-206-3p are down-regulated, which are predicted to be involved in osteogenic differentiation (96). Hao et al. found that miR-708 may enhance the osteogenic effect of mesenchymal stem cells and inhibit their adipogenic differentiation ability by targeting Smad3 (8). Yamasaki et al. confirmed that miR-210 (angiogenic miRNA) is highly expressed in non-invasive ONFH and may regulate angiogenesis in ONFH (56, 97, 98). Sun et al. confirmed that miR-548d-5p promotes the osteogenic differentiation of mesenchymal stem cells by acting on PPARγ, and may inhibit glucocorticoid-induced ONFH (99). In addition, miR-27 has also been shown to inhibit adipogenesis and enhance bone formation by regulating the expression of GREM1 and PPARγ (83, 100–103). These findings indicate that miRNAs secreted in the bone marrow microenvironment play an irreplaceable role in the pathogenesis of steroid-induced ONFH and the balance between osteogenic differentiation and adipogenic differentiation of mesenchymal stem cells.
As a regulatory RNA, long non-coding RNA (lncRNA) has been shown to play a key role in various cellular physiological functions including cell proliferation, invasion, metabolism, apoptosis, and stem cell differentiation. Recent studies have shown that lncRNA is directly involved in the pathogenesis of many orthopedic diseases and also plays an important role in the process of bone development and regeneration. For example, long non-coding RNA (lncRNA) has been shown to be an important exosomal content in OA, widely involved in the regulation of various pathological and physiological processes (103, 104). Exosomes from adipose-derived stem cells (ADSCs-EXOS) have been verified that play an effective part in the repair of different tissues and organs. ADSCs-EXOS have also been confirmed to help in the treatment of osteoporosis (105). However, Wang et al. believed that compared with ADSC-EXOS, KCNQ1OT1-ExOS, as a kind of lncRNA closely related to cell proliferation, migration and apoptosis, had a more significant inhibitory effect on TNF-α -induced cytotoxicity and apoptosis (106).
Recent research results indicate that lncRNA also plays an important regulatory role in the pathogenesis and repair of ONFH. LncRNA was found to be differentially expressed in ONFH tissues, bone marrow mesenchymal stem cells and bone microvascular endothelial cells which isolated from ONFH patients (9, 107, 108). Functional research has further clarified its important role in the survival of osteoblasts closely related to ONFH and the osteogenic differentiation of bone marrow mesenchymal stem cells. Liu et al. reported that MSC-Exos mainly up-regulated Col2a1 and proteoglycan levels through lncRNA-KLF3-AS1.239 released from exosomes, and down-regulated the expression of MMP13 and Rux2, which promoted the survival of IL-1β-treated chondrocytes (109). According to reports, as a differentially expressed lncRNA isolated from steroid-induced ONFH patients, forced expression of RP11-154D6 can promote the increase in the expression of osteogenic differentiation markers (osteocalcin (OCN) and RUNX2) and reduce the expression of adipogenic differentiation markers (such as lipoprotein lipase (LPL) and peroxisome proliferator-activated receptor gamma (PPAR gamma)), these effects ultimately lead to enhanced bone formation (108). In another study, Wei et al. found that HOTAIR can negatively regulate the proliferation and osteogenic differentiation of mesenchymal stem cells by regulating the expression of miR-17-5p and Smad7, and can be used as a therapeutic target for non-invasive ONFH (110). Wang et al. used the reconstruction of the coding-noncoding gene co-expression (CNC) network to reveal the key role of two lncRNAs (HOTAIR and RP1-193H18.2) in regulating the osteogenic and adipogenic differentiation of bone marrow MSCs (111). In addition, Yu et al. analyzed the BMEC (bone microvascular endothelial cells) of patients who underwent a conventional total hip replacement and exposed the cells to hydrocortisone (0.1 mg/ml) for 24 h using the co-expression analysis technology of non-coding RNA and related mRNA, the results reveal that FoxO transcription factors are closely related to the regulation of angiogenesis (112, 113). Furthermore, the overexpression of MIAT in the bone marrow microenvironment may lead to steroid-related ONFH by inhibiting the osteogenic differentiation of MSC, and this process can be blocked by the epigenetic silencing of MIAT by HXTL (114). Fan and colleagues confirmed that MALAT1 can protect human osteoblasts from dexamethasone-induced cell death. Specifically, MALAT1 prevents steroid-induced ONFH by regulating PPM1E-AMPK-NRF2-oxidative stress and the miR-214-ATF4 axis (32).
Circular RNA (circRNA) is a member of the non-coding RNA family. Unlike linear RNAs such as miRNA or lncRNA, it forms a covalently closed continuous loop, making them resistant to digestion by RNA exonuclease. Accumulated evidence shows that circRNA can perform biological functions by acting as a microRNA sponge, encoding proteins, and binding to proteins (115–121). Research on circRNA is later than most linear RNAs, but in recent studies, circRNA has also been found to be involved in bone metabolism in many diseases (including GC-induced osteoarthrosis).
For instance, Feng et al. found that hsa_circ_0006859 in exosomes of osteoporosis patients can inhibit osteoblast differentiation and promote adipose decomposition of human bone marrow mesenchymal stem cells (hBMSCs). Hsa_circ_0006859 acts, as a competitive endogenous RNA (ceRNA) of Mir-431-5p, directly binds to Mir-431-5p and promotes the expression of ROCK1 which was confirmed as a novel target gene of Mir-431-5p (122).
Generally, the weakened osteogenic differentiation and increased adipogenic differentiation of BMSCs are closely related to the formation of ONFH (102). Xiang et al. have identified 90 up-regulated and 141 down-regulated differentially expressed circRNAs in steroid-induced ONFH (SONFH) BMSCs (123). Further functional studies have found that circRNA immunoglobulin superfamily member 11 can promote osteoblast differentiation in BMSC osteogenesis through glycogen synthase kinase 3β/β-catenin signaling pathway, and knocking down this circRNA can increase miR199b-5p expression (123–125). In addition, some studies have found that circRNA plays a key role in the regulation of bone metabolism mainly by acting as a molecular sponge of miRNA. For example, Kuang et al. proved that in the steroid-induced ONFH rat model, circRNA ubiquitin-specific protease 45 can upregulate phosphatase and tensin homologs by binding to miR-127-5p, thereby inhibiting the protein kinase B pathway and regulate the bone mass of rats (126). In addition, the mode of action of the miRNA-mRNA axis targeted by circ19142/circ5846, circ19142 and circ5846 have been shown to act as sponges for miR-7067-5p in osteoblast differentiation (127). Besides, circRNA FOXP1 has also been shown to play a key role by acting as a sponge for several miRNAs in the regulation of MSC differentiation, which is closely related to the pathogenesis of ONFH (128). Although there are few studies on another important pathogenic mechanism (adipogenic differentiation of mesenchymal stem cells) that affects osteogenesis, The above observation results also show that there is a close correlation between circRNA and SONFH, which can be used for follow-up research and clinical treatment, and provides a good guide for finding therapeutic targets. In another core pathogenic mechanism of ONFH, endothelial cell damage and angiogenesis disorder, circRNA has also been shown to play an important biological role (129). For example, CircRNA0010729 mediates the apoptosis and proliferation of vascular endothelial cells by targeting the miR-186/hypoxia-inducible factor-1α axis (130). Furthermore, circRNA0003575 is up-regulated in human umbilical vein endothelial cells (HUVEC) induced by oxidized low-density lipoprotein and promotes HUVEC proliferation and angiogenesis (131). Although there is no research on circRNA directly targeting endothelial cells in the bone marrow microenvironment, these findings also indicate that circRNA may play an important role in the activation mechanism of ONFH. The above research results indicate that circRNA plays a unique role in the formation of ONFH, and due to its unique stability, may play an irreplaceable role in the treatment of ONFH.
As a kind of exosomal load, many types of specific cell proteins have been shown to contribute to the communication and signal transduction between cells (132–137). In the study of bone-related exosomal proteins, Tsuno et al. used 2D-DIGE and mass spectrometry to identify serum exosomal proteins extracted from the healthy group and the OA group. They found that the exosome between the OA group and the healthy group has 21 spots in the somatic protein profile with different intensities, such as cathepsin F and Igalpha-2 chain C region, indicating the potential role of these proteins in OA (138). At the same time, recent studies have also discovered the role of exosomal proteins in regulating the biological response of chondrocytes. Zhang et al. found the expression of CD73/ecto-5'-nucleotidase in MSC-derived exosomes and found that the CD73 inhibitor AMPCP or the non-selective adenosine receptor antagonist theophylline can reduce MSC Exosomes-induced phosphorylation of AKT and ERK in chondrocytes (139). The results above indicate that the role of the protein-loaded exosomes in the differentiation and development of bone cells still needs further exploration, although the existing evidence has suggested its regulation of cartilage and MSC.
Since the study of exosomal DNA is later than the study of RNA, only a small amount of literature has reported that carrying cytoplasmic DNA in exosomes can prevent cell senescence and cell death caused by DNA damage (140, 141). Moreover, exosomal DNA can exert effects because cells can secrete exosomes and remove harmful DNA in the extracellular matrix. In addition to double-stranded DNA, exosomes also contain single-stranded DNA, but we still know little about the biological role of this DNA. Therefore, it is necessary to study the expression and function of these DNAs in the bone marrow microenvironment.
Exosomes carrying contents like DNA or RNA family serve as crucial vehicles for intercellular communication. Although there is a broad range of potential applications and uses of exosomes, it still appears to be some problems of methods for exosome isolation and analysis. Primarily, the quantities of exosomes released by mammalian cells is relatively low and the purification of exosomes is burdensome. Enhancing the ability to load a variety of cargoes and targeting capabilities without corrupting exosomes is also very important for the utility of this delivery technology. It is hoped that more researchers will participate in the exploration of these problems from bench to bedside in the future. Exosomes show important regulatory effects in different stages and different pathological mechanisms in osteoarthrosis caused by GC, which shows the usefulness and potential of exosomes in the treatment of steroid-related osteoarthrosis. Up to now, take GC-induced ONFH’s treatment for example, it has mainly relied on drug therapy, core decompression, interventional therapy, and cell therapy as early intervention methods, but usually, 65%–85% of patients will continue to develop femoral head collapse (85). Once it develops into the terminal stage of the disease, total hip replacement surgery becomes the only viable option, and this will bring tremendous pressure on the patient's economy and life. Furthermore, for those young patients, ONFH often means that multiple revision surgeries may be required in the future (because the life of the prosthesis is limited), which aggravates the patient's physical and psychological burden. Therefore, as a promising alternative to the traditional treatment of osteoarthrosis, exosomes have many incomparable advantages in the early intervention of osteoarthrosis, and they have received widespread attention as a new treatment for osteoarthrosis (34, 142, 143). Firstly, exosomes have multiple advantages in immunogenicity, and allogeneic exosome injection may not cause obvious complications and rejection in terms of immunogenicity (66). Secondly, exons show good stability and pertinence, because they maintain the properties of their parent cells for a long period and maintain their inherent integrity, which makes them more effective in the treatment of osteoarthrosis. Easily target cells without causing systemic adverse reactions (144–146). Finally, exosomes also show certain advantages in biodistribution and pharmacokinetics. Due to their small size, these nanoparticles can easily reach the wound site. Exosomes can be transformed to express specific surface molecules and can selectively bind to molecules overexpressed on target cells, and exosomes can use their unique functions to extend their half-life (147–152). However, exosomes still face many challenges before entering clinical applications, and the main resistance comes from the separation and purification of exosomes, the modification of exosomes, and the heterogeneity of exosomes. Concerning the role of exosomes in GC-induced osteoarthrosis, research on the underlying mechanism and diagnostic/therapeutic applications have just begun. Although there are still many problems to be solved in this field, we speculate that technological advancement will give an optimistic outlook for the treatment of GC-induced osteoarthrosis based on exosomes.
The original contributions presented in the study are included in the article/Supplementary Material, further inquiries can be directed to the corresponding author/s.
BL wrote the manuscript; ZRC revised the manuscript; YJY and YHC performed the literature search; WKG, SL, and KCZ compiled the graphs; CY and YKZ designed the study. All authors contributed to the article and approved the submitted version.
This study was supported by the grants from the National Natural Science Foundation of China (81974348, 81902260).
The authors declare that the research was conducted in the absence of any commercial or financial relationships that could be construed as a potential conflict of interest.
All claims expressed in this article are solely those of the authors and do not necessarily represent those of their affiliated organizations, or those of the publisher, the editors and the reviewers. Any product that may be evaluated in this article, or claim that may be made by its manufacturer, is not guaranteed or endorsed by the publisher.
1. Li J, Ge Z, Fan L, Wang K. Protective effects of molecular hydrogen on steroid-induced osteonecrosis in rabbits via reducing oxidative stress and apoptosis. BMC Musculoskelet Disord. (2017) 18(1):58. doi: 10.1186/s12891-017-1431-6
2. Nan K, Zhang Y, Zhang X, Li D, Zhao Y, Jing Z, et al. Exosomes from miRNA-378-modified adipose-derived stem cells prevent glucocorticoid-induced osteonecrosis of the femoral head by enhancing angiogenesis and osteogenesis via targeting miR-378 negatively regulated suppressor of fused (Sufu). Stem Cell Res Ther. (2021) 12(1):331. doi: 10.1186/s13287-021-02390-x
3. Laurent MR, Goemaere S, Verroken C, Bergmann P, Body JJ, Bruyère O, et al. Prevention and treatment of glucocorticoid-induced osteoporosis in adults: consensus recommendations from the belgian bone club. Front Endocrinol (Lausanne). (2022) 13:908727. doi: 10.3389/fendo.2022.908727
4. Walsh S, Jordan GR, Jefferiss C, Stewart K, Beresford JN. High concentrations of dexamethasone suppress the proliferation but not the differentiation or further maturation of human osteoblast precursors in vitro: relevance to glucocorticoid-induced osteoporosis. Rheumatology (Oxford). (2001) 40(1):74–83. doi: 10.1093/rheumatology/40.1.74
5. Yang Y, Nian H, Tang X, Wang X, Liu R. Effects of the combined Herba Epimedii and Fructus Ligustri Lucidi on bone turnover and TGF-β1/Smads pathway in GIOP rats. J Ethnopharmacol. (2017) 201:91–9. doi: 10.1016/j.jep.2017.02.033
6. Moya-Angeler J, Gianakos AL, Villa JC, Ni A, Lane JM. Current concepts on osteonecrosis of the femoral head. World J Orthop. (2015) 6(8):590–601. doi: 10.5312/wjo.v6.i8.590
7. Shah KN, Racine J, Jones LC, Aaron RK. Pathophysiology and risk factors for osteonecrosis. Curr Rev Musculoskelet Med. (2015) 8(3):201–9. doi: 10.1007/s12178-015-9277-8
8. Hao C, Yang S, Xu W, Shen JK, Ye S, Liu X, et al. MiR-708 promotes steroid-induced osteonecrosis of femoral head, suppresses osteogenic differentiation by targeting SMAD3. Sci Rep. (2016) 6:22599. doi: 10.1038/srep22599
9. Huang G, Zhao G, Xia J, Wei Y, Chen F, Chen J, et al. FGF2 and FAM201A affect the development of osteonecrosis of the femoral head after femoral neck fracture. Gene. (2018) 652:39–47. doi: 10.1016/j.gene.2018.01.090
10. Cui L, Zhuang Q, Lin J, Jin J, Zhang K, Cao L, et al. Multicentric epidemiologic study on six thousand three hundred and ninety five cases of femoral head osteonecrosis in China. Int Orthop. (2016) 40(2):267–76. doi: 10.1007/s00264-015-3061-7
11. Lespasio MJ, Sodhi N, Mont MA. Osteonecrosis of the hip: a primer. Perm J. (2019) 23. doi: 10.7812/TPP/18-100
12. Papakostidis C, Tosounidis TH, Jones E, Giannoudis PV. The role of “cell therapy” in osteonecrosis of the femoral head. A systematic review of the literature and meta-analysis of 7 studies. Acta Orthop. (2016) 87(1):72–8. doi: 10.3109/17453674.2015.1077418
13. Jilka RL, Weinstein RS, Bellido T, Roberson P, Parfitt AM, Manolagas SC. Increased bone formation by prevention of osteoblast apoptosis with parathyroid hormone. J Clin Invest. (1999) 104(4):439–46. doi: 10.1172/JCI6610
14. Sheng H, Sheng CJ, Cheng XY, Zhang G, Lee KM, Leung KS, et al. Pathomorphological changes of bone marrow adipocytes in process of steroid-associated osteonecrosis. Int J Clin Exp Pathol. (2013) 6(6):1046–50.23696921
15. Balsam LB, Wagers AJ, Christensen JL, Kofidis T, Weissman IL, Robbins RC. Haematopoietic stem cells adopt mature haematopoietic fates in ischaemic myocardium. Nature. (2004) 428(6983):668–73. doi: 10.1038/nature02460
16. Weinstein RS, Jilka RL, Parfitt AM, Manolagas SC. Inhibition of osteoblastogenesis and promotion of apoptosis of osteoblasts and osteocytes by glucocorticoids. Potential mechanisms of their deleterious effects on bone. J Clin Invest. (1998) 102(2):274–82. doi: 10.1172/JCI2799
17. Wang X, Liu Y, Wang X, Liu R, Li J, Zhang G, et al. The role of (99 m)Tc-Annexin V apoptosis scintigraphy in visualizing early stage glucocorticoid-induced femoral head osteonecrosis in the rabbit. Biomed Res Int. (2016) 2016:7067259. doi: 10.1155/2016/7067259
18. Guo S, Mao L, Ji F, Wang S, Xie Y, Fei H, et al. Activating AMP-activated protein kinase by an α1 selective activator compound 13 attenuates dexamethasone-induced osteoblast cell death. Biochem Biophys Res Commun. (2016) 471(4):545–52. doi: 10.1016/j.bbrc.2016.02.036
19. Guo S, Xie Y, Fan JB, Ji F, Wang S, Fei H. α-Melanocyte stimulating hormone attenuates dexamethasone-induced osteoblast damages through activating melanocortin receptor 4-SphK1 signaling. Biochem Biophys Res Commun. (2016) 469(2):281–7. doi: 10.1016/j.bbrc.2015.11.104
20. Zhang C, Zou YL, Ma J, Dang XQ, Wang KZ. Apoptosis associated with Wnt/β-catenin pathway leads to steroid-induced avascular necrosis of femoral head. BMC Musculoskelet Disord. (2015) 16:132. doi: 10.1186/s12891-015-0606-2
21. Ogata K, Katagiri W, Osugi M, Kawai T, Sugimura Y, Hibi H, et al. Evaluation of the therapeutic effects of conditioned media from mesenchymal stem cells in a rat bisphosphonate-related osteonecrosis of the jaw-like model. Bone. (2015) 74:95–105. doi: 10.1016/j.bone.2015.01.011
22. Kerachian MA, Harvey EJ, Cournoyer D, Chow TY, Séguin C. Avascular necrosis of the femoral head: vascular hypotheses. Endothelium. (2006) 13(4):237–44. doi: 10.1080/10623320600904211
23. Hao ZC, Lu J, Wang SZ, Wu H, Zhang YT, Xu SG. Stem cell-derived exosomes: a promising strategy for fracture healing. Cell Prolif. (2017) 50(5):e12359. doi: 10.1111/cpr.12359
24. Qi Y, Zhu Y, Cao Y, Wu H, Sun M, Wu H, et al. Association between MMP-3 polymorphisms among Chinese patients with osteonecrosis of the femoral head. Oncotarget. (2017) 8(65):108859–66. doi: 10.18632/oncotarget.22313
25. Gangji V, Hauzeur JP. Treatment of osteonecrosis of the femoral head with implantation of autologous bone-marrow cells. Surgical technique. J Bone Joint Surg Am. (2005) 87(Suppl 1(Pt 1)):106–12.15743852
26. Mont MA, Etienne G, Ragland PS. Outcome of nonvascularized bone grafting for osteonecrosis of the femoral head. Clin Orthop Relat Res. (2003) (417):84–92. doi: 10.1097/01.blo.0000096826.67494.38
27. Kim SJ, Bahk WJ, Chang CH, Jang JD, Suhl KH. Treatment of osteonecrosis of the femoral head using autologous cultured osteoblasts: a case report. J Med Case Rep. (2008) 2:58. doi: 10.1186/1752-1947-2-58
28. Houdek MT, Wyles CC, Sierra RJ. Osteonecrosis of the femoral head: treatment with ancillary growth factors. Curr Rev Musculoskelet Med. (2015) 8(3):233–9. doi: 10.1007/s12178-015-9281-z
29. Zhang W, Ouyang H, Dass CR, Xu J. Current research on pharmacologic and regenerative therapies for osteoarthritis. Bone Res. (2016) 4:15040. doi: 10.1038/boneres.2015.40
30. Zhao D, Cui D, Wang B, Tian F, Guo L, Yang L, et al. Treatment of early stage osteonecrosis of the femoral head with autologous implantation of bone marrow-derived and cultured mesenchymal stem cells. Bone. (2012) 50(1):325–30. doi: 10.1016/j.bone.2011.11.002
31. Yuan HF, Von Roemeling C, Gao HD, Zhang J, Guo CA, Yan ZQ. Analysis of altered microRNA expression profile in the reparative interface of the femoral head with osteonecrosis. Exp Mol Pathol. (2015) 98(2):158–63. doi: 10.1016/j.yexmp.2015.01.002
32. Wang B, Yu P, Li T, Bian Y, Weng X. MicroRNA expression in bone marrow mesenchymal stem cells from mice with steroid-induced osteonecrosis of the femoral head. Mol Med Rep. (2015) 12(5):7447–54. doi: 10.3892/mmr.2015.4386
33. Cui Y, Guo Y, Kong L, Shi J, Liu P, Li R, et al. A bone-targeted engineered exosome platform delivering siRNA to treat osteoporosis. Bioact Mater. (2022) 10:207–21. doi: 10.1016/j.bioactmat.2021.09.015
34. De Jong OG, Van Balkom BW, Schiffelers RM, Bouten CV, Verhaar MC. Extracellular vesicles: potential roles in regenerative medicine. Front Immunol. (2014) 5:608. doi: 10.3389/fimmu.2014.00608
35. Wiklander OPB, Brennan M, Lötvall J, Breakefield XO, El Andaloussi S. Advances in therapeutic applications of extracellular vesicles. Sci Transl Med. (2019) 11(492):eaav8521. doi: 10.1126/scitranslmed.aav8521
36. Théry C, Zitvogel L, Amigorena S. Exosomes: composition, biogenesis and function. Nat Rev Immunol. (2002) 2(8):569–79. doi: 10.1038/nri855
37. Valadi H, Ekström K, Bossios A, Sjöstrand M, Lee JJ, Lötvall JO. Exosome-mediated transfer of mRNAs and microRNAs is a novel mechanism of genetic exchange between cells. Nat Cell Biol. (2007) 9(6):654–9. doi: 10.1038/ncb1596
38. Skog J, Würdinger T, van Rijn S, Meijer DH, Gainche L, Sena-Esteves M, et al. Glioblastoma microvesicles transport RNA and proteins that promote tumour growth and provide diagnostic biomarkers. Nat Cell Biol. (2008) 10(12):1470–6. doi: 10.1038/ncb1800
39. Li H, Qian W, Weng X, Wu Z, Li H, Zhuang Q, et al. Glucocorticoid receptor and sequential P53 activation by dexamethasone mediates apoptosis and cell cycle arrest of osteoblastic MC3T3-E1 cells. PLoS One. (2012) 7(6):e37030. doi: 10.1371/journal.pone.0037030
40. Deng S, Dai G, Chen S, Nie Z, Zhou J, Fang H, et al. Dexamethasone induces osteoblast apoptosis through ROS-PI3K/AKT/GSK3β signaling pathway. Biomed Pharmacother. (2019) 110:602–8. doi: 10.1016/j.biopha.2018.11.103
41. Yao W, Dai W, Jiang JX, Lane NE. Glucocorticoids and osteocyte autophagy. Bone. (2013) 54(2):279–84. doi: 10.1016/j.bone.2013.01.034
42. Plotkin LI, Manolagas SC, Bellido T. Glucocorticoids induce osteocyte apoptosis by blocking focal adhesion kinase-mediated survival. Evidence for inside-out signaling leading to anoikis. J Biol Chem. (2007) 282(33):24120–30. doi: 10.1074/jbc.M611435200
43. Plotkin LI, Weinstein RS, Parfitt AM, Roberson PK, Manolagas SC, Bellido T. Prevention of osteocyte and osteoblast apoptosis by bisphosphonates and calcitonin. J Clin Invest. (1999) 104(10):1363–74. doi: 10.1172/JCI6800
44. Harding HP, Zhang Y, Zeng H, Novoa I, Lu PD, Calfon M, et al. An integrated stress response regulates amino acid metabolism and resistance to oxidative stress. Mol Cell. (2003) 11(3):619–33. doi: 10.1016/S1097-2765(03)00105-9
45. Mathieu M, Martin-Jaular L, Lavieu G, Théry C. Specificities of secretion and uptake of exosomes and other extracellular vesicles for cell-to-cell communication. Nat Cell Biol. (2019) 21(1):9–17. doi: 10.1038/s41556-018-0250-9
46. Pluchino S, Smith JA. Explicating exosomes: reclassifying the rising stars of intercellular communication. Cell. (2019) 177(2):225–7. doi: 10.1016/j.cell.2019.03.020
47. Yang BC, Kuang MJ, Kang JY, Zhao J, Ma JX, Ma XL. Human umbilical cord mesenchymal stem cell-derived exosomes act via the miR-1263/Mob1/Hippo signaling pathway to prevent apoptosis in disuse osteoporosis. Biochem Biophys Res Commun. (2020) 524(4):883–9. doi: 10.1016/j.bbrc.2020.02.001
48. Ren L, Song ZJ, Cai QW, Chen RX, Zou Y, Fu Q, et al. Adipose mesenchymal stem cell-derived exosomes ameliorate hypoxia/serum deprivation-induced osteocyte apoptosis and osteocyte-mediated osteoclastogenesis in vitro. Biochem Biophys Res Commun. (2019) 508(1):138–44. doi: 10.1016/j.bbrc.2018.11.109
49. Tao SC, Yuan T, Rui BY, Zhu ZZ, Guo SC, Zhang CQ. Exosomes derived from human platelet-rich plasma prevent apoptosis induced by glucocorticoid-associated endoplasmic reticulum stress in rat osteonecrosis of the femoral head via the Akt/Bad/Bcl-2 signal pathway. Theranostics. (2017) 7(3):733–50. doi: 10.7150/thno.17450
50. Ho L, Tan SY, Wee S, Wu Y, Tan SJ, Ramakrishna NB, et al. ELABELA is an endogenous growth factor that sustains hESC self-renewal via the PI3K/AKT pathway. Cell Stem Cell. (2015) 17(4):435–47. doi: 10.1016/j.stem.2015.08.010
51. Zhang S, Chu WC, Lai RC, Lim SK, Hui JH, Toh WS. Exosomes derived from human embryonic mesenchymal stem cells promote osteochondral regeneration. Osteoarthritis Cartilage. (2016) 24(12):2135–40. doi: 10.1016/j.joca.2016.06.022
52. Guo SC, Tao SC, Yin WJ, Qi X, Sheng JG, Zhang CQ. Exosomes from human synovial-derived mesenchymal stem cells prevent glucocorticoid-induced osteonecrosis of the femoral head in the rat. Int J Biol Sci. (2016) 12(10):1262–72. doi: 10.7150/ijbs.16150
53. Kuang MJ, Huang Y, Zhao XG, Zhang R, Ma JX, Wang DC, et al. Exosomes derived from Wharton's jelly of human umbilical cord mesenchymal stem cells reduce osteocyte apoptosis in glucocorticoid-induced osteonecrosis of the femoral head in rats via the miR-21-PTEN-AKT signalling pathway. Int J Biol Sci. (2019) 15(9):1861–71. doi: 10.7150/ijbs.32262
54. Iuchi T, Akaike M, Mitsui T, Ohshima Y, Shintani Y, Azuma H, et al. Glucocorticoid excess induces superoxide production in vascular endothelial cells and elicits vascular endothelial dysfunction. Circ Res. (2003) 92(1):81–7. doi: 10.1161/01.RES.0000050588.35034.3C
55. El Zaoui I, Behar-Cohen F, Torriglia A. Glucocorticoids exert direct toxicity on microvasculature: analysis of cell death mechanisms. Toxicol Sci. (2015) 143(2):441–53. doi: 10.1093/toxsci/kfu243
56. Kerachian MA, Séguin C, Harvey EJ. Glucocorticoids in osteonecrosis of the femoral head: a new understanding of the mechanisms of action. J Steroid Biochem Mol Biol. (2009) 114(3-5):121–8. doi: 10.1016/j.jsbmb.2009.02.007
57. Williams TA, Verhovez A, Milan A, Veglio F, Mulatero P. Protective effect of spironolactone on endothelial cell apoptosis. Endocrinology. (2006) 147(5):2496–505. doi: 10.1210/en.2005-1318
58. Zhang Q, Li T, Li Z, Lu J, Wu X, Gao F, et al. Autocrine activity of extracellular vesicles induced by icariin and its effectiveness in glucocorticoid-induced injury of bone microvascular endothelial cells. Cells. (2022) 11(12):1921. doi: 10.3390/cells11121921
59. Greenberger S, Boscolo E, Adini I, Mulliken JB, Bischoff J. Corticosteroid suppression of VEGF-A in infantile hemangioma-derived stem cells. N Engl J Med. (2010) 362(11):1005–13. doi: 10.1056/NEJMoa0903036
60. Aschbacher K, Derakhshandeh R, Flores AJ, Narayan S, Mendes WB, Springer ML. Circulating angiogenic cell function is inhibited by cortisol in vitro and associated with psychological stress and cortisol in vivo. Psychoneuroendocrinology. (2016) 67:216–23. doi: 10.1016/j.psyneuen.2016.02.019
61. Nuttall ME, Gimble JM. Is there a therapeutic opportunity to either prevent or treat osteopenic disorders by inhibiting marrow adipogenesis? Bone. (2000) 27(2):177–84. doi: 10.1016/S8756-3282(00)00317-3
62. Hu GW, Li Q, Niu X, Hu B, Liu J, Zhou SM, et al. Exosomes secreted by human-induced pluripotent stem cell-derived mesenchymal stem cells attenuate limb ischemia by promoting angiogenesis in mice. Stem Cell Res Ther. (2015) 6(1):10. doi: 10.1186/scrt546
63. Anderson JD, Johansson HJ, Graham CS, Vesterlund M, Pham MT, Bramlett CS, et al. Comprehensive proteomic analysis of mesenchymal stem cell exosomes reveals modulation of angiogenesis via nuclear factor-kappaB signaling. Stem Cells. (2016) 34(3):601–13. doi: 10.1002/stem.2298
64. Salomon C, Ryan J, Sobrevia L, Kobayashi M, Ashman K, Mitchell M, et al. Exosomal signaling during hypoxia mediates microvascular endothelial cell migration and vasculogenesis. PLoS One. (2013) 8(7):e68451. doi: 10.1371/journal.pone.0068451
65. Shabbir A, Cox A, Rodriguez-Menocal L, Salgado M, Van Badiavas E. Mesenchymal stem cell exosomes induce proliferation and migration of normal and chronic wound fibroblasts, and enhance angiogenesis in vitro. Stem Cells Dev. (2015) 24(14):1635–47. doi: 10.1089/scd.2014.0316
66. Kalluri R, LeBleu VS. The biology, function, and biomedical applications of exosomes. Science. (2020) 367(6478). doi: 10.1126/science.aau6977
67. Yuan T, Guo SC, Han P, Zhang CQ, Zeng BF. Applications of leukocyte- and platelet-rich plasma (L-PRP) in trauma surgery. Curr Pharm Biotechnol. (2012) 13(7):1173–84. doi: 10.2174/138920112800624445
68. Qi X, Zhang J, Yuan H, Xu Z, Li Q, Niu X, et al. Exosomes secreted by human-induced pluripotent stem cell-derived mesenchymal stem cells repair critical-sized bone defects through enhanced angiogenesis and osteogenesis in osteoporotic rats. Int J Biol Sci. (2016) 12(7):836–49. doi: 10.7150/ijbs.14809
69. Zuo R, Kong L, Wang M, Wang W, Xu J, Chai Y, et al. Exosomes derived from human CD34(+) stem cells transfected with miR-26a prevent glucocorticoid-induced osteonecrosis of the femoral head by promoting angiogenesis and osteogenesis. Stem Cell Res Ther. (2019) 10(1):321. doi: 10.1186/s13287-019-1426-3
70. Hardy RS, Zhou H, Seibel MJ, Cooper MS. Glucocorticoids and bone: consequences of endogenous and exogenous excess and replacement therapy. Endocr Rev. (2018) 39(5):519–48. doi: 10.1210/er.2018-00097
71. Hartmann K, Koenen M, Schauer S, Wittig-Blaich S, Ahmad M, Baschant U, et al. Molecular actions of glucocorticoids in cartilage and bone during health, disease, and steroid therapy. Physiol Rev. (2016) 96(2):409–47. doi: 10.1152/physrev.00011.2015
72. Weinstein RS. Clinical practice. Glucocorticoid-induced bone disease. N Engl J Med. (2011) 365(1):62–70. doi: 10.1056/NEJMcp1012926
73. Israel E, Banerjee TR, Fitzmaurice GM, Kotlov TV, LaHive K, LeBoff MS. Effects of inhaled glucocorticoids on bone density in premenopausal women. N Engl J Med. (2001) 345(13):941–7. doi: 10.1056/NEJMoa002304
74. Phillips JE, Gersbach CA, Wojtowicz AM, García AJ. Glucocorticoid-induced osteogenesis is negatively regulated by Runx2/Cbfa1 serine phosphorylation. J Cell Sci. (2006) 119(Pt 3):581–91. doi: 10.1242/jcs.02758
75. Walsh LJ, Lewis SA, Wong CA, Cooper S, Oborne J, Cawte SA, et al. The impact of oral corticosteroid use on bone mineral density and vertebral fracture. Am J Respir Crit Care Med. (2002) 166(5):691–5. doi: 10.1164/rccm.2110047
76. Helfrich MH, Nesbitt SA, Lakkakorpi PT, Barnes MJ, Bodary SC, Shankar G, et al. Beta 1 integrins and osteoclast function: involvement in collagen recognition and bone resorption. Bone. (1996) 19(4):317–28. doi: 10.1016/S8756-3282(96)00223-2
77. Crockett JC, Mellis DJ, Scott DI, Helfrich MH. New knowledge on critical osteoclast formation and activation pathways from study of rare genetic diseases of osteoclasts: focus on the RANK/RANKL axis. Osteoporos Int. (2011) 22(1):1–20. doi: 10.1007/s00198-010-1272-8
78. Li J, Liu K, Liu Y, Xu Y, Zhang F, Yang H, et al. Exosomes mediate the cell-to-cell transmission of IFN-α-induced antiviral activity. Nat Immunol. (2013) 14(8):793–803. doi: 10.1038/ni.2647
79. Cui Y, Luan J, Li H, Zhou X, Han J. Exosomes derived from mineralizing osteoblasts promote ST2 cell osteogenic differentiation by alteration of microRNA expression. FEBS Lett. (2016) 590(1):185–92. doi: 10.1002/1873-3468.12024
80. Wei J, Li H, Wang S, Li T, Fan J, Liang X, et al. let-7 enhances osteogenesis and bone formation while repressing adipogenesis of human stromal/mesenchymal stem cells by regulating HMGA2. Stem Cells Dev. (2014) 23(13):1452–63. doi: 10.1089/scd.2013.0600
81. Zhao P, Xiao L, Peng J, Qian YQ, Huang CC. Exosomes derived from bone marrow mesenchymal stem cells improve osteoporosis through promoting osteoblast proliferation via MAPK pathway. Eur Rev Med Pharmacol Sci. (2018) 22(12):3962–70.29949171
82. Furuta T, Miyaki S, Ishitobi H, Ogura T, Kato Y, Kamei N, et al. Mesenchymal stem cell-derived exosomes promote fracture healing in a mouse model. Stem Cells Transl Med. (2016) 5(12):1620–30. doi: 10.5966/sctm.2015-0285
83. Qin Y, Wang L, Gao Z, Chen G, Zhang C. Bone marrow stromal/stem cell-derived extracellular vesicles regulate osteoblast activity and differentiation in vitro and promote bone regeneration in vivo. Sci Rep. (2016) 6:21961. doi: 10.1038/srep21961
84. Liao W, Ning Y, Xu HJ, Zou WZ, Hu J, Liu XZ, et al. BMSC-derived exosomes carrying microRNA-122-5p promote proliferation of osteoblasts in osteonecrosis of the femoral head. Clin Sci (Lond). (2019) 133(18):1955–75. doi: 10.1042/CS20181064
85. Raposo G, Nijman HW, Stoorvogel W, Liejendekker R, Harding CV, Melief CJ, et al. B lymphocytes secrete antigen-presenting vesicles. J Exp Med. (1996) 183(3):1161–72. doi: 10.1084/jem.183.3.1161
86. Zitvogel L, Regnault A, Lozier A, Wolfers J, Flament C, Tenza D, et al. Eradication of established murine tumors using a novel cell-free vaccine: dendritic cell-derived exosomes. Nat Med. (1998) 4(5):594–600. doi: 10.1038/nm0598-594
87. Théry C, Witwer KW, Aikawa E, Alcaraz MJ, Anderson JD, Andriantsitohaina R, et al. Minimal information for studies of extracellular vesicles 2018 (MISEV2018): a position statement of the International Society for Extracellular Vesicles and update of the MISEV2014 guidelines. J Extracell Vesicles. (2018) 7(1):1535750. doi: 10.1080/20013078.2018.1535750
88. Escola JM, Kleijmeer MJ, Stoorvogel W, Griffith JM, Yoshie O, Geuze HJ. Selective enrichment of tetraspan proteins on the internal vesicles of multivesicular endosomes and on exosomes secreted by human B-lymphocytes. J Biol Chem. (1998) 273(32):20121–7. doi: 10.1074/jbc.273.32.20121
89. Xu Q, Cui Y, Luan J, Zhou X, Li H, Han J. Exosomes from C2C12 myoblasts enhance osteogenic differentiation of MC3T3-E1 pre-osteoblasts by delivering miR-27a-3p. Biochem Biophys Res Commun. (2018) 498(1):32–7. doi: 10.1016/j.bbrc.2018.02.144
90. Kulkarni R, Bajaj M, Ghode S, Jalnapurkar S, Limaye L, Kale VP. Intercellular transfer of microvesicles from young mesenchymal stromal cells rejuvenates aged murine hematopoietic stem cells. Stem Cells. (2018) 36(3):420–33. doi: 10.1002/stem.2756
91. Toh WS, Lai RC, Hui JHP, Lim SK. MSC exosome as a cell-free MSC therapy for cartilage regeneration: Implications for osteoarthritis treatment. Semin Cell Dev Biol. (2017) 67:56–64. doi: 10.1016/j.semcdb.2016.11.008
92. Li Z, Wang Y, Xiang S, Zheng Z, Bian Y, Feng B, et al. Chondrocytes-derived exosomal miR-8485 regulated the Wnt/β-catenin pathways to promote chondrogenic differentiation of BMSCs. Biochem Biophys Res Commun. (2020) 523(2):506–13. doi: 10.1016/j.bbrc.2019.12.065
93. Lu J, Zhang Y, Liang J, Diao J, Liu P, Zhao H. Role of exosomal microRNAs and their crosstalk with oxidative stress in the pathogenesis of osteoporosis. Oxid Med Cell Longev. (2021) 2021:6301433. doi: 10.1155/2021/6301433
94. Chen G, Huang G, Lin H, Wu X, Tan X, Chen Z. MicroRNA-425-5p modulates osteoporosis by targeting annexin A2. Immun Ageing. (2021) 18(1):45. doi: 10.1186/s12979-021-00256-7
95. Wu X, Zhang Y, Guo X, Xu H, Xu Z, Duan D, et al. Identification of differentially expressed microRNAs involved in non-traumatic osteonecrosis through microRNA expression profiling. Gene. (2015) 565(1):22–9. doi: 10.1016/j.gene.2015.03.072
96. Bian Y, Qian W, Li H, Zhao RC, Shan WX, Weng X. Pathogenesis of glucocorticoid-induced avascular necrosis: a microarray analysis of gene expression in vitro. Int J Mol Med. (2015) 36(3):678–84. doi: 10.3892/ijmm.2015.2273
97. Yuan HF, Christina VR, Guo CA, Chu YW, Liu RH, Yan ZQ. Involvement of microRNA-210 demethylation in steroid-associated osteonecrosis of the femoral head. Sci Rep. (2016) 6:20046. doi: 10.1038/srep20046
98. Yamasaki K, Nakasa T, Miyaki S, Yamasaki T, Yasunaga Y, Ochi M. Angiogenic microRNA-210 is present in cells surrounding osteonecrosis. J Orthop Res. (2012) 30(8):1263–70. doi: 10.1002/jor.22079
99. Sun J, Wang Y, Li Y, Zhao G. Downregulation of PPARγ by miR-548d-5p suppresses the adipogenic differentiation of human bone marrow mesenchymal stem cells and enhances their osteogenic potential. J Transl Med. (2014) 12:168. doi: 10.1186/1479-5876-12-168
100. Aoyama T, Fujita Y, Madoba K, Nankaku M, Yamada M, Tomita M, et al. Rehabilitation program after mesenchymal stromal cell transplantation augmented by vascularized bone grafts for idiopathic osteonecrosis of the femoral head: a preliminary study. Arch Phys Med Rehabil. (2015) 96(3):532–9. doi: 10.1016/j.apmr.2014.09.040
101. Zhao D, Liu B, Wang B, Yang L, Xie H, Huang S, et al. Autologous bone marrow mesenchymal stem cells associated with tantalum rod implantation and vascularized iliac grafting for the treatment of end-stage osteonecrosis of the femoral head. Biomed Res Int. (2015) 2015:240506.25802840
102. Wang C, Wang Y, Meng HY, Yuan XL, Xu XL, Wang AY, et al. Application of bone marrow mesenchymal stem cells to the treatment of osteonecrosis of the femoral head. Int J Clin Exp Med. (2015) 8(3):3127–35.26064202
103. Zhao Y, Xu J. Synovial fluid-derived exosomal lncRNA PCGEM1 as biomarker for the different stages of osteoarthritis. Int Orthop. (2018) 42(12):2865–72. doi: 10.1007/s00264-018-4093-6
104. Dragomir M, Chen B, Calin GA. Exosomal lncRNAs as new players in cell-to-cell communication. Transl Cancer Res. (2018) 7(Suppl 2):S243–52. doi: 10.21037/tcr.2017.10.46
105. Weiliang Z, Lili G. Research advances in the application of adipose-derived stem cells derived exosomes in cutaneous wound healing. Ann Dermatol. (2021) 33(4):309–17. doi: 10.5021/ad.2021.33.4.309
106. Wang SZ, Jia J, Chen CH. lncRNA-KCNQ1OT1: a potential target in exosomes derived from adipose-derived stem cells for the treatment of osteoporosis. Stem Cells Int. (2021) 2021:7690006. doi: 10.1155/2021/7690006
107. Luo H, Lan W, Li Y, Lian X, Zhang N, Lin X, et al. Microarray analysis of long-noncoding RNAs and mRNA expression profiles in human steroid-induced avascular necrosis of the femoral head. J Cell Biochem. (2019) 120(9):15800–113. doi: 10.1002/jcb.28850
108. Xiang S, Li Z, Weng X. The role of lncRNA RP11-154D6 in steroid-induced osteonecrosis of the femoral head through BMSC regulation. J Cell Biochem. (2019) 120(10):18435–45. doi: 10.1002/jcb.29161
109. Liu Y, Lin L, Zou R, Wen C, Wang Z, Lin F. MSC-derived exosomes promote proliferation and inhibit apoptosis of chondrocytes via lncRNA-KLF3-AS1/miR-206/GIT1 axis in osteoarthritis. Cell Cycle. (2018) 17(21–22):2411–22. doi: 10.1080/15384101.2018.1526603
110. Wei B, Wei W, Zhao B, Guo X, Liu S. Long non-coding RNA HOTAIR inhibits miR-17-5p to regulate osteogenic differentiation and proliferation in non-traumatic osteonecrosis of femoral head. PLoS One. (2017) 12(2):e0169097. doi: 10.1371/journal.pone.0169097
111. Harding C, Heuser J, Stahl P. Receptor-mediated endocytosis of transferrin and recycling of the transferrin receptor in rat reticulocytes. J Cell Biol. (1983) 97(2):329–39. doi: 10.1083/jcb.97.2.329
112. Yu QS, Guo WS, Cheng LM, Lu YF, Shen JY, Li P. Glucocorticoids significantly influence the transcriptome of bone microvascular endothelial cells of human femoral head. Chin Med J (Engl). (2015) 128(14):1956–63. doi: 10.4103/0366-6999.160564
113. Potente M, Urbich C, Sasaki K, Hofmann WK, Heeschen C, Aicher A, et al. Involvement of Foxo transcription factors in angiogenesis and postnatal neovascularization. J Clin Invest. (2005) 115(9):2382–92. doi: 10.1172/JCI23126
114. Fang B, Li Y, Chen C, Wei Q, Zheng J, Liu Y, et al. Huo Xue Tong Luo capsule ameliorates osteonecrosis of femoral head through inhibiting lncRNA-Miat. J Ethnopharmacol. (2019) 238:111862. doi: 10.1016/j.jep.2019.111862
115. Memczak S, Jens M, Elefsinioti A, Torti F, Krueger J, Rybak A, et al. Circular RNAs are a large class of animal RNAs with regulatory potency. Nature. (2013) 495(7441):333–8. doi: 10.1038/nature11928
116. Hansen TB, Jensen TI, Clausen BH, Bramsen JB, Finsen B, Damgaard CK, et al. Natural RNA circles function as efficient microRNA sponges. Nature. (2013) 495(7441):384–8. doi: 10.1038/nature11993
117. Li Z, Huang C, Bao C, Chen L, Lin M, Wang X, et al. Exon-intron circular RNAs regulate transcription in the nucleus. Nat Struct Mol Biol. (2015) 22(3):256–64. doi: 10.1038/nsmb.2959
118. Kulcheski FR, Christoff AP, Margis R. Circular RNAs are miRNA sponges and can be used as a new class of biomarker. J Biotechnol. (2016) 238:42–51. doi: 10.1016/j.jbiotec.2016.09.011
119. Bretscher MS. Direct translation of a circular messenger DNA. Nature. (1968) 220(5172):1088–91. doi: 10.1038/2201088a0
120. Abe N, Matsumoto K, Nishihara M, Nakano Y, Shibata A, Maruyama H, et al. Rolling circle translation of circular RNA in living human cells. Sci Rep. (2015) 5:16435. doi: 10.1038/srep16435
121. Du WW, Zhang C, Yang W, Yong T, Awan FM, Yang BB. Identifying and characterizing circRNA-protein interaction. Theranostics. (2017) 7(17):4183–91. doi: 10.7150/thno.21299
122. Zhi F, Ding Y, Wang R, Yang Y, Luo K, Hua F. Exosomal hsa_circ_0006859 is a potential biomarker for postmenopausal osteoporosis and enhances adipogenic versus osteogenic differentiation in human bone marrow mesenchymal stem cells by sponging miR-431-5p. Stem Cell Res Ther. (2021) 12(1):157. doi: 10.1186/s13287-021-02214-y
123. Zhang M, Jia L, Zheng Y. circRNA expression profiles in human bone marrow stem cells undergoing osteoblast differentiation. Stem Cell Rev Rep. (2019) 15(1):126–38. doi: 10.1007/s12015-018-9841-x
124. Zhao R, Li Y, Lin Z, Wan J, Xu C, Zeng Y, et al. miR-199b-5p modulates BMSC osteogenesis via suppressing GSK-3β/β-catenin signaling pathway. Biochem Biophys Res Commun. (2016) 477(4):749–54. doi: 10.1016/j.bbrc.2016.06.130
125. Huang L, Wang Y, Jiang Y, Wu Y, Hu C, Ouyang H. High levels of GSK-3β signalling reduce osteogenic differentiation of stem cells in osteonecrosis of femoral head. J Biochem. (2018) 163(3):243–51. doi: 10.1093/jb/mvx076
126. Kuang MJ, Xing F, Wang D, Sun L, Ma JX, Ma XL. CircUSP45 inhibited osteogenesis in glucocorticoid-induced osteonecrosis of femoral head by sponging miR-127-5p through PTEN/AKT signal pathway: Experimental studies. Biochem Biophys Res Commun. (2019) 509(1):255–61. doi: 10.1016/j.bbrc.2018.12.116
127. Qian DY, Yan GB, Bai B, Chen Y, Zhang SJ, Yao YC, et al. Differential circRNA expression profiles during the BMP2-induced osteogenic differentiation of MC3T3-E1 cells. Biomed Pharmacother. (2017) 90:492–9. doi: 10.1016/j.biopha.2017.03.051
128. Cherubini A, Barilani M, Rossi RL, Jalal MMK, Rusconi F, Buono G, et al. FOXP1 circular RNA sustains mesenchymal stem cell identity via microRNA inhibition. Nucleic Acids Res. (2019) 47(10):5325–40. doi: 10.1093/nar/gkz199
129. Boeckel JN, Jaé N, Heumüller AW, Chen W, Boon RA, Stellos K, et al. Identification and characterization of hypoxia-regulated endothelial circular RNA. Circ Res. (2015) 117(10):884–90. doi: 10.1161/CIRCRESAHA.115.306319
130. Dang RY, Liu FL, Li Y. Circular RNA hsa_circ_0010729 regulates vascular endothelial cell proliferation and apoptosis by targeting the miR-186/HIF-1α axis. Biochem Biophys Res Commun. (2017) 490(2):104–10. doi: 10.1016/j.bbrc.2017.05.164
131. Li CY, Ma L, Yu B. Circular RNA hsa_circ_0003575 regulates oxLDL induced vascular endothelial cells proliferation and angiogenesis. Biomed Pharmacother. (2017) 95:1514–9. doi: 10.1016/j.biopha.2017.09.064
132. Gross JC, Chaudhary V, Bartscherer K, Boutros M. Active Wnt proteins are secreted on exosomes. Nat Cell Biol. (2012) 14(10):1036–45. doi: 10.1038/ncb2574
133. Buschow SI, Liefhebber JM, Wubbolts R, Stoorvogel W. Exosomes contain ubiquitinated proteins. Blood Cells Mol Dis. (2005) 35(3):398–403. doi: 10.1016/j.bcmd.2005.08.005
134. Théry C, Regnault A, Garin J, Wolfers J, Zitvogel L, Ricciardi-Castagnoli P, et al. Molecular characterization of dendritic cell-derived exosomes. Selective accumulation of the heat shock protein hsc73. J Cell Biol. (1999) 147(3):599–610. doi: 10.1083/jcb.147.3.599
135. Yuan D, Zhao Y, Banks WA, Bullock KM, Haney M, Batrakova E, et al. Macrophage exosomes as natural nanocarriers for protein delivery to inflamed brain. Biomaterials. (2017) 142:1–12. doi: 10.1016/j.biomaterials.2017.07.011
136. Whiteside TL. Exosomes carrying immunoinhibitory proteins and their role in cancer. Clin Exp Immunol. (2017) 189(3):259–67. doi: 10.1111/cei.12974
137. Goetzl EJ, Kapogiannis D, Schwartz JB, Lobach IV, Goetzl L, Abner EL, et al. Decreased synaptic proteins in neuronal exosomes of frontotemporal dementia and Alzheimer's disease. Faseb J. (2016) 30(12):4141–8. doi: 10.1096/fj.201600816R
138. Tsuno H, Arito M, Suematsu N, Sato T, Hashimoto A, Matsui T, et al. A proteomic analysis of serum-derived exosomes in rheumatoid arthritis. BMC Rheumatol. (2018) 2:35. doi: 10.1186/s41927-018-0041-8
139. Kawakami K, Fujita Y, Matsuda Y, Arai T, Horie K, Kameyama K, et al. Gamma-glutamyltransferase activity in exosomes as a potential marker for prostate cancer. BMC Cancer. (2017) 17(1):316. doi: 10.1186/s12885-017-3301-x
140. Takahashi A, Okada R, Nagao K, Kawamata Y, Hanyu A, Yoshimoto S, et al. Exosomes maintain cellular homeostasis by excreting harmful DNA from cells. Nat Commun. (2017) 8:15287. doi: 10.1038/ncomms15287
141. Hoeijmakers JH. DNA damage, aging, and cancer. N Engl J Med. (2009) 361(15):1475–85. doi: 10.1056/NEJMra0804615
142. Burger D, Viñas JL, Akbari S, Dehak H, Knoll W, Gutsol A, et al. Human endothelial colony-forming cells protect against acute kidney injury: role of exosomes. Am J Pathol. (2015) 185(8):2309–23. doi: 10.1016/j.ajpath.2015.04.010
143. Xin H, Li Y, Chopp M. Exosomes/miRNAs as mediating cell-based therapy of stroke. Front Cell Neurosci. (2014) 8:377. doi: 10.3389/fncel.2014.00377
144. Kalra H, Adda CG, Liem M, Ang CS, Mechler A, Simpson RJ, et al. Comparative proteomics evaluation of plasma exosome isolation techniques and assessment of the stability of exosomes in normal human blood plasma. Proteomics. (2013) 13(22):3354–64. doi: 10.1002/pmic.201300282
145. Mendt M, Kamerkar S, Sugimoto H, McAndrews KM, Wu CC, Gagea M, et al. Generation and testing of clinical-grade exosomes for pancreatic cancer. JCI Insight. (2018) 3(8). doi: 10.1172/jci.insight.99263
146. Koga Y, Yasunaga M, Moriya Y, Akasu T, Fujita S, Yamamoto S, et al. Exosome can prevent RNase from degrading microRNA in feces. J Gastrointest Oncol. (2011) 2(4):215–22. doi: 10.3978/j.issn.2078-6891.2011.015
147. Kamerkar S, LeBleu VS, Sugimoto H, Yang S, Ruivo CF, Melo SA, et al. Exosomes facilitate therapeutic targeting of oncogenic KRAS in pancreatic cancer. Nature. (2017) 546(7659):498–503. doi: 10.1038/nature22341
148. Grange C, Tapparo M, Bruno S, Chatterjee D, Quesenberry PJ, Tetta C, et al. Biodistribution of mesenchymal stem cell-derived extracellular vesicles in a model of acute kidney injury monitored by optical imaging. Int J Mol Med. (2014) 33(5):1055–63. doi: 10.3892/ijmm.2014.1663
149. Peinado H, Alečković M, Lavotshkin S, Matei I, Costa-Silva B, Moreno-Bueno G, et al. Melanoma exosomes educate bone marrow progenitor cells toward a pro-metastatic phenotype through MET. Nat Med. (2012) 18(6):883–91. doi: 10.1038/nm.2753
150. Kotmakçı M, Bozok Çetintaş V. Extracellular vesicles as natural nanosized delivery systems for small-molecule drugs and genetic material: steps towards the future nanomedicines. J Pharm Pharm Sci. (2015) 18(3):396–413. doi: 10.18433/J36W3X
151. Tian Y, Li S, Song J, Ji T, Zhu M, Anderson GJ, et al. A doxorubicin delivery platform using engineered natural membrane vesicle exosomes for targeted tumor therapy. Biomaterials. (2014) 35(7):2383–90. doi: 10.1016/j.biomaterials.2013.11.083
152. Ohno S, Takanashi M, Sudo K, Ueda S, Ishikawa A, Matsuyama N, et al. Systemically injected exosomes targeted to EGFR deliver antitumor microRNA to breast cancer cells. Mol Ther. (2013) 21(1):185–91. doi: 10.1038/mt.2012.180
Keywords: Exosomes, glucocorticoid, osteonecrosis, femoral head, treatment
Citation: Lv B, Cheng Z, Yu Y, Chen Y, Gan W, Li S, Zhao K, Yang C and Zhang Y (2022) Therapeutic perspectives of exosomes in glucocorticoid-induced osteoarthrosis. Front. Surg. 9:836367. doi: 10.3389/fsurg.2022.836367
Received: 15 December 2022; Accepted: 8 July 2022;
Published: 2 August 2022.
Edited by:
Carlos Suarez-Ahedo, Hospital Médica Sur, MexicoReviewed by:
Yuan Xiao, Shanghai Jiao Tong University, China© 2022 Lv, Cheng, Yu, Chen, Gan, Li, Zhao, Yang and Zhang. This is an open-access article distributed under the terms of the Creative Commons Attribution License (CC BY). The use, distribution or reproduction in other forums is permitted, provided the original author(s) and the copyright owner(s) are credited and that the original publication in this journal is cited, in accordance with accepted academic practice. No use, distribution or reproduction is permitted which does not comply with these terms.
*Correspondence: Yukun Zhang emhhbmd5dWt1bmNvbUAxMjYuY29t Cao Yang Y2FveWFuZ3VuaW9uQGh1c3QuZWR1LmNu Kangcheng Zhao emhhb191aEBodXN0LmVkdS5jbg== Bin Lv ZHJfbHZiaW5Ac2luYS5jb20=
†These authors have contributed equally to this work
Specialty Section: This article was submitted to Orthopedic Surgery, a section of the journal Frontiers in Surgery
Disclaimer: All claims expressed in this article are solely those of the authors and do not necessarily represent those of their affiliated organizations, or those of the publisher, the editors and the reviewers. Any product that may be evaluated in this article or claim that may be made by its manufacturer is not guaranteed or endorsed by the publisher.
Research integrity at Frontiers
Learn more about the work of our research integrity team to safeguard the quality of each article we publish.