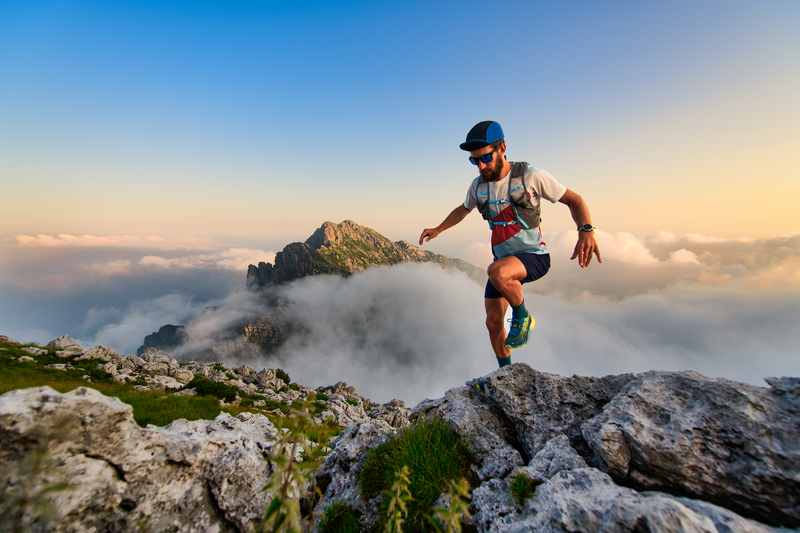
95% of researchers rate our articles as excellent or good
Learn more about the work of our research integrity team to safeguard the quality of each article we publish.
Find out more
MINI REVIEW article
Front. Surg. , 16 August 2021
Sec. Reconstructive and Plastic Surgery
Volume 8 - 2021 | https://doi.org/10.3389/fsurg.2021.680186
Importance: Reconstruction of facial deformity poses a significant surgical challenge due to the psychological, functional, and aesthetic importance of this anatomical area. There is a need to provide not only an excellent colour and contour match for skin defects, but also a durable cartilaginous structural replacement for nasal or auricular defects. The purpose of this review is to describe the history of, and state-of-the-art techniques within, facial cartilaginous surgery, whilst highlighting recent advances and future directions for this continually advancing specialty.
Observations: Limitations of synthetic implants for nasal and auricular reconstruction, such as silicone and porous polyethylene, have meant that autologous cartilage tissue for such cases remains the current gold standard. Similarly, tissue engineering approaches using unrelated cells and synthetic scaffolds have shown limited in vivo success. There is increasing recognition that both the intrinsic and extrinsic microenvironment are important for tissue engineering and synthetic scaffolds fail to provide the necessary cues for cartilage matrix secretion.
Conclusions and Relevance: We discuss the first-in-man studies in the context of biomimetic and developmental approaches to engineering durable cartilage for clinical translation. Implementation of engineered autologous tissue into clinical practise could eliminate donor site morbidity and represent the next phase of the facial reconstruction evolution.
The reconstruction of facial defects continues to pose a significant surgical challenge. Facial disfigurements, including nasal and auricular defects following trauma, burns, skin cancer resection, and congenital conditions requiring reconstruction affect 569,000 (or 1 in 111) people in the United Kingdom (1). Facial deformity and scarring can have a devastating effect on an individual's appearance, psychological health and subsequently on their quality of life (2–4). When reconstructing facial defects all rungs of the reconstructive ladder must therefore be utilised to replace tissue like-for-like and ultimately optimise both the functional and cosmetic outcomes.
Skin grafts and local flaps are widely used within facial plastic surgery where there is a cutaneous defect (5–8). For larger areas, or in sites of reduced vascularity, the only viable option may be reconstruction with a free flap (9, 10). The anterolateral thigh and radial forearm flaps have traditionally been used in head and neck reconstruction with the free fibula flap also providing an autologous source of bone (11). Revision procedures in order to de-bulk the flap and improve cosmesis, however, are not uncommon (10, 12).
Despite advances in surgical techniques, one area that remains a challenge within facial plastic surgery is the reconstruction of defects where there is a deficiency of cartilage. This is in part due to cartilage, unlike other tissue types, having no ability for repair and regeneration. Although synthetic options for nasal (13) and auricular (14) reconstruction have been described, the use of autologous tissue for such cases remains the current gold standard (Table 1) (15). Such grafts can be harvested from the ear, septum or costal cartilages. However, as with all autologous reconstruction there is a limited amount of tissue available as well as the potential for donor site morbidity. In order to address this problem recent advances in tissue engineering and 3D bioprinting aim to provide clinicians with the ability to create autologous cartilage within the laboratory setting, potentially changing the field of facial reconstructive surgery forever (16, 17). The purpose of this review is to describe the history of, and current techniques within, facial cartilaginous surgery whilst also outlining future directions for this continually advancing specialty.
The first mention of the treatment of facial trauma is found in the Edwin Smith Surgical Papyrus dated circa 3000 BC (18). Total nasal reconstruction and partial auricular reconstruction around 600 BC constitute the first chapter in the history of not only facial reconstruction but plastic reconstructive surgery in general (Figure 1) (19, 20). These principles were handed down through civilisations, with the renaissance of the 14th century bringing a rebirth of reconstructive surgery, particularly through the work of Italian surgeons Branca (1430) and Benedetti (1497) who were instrumental in developing rhinoplasty techniques and Tagliacozzi (1597) who developed numerous auricular reconstructive techniques (Figure 1).
World War I produced the greatest number of facial injuries and burns in the history of warfare and led to the development of modern facial reconstruction by Gillies (20). Gillies developed tubed pedicled flaps for facial reconstruction, as well as pioneering rib autografts and allografts for auricular reconstruction and composite chondrocutaneous grafts for nasal reconstruction (Figure 1) (21–24). These early cartilage grafts did, however, suffer from significant rates of resorption (22). More recent attempts to use irradiated allogenic grafts from cadaveric donors also demonstrated over 70% resorption, as well as concerns regarding risk of disease transmission (23, 24).
The use of alloplastic implants for facial reconstruction has been widely described. These synthetic materials have pores of varying sizes that, when present, allow for tissue ingrowth but can also result in bacterial colonisation and subsequent infection (13). Cronin (14) was the first to introduce silicone ear frameworks. Although silicone is easily moulded into the desired shape, its nonporous structure prevents tissue ingrowth which can result in capsule formation and subsequent implant distortion (13). Cronin (14) also reported a high incidence of extrusion, the risk of which is lifelong (13). In 1991 Reinsich introduced porous polyethylene implants (Medpor® Stryker, Kalmanzoo, MI, USA) for reconstructing cartilaginous facial defects, which although still in use today, are limited by high rates of infection, functional compromise such as nasal blockage and a sense of reconstructive inadequacy and dissatisfaction from the patient's perspective (13, 25–27). Particle formation followed by the subsequent inflammatory reaction also remains a concern with these implants (13).
Allografts have primarily been used for auricular and nasal reconstruction. Although avoiding the problem of donor site morbidity, early grafts suffered from significant rates of resorption (22). More recent attempts to use irradiated allogenic grafts from cadaveric donors also demonstrated over 70% resorption, as well as concerns regarding risk of disease transmission (23, 24). Concerns regarding changes in the surface topography of the graft over time and graft warping/distortion leading to deformity have also been raised (13).
The limitations of allografts and alloplastic implants have meant that much of the focus of modern cartilaginous auricular reconstruction has been on autologous cartilage, which was first described by Tanzer (28) and subsequently refined by Brent (29), Park (30), Nagata (31), and Firmin (15). The technique of using carved autologous costal cartilage remains the current gold standard for total auricular reconstruction worldwide today (15, 31). Smaller facial cartilaginous defects can be reconstructed using autologous cartilage grafts from either the costal, auricular or nasoseptal regions (32). The benefits of autologous reconstruction compared to synthetic implants are high biocompatibility, immunocompatibility and the ability to grow with the patient (16). However, several factors limit their utility, including the finite amount of cartilage available, associated morbidity of surgical harvest and complex three-dimensional geometry, which requires a high level of technical surgical skill (16). The risk of graft warping leading to poor long-term outcomes also remains an issue with autografts. This change in shape is thought to be due to protein polysaccharides contained within the cartilage producing tensile stresses in response to graft carving (23). It commences within the first 30 minutes after the graft is carved and can continue for weeks afterwards (23).
Auricular reconstruction is an ideal example of how refinement in surgical techniques over many years can give excellent results in expert hands. There have been various advances in the surgical approach to autologous auricular reconstruction; these include the transition towards single-stage procedures (30) as well as the use of 3D imaging and 3D models to aid surgical planning (33, 34). However, as with any autologous technique, facial cartilaginous reconstruction is limited by donor site morbidity. Engineering autologous cartilage could overcome these limitations but remains at the proof-of-concept stage and is not yet ready for widespread clinical application.
Although Buncke and Schulz (35) established the technical details surrounding microvascular ear replantation in 1964, it was not until 1980 that the first successful clinical case was reported in the literature (36). Nasal and auricular replantation using microsurgical techniques have been described in recent years with (37) and without (38, 39) venous anastomoses. Advancement of microsurgical techniques and immunosuppression made composite tissue allotransplantation a reality with the first facial transplant reported in 2005 (40).
Despite these developments, we are still confronted with shortcomings relating to the availability of donor tissues and complications of long-term immunosuppression. In order to overcome this, novel approaches have been investigated that combine advancements in nanotechnology (41), cell biology, biomaterials (42) and 3D printing (43), to engineer autologous tissues in the laboratory with a real potential for a paradigm shift in reconstructive surgery (17). Implementation of engineered autologous tissue into clinical practice could potentially eliminate the need for donor sites and their morbidity, and in addition to this reduce hospital stay and associated costs in the long term (44, 45). The surgical community worldwide is becoming increasingly aware of the importance of this field of research, and The American Society of Plastic surgeons have highlighted the need to continue to translate bench research in tissue engineering into clinical practise (46). Furthermore, the United Kingdom Government highlighted regenerative medicine as one of the “eight great technologies” worthy of significant investment with great potential to impact the health service (47).
In order to create durable cartilage, it is important to first understand its native macro, micro, and nano-architecture (48) as well as developmental and maturation processes (16). As it is avascular, aneural, and immune-privileged, cartilage is perceived to be a relatively simple tissue to replicate (49). Cartilage consists of isolated chondrocytes within lacunae amidst extracellular matrix containing type II collagen, proteoglycans, elastic fibres, and other proteins that satisfy its structural and functional role. Articular cartilage is recognised to have a well-defined zonal organisation, where extracellular matrix and cellular organisation varies with tissue depth, and this has been shown to affect its physical properties (50). Nasoseptal cartilage has generally been assumed to be isotropic, but we know that malrotation of surgical cartilage grafts have been reported to lead to increased graft absorption or distortion (51, 52). This suggests that orientation affects the strength of the graft and indicates anisotropy which is confirmed by studies demonstrating zonal organisation, decreased cell to matrix ratio and water content but an increase in glycosaminoglycans in mature compared to immature cartilage, correlating with greater compressive stiffness (53–55).
Much like immature native cartilage, tissue engineered neocartilage, often consisting of cellular, isotropic and homogenous tissue, has also experienced problematic reabsorption rates in the literature (56, 57). The reasons for this are likely to be multifactorial, but the importance of anisotropy for engineering durable tissue cannot be overlooked, as we know it affects biomechanical strength of tissues (51, 52).
The landmark study of auricular tissue engineering by the Vacanti group led to the iconic image of the tissue engineered ear-shaped appendage xenografted onto the back of immunocompromised mice (56). The implanted constructs were made from bovine chondrocytes seeded onto polyglycolic acid-polylactic acid scaffolds and supported by externally fixed stents. However, once the stents were removed, the 3D shape eventually deformed, highlighting the often-overlooked features of tissue-engineered constructs, including the lack of long-term biochemical and biomechanical stability (16). This has largely been attributed to a failure in adequately maturing the constructs prior to implantation. This leads to a failure in achieving the matured anisotropic microarchitecture and thus biomechanical strength of native cartilage with its ability to withstand the forces of soft tissue cover. This paper marked the start of attempts to engineer cartilage tissue for facial reconstruction, with most studies being in vitro or using nude mice (58–60), with very few using immunocompetent animal models or in vitro maturation prior to implantation (61–63).
The biomimetic approach contrasts with these recent attempts to tissue engineer cartilage which have been based on synthetic scaffolds and unrelated mesenchymal stem cells (MSCs). This is largely because the goal by various groups involved has been to produce constructs with mechanical properties that match autologous cartilage for immediate implantation rather than supporting the cell itself to secrete autologous cartilage matrix prior to implantation. A wide variety of synthetic polymers have previously been trialled but were shown to be suboptimal due to increased susceptibility to infection, extrusion, and immune response to degradation products, all of which are reminiscent of the historical lessons of alloplastic implants (14, 64, 65). The use of synthetic nanocomposites seeded with autologous bone marrow cells for tracheobronchial transplantation have led to well-publicised controversies in the tissue engineering field (66). There have been attempts to improve biocompatibility of synthetic scaffolds. Oseni et al. for example have used nanocomposite polymer polyhedral oligomeric silsesquioxane nanocages to improve the biocompatibility and biostability of polycarbonate urethane with MSCs (67).
It was more than 20 years after the Vacanti mouse when Cao's group in Beijing used implanted tissue engineered ear-shaped cartilage in a single-stage for total auricular reconstruction in five microtia patients (68). The group used chondrocytes isolated from microtia cartilage which was seeded on polycaprolactone mesh, wrapped with polyglycolic acid, and subsequently coated with polylactic acid to engineer the cartilage in vitro prior to its implantation. Follow up ranged from 2 months to two and a half years and revealed that four out of five cases demonstrated cartilage on post implantation biopsy. The detailed 3D structure was, however, compromised and this may be related to degradation of the inner polycaprolactone core. Longer term follow-up is required in order to establish clinical outcomes after complete degradation of the polycaprolactone core. Other scaffold-free approaches to clinical translation of tissue-engineered cartilage includes a two-stage approach. This involves cells being injected subcutaneously into the lower abdomen, and the in-vivo regenerated cartilage being subsequently further hand-carved into an ear-shaped framework and re-implanted into the final position (69, 70). However, with these grafts having to be carved one must question whether graft warping would occur which is an issue that has been seen with autologous grafts and must be addressed with newer technologies moving forward.
Ivan Martin's group in Basel used autologous tissue engineered constructs in a first in human trial for nasal reconstruction (71). The trial consisted of using nasal septum chondrocytes seeded onto fibrous collagen scaffolds for nasal ala reconstruction following non-melanoma skin cancer excision. In total, five adult patients were involved and follow up after 1 year revealed good patient satisfaction with both the aesthetic and functional outcomes (71). However, these were small defects and in addition no post-implantation biopsy was performed to confirm that cartilage rather than scar tissue had formed. To date, there have been no reports of tissue engineering applications for total nasal reconstruction.
There is increasing recognition that both the cell (intrinsic) and microenvironment (extrinsic) are important for tissue engineering and that synthetic scaffolds fail to provide the necessary cues for cartilage matrix secretion (72). The importance of cells is supported by the literature, where cells alone were able to regenerate cartilage in vivo and formed the basis of autologous chondrocyte implantation treatments for osteoarthritis (69, 73). Other groups have used either bovine auricular chondrocytes (74) or equine auricular progenitor cells (75) to demonstrate feasibility of biomimetic approaches through seeding on natural scaffolds. Ivan Martin's group, using human nasoseptal chondrocytes and natural scaffolds (hyaluronan and collagen), recognised that in vitro pre-culture prior to in vivo implantation enhances mechanical properties of cartilage grafts (76). This may explain why this approach achieved the first successful in-human tissue engineered cartilage in facial reconstruction where other groups failed (71).
It is widely believed that to be able to engineer durable native cartilage in vitro, it is important to not only understand native macro- (i.e., overall shape of the tissue or organ), micro- (composition of extracellular matrix, pore shape and size vs. cell shape and size) and nanoarchitecture (nanotopography and biomolecule attachments of extracellular matrix for optimal cell adhesion and proliferation) of the tissue (48, 76), but also the developmental pathway involved in creating that architecture (77). Cartilage development is largely controlled by complex FGF-TGFβ-Wnt crosstalk that occurs in vivo to allow proliferation of undifferentiated MSCs prior to condensation and chondrogenic differentiation (77). Part of the developmental principle approach is to use cartilage specific progenitor/stem cells, superior to unrelated MSCs for cartilage matrix secretion (78, 79), platform technologies such as 3D bioprinting, to replicate native microscopic anisotropy and macroscopic 3D anatomy and thereby functionality (16, 45), as well as natural scaffolds or bioinks that encourage both cell adhesion and chondrogenesis (80). Induction and maintenance of maturation either through simple in vitro pre-culture or physiological culture conditions in bioreactors will be important for providing durability of the tissue-engineered construct (16, 45). The potential success of these approaches does, however, remain to be seen in first-in-man studies and could represent the next phase of the facial reconstruction evolution.
ZJ is the lead author of the paper and along with IW founded the idea for the review. Both contributed singificantly to the preparation of the manuscript. TD, AH, and KS assisted with the literature search whilst also contributing to the writing of the manuscript. All authors have reviewed and approved the final manuscript and agreed to be accountable for all aspects of the work.
Research reported in this study was supported by the Medical Research Council (MR/N002431/1), The Royal College of Surgeons of England, The British Association of Plastic, Reconstructive, and Aesthetic Surgeons (BAPRAS), The Scar Free Foundation and the Fulbright Commission. ZJ and TD were funded by the Welsh Clinical Academic Training (WCAT) Fellowship.
The authors declare that the research was conducted in the absence of any commercial or financial relationships that could be construed as a potential conflict of interest.
All claims expressed in this article are solely those of the authors and do not necessarily represent those of their affiliated organizations, or those of the publisher, the editors and the reviewers. Any product that may be evaluated in this article, or claim that may be made by its manufacturer, is not guaranteed or endorsed by the publisher.
The author would like to acknowledge Steve Atherton, Illustrator Swansea Bay University Health Board.
1. Changing Faces. Disfigurement in the UK. (2017). Available online at: https://www.changingfaces.org.uk/wp-content/uploads/2021/05/disfigurement-in-the-uk-report-2017.pdf (accessed March 1, 2021).
2. Hurvitz KA, Kobayashi M, Evans GRD. Current options in head and neck reconstruction. Plast Reconstr Surg. (2006) 118:122e−33e. doi: 10.1097/01.prs.0000237094.58891.fb
3. Jiamei D, Jiake C, Hongxing Z, Wanhou G, Yan W, Gaifen L. An investigation of psychological profiles and risk factors in congenital microtia patients. J Plast Reconstr Aesthet Surg. (2008) 61(Suppl. 1):S37–S43. doi: 10.1016/j.bjps.2007.09.002
4. Gibson JAG Ackling E Bisson JI Dobbs TD Whitaker IS. The association of affective disorders and facial scarring: systematic review and meta-analysis. J Affect Disord. (2018) 239:1–10. doi: 10.1016/j.jad.2018.06.013
5. Johnson TM, Ratner D, Nelson BR. Soft tissue reconstruction with skin grafting. J Am Acad Dermatol. (1992) 27(2 Pt 1):151–65. doi: 10.1016/0190-9622(92)70164-B
6. Summers BK, Siegle RJ. Facial cutaneous reconstructive surgery: general aesthetic principles. J Am Acad Dermatol. (1993) 29(5 Pt 1):669–81. doi: 10.1016/0190-9622(93)70230-Q
7. Lindsay KJ, Morton JD. Flap or graft: the best of both in nasal ala reconstruction. J Plast Reconstr Aesthet Surg. (2015) 68:1352–7. doi: 10.1016/j.bjps.2015.05.036
8. Ebrahimi A, Ashayeri M, Rasouli HR. Comparison of local flaps and skin grafts to repair cheek skin defects. J Cutan Aesthet Surg. (2015) 8:92–6. doi: 10.4103/0974-2077.158444
9. Schliephake H, Neukam FW, Schmelzeisen R, Reiche C. Reconstruction of facial soft tissues after resection of skin tumours. J Craniomaxillofac Surg. (1994) 22:342–8. doi: 10.1016/S1010-5182(05)80115-5
10. Chang EI, Hanasono MM. State-of-the-art reconstruction of midface and facial deformities. J Surg Oncol. (2016) 113:962–70. doi: 10.1002/jso.24150
11. Futran ND, Wadsworth JT, Villaret D, Farwell DG. Midface reconstruction with the fibula free flap. Arch Otolaryngol Head Neck Surg. (2002) 128:161–6. doi: 10.1001/archotol.128.2.161
12. Hartman EHM, Spauwen PHM, Jansen JA. Donor site complications in vascularized bone flap surgery. J Invest Surg. (2002) 15:185–97. doi: 10.1080/08941930290085967
13. Lovice DB, Mingrone MD, Toriumi DM. Grafts and implants in rhinoplasty and nasal reconstruction. Otolaryngol Clin North Am. (1999) 32:113–41. doi: 10.1016/S0030-6665(05)70118-3
14. Cronin TD. Use of a silastic frame for total and subtotal reconstruction of the external ear: preliminary report. Plast Reconstr Surg. (1966) 37:399–405. doi: 10.1097/00006534-196605000-00003
15. Firmin F. Ear reconstruction in cases of typical microtia. Personal experience based on 352 microtic ear corrections. Scand J Plast Reconstr Surg Hand Surg. (1998) 32:35–47. doi: 10.1080/02844319850158930
16. Jessop ZM, Javed M, Otto IA, Combellack EJ, Morgan S, Breugem CC, et al. Combining regenerative medicine strategies to provide durable reconstructive options: auricular cartilage tissue engineering. Stem Cell Res Ther. (2016) 7:19. doi: 10.1186/s13287-015-0273-0
17. Jessop ZM, Al-Sabah A, Gardiner MD, Combellack E, Hawkins K, Whitaker IS. 3D bioprinting for reconstructive surgery: principles, applications + challenges. J Plast Reconstr Aesthet Surg. (2017) 70: 1155–70. doi: 10.1016/j.bjps.2017.06.001
18. Myres JL. The Edwin Smith surgical papyrus. Antiquity. (1933) 7:244–46. doi: 10.1017/S0003598X00008073
19. Nichter LS, Morgan RF, Nichter MA. The impact of Indian methods for total nasal reconstruction. Clin Plast Surg. (1983) 10:635–41.
20. Whitaker IS Karoo RO Spyrou G Fenton OM. The birth of plastic surgery: the story of nasal reconstruction from the Edwin Smith Papyrus to the twenty-first century. Plast Reconstr Surg. (2007) 120:327–36. doi: 10.1097/01.prs.0000264445.76315.6d
21. Gillies SH. Practical uses of the tubed pedicle flap. Am J Surg. (1939) 43:201–15. doi: 10.1016/S0002-9610(39)90840-8
22. Steffensen WH. Comments on total reconstruction of the ear. Plast Reconstr Surg. (1952) 10:186–90. doi: 10.1097/00006534-195207000-00002
23. Donald PJ. Cartilage grafting in facial reconstruction with special consideration of irradiated grafts. Laryngoscope. (1986) 96:786–807. doi: 10.1288/00005537-198607000-00015
24. Welling DB, Maves MD, Schuller DE, Bardach J. Irradiated homologous cartilage grafts. Long-term results. Arch Otolaryngol Head Neck Surg. (1988) 114:291–5. doi: 10.1001/archotol.1988.01860150073018
25. Amodeo CA. The central role of the nose in the face and the psyche: review of the nose and the psyche. Aesthetic Plast Surg. (2007) 31:406–10. doi: 10.1007/s00266-006-0241-2
26. Nabadalung DP. Prosthetic rehabilitation of a total rhinectomy patient resulting from squamous cell carcinoma of the nasal septum: a clinical report. J Prosthet Dent. (2003) 89:234–8. doi: 10.1067/mpr.2003.45
27. Matarasso A, Elias AC, Elias RL. Labial incompetence: a marker for progressive bone resorption in silastic chin augmentation: an update. Plast Reconstr Surg. (2003) 112:676–8; discussion 679–80. doi: 10.1097/01.PRS.0000070941.97090.9E
28. Tanzer RC. Total reconstruction of the external ear. Plast Reconstr Surg. (1959) 23:1–15. doi: 10.1097/00006534-195901000-00001
29. Brent B. The correction of microtia with autogenous cartilage grafts: I. The classic deformity? Plast Reconstr Surg. (1980) 66:1–12. doi: 10.1097/00006534-198007000-00003
30. Park C, Lee TJ, Shin KS, Kim YW. A single-stage two-flap method of total ear reconstruction. Plast Reconstr Surg. (1991) 88:404–12. doi: 10.1097/00006534-199109000-00004
31. Nagata S. A new method of total reconstruction of the auricle for microtia. Plast Reconstr Surg. (1993) 92:187–201. doi: 10.1097/00006534-199308000-00001
32. Tardy ME Jr, Denneny J, Fritsch MH. The versatile cartilage autograft in reconstruction of the nose and face. Laryngoscope. (1984) 95:523–33. doi: 10.1288/00005537-198505000-00003
33. Nimeskern L, Feldmann EM, Kuo W, Schwarz S, Goldberg-Bockhorn E, Durr S, et al. Magnetic resonance imaging of the ear for patient-specific reconstructive surgery. PLoS One. (2014) 9:e104975. doi: 10.1371/journal.pone.0104975
34. Chen ZC, Chen PKT, Hung KF, Lo LJ, Chen YR. Microtia reconstruction with adjuvant 3-dimensional template model. Ann Plast Surg. (2004) 53:282–7. doi: 10.1097/01.sap.0000106434.69246.29
35. Buncke HJ Jr, Schulz WP. Total ear reimplantation in the rabbit utilising microminiature vascular anastomoses. Br J Plast Surg. (1966) 19:15–22. doi: 10.1016/S0007-1226(66)80003-6
36. Pennington DG, Lai MF, Pelly AD. Successful replantation of a completely avulsed ear by microvascular anastomosis. Plast Reconstr Surg. (1980) 65:820–3. doi: 10.1097/00006534-198006000-00017
37. Hammond DC, Bouwense CL, Hankins WT, Maxwell-Davis GS, Furdyna J, Capraro PA. Microsurgical replantation of the amputated nose. Plast Reconstr Surg. (2000) 105:2202–16; discussion 2217–8. doi: 10.1097/00006534-200005000-00034
38. Kayikçioglu A, Karamürsel S, Keçik A. Replantation of nearly total nose amputation without venous anastomosis. Plast Reconstr Surg. (2001) 108:702–4. doi: 10.1097/00006534-200109010-00015
39. Talbi M, Stussi JD, Meley M. Microsurgical replantation of a totally amputated ear without venous repair. J Reconstr Microsurg. (2001) 17:417–20. doi: 10.1055/s-2001-16354
40. Devauchelle B, Badet L, Lengelé B, Morelon E, Testelin S, Michallet M, et al. First human face allograft: early report. Lancet. (2006) 368:203–9. doi: 10.1016/S0140-6736(06)68935-6
41. Nodzo SR, Hohman DW, Chakravarthy K. Nanotechnology: why should we care? Am J Orthop. (2015) 44:E87–8.
42. Naderi H, Martin MM, Bahrami AR. Review paper: critical issues in tissue engineering: biomaterials, cell sources, angiogenesis, and drug delivery sytems. J Biomater Appl. (2011) 26:383–417. doi: 10.1177/0885328211408946
43. Kamali P, Dean D, Skoracki R, Koolen PGL, Paul MA, Ibrahim AMS, et al. The current role of three-dimensional (3D) printing in plastic surgery. Plast Reconstr Surg. (2016) 137:1045–55. doi: 10.1097/01.prs.0000479977.37428.8e
44. Golas AR, Hernandez KA, Spector JA. Tissue engineering for plastic surgeons: a primer. Aesthetic Plast Surg. (2014) 38:207–21. doi: 10.1007/s00266-013-0255-5
45. Al-Himdani S, Jessop ZM, Al-Sabah A, Combellack E, Ibrahim A, Doak SH, et al. Tissue-engineered solutions in plastic and reconstructive surgery: principles and practice. Front Surg. (2017) 4:4. doi: 10.3389/fsurg.2017.00004
46. D'Amico RA, Rubin JP. Regenerative medicine and the future of plastic surgery. Plast Reconstr Surg. (2014) 133:958–64. doi: 10.1097/PRS.0000000000000212
47. O'Dowd A. Peers call for UK to harness “enormous” potential of regenerative medicine. Br Med J. (2013) 347:f4248. doi: 10.1136/bmj.f4248
48. Chia HN, Wu BM. Recent advances in 3D printing of biomaterials. J Biol Eng. (2015) 9:4. doi: 10.1186/s13036-015-0001-4
49. Oseni AO, Butler PE, Seifalian AM. Optimization of chondrocyte isolation and characterization for large-scale cartilage tissue engineering. J Surg Res. (2013) 181:41–8. doi: 10.1016/j.jss.2012.05.087
51. Grellmann W, Berghaus A, Haberland EJ, Jamali Y, Holweg K, Reincke K, et al. Determination of strength and deformation behaviour of human cartilage for the definition of significant parameters. J Biomed Mater Res A. (2006) 78:168–74. doi: 10.1002/jbm.a.30625
52. Split-line orientation of the talar dome articular cartilage. Arthroscopy. (2005) 21:570–3. doi: 10.1016/j.arthro.2005.01.010
53. Richmon JD, Sage A, Wong WV, Chen AC, Sah RL, Watson D. Compressive biomechanical properties of human nasal septal cartilage. Am J Rhinol. (2006) 20:496–501. doi: 10.2500/ajr.2006.20.2932
54. Xia Y, Zheng S, Szarko M, Lee J. Anisotropic properties of bovine nasal cartilage. Microsc Res Tech. (2012) 75:300–6. doi: 10.1002/jemt.21058
55. Jessop ZM, Zhang Y, Simoes IN, Al-Sabah A, Badiei N, Gazze SA, et al. Morphological and biomechanical characterization of immature and mature nasoeptal cartilage. Sci Rep. (2019) 9:12464. doi: 10.1038/s41598-019-48578-3
56. Cao Y, Vacanti JP, Paige KT, Upton J, Vacanti CA. Transplantation of chondrocytes utilizing a polymer-cell construct to produce tissue-engineered cartilage in the shape of a human ear. Plast Reconstr Surg. (1997) 100:297–302; discussion 303–4. doi: 10.1097/00006534-199708000-00001
57. Christophel JJ, Chang JS, Park SS. Transplanted tissue-engineered cartilage. Arch Facial Plast Surg. (2006) 8:117–22. doi: 10.1001/archfaci.8.2.117
58. Kafienah WE, Jakob M, Dèmarteau O, Frazer A, Barker MD, Martin I, et al. Three-dimensional tissue engineering of hyaline cartilage: comparison of adult nasal and articular chondrocytes. Tissue Eng. (2002) 8:817–26. doi: 10.1089/10763270260424178
59. Chung C, Mesa J, Miller GJ, Randolph MA, Gill TJ, Burdick JA. Effects of auricular chondrocyte expansion on neocartilage formation in photocrosslinked hyaluronic acid networks. Tissue Eng. (2006) 12:2665–73. doi: 10.1089/ten.2006.12.2665
60. Xu Y, Fan F, Kang N, Wang S, You J, Wang H, et al. Tissue engineering of human nasal alar cartilage precisely by using three-dimensional printing. Plast Reconstr Surg. (2015) 135:451–8. doi: 10.1097/PRS.0000000000000856
61. Pomerantseva I, Bichara DA, Tseng A. Ear-shaped stable auricular cartilage engineered from extensively expanded chondrocytes in an immunocompetent experimental animal model. Tissue Eng Part A. (2016) 22:197–207. doi: 10.1089/ten.tea.2015.0173
62. Bichara DA, Pomerantseva I, Zhao X, Zhou L, Kulig KM, Tseng A, et al. Successful creation of tissue-engineered autologous auricular cartilage in an immunocompetent large animal model. Tissue Eng Part A. (2014) 20:303–12. doi: 10.1089/ten.tea.2013.0150
63. Sterodimas A, De Faria J. Human auricular tissue engineering in an immunocompetent animal model. Aesthet Surg J. (2013) 33:283–9. doi: 10.1177/1090820X12472902
64. Shieh SJ, Terada S, Vacanti JP. Tissue engineering auricular reconstruction: in vitro and in vivo studies. Biomaterials. (2004) 25:1545–57. doi: 10.1016/S0142-9612(03)00501-5
65. Bichara DA, O'Sullivan NA, Pomerantseva I, Zhao X, Sundback CA, Vacanti JP, et al. The tissue-engineered auricle: past, present, and future. Tissue Eng Part B Rev. (2012) 18:51–61. doi: 10.1089/ten.teb.2011.0326
66. Jungebluth P, Alici E, Baiguera S, Blomberg P, Bozoky B, Crowley C, et al. Tracheobronchial transplantation with a stem-cell-seeded bioartificial nanocomposite: a proof-of-concept study. Lancet. (2011) 378:1997–2004. doi: 10.1016/S0140-6736(11)61715-7
67. Oseni A, Crowley C, Lowdell M, Birchall M, Butler PE, Seifalian AM. Advancing nasal reconstructive surgery: the application of tissue engineering technology. J Tissue Eng Regen Med. (2012) 6:757–68. doi: 10.1002/term.487
68. Zhou G, Jiang H, Yin Z, Liu Y, Zhang Q, Zhang C, et al. In vitro regeneration of patient-specific ear-shaped cartilage and its first clinical application for auricular reconstruction. EBioMed. (2018) 28:287–302. doi: 10.1016/j.ebiom.2018.01.011
69. Yanaga H, Imai K, Fujimoto T, Yanaga K. Generating ears from cultured autologous auricular chondrocytes by using two-stage implantation in treatment of microtia. Plast Reconstr Surg. (2009) 124:817–25. doi: 10.1097/PRS.0b013e3181b17c0e
70. Yanaga H, Imai K, Tanaka Y, Yanaga K. Two-stage transplantation of cell-engineered autologous auricular chondrocytes to regenerate chondrofat composite tissue: clinical application in regenerative surgery. Plast Reconstr Surg. (2013) 132:1467–77. doi: 10.1097/01.prs.0000434408.32594.52
71. Fulco I, Miot S, Haug MD, Barbero A, Wixmerten A, Feliciano S, et al. Engineered autologous cartilage tissue for nasal reconstruction after tumour resection: an observational first-in-human trial. Lancet. (2014) 384:337–46. doi: 10.1016/S0140-6736(14)60544-4
72. Sachs PC, Mollica PA, Bruno RD. Tissue specific microenvironments: a key tool for tissue engineering and regenerative medicine. J Biol Eng. (2017) 11:34. doi: 10.1186/s13036-017-0077-0
73. Peterson L, Minas T, Brittberg M, Nilsson A, Sjogren-Jansson E, Lindahl A. Two- to 9-year outcome after autologous chondrocyte transplantation of the knee. Clin Orthop Relat Res. (2000) 374:212–34. doi: 10.1097/00003086-200005000-00020
74. Reiffel AJ, Kafka C, Hernandez KA, Popa S, Perez JL, Zhou S, et al. High-fidelity tissue engineering of patient-specific auricles for reconstruction of pediatric microtia and other auricular deformities. PLoS One. (2013) 8:e056506. doi: 10.1371/journal.pone.0056506
75. Otto IA, Levato R, Webb WR, Khan IM, Breugem CC, Malda J. Progenitor cells in auricular cartilage demonstrate cartilage-forming capacity in 3D hydrogel culture. Eur Cell Mater. (2018) 35:132–50. doi: 10.22203/eCM.v035a10
76. Farhadi J, Fulco I, Miot S, Wirz D, Haug M, Dickinson SC, et al. Precultivation of engineered human nasal cartilage enhances the mechanical properties relevant for use in facial reconstructive surgery. Ann Surg. (2006) 244:978–58. doi: 10.1097/01.sla.0000247057.16710.be
77. Cleary MA, Osch GJVMV, Brama PA, Hellingman CA, Narcisi R. FGF, TGFβ and Wnt crosstalk: embryonic to in vitro cartilage development from mesenchymal stem cells. J Tissue Eng Regen Med. (2015) 9:332–42. doi: 10.1002/term.1744
78. Jessop ZM, Al-Sabah A, Simoes IN, Burnell SEA, Pieper IL, Thornton CA, et al. Isolation and characterisation of nasoseptal cartilage stem/progenitor cells and their role in the chondrogenic niche. Stem Cell Res Ther. (2020) 11:177. doi: 10.1186/s13287-020-01663-1
79. Jessop ZM Manivannan S Zhang Y Thornton CA Narayan R Whitaker IS. Tissue specific stem/progenitor cells for cartilage tissue engineering: a systematic review of the literature. Appl Phys Rev. (2019) 6:031301. doi: 10.1063/1.5050814
Keywords: facial reconstruction, cartilage, tissue engineering, regenerative medicine, plastic and reconstructive surgery
Citation: Jessop ZM, Hague A, Dobbs TD, Stewart KJ and Whitaker IS (2021) Facial Cartilaginous Reconstruction—A Historical Perspective, State-of-the-Art, and Future Directions. Front. Surg. 8:680186. doi: 10.3389/fsurg.2021.680186
Received: 13 March 2021; Accepted: 19 July 2021;
Published: 16 August 2021.
Edited by:
Jason K. F. Wong, The University of Manchester, United KingdomReviewed by:
Fatih Zor, Wake Forest School of Medicine, United StatesCopyright © 2021 Jessop, Hague, Dobbs, Stewart and Whitaker. This is an open-access article distributed under the terms of the Creative Commons Attribution License (CC BY). The use, distribution or reproduction in other forums is permitted, provided the original author(s) and the copyright owner(s) are credited and that the original publication in this journal is cited, in accordance with accepted academic practice. No use, distribution or reproduction is permitted which does not comply with these terms.
*Correspondence: Iain S. Whitaker, aWFpbndoaXRha2VyQGZhc3RtYWlsLmZt
Disclaimer: All claims expressed in this article are solely those of the authors and do not necessarily represent those of their affiliated organizations, or those of the publisher, the editors and the reviewers. Any product that may be evaluated in this article or claim that may be made by its manufacturer is not guaranteed or endorsed by the publisher.
Research integrity at Frontiers
Learn more about the work of our research integrity team to safeguard the quality of each article we publish.