- 1ARTORG Center for Biomedical Engineering, University of Bern, Bern, Switzerland
- 2Department of Otorhinolaryngology, Head and Neck Surgery, Inselspital, University Hospital Bern, Bern, Switzerland
- 3Department of Otorhinolaryngology, University Hospital Düsseldorf, Düsseldorf, Germany
Objective: Despite three decades of pre-clinical and clinical research into image guidance solutions as a more accurate and less invasive alternative for instrument and anatomy localization, translation into routine clinical practice for surgery in the lateral skull has not yet happened. The aim of this review is to identify challenges that need to be solved in order to provide image guidance solutions that are safe and beneficial for use during lateral skull surgery and to synthesize factors that facilitate the development of such solutions.
Methods: Literature search was conducted via PubMed using terms relating to image guidance and the lateral skull. Data extraction included the following variables: image guidance error, imaging resolution, image guidance system, tracking technology, registration method, study endpoints, clinical target application, and publication year. A subsequent search of FDA 510(k) database for identified image guidance systems and extraction of the year of approval, intended use, and indications for use was performed. The study objectives and endpoints were subdivided in three time phases and summarized. Furthermore, it was analyzed which factors correlated with the image guidance error. Factor values for which an error ≤0.5 mm (μerror + 3σerror) was measured in more than one study were identified and inspected for time trends.
Results: A descriptive statistics-based summary of study objectives and findings separated in three time intervals is provided. The literature provides qualitative and quantitative evidence that image guidance systems must provide an accuracy ≤0.5 mm (μerror + 3σerror) for their safe and beneficial application during surgery in the lateral skull. Spatial tracking accuracy and precision and medical image resolution both correlate with the image guidance accuracy, and all of them improved over the years. Tracking technology with accuracy ≤0.05 mm, computed tomography imaging with slice thickness ≤0.2 mm, and registration based on bone-anchored titanium fiducials are components that provide a sufficient setting for the development of sufficiently accurate image guidance.
Conclusion: Image guidance systems must reliably provide an accuracy ≤0.5 mm (μerror + 3σerror) for their safe and beneficial use during surgery in the lateral skull. Advances in tracking and imaging technology contribute to the improvement of accuracy, eventually enabling the development and wide-scale adoption of image guidance solutions that can be used safely and beneficially during lateral skull surgery.
Introduction
Microsurgical procedures in the lateral skull present a challenge for surgeons. The geometric scale of the anatomical structures in the lateral skull in the submillimeter range (1) requires surgeons to work at the limits of their visual and tactile capabilities. Instrument and anatomy localization are at the expense of invasiveness caused by exposing structures to be preserved and their use as orientation landmarks. In the absence of more precise and less invasive alternatives, surgery of lesions poses a considerable risk of iatrogenic morbidity (2, 3). Therefore, particularly in cases of benign tumors, the preservation of function and anatomical structures is often prioritized over surgical radicality. Consequentially and in combination with uncertainty in anatomy localization, pathological structures cannot be sufficiently exposed, contributing to high recurrence rates, for example, in cholesteatoma surgery (4).
Image guidance constitutes a technological solution for ensuring accurate anatomy and instrument localization. It drives the limits of surgery beyond what is possible by human visual and tactile perception alone, potentially improving the efficacy and the safety of procedures. Its value has pushed these systems into routine clinical practice in various medical fields such as orthopedics (5), neurosurgery (6), radio surgery (7), interventional oncology (8), and rhinology (9). Nonetheless, more than three decades after the introduction of frameless stereotaxy and numerous research applications, the technology is not routinely applied in lateral skull surgery.
The aim of this review is to identify challenges that need to be solved in order to provide image guidance solutions safe and beneficial for use during lateral skull surgery and to synthesize factors that facilitate the development of such solutions.
Methods
Search Strategy
Medical subject headings (MeSH) were used for electronic database searches from January 1, 1989 up to March 31, 2020. The search was conducted in English in MEDLINE and the COCHRANE Library with MeSH terms relating to image guidance (“Surgery, Computer-Assisted,” “Neuronavigation,” “Stereotaxic Techniques”) and the lateral skull (“Cranial Fossa, Middle,” “Cranial Fossa, Posterior,” “Temporal Bone,” “Otolaryngology”). The exact search term can be found in the attachment.
Selection of Studies and Eligibility Criteria
A total of 296 articles were found. Subsequent exclusion of non-English-language articles to enable international verifiability, theoretical or technical evaluations, frame-based neurosurgery applications, non-lateral skull applications, applications where the system was purely used as distance measurement tool, image processing, segmentation, and surgical planning applications, articles about image-based treatment validation, duplicate articles, and review articles, letters, or editorials (Figure 1) resulted in 81 articles included in this review.
Data Collection
For each article, the image guidance error (mean, standard deviation, error definition, and measurement method), imaging modality and slice thickness, tracking modality, tracking system manufacturer and model, instrument controller modality, image guidance system manufacturer and model, registration method, sample size, study objective, study endpoints, clinical target application and publication year, and other metadata were identified (Figure 1).
If the guidance error was reported using mean and maximum measured values, the reported maximum error was assumed to have happened with a probability of 0.5%; thus, the standard deviation was calculated as σ = (maximum value − μ)/2.5. For articles that did not report mean and standard deviation values but provided the measured errors, mean and standard deviation values were calculated. For articles that compared errors, the lowest error (μerror + 3σerror) was considered. The error definitions were categorized into three- and two-dimensional position errors.
The tracking modalities were grouped as optical, electromagnetic, mechanical, acoustic, and no tracking. Instrument controlling modalities were grouped as freehand-controlled by the surgeon, robot-controlled, controlled through a stereotactic frame, controlled through a robotic stereotactic frame, and freehand-controlled drill by a surgeon with automatic on/off controller.
The clinical target applications were grouped into categories of cochlear implant surgery, internal auditory canal surgery, and general surgery in the lateral skull if otherwise.
The registration methods were grouped as anatomical landmark, skin-affixed fiducial, bone-anchored fiducial, skin surface-matching, bone surface-matching, dental bite block, template-assisted, and precalibrated imaging device registration.
For each identified image guidance system, the year of the first market approval (CE mark or FDA approval), the intended use, and the indications for use were identified by searching the FDA 510k, DeNovo, and PMA databases (10), which store information about cleared devices, on the official websites of the manufacturers or in press releases (Figure 1). For each system, the target market was identified by checking the marketing on the official webpage and grouped as “Lateral Skull” if the marketing targeted ear or lateral skull surgery and “Other” if otherwise.
Data Analysis
To provide an overview of the research since 1989, market approvals and clinical studies were depicted in a timeline from 1989 to 2020. The study objectives and the endpoints were subdivided in three time phases and summarized.
The identified image guidance systems, tracking modalities and systems, controlling modalities, registration methods, clinical target applications, error measurement methods, and slice thickness values were visually assessed for differences in the image guidance error and values identified that were used in applications with an image guidance error ≤0.5 mm. The same factors and the image guidance error were assessed for correlation (Pearson) with the publication year, and any trends were identified. Additionally, use cases indicated in the indications for use and intended uses of the previously identified commercial image guidance systems were counted and consolidated into anatomical regions, and the respective proportions were calculated. Analysis was conducted in R (11), and the figures were produced using the package ggplot2 (12).
Results
An overview of research conducted since 1989 highlighting the main findings is presented in the next three sections and in Figure 2. In the subsequent section, factors that allow the development of image guidance solutions that are safe and beneficial for use during surgery in the lateral skull are presented. In this context, image guidance components associated with low image guidance error and respective temporal evolution are presented. All included articles and extracted values can be found in the attachment in the form of a table and linked figures.
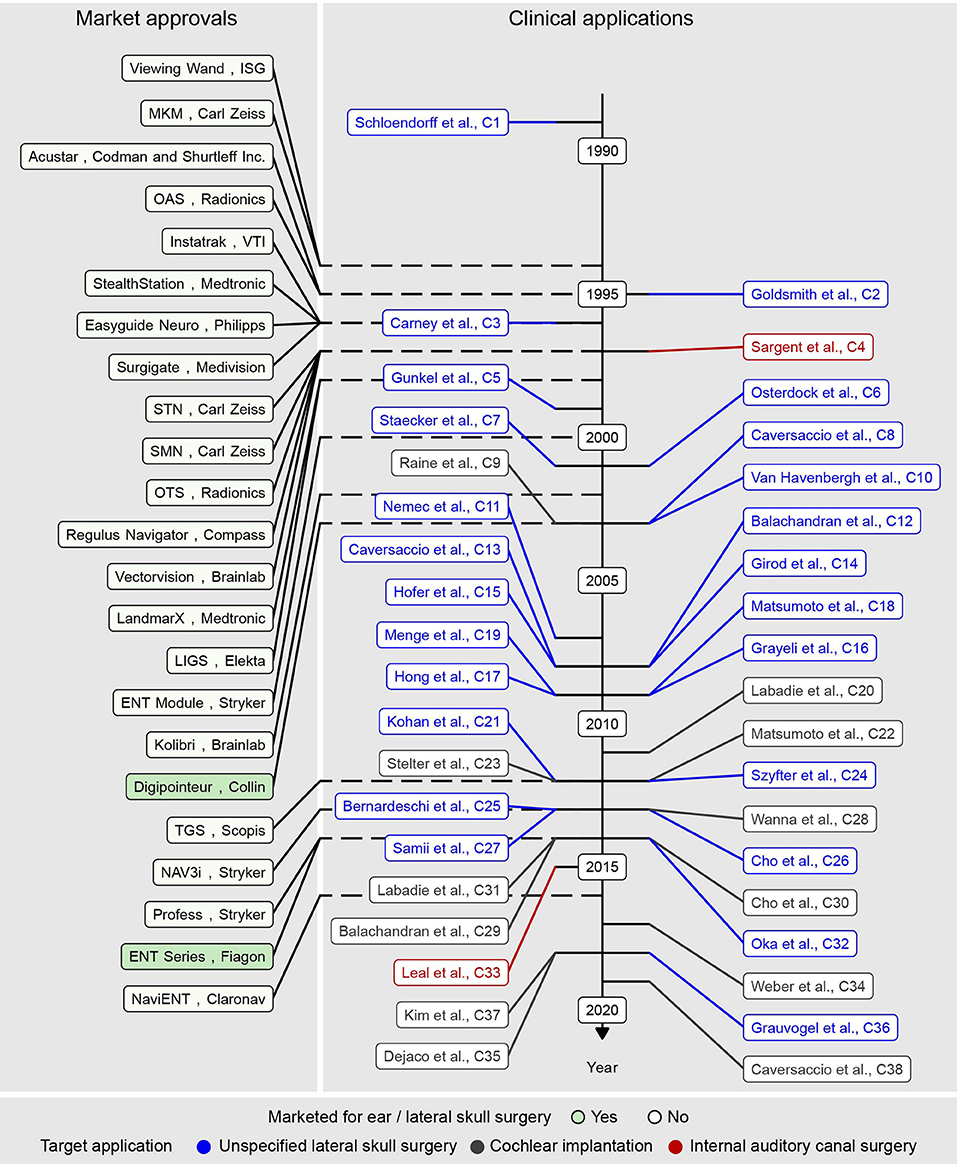
Figure 2. Market approval year of commercial image guidance systems (left) and clinical studies (middle, right) included in the present literature review in chronological order.
1989–2000: Identification of Clinical Use Cases and Envisionment of Positive Effects on Clinical Outcomes
The use of image guidance in lateral skull surgery was reported for the first time in 1989 by Schlöndorff et al. (13). A mechanical arm for spatial tracking, computed tomography (CT, slice thickness: 2 mm), and skin-affixed fiducial registration enabled the visualization of the arm-attached stylus location in the CT images on a computer (13). The system was applied in more than 100 ear, nose, and throat applications. Sinus, skull base, and tumor surgery were subjectively identified as applications that benefit from image guidance (14).
Early clinical image guidance applications in lateral skull surgery discussed the value of image guidance for different surgical applications. The technology was, albeit without significant evidence, reported as useful for the spatial identification of anatomy and pathology during surgical access creation to the petrous apex (15, 16) and the internal auditory canal (15–17), resection of (pseudo)neoplasms (15, 16, 18), and revision surgery (16). Reduced invasiveness (15–17) and iatrogenic morbidity (15–17) and increased efficacy (15, 16) of surgical treatments were envisioned. Advantages of non-over-mechanically linked systems (17), advantages of using a skull-attached patient tracker over patient fixation with a Mayfield clamp (16), advantages and disadvantages of different registration methods (19, 20), and adequately tracked instrumentation (15, 16) were also discussed. The routine applicability of available systems for microsurgery in the lateral skull was demonstrated (19).
By the year 2000, 16 image guidance systems from 11 companies were commercially available and cleared through the premarket notification [510(k)] process, stating their substantial equivalence to legally marketed predicate devices (Figure 2). Eleven, three, and two systems used infrared-based optical, mechanical, and electromagnetic instrument tracking, respectively. Among the optical tracking-based systems, nine systems used a passive Polaris camera (NDI, Canada), and the other two used an active Optotrak (NDI, Canada) and an active Stryker (Kalamazoo, USA) proprietary camera, respectively.
2000–2010: Technological Developments Without Clinical Progress
The clinical availability of image guidance systems resulted in multiple clinical studies and case reports investigating the clinical effects of image guidance. Despite the high expectations, no significant effect has yet been proven. Clinical applications reported few to no complications (21–25), complete tumor resection (23, 25), no tumor recurrence (25, 26), good audiological outcomes (21, 22), low number of required puncture attempts in otogenic brain abscess surgery (27), higher security and less stress for the surgeon (21, 22), and favorable reconstruction of the external ear canal (21), however without comparison to non-image-guided surgery. A decrease (16, 21) as well as an increase (26) in operating time was measured. Furthermore, image guidance was used to control the surgical burr motor and automatically stop the rotation in case of critical proximity to structures to be preserved; however, the anticipated decrease of complications during temporal bone drilling could not be proven (28). The status quo was similar to that of 10 years before: image guidance was reported as useful for identifying anatomy and pathology in pseudo(neoplasm) resection (22, 23, 25), cochlear implantation (24, 29), congenital aural atresia (21), and auricular implant (30) surgery, but no significant positive effects were demonstrated.
While sinus (9) and neurosurgery (6) benefited from the routine application of image guidance by 2010, the systems remained left out of routine clinical use in lateral skull surgery.
As between 2000 and 2010 the gap between promised effects and clinical reality became evident, an accuracy of commercially available systems of 1–3 mm (μerror + 3σerror) was measured in independent studies (25, 31–34), and insufficient image guidance accuracy was identified as a reason (35–38). High costs in terms of additional radiation (29, 39), time consumption (26), and surgical invasiveness (40) contributing to the unfavorable cost–benefit ratio were also identified as decisive factors for driving the absence of these systems during lateral skull surgery. Even though the reduction of costs via non-invasive and quicker registration methods (40–42) was successful, the continued lack of accuracy limited operators from exploiting the benefits of image guidance in clinics.
2010–2020: Stagnation of Commercial Development, the Use Case of Cochlear Implantation, and the Fight for Microns
Between 2010 and 2020, the commercial development of image guidance for lateral skull surgery stagnated. During this period, five systems entered the market (Figure 2), including two systems from manufacturers who already invested in the market with other systems. All 23 systems identified in this study were cleared through the 510(k) route, indicating substantial equivalence to predicate devices. Overall, the available image guidance systems were and still are primarily developed and indicated for nose, anterior skull base, and neurosurgery (Figure 3).
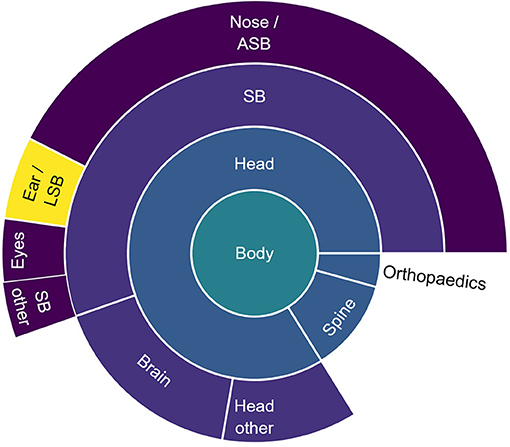
Figure 3. Proportion of the number of use cases for freehand image guidance per anatomical region. The use cases were extracted from the indications for use of commercial freehand image guidance systems used in the studies included in this literature review and were categorized into anatomical regions. SB, skull base; ASB, anterior skull base; LSB, latera skull base.
Between 2010 and 2020, a large portion of research (25/50 included articles) focused on image-guided cochlear implantation. Image guidance technology conceptually enables the creation of a keyhole access tunnel from the mastoid surface to the middle and the inner ear which can be used for cochlear implantation, thus reducing the volume of bone removed. The practicability of creating a keyhole access route (diameter ~2 mm) to the middle ear with a freehand-controlled drill and relying on image guidance was investigated in preclinical experiments (43, 44) and found to be difficult and dangerous (45). Middle ear access creation for cochlear implantation requires drilling of bone between the facial nerve and chorda tympani [in-between distance: ~2.5 mm (46)]. To overcome human limitations in dexterity in creating keyhole access routes (45), the use of image-guided drill guides (45) (microstereotactic frames) and robotic manipulators (47, 48) was investigated. However, in preclinical studies, the lack of accuracy of both led to unacceptable rates of damage to the facial nerve, the chorda tympani, the ear canal wall, and the ossicles (47, 48). Empirical accuracy requirements of 0.5 mm (38) and 1 mm (24, 43) for safe application in cochlear implantation were discussed. However, it was only until recently and quantitative analysis (46) that the research community and medical device manufacturers started understanding the stringent maximum error requirements of ~≤0.5 mm (μerror + 3σerror) needed for a safe, purely image-guided middle ear access creation in a large portion of the population with available and suitable instrumentation (drill diameter: 1–2 mm). Means to increase the accuracy of image guidance systems were investigated (49–54) with successful results (49, 50, 53) (Figure 4). Reliably accurate image guidance in combination with additional safety measures (55) has since enabled the successful clinical realization of the middle ear keyhole access (diameter: ~2 mm) using micro-stereotactic frames (56) and an image-guided robot (57) in seven of nine and six of nine patients, respectively. During the study with microstereotactic frames, one case was converted to conventional surgery and one case suffered from facial paresis (56). During the study with an image-guided robot, three cases were converted to conventional surgery (57). Safe operations and successful implantations in all patients but one demonstrated the safety and the feasibility of cochlear implantation through the associated keyhole approach. The clinical feasibility of this novel image-guided approach and the inherent benefits of reduced invasiveness, which cannot be exploited without image guidance, confirmed cochlear implantation as a use case for image guidance. However, the surgery time in these studies was substantially higher than in conventional surgery. Furthermore, the treatment caused additional radiation exposure to the patients and required additional personnel in the operating room (56, 57). Nevertheless, due to the promising clinical results and the potential benefits of the technology, a new market that has been targeted by multiple start-up companies (OtoJig, Germany; CAScination, Switzerland; Eindhoven Medical Robotics, Netherlands) has emerged.
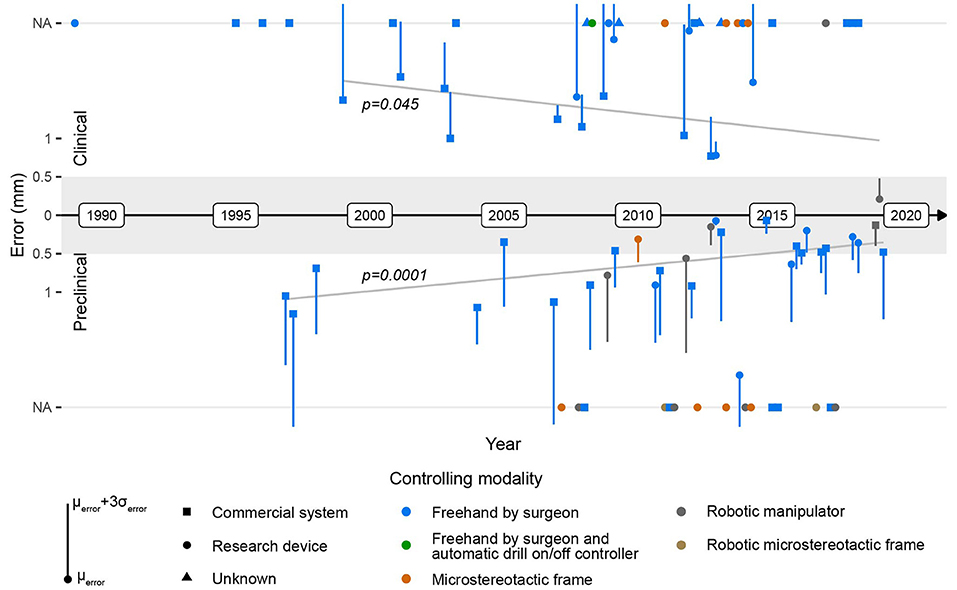
Figure 4. Evolution of the image guidance error in clinical (top) and preclinical cadaver (bottom) applications. The gray area depicts an error range ≤0.5 mm, and the gray lines depict the error trendlines. NA, value not available/reported.
Similar to those in previous decades, clinical applications of freehand image guidance in lateral skull procedures have suggested potential usefulness in otologic, petrous apex, and internal auditory canal surgery (58–61). Preclinical research has focused on finding accurate yet non-invasive (53, 54, 62) and automatic (42, 63) registration strategies, developing less-invasive access routes to the petrous apex and internal auditory canal (64–70) and assessing the accuracy of available technology (71, 72). The persistent lack of proven effects and absence in routine use indicate, however, that the clinically available systems still do not meet the needs for lateral skull surgery. State-of-the-art image guidance technology and research systems have reached an accuracy level (μerror + 3σerror ≤0.5 mm) that has been reported in the literature as a threshold for useful image guidance in the latera skull base. Commercialization of the technology is underway, paving the way for it to potentially become available to patients.
Factors Facilitating the Development of Safe and Beneficial Image Guidance for Lateral Skull Surgery
The intended use of freehand image guidance systems is to precisely locate anatomical structures in surgery and, with this, potentially increase the safety and the efficacy of surgery. Insufficient accuracy of image guidance systems has been identified (35–38) as a decisive reason for their poor performance during lateral skull surgery and the consequential absence of positive effects on surgical safety and efficacy. The literature provides qualitative (38) and quantitative (46) evidence that an image guidance accuracy ≤0.5 mm (μerror + 3σerror) is required for the safe and beneficial application of such technology in lateral skull surgery. Although the image guidance error continuously decreases over time (significant correlation, Pearson coefficients for clinical data: p = 0.045; preclinical data: p = 0.0001; combined: p = 4.1e-6), to date no commercial image guidance system that meets this requirement exists (Figure 4). However, during the last decade, a few research devices with an image guidance error ≤0.5 mm (μerror + 3σerror) have been successfully developed, and their functionality and safety have been clinically validated, potentially suggesting their future beneficial application in lateral skull surgery (Figure 4).
To synthesize factors that allow a sufficiently accurate image-guidance, it was visually analyzed which factors allow low image-guidance error. Factor values for which an error ≤0.5 mm (μerror + 3σerror) was measured in more than one study were inspected for time correlations.
Image guidance systems for which an error ≤0.5 mm (μerror + 3σerror) was measured in more than one study conducted imaging with a slice thickness ≤0.2 mm, used optical tracking with a spatial localization accuracy and precision of ≤0.05 mm [P CamBar B1, Axios3d, Germany (73)], and used bone screw registration (Figure 5). The slice thickness of CT imaging decreased from 2 mm in 1989 to 0.1 mm in 2019 (Pearson coefficient p = 1.2e-10, Figure 6). A slice thickness ≤0.2 mm was uncommon before 2010 (2/25 studies which declared the used slice thickness). Also, until 2010, no study used optical tracking with spatial localization accuracy and precision ≤0.05 mm. The CamBar B1 optical tracking camera (Axios3D, Germany) has been marketed since 2010.
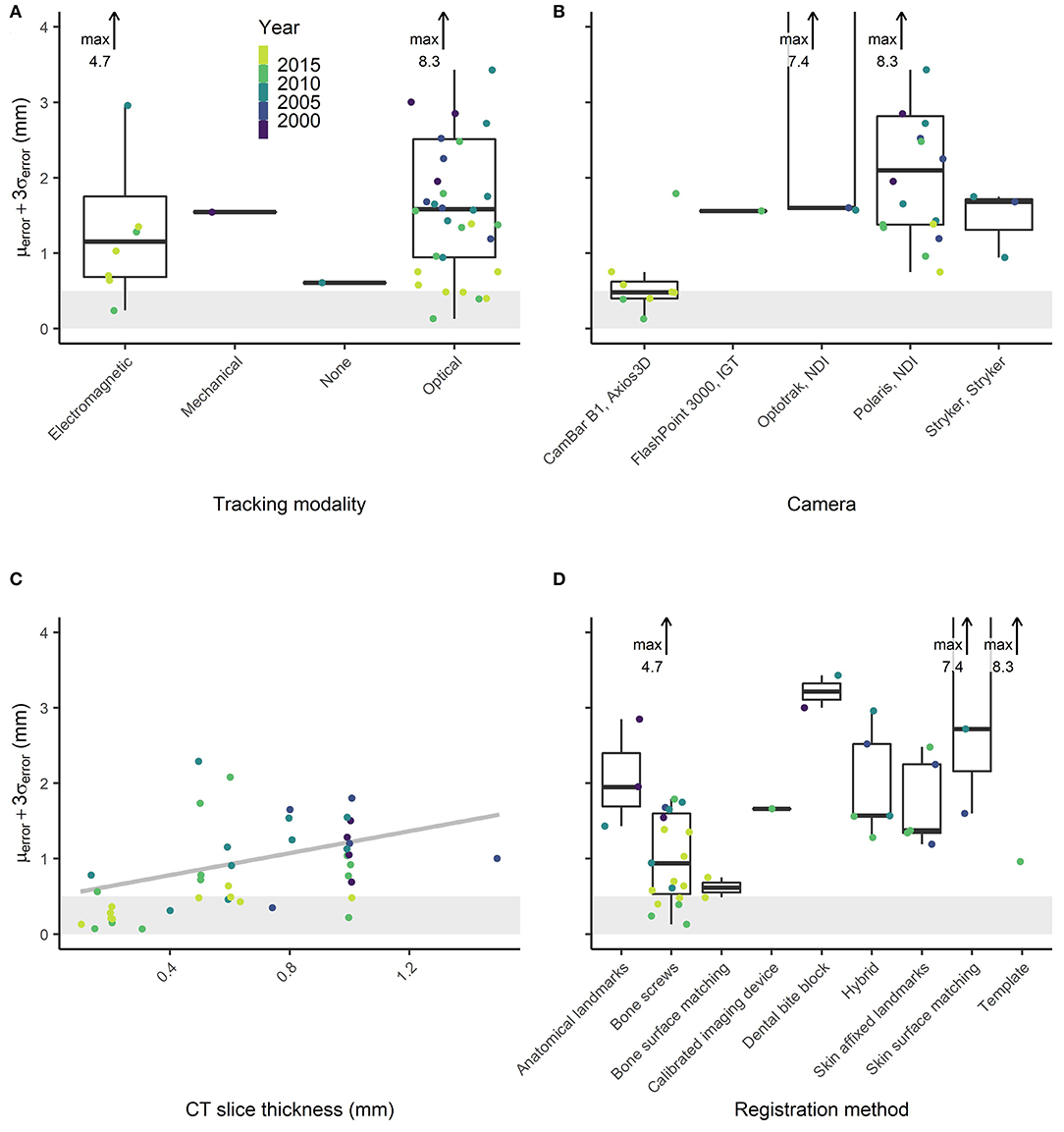
Figure 5. Reported image guidance errors for different (A) tracking modalities (none refers to stereotactic frames), (B) optical tracking cameras, (C) slice thickness values, and (D) registration methods.
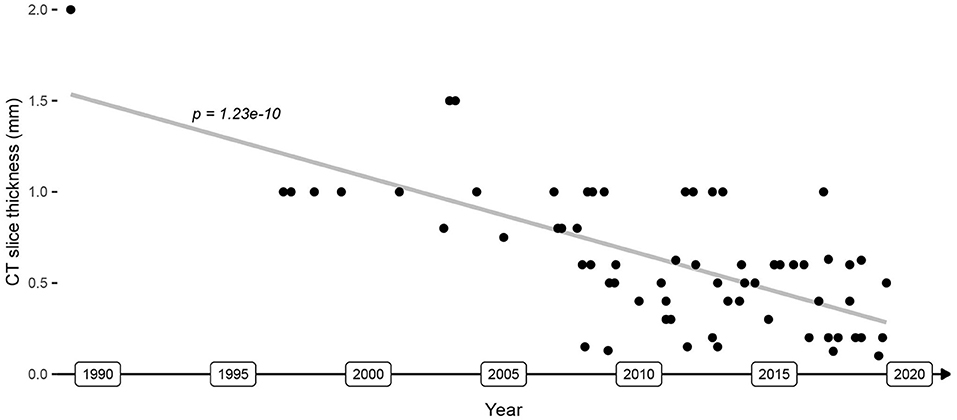
Figure 6. Evolution of CT image slice thickness used in image guidance applications. The gray line depicts the image resolution trendline.
To answer the study objective, image guidance systems must reliably provide an accuracy ≤0.5 mm (μerror + 3σerror) for their safe and beneficial use in lateral skull surgery. Instrument and patient tracking with an error ≤0.05 mm, CT imaging with a slice thickness ≤0.2 mm, and registration based on bone screws present components with which it is possible to develop clinically applicable and sufficiently accurate image guidance.
Discussion
A clinical need for a non-invasive, anatomy-independent, and accurate means of anatomy localization and instrument navigation during lateral skull surgery has been reported from the early stages of standardized surgery in the lateral skull in the late nineteenth century and continuously since then (74). Methods have been sought in vain to reduce the risk of iatrogenic injury to anatomical structures, in particular, to the facial nerve (74). Over the last three decades, work on an image guidance-based solution has been carried out under continuous clinical evaluation. However, translation into routine clinical practice has not happened yet. Herein the major findings of three decades of research are summarized. Essential factors that will facilitate the development and wide-scale adoption for surgical use of image guidance solutions that are safe and beneficial for lateral skull surgery are synthesized and presented.
Clinical Implications of the (Un)available Accuracy
The smallest and most delicate structures of the lateral skull are located in the temporal bone surrounding the labyrinth. Therefore, transmastoidal, transtympanic, and all transpetrosal access routes and surgery performed on the petrous bone embedding the internal auditory canal and labyrinth require high, yet clinically unavailable, levels of image guidance accuracy. As a consequence of the high recurrence rates after, the iatrogenic morbidity of conventional surgical treatments, and the technological progress in the domain of radiation therapy, temporal bone tumors are being irradiated with increasing frequency. Only recently the research community and medical device manufacturers have realized the stringent and technically challenging image guidance accuracy levels required to exploit the benefits of image guidance in large parts of the lateral skull. With state-of-the art imaging and tracking technology, the clinical feasibility of image guidance with suitable accuracy (μerror + 3σerror <0.5 mm) was demonstrated. Whether the ratio between the consequential benefits and the costs incurred favors, its application should be reassessed. Positive results would encourage translation of the technology into commercial systems and routine clinical practice and make it available to patients.
Transcranial access routes to the lateral skull base such as retrosigmoid or subtemporal access used to resect medium- to large-sized pathology, also extending into the brain from the internal auditory canal, clivus, or cerebellopontine angle, largely bypass the small temporal bone anatomy. As a consequence, a lower accuracy is needed to achieve the beneficial application of image guidance. Such procedures nowadays already benefit from commercially available systems, enabling the conduct of disease-tailored treatments through microscope injection of a surgical treatment plan that considers craniotomy size, access route, and target pathology. For this domain of neurosurgery, image guidance is advancing in terms of image processing algorithms, registration methods, visualization features, and usability and is therefore of increasing usefulness.
Furthermore, microsurgical procedures in the lateral skull are predominantly conducted using a microscope. Image-guided microscopic surgery requires focusing on either the microscope view or on the image guidance screen. Injection of the image guidance information into the microscope view potentially provides a solution but is subject to additional errors from microscope calibration.
To date no image guidance system can replace anatomical expertise, surgical training, or experience (16, 22, 25, 26, 31, 37).
Accuracy Requirements
The accuracy requirements for image guidance in the lateral skull have been discussed various times, and maximum values of 0.5 mm (38) and 1 mm (16, 24, 35, 36) have been reported. The population size for which, depending on system accuracy and instrument diameter, keyhole access to the middle ear is safely realizable was quantitatively analyzed. With a system accuracy of 0.39 mm (μerror + 3σerror) and a tool diameter of 1.8 mm, 47% of the adult population can be safely treated, indicating even stricter accuracy requirements (46). Similarly, the geometric size of the anatomical structures in the lateral skull (1), such as the chorda tympani diameter <0.5 mm, also indicates stricter accuracy requirements. The exact accuracy requirement values are, however, application and system specific. The accuracy requirement of 0.5 mm (μerror + 3σerror) mentioned in this article is also referred to elsewhere in the literature (38) and is, in our opinion, an upper limit, below which the application of navigation becomes safe and its usefulness may exceed the “2nd-opinion” value.
Risk of Bias in Error Definitions and Measurement Methodologies
The error of an image guidance system is defined as the spatial deviation of the instrument position reported by the system from its true position (75). The assessment of the quantitative error is susceptible to measurement bias where the measurement error corresponds to the degree of bias. To reduce the measurement bias during error assessments to a negligible level, the measurement error must be at least four times and preferably 10 times lower than the expected errors to be measured (76). For image guidance dedicated to lateral skull surgery, the measurement error that biases the measurement method must be ≲0.1 mm. To measure the image guidance error, the true spatial location of the instrument position at the time of measurement must be known. A common method (reported by 21/48 studies that declared their measurement methods) is to visually identify and manually select the position of an anatomical target landmark in the image data. Visual identification and manual selection of a landmark in the image data with a resolution of 0.1–1 mm are prone to errors, rendering this approach unsuitable for assessing the error of image guidance systems used for lateral skull surgery. As this is almost the only applicable assessment method in a clinical application [11/13 clinical applications, the other two used automatic detection of titanium target structures (34, 57)], effective error assessment is hardly possible in this case. The other 27 articles use, albeit unvalidated, more sophisticated error measurement methods. Validated means for preclinical and clinical assessments of the end-to-end error of freehand image guidance systems remain as unsolved challenges to date. Furthermore, the articles included in the review differ in terms of the definition and the measurement method of the image guidance error. The error measurement methods of the articles included in this review vary in target fiducials (24× anatomical vs. 17× artificial vs. 5× unknown fiducials), identification of the true target position in image data (31× manual vs. 10× automatic vs. 5× unknown identification), and spatial deviation measurement (8× two-dimensional vs. 26× three-dimensional vs. 12× unknown error definition).
While the reported error may be sensible for the respective applications, due to different error definitions and measurement methods, the errors must be interpreted with caution. The risk of bias of each included study is indicated in the literature table in the attachment with low (artificial target, automatic ground truth target location identification), medium (artificial target, visual/manual ground truth target location identification), and high (anatomical target, visual/manual ground truth target location identification) measurement bias color-coded in green, orange, and red shades, respectively. A consequence of high measurement bias is that the error assessment of an image guidance system yields a quantitative estimate of the measurement bias rather than the image guidance error. The measured values overestimate the true image guidance error and are of limited value to draw conclusions about the systems error or determine potential improvements due to further development of a system. Since this study reports on trends and correlations and does not derive quantitative statistical error values from the collected errors, the measurement bias inherent in the source data is acceptable.
Limitations
This literature review analyses correlations and time trends. Therefore, it is statistically impossible to imply causations. The use of adequate tracking technology, imaging protocols, and registration means does not necessarily lead to a system with sufficiently high accuracy as there might be other relevant factors that were not considered in this review, such as material and geometrical properties of the tracked instrumentation. Nevertheless, it was demonstrated (53, 55) that the use of these components allows the development of a clinically applicable system with sufficient image guidance accuracy for lateral skull surgery. The presented correlations and time trends provide evidence that the use of advanced tracking and imaging technology facilitates the development of a system with sufficient accuracy.
Surgery on the lateral skull includes a wide range of procedures. The submillimeter accuracy levels reported in the cited studies are only valid in bone and embedded structures. In soft tissue, the effective accuracy is much lower due to tissue shift. Therefore, the reported results are especially relevant for intra-temporal access routes and surgery, with access routes to and surgery in the lateral skull base being the most critical.
Conclusion
After three decades of development and clinical application, no significant positive effects of image guidance on patient outcomes or other clinically relevant parameters in lateral skull surgery have been proven. Lack of available image guidance accuracy was identified by the research community as the predominant reason. Despite the prevailing need and long-known use cases, the existing commercially available systems are neither intended nor routinely used for lateral skull surgery.
Image guidance systems must reliably provide an accuracy ≤0.5 mm (μerror + 3σerror) for their safe and beneficial use during surgery in the lateral skull. The CT slice thickness and the tracking accuracy improve continuously over time, which correlates positively with the temporal evolution of the image guidance error. The use of state-of-the-art spatial tracking with an error ≤0.05 mm, CT imaging with a slice thickness ≤0.2 mm, and bone-anchored titanium fiducials allows the development of image guidance systems with sufficient accuracy. While the translation into commercially available products and routine clinical practice has not happened yet, such technology has led to the development of image guidance systems with suitable accuracy for lateral skull surgery, and recent clinical evaluations have provided promising results, particularly in cochlear implantation.
Data Availability Statement
The original contributions presented in the study are included in the article/supplementary materials, further inquiries can be directed to the corresponding author/s.
Author Contributions
DS initiated the review, collected the data, reviewed data collected by JH and FM, conducted the data analysis, and wrote the manuscript. JH and FM assisted data collection, reviewed data collected by DS, reviewed the analysis, and reviewed the written manuscript. SW reviewed the data analysis and surveyed the literature review methodology. GB, LA, and MC reviewed the written manuscript. LN and TK contributed to the data collection, surveyed the literature review methodology, and reviewed the written manuscript. All authors contributed to the article and approved the submitted version.
Conflict of Interest
SW is cofounder, shareholder, and chief executive officer of CAScination AG (Bern, Switzerland).
The remaining authors declare that the research was conducted in the absence of any commercial or financial relationships that could be construed as a potential conflict of interest.
Supplementary Material
The Supplementary Material for this article can be found online at: https://www.frontiersin.org/articles/10.3389/fsurg.2020.604362/full#supplementary-material
References
1. Krombach GA, van den Boom M, Di Martino E, Schmitz-Rode T, Westhofen M, Prescher A, et al. Computed tomography of the inner ear: size of anatomical structures in the normal temporal bone and in the temporal bone of patients with Menière's disease. Eur Radiol. (2005) 15:1505–13. doi: 10.1007/s00330-005-2750-9
2. Betka J, Zvěrina E, Balogová Z, Profant O, Skrivan J, Kraus J, et al. Complications of microsurgery of vestibular schwannoma. Biomed Res Int. (2014) 2014:315952. doi: 10.1155/2014/315952
3. Obaid S, Nikolaidis I, Alzahrani M, Moumdjian R, Saliba I. Morbidity rate of the retrosigmoid versus translabyrinthine approach for vestibular schwannoma resection. J Audiol Otol. (2018) 22:236–43. doi: 10.7874/jao.2018.00164
4. Kerckhoffs KGP, Kommer MBJ, van Strien THL, Visscher SJA, Bruijnzeel H, Smit AL, et al. The disease recurrence rate after the canal wall up or canal wall down technique in adults. Laryngoscope. (2016) 126:980–7. doi: 10.1002/lary.25591
5. Kendoff ADP, Stueber V, Musahl VJAR. Perioperative management of unicompartmental knee arthroplasty using the MAKO robotic arm system (MAKOplasty). Am J Orthop. (2009) 38(2 Suppl.):16–9.
6. Smith JA, Jivraj J, Wong R, Yang V. 30 years of neurosurgical robots: review and trends for manipulators and associated navigational systems. Ann Biomed Eng. (2016) 44:836–46. doi: 10.1007/s10439-015-1475-4
7. Lunsford LD, Flickinger J, Lindner G, Maitz A. Stereotactic radiosurgery of the brain using the first United States 201 cobalt-60 source gamma knife. Neurosurgery. (1989) 24:151–9. doi: 10.1227/00006123-198902000-00001
8. Koethe Y, Xu S, Velusamy G, Wood BJ, Venkatesan AM. Accuracy and efficacy of percutaneous biopsy and ablation using robotic assistance under computed tomography guidance: a phantom study. Eur Radiol. (2014) 24:723–30. doi: 10.1007/s00330-013-3056-y
9. Oakley GM, Barham HP, Harvey RJ. Utility of image-guidance in frontal sinus surgery. Otolaryngol Clin North America. (2016) 49:975–88. doi: 10.1016/j.otc.2016.03.021
10. FDA. Medical Device Databases. FDA. (2020). Available online at: https://www.fda.gov/medical-devices/device-advice-comprehensive-regulatory-assistance/medical-device-databases (accessed June 29, 2020).
11. R Core Team. R: A Language and Environment for Statistical Computing. Vienna: R Foundation for Statistical Computing (2020). Available online at: http://www.R-project.org/
13. Schlöndorff G, Mösges R, Meyer-Ebrecht D, Krybus W, Adams L. CAS (computer assisted surgery). A new procedure in head and neck surgery. HNO. (1989) 37:187–90.
14. Adams L, Gilsbach JM, Krybus W, Meyer-Ebrecht D, Moses R, Schlondorff G. CAS — a Navigation Support for Surgery. 3D Imaging Med. (1990) 26:411–23. doi: 10.1007/978-3-642-84211-5_26
15. Carney AS, Patel N, Baldwin DL, Coakham HB, Sandeman DR. Intra-operative image guidance in otolaryngology–the use of the ISG viewing wand. J Laryngol Otol. (1996) 110:322–7. doi: 10.1017/S0022215100133559
16. Staecker H, O'Malley BW, Eisenberg H, Yoder BE. Use of the LandmarXTM surgical navigation system in lateral skull base and temporal bone surgery. Skull Base. (2001) 11:245–55. doi: 10.1055/s-2001-18631
17. Sargent EW, Bucholz RD. Middle cranial fossa surgery with image-guided instrumentation. Otolaryngol Head Neck Surg. (1997) 117:131–4. doi: 10.1016/S0194-5998(97)70222-5
18. Osterdock RJ, Greene S, Mascott CR, Amedee R, Crawford BE. Primary myxoma of the temporal bone in a 17-year-old boy: case report. Neurosurgery. (2001) 48:945–8. doi: 10.1227/00006123-200104000-00055
19. Gunkel AR, Vogele M, Martin A, Bale RJ, Thumfart WF, Freysinger W. Computer-aided surgery in the petrous bone. Laryngoscope. (1999) 109:1793–9. doi: 10.1097/00005537-199911000-00013
20. Vrionis FD, Robertson JH, Foley KT, Gardner G. Image-interactive orientation in the middle cranial fossa approach to the internal auditory canal: an experimental study. Comput Aided Surg. (1997) 2:34–41. doi: 10.1002/(SICI)1097-0150(1997)2:1<34::AID-IGS6>3.0.CO;2-O
21. Caversaccio M, Romualdez J, Baechler R, Nolte LP, Kompis M, Häusler R. Valuable use of computer-aided surgery in congenital bony aural atresia. J Laryngol Otol. (2003) 117:241–8. doi: 10.1258/00222150360600814
22. Caversaccio M, Panosetti E, Ziglinas P, Lukes A, Häusler R. Cholesterol granuloma of the petrous apex: benefit of computer-aided surgery. Eur Arch Otorhinolaryngol. (2009) 266:47–50. doi: 10.1007/s00405-008-0719-4
23. Nemec SF, Donat MA, Mehrain S, Friedrich K, Krestan C, Matula C, et al. CT-MR image data fusion for computer assisted navigated neurosurgery of temporal bone tumors. Eur J Radiol. (2007) 62:192–8. doi: 10.1016/j.ejrad.2006.11.029
24. Stelter K, Ledderose G, Hempel JM, Morhard DFB, Flatz W, Krause E, et al. Image guided navigation by intraoperative CT scan for cochlear implantation. Comput Aided Surg. (2012) 17:153–60. doi: 10.3109/10929088.2012.668937
25. Van Havenbergh T, Koekelkoren E, De Ridder D, Van De Heyning P, Verlooy J. Image guided surgery for petrous apex lesions. Acta Neurochir. (2003) 145:737–42. doi: 10.1007/s00701-003-0054-x
26. Kohan D, Jethanamest D. Image-guided surgical navigation in otology. Laryngoscope. (2012) 122:2291–9. doi: 10.1002/lary.23522
27. Szyfter W, Kruk-Zagajewska A, Borucki U, Bartochowska A. Evolution in management of otogenic brain abscess. Otol Neurotol. (2012) 33:393–5. doi: 10.1097/MAO.0b013e3182488007
28. Hofer M, Dittrich E, Scholl C, Neumuth T. First clinical evaluation of the navigated controlled drill at the lateral skull base. Stud Health Technol Inform. (2008) 132:171–3.
29. Raine CH, Strachan DR, Gopichandran T. How we do it: using a surgical navigation system in the management of the ossified cochlea. Cochlear Implants Int. (2003) 4:96–101. doi: 10.1179/cim.2003.4.2.96
30. Girod SC, Rohlfing T, Maurer CR. Image-guided surgical navigation in implant-based auricular reconstruction. J Oral Maxillofac Surg. (2008) 66:1302–6. doi: 10.1016/j.joms.2007.06.636
31. Copeland BJ, Senior BA, Buchman CA, Pillsbury HC. The accuracy of computer-aided surgery in neurotologic approaches to the temporal bone: a cadaver study. Otolaryngol Head Neck Surg. (2005) 132:421–8. doi: 10.1016/j.otohns.2004.10.013
32. Ledderose GJ, Stelter K, Leunig A, Hagedorn H. Surface laser registration in ENT-surgery: accuracy in the paranasal sinuses—a cadaveric study. Rhinology. (2007) 45:281–5.
33. Pillai P, Sammet S, Ammirati M. Application accuracy of computed tomography-based, image-guided navigation of temporal bone. Neurosurgery. (2008) 63(Suppl.):67. doi: 10.1227/01.NEU.0000316429.19314.67
34. Balachandran R, Fitzpatrick JM, Labadie RF. Accuracy of image-guided surgical systems at the lateral skull base as clinically assessed using bone-anchored hearing aid posts as surgical targets. Otol Neurotol. (2008) 29:1050–5. doi: 10.1097/MAO.0b013e3181859a08
35. Labadie RF, Majdani O, Fitzpatrick JM. Image-guided technique in neurotology. Otolaryngol Clin North Am. (2007) 40:611–24. doi: 10.1016/j.otc.2007.03.006
36. Labadie RF, Shah RJ, Harris SS, Cetinkaya E, Haynes DS, Fenlon MR, et al. Submillimetric target-registration error using a novel, non-invasive fiducial system for image-guided otologic surgery. Comput Aided Surg. (2004) 9:145–53. doi: 10.1080/10929080500066922
37. Labadie RF, Davis BM, Fitzpatrick JM. Image-guided surgery: what is the accuracy? Curr Opin Otolaryngol Head Neck Surg. (2005) 13:27–31. doi: 10.1097/00020840-200502000-00008
38. Schipper J, Aschendorff A, Arapakis I, Klenzner T, Teszler CB, Ridder GJ, et al. Navigation as a quality management tool in cochlear implant surgery. J Laryngol Otol. (2004) 118:764–70. doi: 10.1258/0022215042450643
39. Stolka PJ, Henrich D, Tretbar SH, Federspil PA. First 3D ultrasound scanning, planning, and execution of CT-free milling interventions with a surgical robot. Proc 30th Annu Int Conf IEEE Eng Med Biol Soc EMBS'08. (2008) 2008:5605–10. doi: 10.1109/IEMBS.2008.4650485
40. Matsumoto N, Hong J, Hashizume M, Komune S. A minimally invasive registration method using Surface Template-Assisted Marker Positioning (STAMP) for image-guided otologic surgery. Otolaryngol Head Neck Surg. (2009) 140:96–102. doi: 10.1016/j.otohns.2008.10.005
41. Hong J, Matsumoto N, Ouchida R, Komune S, Hashizume M. Medical navigation system for otologic surgery based on hybrid registration and virtual intraoperative computed tomography. IEEE Trans Biomed Eng. (2009) 56:426–32. doi: 10.1109/TBME.2008.2008168
42. Dalgorf D, Daly M, Chan H, Siewerdsen J, Irish J. Accuracy and reproducibility of automatic vs. manual registration using a cone-beam CT image guidance system. J Otolaryngol Head Neck Surg. (2011) 40:75–80.
43. Majdani O, Bartling SH, Leinung M, Stöver T, Lenarz M, Dullin C, et al. A true minimally invasive approach for cochlear implantation. Otol Neurotol. (2008) 29:120–3. doi: 10.1097/mao.0b013e318157f7d8
44. Labadie RF, Chodhury P, Cetinkaya E, Balachandran R, Haynes DS, Fenlon MR, et al. Minimally invasive, image-guided, facial-recess approach to the middle ear: demonstration of the concept of percutaneous cochlear access in vitro. Otol Neurotol. (2005) 26:557–62. doi: 10.1097/01.mao.0000178117.61537.5b
45. Warren FM, Balachandran R, Fitzpatrick JM, Labadie RF. Percutaneous cochlear access using bone-mounted, customized drill guides: demonstration of concept in vitro. Otol Neurotol. (2007) 28:325–9. doi: 10.1097/01.mao.0000253287.86737.2e
46. Williamson T, Gavaghan K, Gerber N, Weder S, Anschuetz L, Wagner F, et al. Population statistics approach for safety assessment in robotic cochlear implantation. Otol Neurotol. (2017) 38:759–64. doi: 10.1097/MAO.0000000000001357
47. Bell B, Stieger C, Gerber N, Arnold A, Nauer C, Hamacher V, et al. A self-developed and constructed robot for minimally invasive cochlear implantation. Acta Otolaryngol. (2012) 132:355–60. doi: 10.3109/00016489.2011.642813
48. Majdani O, Rau TS, Baron S, Eilers H, Baier C, Heimann B, et al. A robot-guided minimally invasive approach for cochlear implant surgery: preliminary results of a temporal bone study. Int J Comput Assist Radiol Surg. (2009) 4:475–86. doi: 10.1007/s11548-009-0360-8
49. Bell B, Gerber N, Williamson T, Gavaghan K, Wimmer W, Caversaccio M, et al. In vitro accuracy evaluation of image-guided robot system for direct cochlear access. Otol Neurotol. (2013) 34:1284–90. doi: 10.1097/MAO.0b013e31829561b6
50. Gerber N, Gavaghan K, Bell B, Williamson TM. High-accuracy patient-to-image registration for the facilitation of image-guided robotic microsurgery on the head. IEEE Trans Biomed Eng. (2013) 60:960–8. doi: 10.1109/TBME.2013.2241063
51. Kral F, Puschban EJ, Riechelmann H, Freysinger W. Comparison of optical and electromagnetic tracking for navigated lateral skull base surgery. Int J Med Robot. (2013) 9:247–52. doi: 10.1002/rcs.1502
52. Ke J, Zhang S, Li C, Zhu Y, Hu L, Ma F. Application of bonebed-malleus short process registration in minimally invasive cochlear implantation. Comput Assist Surg. (2016) 21:30–6. doi: 10.1080/24699322.2016.1240306
53. Zhou C, Anschuetz L, Weder S, Xie L, Caversaccio M, Weber S, et al. Surface matching for high-accuracy registration of the lateral skull base. Int J Comput Assist Radiol Surg. (2016) 11:1–7. doi: 10.1007/s11548-016-1394-3
54. Schneider D, Hermann J, Gerber KA, Ansó J, Caversaccio M, Weber S, et al. Noninvasive registration strategies and advanced image guidance technology for submillimeter surgical navigation accuracy in the lateral skull base. Otol Neurotol. (2018) 39:1326–35. doi: 10.1097/MAO.0000000000001993
55. Weber S, Gavaghan K, Wimmer W, Williamson T, Gerber N, Ansó J, et al. Instrument flight to the inner ear. Sci Robot. (2017) 2:eaal4916. doi: 10.1126/scirobotics.aal4916
56. Labadie RF, Balachandran R, Noble JH, Blachon GS, Mitchell JE, Reda FA, et al. Minimally invasive image-guided cochlear implantation surgery: first report of clinical implementation. Laryngoscope (2014) 124:1915–22. doi: 10.1002/lary.24520
57. Caversaccio M, Wimmer W, Ansó J, Mantokoudis G, Gerber N, Rathgeb C, et al. Robotic middle ear access for cochlear implantation: first in man. PLoS ONE. (2019) 14:1–12. doi: 10.1371/journal.pone.0220543
58. Cho B, Oka M, Matsumoto N, Ouchida R, Hong J, Hashizume M. Warning navigation system using real-time safe region monitoring for otologic surgery. Int J Comput Assist Radiol Surg. (2013) 8:395–405. doi: 10.1007/s11548-012-0797-z
59. Samii M, Metwali H, Samii A, Gerganov V. Retrosigmoid intradural inframeatal approach: indications and technique. Oper Neurosurg. (2013) 73(Suppl.1):ons53–60. doi: 10.1227/NEU.0b013e3182889e59
60. Grauvogel J, Scheiwe C, Masalha W, Grauvogel T, Kaminsky J, Vasilikos I. Piezosurgery-, neuroendoscopy-, and neuronavigation-assisted intracranial approach for removal of a recurrent petrous apex cholesteatoma: technical note. J Neurosurg Pediatr. (2018) 21:322–8. doi: 10.3171/2017.8.PEDS17327
61. Leal AG, da Silva EB, Ramina R. Surgical exposure of the internal auditory canal through the retrosigmoid approach with semicircular canals anatomical preservation. Arq Neuropsiquiatr. (2015) 73:425–30. doi: 10.1590/0004-282X20150020
62. Kristin J, Mucha D, Schipper J, Klenzner T. Registrierstrategien für die Anwendung des Navigationssystems FIAGON an der lateralen Schädelbasis. Laryngorhinootologie. (2012) 91:306–10. doi: 10.1055/s-0031-1299755
63. Kristin J, Burggraf M, Mucha D, Malolepszy C, Anderssohn S, Schipper J, et al. Automatic registration for navigation at the anterior and lateral skull base. Ann Otol Rhinol Laryngol. (2019) 128:894–902. doi: 10.1177/0003489419849086
64. Rathgeb C, Wagner F, Wimmer W, Gerber N, Williamson T, Anschuetz L, et al. The accuracy of image-based safety analysis for robotic cochlear implantation. Int J Comput Assist Radiol Surg. (2018) 14:83–92. doi: 10.1007/s11548-018-1834-3
65. Kempfle J, Kozin ED, Remenschneider AK, Eckhard A, Edge A, Lee DJ. Endoscopic transcanal retrocochlear approach to the internal auditory canal with cochlear preservation: pilot cadaveric study. Otolaryngol Head Neck Surg. (2016) 154:920–3. doi: 10.1177/0194599816630979
66. Sobhy Afifi WF, Guigou C, Mazalaigue S, Camuset JP, Ricolfi F, Bozorg Grayeli A. Navigation-guided transmodiolar approach for auditory nerve implantation via the middle ear in humans. Audiol Neurootol. (2015) 20:128–35. doi: 10.1159/000366513
67. Colasanti R, Tailor AR, Lamki T, Zhang J, Ammirati M. Maximizing the petroclival region exposure via a suboccipital retrosigmoid approach: where is the intrapetrous internal carotid artery? Oper Neurosurg. (2015) 11:329–36. doi: 10.1227/NEU.0000000000000749
68. Ding Z, Wang Q, Lu X, Qian X. Endoscopic intradural subtemporal keyhole approach with neuronavigational assistance to the suprasellar, petroclival, and ventrolateral brainstem regions: an anatomic study. World Neurosurg. (2017) 101:606–14. doi: 10.1016/j.wneu.2017.02.052
69. Matsushima K, Komune N, Matsuo S, Kohno M. Microsurgical and endoscopic anatomy for intradural temporal bone drilling and applications of the electromagnetic navigation system: various extensions of the retrosigmoid approach. World Neurosurg. (2017) 103:620–30. doi: 10.1016/j.wneu.2017.04.079
70. Rathgeb C, Anschuetz L, Schneider D, Dür C, Caversaccio M, Weber S, et al. Accuracy and feasibility of a dedicated image guidance solution for endoscopic lateral skull base surgery. Eur Arch Oto-Rhino-Laryngol. (2018) 275:905–11. doi: 10.1007/s00405-018-4906-7
71. Komune N, Matsushima K, Matsuo S, Safavi-abbasi S, Matsumoto N, Rhoton AL. The accuracy of an electromagnetic navigation system in lateral skull base approaches. Laryngoscope. (2017) 127:450–9. doi: 10.1002/lary.25998
72. Schwam ZG, Kaul VZ, Cosetti MK, Wanna GB. Accuracy of a modern intraoperative navigation system for temporal bone surgery in a cadaveric model. Otolaryngol Neck Surg. (2019) 161:842–5. doi: 10.1177/0194599819864292
73. GmbH. CamBar B1—Axios 3D® Services GmbH. (2020). Available online at: https://axios3d.de/optische-messsysteme/cambar-b1/?lang=en (accessed April 16, 2020).
74. Bento RF, De Oliveira Fonseca AC. A brief history of mastoidectomy. Int Archiv Otorhinolaryngol. (2013) 17:168–78. doi: 10.7162/S1809-97772013000200009
75. Labadie RF, Fitzpatrick JM. Image-Guided Surgery: Fundamentals and Clinical Applications in Otolaryngology, 1st edn. San Diego, CA: Plural Publishing (2016). p. 130.
76. ASTM International. ASTM F2554 - 18 - Standard Practice for Measurement of Positional Accuracy of Computer Assisted Surgical Systems. (2018). Available online at: https://www.astm.org/Standards/F2554.htm (accessed May 15, 2020).
Keywords: lateral skull, lateral skull base, neurotology, temporal bone, image-guidance, surgical navigation, accuracy, temporal evolution
Citation: Schneider D, Hermann J, Mueller F, Braga GO'TB, Anschuetz L, Caversaccio M, Nolte L, Weber S and Klenzner T (2021) Evolution and Stagnation of Image Guidance for Surgery in the Lateral Skull: A Systematic Review 1989–2020. Front. Surg. 7:604362. doi: 10.3389/fsurg.2020.604362
Received: 09 September 2020; Accepted: 13 October 2020;
Published: 11 January 2021.
Edited by:
Narayanan Prepageran, University of Malaya, MalaysiaReviewed by:
Ing Ping Tang, Universiti Malaysia Sarawak, MalaysiaA. B. Zulkiflee, University Malaya Medical Centre, Malaysia
Sanjeev Mohanty, MGM Health Care, India
Narayan Jayashankar, Dr. Balabhai Nanavati Hospital, India
Philip Rajan, Raja Permaisuri Bainun Hospital, Malaysia
Copyright © 2021 Schneider, Hermann, Mueller, Braga, Anschuetz, Caversaccio, Nolte, Weber and Klenzner. This is an open-access article distributed under the terms of the Creative Commons Attribution License (CC BY). The use, distribution or reproduction in other forums is permitted, provided the original author(s) and the copyright owner(s) are credited and that the original publication in this journal is cited, in accordance with accepted academic practice. No use, distribution or reproduction is permitted which does not comply with these terms.
*Correspondence: Fabian Mueller, ZmFiaWFuLm11ZWxsZXJAYXJ0b3JnLnVuaWJlLmNo