- 1Laboratory of Physical Activity and Health Promotion, Institute of Physical Education and Sports, University of Rio de Janeiro State, Rio de Janeiro, RJ, Brazil
- 2Postgraduate Program in Clinical and Experimental Physiopathology, Faculty of Medical Sciences, State University of Rio de Janeiro, Rio de Janeiro, RJ, Brazil
- 3Department of Microbiology and Immunology, Tulane National Primate Research Center and Tulane University School of Medicine, Covington, LA, United States
Background: Tryptophan (TRP) metabolism through the kynurenine (KYN) pathway is influenced by inflammatory mediators, generating metabolites that regulate immune and inflammatory responses. Exercise has been proposed as a modulator of this pathway, but its role in health benefits and chronic disease management remains unclear.
Objective: This systematic review examines exercise-induced adaptations in the KYN pathway and their potential implications for health and disease management. Additionally, we identify key methodological considerations for future research.
Methods: A structured search of PubMed/Medline, Web of Science, and Scopus was conducted up to October 2024 to identify clinical trials investigating the effects of exercise training on the KYN pathway.
Results: Of 2,795 articles initially found, 13 clinical trials involving 592 participants met the inclusion criteria. Most studies reported exercise-induced adaptations in the KYN pathway, particularly in cancer survivors. These adaptations appeared to be influenced by exercise intensity and duration. However, several methodological limitations were noted, and no trials included patients with metabolic or cardiovascular diseases.
Conclusions: Here, we show that exercise training modulates the KYN pathway in both healthy and diseased populations, highlighting its potential for disease prevention and management. However, further randomized-controlled trials are needed to clarify its mechanisms and clinical applications, particularly in metabolic and cardiovascular diseases.
Systematic Review Registration: https://www.crd.york.ac.uk/PROSPERO/view/CRD42022351481, PROSPERO (CRD42022351481).
1 Introduction
Tryptophan (TRP) is an essential amino acid derived entirely from dietary sources required for protein biosynthesis. Discovered by Hopkins and Cole (1) in 1901 and structurally characterized by Ellinger and Flamand (2) in 1907, TRP has since been shown to participate in several metabolic pathways (3). However, only a small percentage of ingested TRP participates in protein biosynthesis; more than 95% is broken down via the kynurenine (KYN) pathway (4–6), producing various metabolites that have significant roles in regulating immune responses, inflammation, neuronal functions, and gut homeostasis (7–9). These metabolites, collectively referred to as KYN, include kynurenine (KYN), kynurenic acid (KYNA), and quinolinic acid (QUINA) (10), and are involved in the production of nicotinic acid, a precursor for nicotinamide adenine dinucleotide (NAD) (3), which is crucial for cellular energy metabolism (11). Except for hepatocytes, few cells have enzymatic apport to fully degrade TRP to NAD. This makes KYN metabolites important mediators of crosstalk between cells or organs, as they can be exchanged between tissues to exert various biological effects (12, 13).
One of the key regulators of the KYN pathway are two enzymes: indoleamine 2,3-dioxygenase (IDO1) and tryptophan 2,3-dioxygenase (TDO). IDO1 is expressed in a wide range of tissues, including the brain, lungs, heart, kidneys, and intestines, while TDO is primarily active in the liver (14). Both enzymes catalyze the initial step of TRP catabolism, converting TRP into formylkynurenine (14), which is further metabolized to KYNA via kynurenine aminotransferases (KAT) or to 3-hydroxykynurenine (3HK) via kynurenine 3-monoxygenase (KMO), and eventually to QUINA (14, 15). Figure 1 illustrates the KYN pathway.
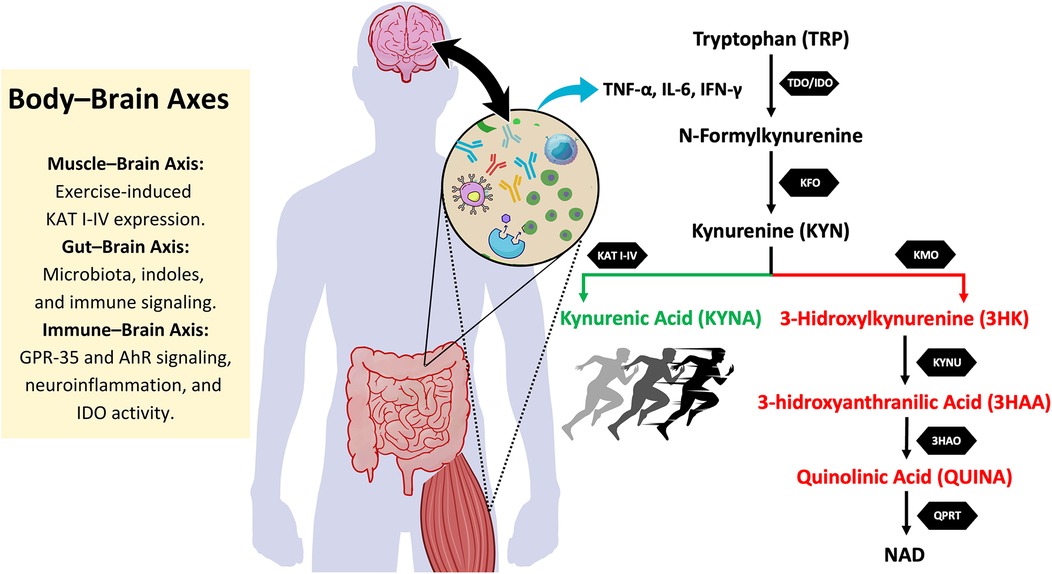
Figure 1. Body-Brain axes and its interaction with the kynurenine pathway. This figure illustrates the kynurenine (KYN) pathway and its interaction with different body-brain axes. Tryptophan (TRP) metabolism is initiated by the enzymes tryptophan 2,3-dioxygenase (TDO) and indoleamine 2,3-dioxygenase (IDO), leading to the formation of N-formylkynurenine, which is further converted into kynurenine (KYN). From this point, KYN follows two main metabolic routes: the neuroprotective pathway (in green), where kynurenine aminotransferases (KAT I-IV) convert KYN into kynurenic acid (KYNA), a metabolite with anti-inflammatory and neuroprotective properties, and the neurotoxic pathway (in red), in which kynurenine 3-monooxygenase (KMO) converts KYN into 3-hydroxykynurenine (3HK). 3HK is further metabolized into 3-hydroxyanthranilic acid (3HAA) and then into quinolinic acid (QUINA), a neurotoxic compound involved in excitotoxicity and neuroinflammation. Exercise promotes KAT expression, favoring KYNA production and shifting the balance towards the neuroprotective pathway. Additionally, the figure highlights key body-brain axes that influence this metabolism. The muscle-brain axis is involved in exercise-induced KAT expression, enhancing KYNA levels. The gut-brain axis regulates kynurenine metabolism through microbiota-derived signals and immune modulation. The immune-brain axis plays a crucial role in shifting TRP metabolism, as pro-inflammatory cytokines such as TNF-α, IL-6, and IFN-γ stimulate IDO activity, favoring the production of neurotoxic metabolites. Together, these interactions influence neuroinflammation, neurodegenerative processes, and exercise-induced neuroprotection. KFO, kynurenine formamidase; KYNU, kynureninase; 3HAO, 3-hydroxyanthranilic acid oxygenase; QPRT, quinolinate phosphoribosyltransferase; NAD, nicotinamide adenine dinucleotide; AhR, aryl hydrocarbon receptor; GPR35, G protein-coupled receptor 35.
The activity of IDO1 and TDO increases in response to cytokine signaling, particularly during inflammatory responses (16, 17). Consequently, the KYN pathway is tightly regulated by cytokines, which can either enhance or suppress its activity depending on the body's inflammatory state (18). In chronic conditions, sustained elevation of inflammatory cytokines leads to excessive activation of this pathway (19–21). This overactivation leads to an increase in the production of neurotoxic metabolites, such as 3HK and QUINA (see Figure 1). 3HK and QUINA exert neuronal excitotoxicity due to its agonist activity at N-methyl-D-aspartate receptors (NMDAR) (22). These metabolites contribute to inflammation, immune tolerance, oxidative stress, and neuronal apoptosis (3, 12, 23, 24), and are implicated in the pathogenesis of several diseases, including neurodegenerative disorders (such as Alzheimer's, Parkinson's, and multiple sclerosis) (3, 25, 26) and cancers (24, 27, 28), both of which show elevated KYN levels. Additionally, aging is associated with alterations in the KYN pathway, as increased levels of KYN were observed in older muscle tissues (29). Elevated KYN levels are also correlated with several metabolic disorders, including obesity, dyslipidemia, insulin resistance, and diabetes (30, 31). In contrast, TRP levels are inversely associated with cardiovascular disease incidence (32, 33).
Despite the harmful effects of KYN metabolites like QUINA (34, 35), the KYN pathway also produces neuroprotective agents, such as KYNA (36, 37). KYNA acts by antagonizing NMDAR and α7 nicotinic acetylcholine receptors (α7nAChR) (38, 39), protecting neurons from excitotoxicity and oxidative damage (40). KYNA also exerts anti-inflammatory effects through its interaction with G protein-coupled receptor 35 (GPR35) in adipocytes, which inhibits TNF-α release by macrophages under inflammatory conditions (41–43). Additionally, KYNA mediates anti-inflammatory responses (44, 45) by activating the aryl hydrocarbon receptor (AhR), which promotes the differentiation of T helper 17 (Th17) cells into regulatory T cells (Treg) (46). These mechanisms suggest that KYNA plays a critical role in maintaining the balance between neurotoxicity and neuroprotection within the KYN pathway (47, 48).
The peripheral KYN pathway also influences the central nervous system (39). While KYN, 3HK, and other metabolites can cross the blood-brain barrier, KYNA and QUINA are generally restricted to peripheral tissues (49). This restriction raises the possibility that altering the balance of KYN metabolism in peripheral tissues, for instance by increasing KYNA production, may help reduce the neurotoxic effects of elevated KYN levels in the brain. Given that TRP, KYN, and 3HK can pass through the blood-brain barrier, strategies aimed at rerouting the KYN pathway toward KYNA production could theoretically provide a therapeutic approach to mitigating neurodegenerative diseases and other central nervous system disorders (39, 50).
Lifestyle-based interventions have recently been suggested to modulate TRP metabolism, aiding in the prevention and treatment of diseases with inflammatory mechanisms (39, 44). Exercise training, in particular, has been shown to increase the expression of KAT, redirecting the KYN pathway towards its protective branch in skeletal muscle in humans (51, 52) and mice (41, 51, 53). Evidence from pre-clinical models shows that this re-routing enhances lipid metabolism, and thermogenesis, and reduces weight gain, inflammation, insulin resistance, and glucose intolerance (41, 54), although energy metabolism was largely unaffected in KMO knockout mice (55). Additionally, clinical evidence supports the beneficial role of physical exercise on the KYN pathway in cancer (56, 57) and central nervous system disorders, such as major psychological disorders (39, 58–60). Conversely, studies in healthy individuals (61) and older adults at risk of dementia have failed to identify changes in KYN pathway and benefits after exercise training (60). Collectively, these findings suggest that the benefits of exercise may be more pronounced in certain populations or disease states. There is also growing interest in the role of exercise-induced adaptations of the KYN pathway in chronic diseases associated with inflammation, such as metabolic disorders (62, 63).
Given the potential exercise-induced adaptations in the KYN pathway and their implications for chronic diseases, further research is needed to elucidate the effects of exercise training and its mechanisms on KYN pathway, and to determine whether these effects translate into meaningful clinical benefits for individuals with different health conditions. This review systematically examines clinical trials investigating the adaptations to exercise training on the KYN pathway and its impact on health and disease. We first explore how physical exercise influences this pathway, discussing the molecular adaptations that may contribute to its protective effects in healthy populations. Next, we provide an overview of the findings of the exercise-induced adaptations on the KYN pathway in various chronic conditions. We then summarize findings, identifying key methodological considerations that may explain discrepancies in literature. Finally, we outline current knowledge gaps and propose future research directions to enhance our understanding of how exercise modulates TRP metabolism and whether these adaptations translate into meaningful clinical benefits.
2 Methods
This systematic review was conducted in accordance with the Preferred Reporting Items for Systematic Reviews and Meta-Analysis Protocols (PRISMA) guidelines (64). The study was registered in the International Prospective Register of Systematic Reviews (PROSPERO) under the number CRD42022351481, and the protocol was strictly followed through all stages of this review. Studies were selected according to the criteria mentioned in the below sections.
2.1 Search strategy
Searches were conducted from inception until August 5, 2022, and updated on October 25, 2024, in MEDLINE (via PubMed), Web of Science, and Scopus databases. No date restrictions were applied, and filters were set for human studies and English language articles. A search strategy using Boolean operators “AND” and “OR” and terms related to “exercise training” and “kynurenine pathway” was applied to identify relevant trials (see Supplementary Appendix 1).
2.2 Study selection
After removing duplicates, two independent investigators (MR and JB) screened studies in two stages: (1) title and abstract review, and (2) full-text evaluation. Studies failing to meet inclusion criteria at any stage were excluded. Reference lists of selected studies were manually reviewed for additional eligible studies. Discrepancies were resolved through discussion between investigators, and if consensus could not be reached, a third reviewer (KGL) was consulted. Agreement on inclusion was validated in a random sample of 50 abstracts, yielding a Cohen's kappa coefficient of 0.84–0.99 (p < 0.05).
2.3 Eligibility criteria
Only original trials investigating the effects of exercise training on KYN pathway metabolites were included. Studies were considered if they met the PICOS criteria, as shown in Table 1. No minimum exercise prescription was required. However, authors should have at least reported three of the variables of exercise training prescription, according to the FIIT principle. This principle, which stands for Frequency, Intensity, Time, and Type of exercise, is a fundamental framework used in exercise prescription and research to describe and standardize exercise interventions (65). Each component helps ensure that exercise regimens are clearly defined, reproducible, and comparable across studies.
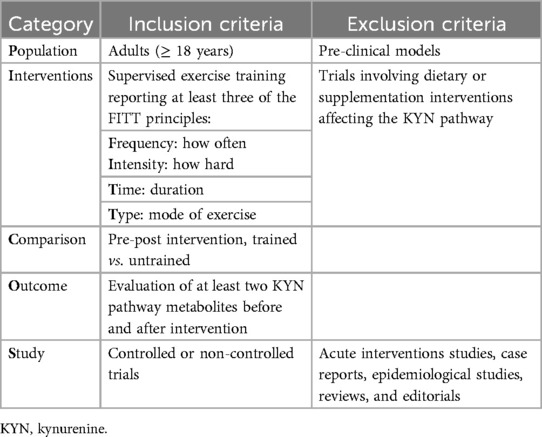
Table 1. Inclusion and exclusion criteria based on PICOS strategy (population, intervention, comparison, outcome and study).
2.4 Quality and risk of bias assessment
All included studies were assessed for methodological quality using the Tool for Assessment of Study Quality and Reporting in Exercise (TESTEX scale) (66, 67). TESTEX is a widely used 15-point scale (5 points for study quality and 10 for reporting), specifically designed for exercise studies, addressing criteria not considered in other quality assessment tools. It was chosen due to its validation in evaluating exercise intervention trials and its ability to capture the nuances of exercise prescription fidelity (66).
Additionally, studies were assessed for risk of bias using the Cochrane Collaboration's (RoB, Risk of Bias 2) tool (67, 68). This tool evaluates five domains of bias: Randomization process, Deviations from intended interventions, Missing outcome data, Measurement of the outcome, Selection of the reported result, and Overall bias. Assessments were independently conducted by two authors (MR and JB), and mean scores were assigned for each evaluation method.
3 Results
Figure 2 displays the PRISMA flowchart summarizing article selection, while Table 2 presents the methodological quality scores based on TESTEX. Of the 2,796 articles initially found in databases and reference list, 1,038 duplicates were removed, and 1,737 were excluded after title and abstract screening, leaving 21 articles for full evaluation. Of those, 13 articles met the inclusion criteria. Overall, study quality ranged from poor to moderate, with TESTEX scores between 3 and 11 (median score 7). Two trials were rated as high quality (73% of items satisfied), 4 as moderate (50%–72% of items satisfied), and 7 as very low quality (satisfying less than 50% of the items). The risk of bias assessment for each study is presented in Supplementary Appendix 2, with a summary provided in Figure 3. The assessment revealed that most of the included studies (9 out of 13) had some concerns regarding bias. Three studies were classified as having a high risk of bias, while only one was deemed to have a low risk after evaluation.
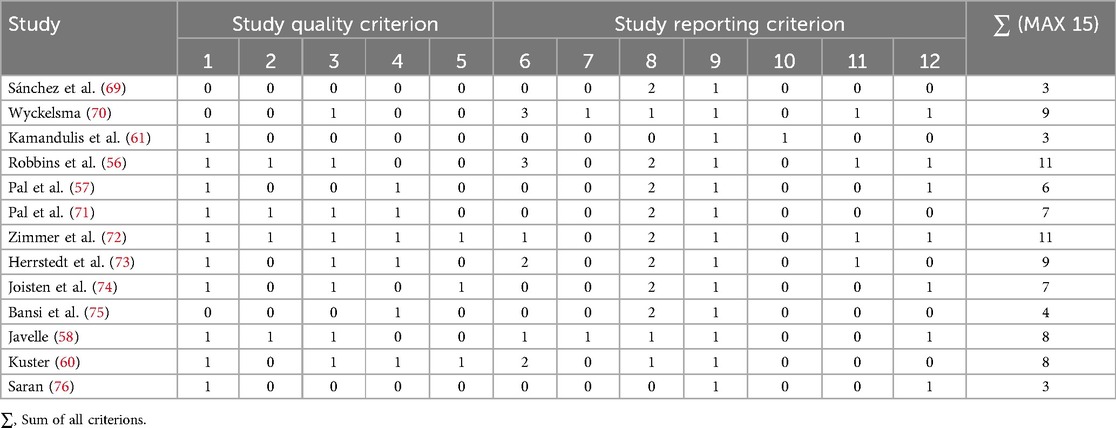
Table 2. TESTEX assessment of the quality and reporting of studies about adaptations to exercise training on the kynurenine pathway in health and disease.
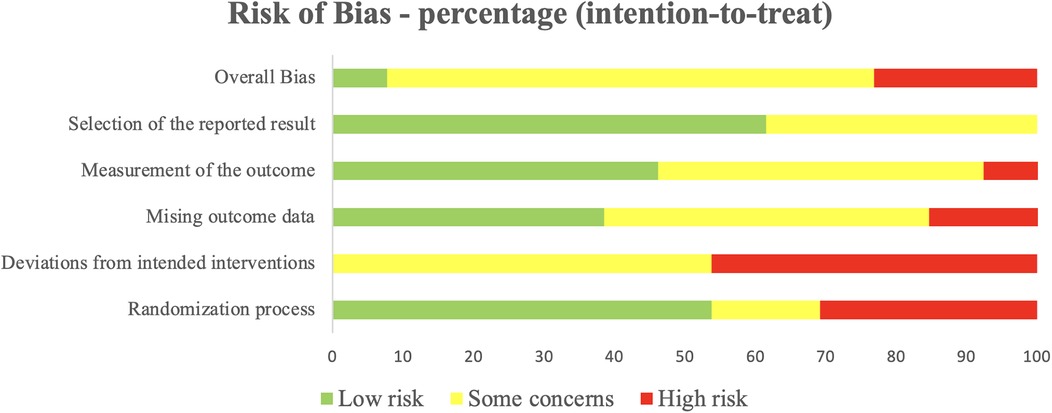
Figure 3. Percentage of studies examining the efficacy of exercise training in modulating the kynurenine pathway with low, some concerns, and high risk of bias for each feature of the cochrane risk of bias tool.
Table 3 summarizes the characteristics of the included studies, such as sample, interventions, and outcomes. Of the 13 studies, 5 were randomized controlled trials, and 8 were non-randomized experiments. Eleven studies (84.6%) were conducted in Europe (57, 58, 60, 61, 70–76), with the remaining 2 in the Americas (56, 69). Regarding study populations, only three studies (23%) involved healthy volunteers (61, 69, 70), while 10 (77%) focused on patients with chronic conditions, including cancer (56, 57, 71–73), multiple sclerosis (74, 75), emotionally impulsivity (58), dementia risk (60), and chronic low back pain (76).
Among the 13 included studies, 11 reported evidences of exercise-induced adaptations in the KYN pathway (56–58, 69–76). The two studies (60, 61) that failed to observe such adaptations were conducted in healthy individuals (61) (representing 33% of all studies in healthy populations), and in older adults at risk of dementia (60). Exercise-induced adaptations included changes in muscle KAT content (70), Peroxisome proliferator-activated receptor-gamma coactivator (PGC)-1α (56), KYN or TRP (56, 57, 69, 72, 74–76), KYNA (58, 69, 76) and IDO-1 and 2 levels (71, 76).
4 Discussion
We investigated the effects of exercise training on the KYN pathway and its implications in health and chronic conditions. Our findings suggest that exercise-induced adaptations in the KYN pathway differ across populations, with more pronounced effects observed in individuals with chronic diseases. These results contribute to the growing evidence that physical exercise modulates TRP metabolism, promoting neuroprotective and anti-inflammatory effects. Given the increasing interest in the role of the KYN pathway in various pathophysiological conditions, our study provides relevant insights into its responsiveness to exercise interventions.
Limited studies have examined the effect of supervised exercise training on KYN pathway metabolites in healthy individuals. While some research suggests beneficial adaptations (69, 70), findings remain inconsistent (61). In young adults, endurance-based swimming training showed greater reductions in circulating KYN and increases in KYNA compared to tactical immersion training, likely due to differences in oxidative stress and metabolic demands (69). Studies on older adults demonstrated that vigorous sprint interval training significantly reduced plasma QUINA levels and increased the KYNA/QUINA ratio and KAT content (70). However, when exercise was combined with dietary antioxidants, these effects were blunted, suggesting that a pro-oxidant environment may be necessary to drive beneficial shifts in KYN metabolism (70). Additionally, Boßlau, Wasserfurth (77) reported that 12 weeks of unsupervised combined training could redirect the KYN pathway toward KYNA. This shift appears to be associated with mitigating immune senescence in older adults, as evidenced by attenuated CD8+ T-cell differentiation.
Collectively, these findings indicate that exercise intensity plays a critical role in driving KYN pathway adaptations, likely through its influence on oxidative stress and inflammatory signaling. Alongside this finding, another study (78) demonstrated that 4 weeks of unsupervised moderate-intensity home-based exercises failed to improve TRP or KYN pathway in healthy young adults. The authors speculated that a more vigorous exercise regimen would likely have promoted changes in the KYN pathway. However, Kamandulis, Lukonaitiene (61) reported unchanged KYN metabolites after three weeks of combined resistance and high intensity interval training (HIIT), despite improvements in mood profile. Thus, results remain inconsistent, underscoring the need for further research exploring different exercise modalities, including resistance training and HIIT, to determine their impact on KYN metabolism in healthy populations.
The KYN pathway plays a crucial role in immune and neurological regulation (79), and its dysregulation is associated with numerous diseases, including neurodegenerative disorders (80), cancer (81), and metabolic syndrome (82). Chronic inflammation and oxidative stress contribute to pathway overactivation (83), leading to the accumulation of neurotoxic metabolites such as QUINA and 3HK (55). Exercise training appears to counteract these effects by promoting a shift toward KYNA production (54), which exerts neuroprotective and anti-inflammatory properties. Our analysis showed that exercise-induced increases in KYNA and reductions in the KYN/TRP ratio were more consistent in clinical populations, suggesting that individuals with systemic inflammation may experience greater therapeutic benefits from exercise interventions.
Most research investigating exercise-induced KYN pathway adaptations has focused on cancer survivors, particularly in those with pancreatic (57), gastro-esophageal junction (73), prostate (71), and breast cancers (56, 72) (71). Elevated KYN levels are linked to poor prognosis in cancer patients (84). KYN and its metabolites suppress T-cell function, promote regulatory T-cell differentiation, and impair natural killer cell activity (39, 57, 85). Additionally, NAD + synthesis via the KYN pathway fuels oncogenic processes, as cancer cells rely heavily on NAD + to meet increased ATP demands (24).
Exercise has been shown to reduce cancer risk and progression (86), partly by improving the anti-inflammatory profile and reducing systemic inflammation (87). Resistance training and HIIT have demonstrated benefits in modulating KYN metabolism, likely through exercise-induced activation of PGC-1α, which increases skeletal muscle KAT content (54) and shifts the KYN pathway toward KYNA production (21). This helps to mitigate inflammation by activating GPR35 (41) and the KYNA-AhR axis (12). Additionally, increased KAT levels reroute the KYN pathway, preventing the overproduction of immunosuppressive intermediate metabolites, such as anthranilic acid (AA), 3-hydroxylanthranilic acid (3HAA) and QUINA, which promote immune evasion and cancer cell migration (24, 88). Studies in breast cancer survivors reported reduced KYN levels following 12 weeks of resistance training, with untrained controls exhibiting a shift toward neurotoxic KYN metabolites (34). Similar benefits were observed in pancreatic cancer survivors undergoing chemotherapy, where strength training prevented increases in KYN levels and the KYN/TRP ratio (23). In gastro-esophageal junction cancer survivors, concurrent training attenuated inflammatory and neurotoxic metabolites while reducing depression and anxiety symptoms (35). Interestingly, Robbins, Kelleher (56) reported increased PGC-1α activation following exercise training, suggesting that changes in KYN levels were driven by exercise-induced PGC-1α activation, as supported by animal studies (54).
Regarding the intervention types, Pal, Schneider (71) found HIIT-based training more effective than moderate-intensity continuous training (MICT) in modulating KYN pathway metabolism. Polarized endurance training involving HIIT sessions reduced IDO levels, whereas standard training increased them. Although no changes in AhR levels were observed, the authors suggested that polarized training might downregulate the AhR/IDO axis, affecting natural killers (NK) cells. This is relevant since inflammation-induced increases in IDO elevate KYN, acting as potent AhR agonists in the cancer microenvironment, promoting IDO expression in a feedback loop that suppresses innate immune responses by reducing NK cell function (84). Exercise-induced reductions in IDO, KYN, and AhR expression may therefore enhance immune responses in cancer patients (39, 89).
Neurodegenerative disorders and psychiatric conditions, including depression and schizophrenia, are also linked to KYN pathway dysregulation (90). Javelle, Bloch (58) demonstrated that HIIT reduced inflammation and KYN metabolism in emotionally impulsive individuals, improving impulsivity scores. Exercise also reduced IL-6 levels, possibly via KYNA's anti-inflammatory actions through GPR35 activation (39, 91, 92). In contrast, Küster et al. (60) found no exercise-induced adaptations in KYN pathway metabolism among older adults at risk of dementia. In this study, exercise intensity was not controlled, and only two exercise sessions per week were conducted, which may have limited the potential benefits of the exercise training.
Recently, Kupjetz, Patt (93) conducted a randomized controlled trial comparing the effects of endurance training on KYN pathway modulation in individuals with multiple sclerosis. Their findings indicate that both HIIT and MICT similarly reduced most KYN metabolites over time, with baseline systemic inflammation influencing exercise-induced changes. Likewise, Joisten, Rademacher (74) found no significant differences between HIIT vs. MICT for most metabolites, except for an increase in the KYN/TRP ratio. Bansi, Koliamitra (75) also compared these exercise modalities and reported no overall differences, though responses varied by multiple sclerosis subtype. Notably, patients with relapsing-remitting multiple sclerosis, a milder form of the disease, showed an increase in the KYN/TRP ratio compared to those with secondary progressive multiple sclerosis, contradicting the authors' hypothesis that exercise would promote a long-term anti-inflammatory effect. However, these studies employed a three-week intervention, a relatively short duration for promoting chronic adaptations. Additionally, neither study included an untrained control group, making it difficult to determine whether exercise intervention prevented a worsening of KYN metabolism (74). These methodological limitations restrict the generalizability of the findings.
Among all studies examining exercise-induced adaptations in the KYN pathway for disease, only one was conducted outside of cancer or central nervous system disorder populations. Saran, Turska (76) demonstrated that two weeks of aerobic training decreased KYN and increased KYNA levels in patients with chronic low back pain, though these differences were not observed at the end of the protocol (4 weeks). However, the absence of an untrained control group and lack of control over menstrual cycle phases (among women who comprised most of the sample) should be considered. Thus, the promising findings should be interpreted with caution.
While exercise training appears to induce beneficial shifts in KYN metabolism across various conditions (94, 95), critical gaps remain in literature. Notably, no studies have investigated exercise training's potential effects on the KYN pathway in metabolic or cardiovascular diseases (62, 96), despite strong evidence linking KYN dysregulation to conditions such as diabetes and atherosclerosis (97–100). Evidence in this regard only comes from preclinical studies showing positive results (101). Additionally, inflammation-driven diseases, such as HIV (102, 103) and long COVID disease (104, 105), warrant further exploration to determine whether exercise interventions could mitigate disease-related disruptions in KYN metabolism.
Several inconsistencies remain regarding the optimal exercise modalities and intensities required to induce meaningful changes in the KYN pathway (93, 106). High-intensity exercise appears more effective than moderate-intensity training, but further research is needed to establish standardized exercise prescriptions. Additionally, individual factors such as age, sex, genetic predisposition, and baseline inflammatory status likely influence exercise-induced TRP metabolism changes, necessitating a more personalized approach to exercise interventions.
One of the key limitations in this field is the methodological variability across studies in healthy and diseased populations. Differences in sample size, exercise prescription, and biomarker assessment methods contribute to inconsistent findings. Future studies should prioritize well-designed randomized controlled trials (RCTs) with standardized exercise protocols and rigorous analytical techniques to establish causal relationships between exercise and KYN pathway modulation. Additionally, incorporating multi-omics approaches, including transcriptomics and proteomics, could help identify novel regulatory mechanisms underlying exercise-induced metabolic adaptations.
From a clinical perspective, our findings underscore the potential for targeted exercise interventions to mitigate inflammation and neurotoxicity by modulating the KYN pathway. Personalized exercise prescriptions based on metabolic profiling could optimize therapeutic outcomes, and incorporating KYN biomarkers into clinical assessments may provide valuable insights into inflammatory and metabolic status (107, 108), guiding clinical decision-making (109). Moreover, structured exercise programs could serve as non-pharmacological strategies for managing chronic diseases characterized by KYN dysregulation.
5 Conclusions and future directions
The evidence suggests that exercise training plays a crucial role in modulating KYN pathway metabolism, particularly in individuals with chronic diseases characterized by low-grade inflammation (23). These conditions often drive KYN metabolism toward neurotoxic metabolites (12, 39), whereas exercise training promotes a shift toward the neuroprotective branch. This effect appears more pronounced in cancer patients due to elevated IDO activity, while findings in central nervous system disorders remain inconsistent, possibly due to methodological variations. Additionally, exercise volume and intensity seem to be key moderators of these benefits.
Despite promising results, few studies have explored exercise-induced KYN pathway adaptations in healthy adults. Additionally, most research has yet to establish direct links between KYN pathway changes and clinical outcomes (110). Future research should bridge this gap by integrating mechanistic insights with clinical relevance endpoints, particularly in metabolic, infectious, and cardiovascular diseases. Experimental models, including animal studies, could provide controlled conditions to help clarify dose-response relationship and underlying pathways. Understanding these mechanisms will enhance the therapeutic potential of exercise and refine its application in clinical settings. By addressing these challenges, future research can solidify the role of exercise in mitigating inflammation-driven neurotoxicity and advancing targeted interventions for vulnerable populations.
Data availability statement
The original contributions presented in the study are included in the article/Supplementary Material, further inquiries can be directed to the corresponding author.
Author contributions
MR: Conceptualization, Data curation, Formal Analysis, Investigation, Methodology, Resources, Visualization, Writing – original draft, Writing – review & editing. KL: Investigation, Writing – review & editing. XQ: Investigation, Writing – review & editing. JB: Conceptualization, Data curation, Formal Analysis, Funding acquisition, Investigation, Methodology, Project administration, Resources, Supervision, Validation, Visualization, Writing – original draft, Writing – review & editing.
Funding
The author(s) declare that financial support was received for the research, authorship, and/or publication of this article. This study was partially funded by the Fundação de Amparo à Pesquisa do Estado do Rio de Janeiro — FAPERJ (grant number E-26/200.132/2023, recipient JPB), the Conselho Nacional de Desenvolvimento Científico e Tecnológico — CNPq (grant number 404204/2023-6, recipient JPB), and the Coordenação de Aperfeiçoamento de Pessoal de Nível Superior — CAPES (Finance Code 001, recipient MVSR).
Conflict of interest
The authors declare that the research was conducted in the absence of any commercial or financial relationships that could be construed as a potential conflict of interest.
The author(s) declared that they were an editorial board member of Frontiers, at the time of submission. This had no impact on the peer review process and the final decision.
Generative AI statement
The author(s) declare that no Generative AI was used in the creation of this manuscript.
Publisher's note
All claims expressed in this article are solely those of the authors and do not necessarily represent those of their affiliated organizations, or those of the publisher, the editors and the reviewers. Any product that may be evaluated in this article, or claim that may be made by its manufacturer, is not guaranteed or endorsed by the publisher.
Supplementary material
The Supplementary Material for this article can be found online at: https://www.frontiersin.org/articles/10.3389/fspor.2025.1535152/full#supplementary-material
References
1. Hopkins FG, Cole SW. On the proteid reaction of Adamkiewicz, with contributions to the chemistry of glyoxylic acid. Proc R Soc Lond. (1901) 68:21–33. doi: 10.1098/rspl.1901.0008
2. Ellinger A, Flamand C. Uber die konstitution der indolgruppe im EiweiB. IV. Vor- laufige mitteilung. Synthese des racemischen tryptophans. Ber Dtsch Chem Ges. (1907) 40(3):3029–33. doi: 10.1002/cber.19070400353
3. Comai S, Bertazzo A, Brughera M, Crotti S. Tryptophan in health and disease. Adv Clin Chem. (2020) 95:165–218. doi: 10.1016/bs.acc.2019.08.005
4. Modoux M, Rolhion N, Mani S, Sokol H. Tryptophan metabolism as a pharmacological target. Trends Pharmacol Sci. (2021) 42(1):60–73. doi: 10.1016/j.tips.2020.11.006
5. Xue C, Li G, Zheng Q, Gu X, Shi Q, Su Y, et al. Tryptophan metabolism in health and disease. Cell Metab. (2023) 35(8):1304–26. doi: 10.1016/j.cmet.2023.06.004
6. Pathak S, Nadar R, Kim S, Liu K, Govindarajulu M, Cook P, et al. The influence of kynurenine metabolites on neurodegenerative pathologies. Int J Mol Sci. (2024) 25(2):853. doi: 10.3390/ijms25020853
7. Tsuji A, Ikeda Y, Yoshikawa S, Taniguchi K, Sawamura H, Morikawa S, et al. The tryptophan and kynurenine pathway involved in the development of immune-related diseases. Int J Mol Sci. (2023) 24(6):5742. doi: 10.3390/ijms24065742
8. Stone TW, Williams RO. Modulation of T cells by tryptophan metabolites in the kynurenine pathway. Trends Pharmacol Sci. (2023) 44(7):442–56. doi: 10.1016/j.tips.2023.04.006
9. Kearns R. Gut-brain axis and neuroinflammation: the role of gut permeability and the kynurenine pathway in neurological disorders. Cell Mol Neurobiol. (2024) 44(1):64. doi: 10.1007/s10571-024-01496-z
10. Polyzos KA, Ketelhuth DF. The role of the kynurenine pathway of tryptophan metabolism in cardiovascular disease. An emerging field. Hamostaseologie. (2015) 35(2):128–36. doi: 10.5482/HAMO-14-10-0052
11. Castro-Portuguez R, Sutphin GL. Kynurenine pathway, NAD(+) synthesis, and mitochondrial function: targeting tryptophan metabolism to promote longevity and healthspan. Exp Gerontol. (2020) 132:110841. doi: 10.1016/j.exger.2020.110841
12. Martin KS, Azzolini M, Lira Ruas J. The kynurenine connection: how exercise shifts muscle tryptophan metabolism and affects energy homeostasis, the immune system, and the brain. Am J Physiol Cell Physiol. (2020) 318(5):C818–C30. doi: 10.1152/ajpcell.00580.2019
13. Zhang J, Liu Y, Zhi X, Xu L, Tao J, Cui D, et al. Tryptophan catabolism via the kynurenine pathway regulates infection and inflammation: from mechanisms to biomarkers and therapies. Inflamm Res. (2024) 73(6):979–96. doi: 10.1007/s00011-024-01878-5
14. Joisten N, Kummerhoff F, Koliamitra C, Schenk A, Walzik D, Hardt L, et al. Exercise and the kynurenine pathway: current state of knowledge and results from a randomized cross-over study comparing acute effects of endurance and resistance training. Exerc Immunol Rev. (2020) 26:24–42.32139353
15. Seo SK, Kwon B. Immune regulation through tryptophan metabolism. Exp Mol Med. (2023) 55(7):1371–9. doi: 10.1038/s12276-023-01028-7
16. Ogbechi J, Clanchy FI, Huang YS, Topping LM, Stone TW, Williams RO. IDO Activation, inflammation and musculoskeletal disease. Exp Gerontol. (2020) 131:110820. doi: 10.1016/j.exger.2019.110820
17. Chaves Filho AJM, Lima CNC, Vasconcelos SMM, de Lucena DF, Maes M, Macedo D. IDO Chronic immune activation and tryptophan metabolic pathway: a potential pathophysiological link between depression and obesity. Prog Neuropsychopharmacol Biol Psychiatry. (2018) 80(Pt C):234–49. doi: 10.1016/j.pnpbp.2017.04.035
18. Capuron L, Geisler S, Kurz K, Leblhuber F, Sperner-Unterweger B, Fuchs D. Activated immune system and inflammation in healthy ageing: relevance for tryptophan and neopterin metabolism. Curr Pharm Des. (2014) 20(38):6048–57. doi: 10.2174/1381612820666140317110217
19. Salminen A. Role of indoleamine 2,3-dioxygenase 1 (IDO1) and kynurenine pathway in the regulation of the aging process. Ageing Res Rev. (2022) 75:101573. doi: 10.1016/j.arr.2022.101573
20. Ballesteros J, Rivas D, Duque G. The role of the kynurenine pathway in the pathophysiology of frailty, sarcopenia, and osteoporosis. Nutrients. (2023) 15(14):3132. doi: 10.3390/nu15143132
21. Réus GZ, Manosso LM, Quevedo J, Carvalho AF. Major depressive disorder as a neuro-immune disorder: origin, mechanisms, and therapeutic opportunities. Neurosci Biobehav Rev. (2023) 155:105425. doi: 10.1016/j.neubiorev.2023.105425
22. Zhao J, Chen J, Wang C, Liu Y, Li M, Li Y, et al. Kynurenine-3-monooxygenase (KMO) broadly inhibits viral infections via triggering NMDAR/Ca2 + influx and CaMKII IRF3-mediated IFN-β production. PLoS Pathog. (2022) 18(3):e1010366. doi: 10.1371/journal.ppat.1010366
23. Baumgartner R, Forteza MJ, Ketelhuth DFJ. The interplay between cytokines and the kynurenine pathway in inflammation and atherosclerosis. Cytokine. (2019) 122:154148. doi: 10.1016/j.cyto.2017.09.004
24. Gouasmi R, Ferraro-Peyret C, Nancey S, Coste I, Renno T, Chaveroux C, et al. The kynurenine pathway and cancer: why keep it simple when you can make it complicated. Cancers. (2022) 14(11):2793. doi: 10.3390/cancers14112793
25. Huang YS, Ogbechi J, Clanchy FI, Williams RO, Stone TW. IDO and kynurenine metabolites in peripheral and CNS disorders. Front Immunol. (2020) 11:388. doi: 10.3389/fimmu.2020.00388
26. Fernandes BS, Inam ME, Enduru N, Quevedo J, Zhao Z. The kynurenine pathway in Alzheimer’s disease: a meta-analysis of central and peripheral levels. Braz J Psychiatry. (2023) 45(3):286–97. doi: 10.47626/1516-4446-2022-2962
27. Zhai L, Ladomersky E, Lenzen A, Nguyen B, Patel R, Lauing KL, et al. IDO1 In cancer: a Gemini of immune checkpoints. Cell Mol Immunol. (2018) 15(5):447–57. doi: 10.1038/cmi.2017.143
28. Basson C, Serem JC, Hlophe YN, Bipath P. The tryptophan-kynurenine pathway in immunomodulation and cancer metastasis. Cancer Med. (2023) 12(18):18691–701. doi: 10.1002/cam4.6484
29. Hinkley JM, Yu GX, Standley RA, Distefano G, Tolstikov V, Narain NR, et al. Exercise and ageing impact the kynurenine/tryptophan pathway and acylcarnitine metabolite pools in skeletal muscle of older adults. J Physiol (Lond). (2023) 601(11):2165–88. doi: 10.1113/JP284142
30. Sulo G, Vollset SE, Nygard O, Midttun O, Ueland PM, Eussen SJ, et al. Neopterin and kynurenine-tryptophan ratio as predictors of coronary events in older adults, the Hordaland health study. Int J Cardiol. (2013) 168(2):1435–40. doi: 10.1016/j.ijcard.2012.12.090
31. Engin AB, Engin A. Tryptophan metabolism in obesity: the indoleamine 2,3-dioxygenase-1 activity and therapeutic options. Adv Exp Med Biol. (2024) 1460:629–55. doi: 10.1007/978-3-031-63657-8_21
32. Yu E, Ruiz-Canela M, Guasch-Ferre M, Zheng Y, Toledo E, Clish CB, et al. Increases in plasma tryptophan are inversely associated with incident cardiovascular disease in the prevencion con dieta mediterranea (PREDIMED) study. J Nutr. (2017) 147(3):314–22. doi: 10.3945/jn.116.241711
33. Melhem NJ, Taleb S. Tryptophan: from diet to cardiovascular diseases. Int J Mol Sci. (2021) 22(18):9904. doi: 10.3390/ijms22189904
34. Guillemin GJ. Quinolinic acid, the inescapable neurotoxin. FEBS J. (2012) 279(8):1356–65. doi: 10.1111/j.1742-4658.2012.08485.x
35. Nagy-Grócz G, Spekker E, Kynurenines VL. Neuronal excitotoxicity, and mitochondrial oxidative stress: role of the intestinal flora. Int J Mol Sci. (2024) 25(3):1698. doi: 10.3390/ijms25031698
36. Pearson K, Beier K, Mardis T, Munoz B, Zaidi A. The neurochemistry of depression: the good, the bad and the ugly. Mo Med. (2024) 121(1):68–75.38404431
37. Alves LF, Moore JB, Kell DB. The biology and biochemistry of kynurenic acid, a potential nutraceutical with multiple biological effects. Int J Mol Sci. (2024) 25(16):9082. doi: 10.3390/ijms25169082
38. Birch PJ, Grossman CJ, Hayes AG. Kynurenic acid antagonises responses to NMDA via an action at the strychnine-insensitive glycine receptor. Eur J Pharmacol. (1988) 154(1):85–7. doi: 10.1016/0014-2999(88)90367-6
39. Cervenka I, Agudelo LZ, Ruas JL. Kynurenines: tryptophan’s metabolites in exercise, inflammation, and mental health. Science. (2017) 357(6349):eaaf9794. doi: 10.1126/science.aaf9794
40. Lugo-Huitron R, Blanco-Ayala T, Ugalde-Muniz P, Carrillo-Mora P, Pedraza-Chaverri J, Silva-Adaya D, et al. On the antioxidant properties of kynurenic acid: free radical scavenging activity and inhibition of oxidative stress. Neurotoxicol Teratol. (2011) 33(5):538–47. doi: 10.1016/j.ntt.2011.07.002
41. Agudelo LZ, Ferreira DMS, Cervenka I, Bryzgalova G, Dadvar S, Jannig PR, et al. Kynurenic acid and Gpr35 regulate adipose tissue energy homeostasis and inflammation. Cell Metab. (2018) 27(2):378–92.e5. doi: 10.1016/j.cmet.2018.01.004
42. Zhen D, Liu J, Zhang XD, Song Z. Kynurenic acid acts as a signaling molecule regulating energy expenditure and is closely associated with metabolic diseases. Front Endocrinol. (2022) 13:847611. doi: 10.3389/fendo.2022.847611
43. Wang J, Simonavicius N, Wu X, Swaminath G, Reagan J, Tian H, et al. Kynurenic acid as a ligand for orphan G protein-coupled receptor GPR35. J Biol Chem. (2006) 281(31):22021–8. doi: 10.1074/jbc.M603503200
44. Joisten N, Walzik D, Metcalfe AJ, Bloch W, Zimmer P. Physical exercise as kynurenine pathway modulator in chronic diseases: implications for immune and energy homeostasis. Int J Tryptophan Res. (2020) 13:1178646920938688. doi: 10.1177/1178646920938688
45. Rothhammer V, Quintana FJ. The aryl hydrocarbon receptor: an environmental sensor integrating immune responses in health and disease. Nat Rev Immunol. (2019) 19(3):184–97. doi: 10.1038/s41577-019-0125-8
46. Grishanova AY, Perepechaeva ML. Kynurenic acid/AhR signaling at the junction of inflammation and cardiovascular diseases. Int J Mol Sci. (2024) 25(13):6933. doi: 10.3390/ijms25136933
47. Tóth F, Cseh EK, Vécsei L. Natural molecules and neuroprotection: kynurenic acid, pantethine and α-lipoic acid. Int J Mol Sci. (2021) 22(1):403. doi: 10.3390/ijms22010403
48. Ostapiuk A, Urbanska EM. Kynurenic acid in neurodegenerative disorders-unique neuroprotection or double-edged sword? CNS Neurosci Ther. (2022) 28(1):19–35. doi: 10.1111/cns.13768
49. Savitz J. The kynurenine pathway: a finger in every pie. Mol Psychiatry. (2020) 25(1):131–47. doi: 10.1038/s41380-019-0414-4
50. Huang Y, Zhao M, Chen X, Zhang R, Le A, Hong M, et al. Tryptophan metabolism in central nervous system diseases: pathophysiology and potential therapeutic strategies. Aging Dis. (2023) 14(3):858–78. doi: 10.14336/AD.2022.0916
51. Agudelo LZ, Femenía T, Orhan F, Porsmyr-Palmertz M, Goiny M, Martinez-Redondo V, et al. Skeletal muscle PGC-1α1 modulates kynurenine metabolism and mediates resilience to stress-induced depression. Cell. (2014) 159(1):33–45. doi: 10.1016/j.cell.2014.07.051
52. Schlittler M, Goiny M, Agudelo LZ, Venckunas T, Brazaitis M, Skurvydas A, et al. Endurance exercise increases skeletal muscle kynurenine aminotransferases and plasma kynurenic acid in humans. Am J Physiol Cell Physiol. (2016) 310(10):C836–40. doi: 10.1152/ajpcell.00053.2016
53. Valente-Silva P, Cervenka I, Ferreira DMS, Correia JC, Edman S, Horwath O, et al. Effects of tryptophan supplementation and exercise on the fate of kynurenine metabolites in mice and humans. Metabolites. (2021) 11(8):508. doi: 10.3390/metabo11080508
54. Agudelo LZ, Ferreira DMS, Dadvar S, Cervenka I, Ketscher L, Izadi M, et al. Skeletal muscle PGC-1α1 reroutes kynurenine metabolism to increase energy efficiency and fatigue-resistance. Nat Commun. (2019) 10(1):2767. doi: 10.1038/s41467-019-10712-0
55. Dumont KD, Jannig PR, Porsmyr-Palmertz M, Ruas JL. Constitutive loss of kynurenine-3-monooxygenase changes circulating kynurenine metabolites without affecting systemic energy metabolism. Am J Physiol Endocrinol Metab. (2025) 328(2):E274–E85. doi: 10.1152/ajpendo.00386.2024
56. Robbins RN, Kelleher JL, Vellanki P, O'Connor JC, Mascaro JS, Nocera JR, et al. Kynurenine metabolism as a mechanism to improve fatigue and physical function in postmenopausal breast cancer survivors following resistance training. J Funct Morphol Kinesiology. (2022) 7(2):45. doi: 10.3390/jfmk7020045
57. Pal A, Zimmer P, Clauss D, Schmidt ME, Ulrich CM, Wiskemann J, et al. Resistance exercise modulates kynurenine pathway in pancreatic cancer patients. Int J Sports Med. (2021) 42(1):33–40. doi: 10.1055/a-1186-1009
58. Javelle F, Bloch W, Knoop A, Guillemin GJ, Zimmer P. Toward a neuroprotective shift: eight weeks of high intensity interval training reduces the neurotoxic kynurenine activity concurrently to impulsivity in emotionally impulsive humans—a randomized controlled trial. Brain Behav Immun. (2021) 96:7–17. doi: 10.1016/j.bbi.2021.04.020
59. Metcalfe AJ, Koliamitra C, Javelle F, Bloch W, Zimmer P. Acute and chronic effects of exercise on the kynurenine pathway in humans—a brief review and future perspectives. Physiol Behav. (2018) 194:583–7. doi: 10.1016/j.physbeh.2018.07.015
60. Küster OC, Laptinskaya D, Fissler P, Schnack C, Zügel M, Nold V, et al. Novel blood-based biomarkers of cognition, stress, and physical or cognitive training in older adults at risk of dementia: preliminary evidence for a role of BDNF, Irisin, and the Kynurenine pathway. J Alzheimers Dis. (2017) 59(3):1097–111. doi: 10.3233/JAD-170447
61. Kamandulis S, Lukonaitiene I, Snieckus A, Brazaitis M, Mickevicius M, Cernych M, et al. Mood, cognitive function, and plasma kynurenine metabolites responses following severe changes in physical activity. Med Sci Sports Exerc. (2024) 56(10):2007–15. doi: 10.1249/MSS.0000000000003488
62. Paz GA, Rangel MV, Farias CL, Soares ALC, Langner E, Teixeira T, et al. Acute and chronic effects of physical exercise on atherosclerosis, kynurenine pathway, endothelial function and inflammation in patients with coronary artery disease: a clinical trial protocol. BMJ Open Sport Exerc Med. (2025) 11(1):e002432. doi: 10.1136/bmjsem-2024-002432
63. Dadvar S, Ferreira DMS, Cervenka I, Ruas JL. The weight of nutrients: kynurenine metabolites in obesity and exercise. J Intern Med. (2018) 284(5):519–33. doi: 10.1111/joim.12830
64. Page MJ, McKenzie JE, Bossuyt PM, Boutron I, Hoffmann TC, Mulrow CD, et al. The PRISMA 2020 statement: an updated guideline for reporting systematic reviews. BMJ. (2021) 372:n71. doi: 10.1136/bmj.n71
65. Katsukawa F. FITT principle of exercise in the management of lifestyle-related diseases. Clin Calcium. (2016) 26(3):447–51.26923984
66. Smart NA, Waldron M, Ismail H, Giallauria F, Vigorito C, Cornelissen V, et al. Validation of a new tool for the assessment of study quality and reporting in exercise training studies: TESTEX. Int J Evid Based Healthc. (2015) 13(1):9–18. doi: 10.1097/XEB.0000000000000020
67. Higgins JP, Altman DG, Gotzsche PC, Juni P, Moher D, Oxman AD, et al. The cochrane collaboration’s tool for assessing risk of bias in randomised trials. BMJ. (2011) 343:d5928. doi: 10.1136/bmj.d5928
68. Flemyng E, Moore TH, Boutron I, Higgins JP, Hróbjartsson A, Nejstgaard CH, et al. Using risk of bias 2 to assess results from randomised controlled trials: guidance from cochrane. BMJ Evid Based Med. (2023) 28(4):260–6. doi: 10.1136/bmjebm-2022-112102
69. Sánchez Chapul L, Pérez de la Cruz G, Ramos Chávez LA, Valencia León JF, Torres Beltrán J, Estrada Camarena E, et al. Characterization of redox environment and tryptophan catabolism through kynurenine pathway in military divers’ and swimmers’ serum samples. Antioxidants. (2022) 11(7):1223. doi: 10.3390/antiox11071223
70. Wyckelsma VL, Trepci A, Schwieler L, Venckunas T, Brazaitis M, Kamandulis S, et al. Vitamin C and E treatment blocks changes in kynurenine metabolism triggered by three weeks of sprint interval training in recreationally active elderly humans. Antioxidants. (2021) 10(9):1443. doi: 10.3390/antiox10091443
71. Pal A, Schneider J, Schlüter K, Steindorf K, Wiskemann J, Rosenberger F, et al. Different endurance exercises modulate NK cell cytotoxic and inhibiting receptors. Eur J Appl Physiol. (2021) 121(12):3379–87. doi: 10.1007/s00421-021-04735-z
72. Zimmer P, Schmidt ME, Prentzell MT, Berdel B, Wiskemann J, Kellner KH, et al. Resistance exercise reduces kynurenine pathway metabolites in breast cancer patients undergoing radiotherapy. Front Oncol. (2019) 9:962. doi: 10.3389/fonc.2019.00962
73. Herrstedt A, Bay ML, Simonsen C, Sundberg A, Egeland C, Thorsen-Streit S, et al. Exercise-mediated improvement of depression in patients with gastro-esophageal junction cancer is linked to kynurenine metabolism. Acta Oncol. (2019) 58(5):579–87. doi: 10.1080/0284186X.2018.1558371
74. Joisten N, Rademacher A, Warnke C, Proschinger S, Schenk A, Walzik D, et al. Exercise diminishes plasma neurofilament light chain and reroutes the kynurenine pathway in multiple sclerosis. Neurol Neuroimmunol Neuroinflammation. (2021) 8(3):e982. doi: 10.1212/NXI.0000000000000982
75. Bansi J, Koliamitra C, Bloch W, Joisten N, Schenk A, Watson M, et al. Persons with secondary progressive and relapsing remitting multiple sclerosis reveal different responses of tryptophan metabolism to acute endurance exercise and training. J Neuroimmunol. (2018) 314:101–5. doi: 10.1016/j.jneuroim.2017.12.001
76. Saran T, Turska M, Kocki T, Zawadka M, Zieliński G, Turski WA, et al. Effect of 4-week physical exercises on tryptophan, kynurenine and kynurenic acid content in human sweat. Sci Rep. (2021) 11(1):11092. doi: 10.1038/s41598-021-90616-6
77. Boßlau TK, Wasserfurth P, Reichel T, Weyh C, Palmowski J, Nebl J, et al. 12-week combined strength and endurance exercise attenuates CD8(+) T-cell differentiation and affects the kynurenine pathway in the elderly: a randomized controlled trial. Immun Ageing. (2023) 20(1):19. doi: 10.1186/s12979-023-00347-7
78. Isung J, Granqvist M, Trepci A, Huang J, Schwieler L, Kierkegaard M, et al. Differential effects on blood and cerebrospinal fluid immune protein markers and kynurenine pathway metabolites from aerobic physical exercise in healthy subjects. Sci Rep. (2021) 11(1):1669. doi: 10.1038/s41598-021-81306-4
79. Kondo T, Okada Y, Shizuya S, Yamaguchi N, Hatakeyama S, Maruyama K. Neuroimmune modulation by tryptophan derivatives in neurological and inflammatory disorders. Eur J Cell Biol. (2024) 103(2):151418. doi: 10.1016/j.ejcb.2024.151418
80. Mor A, Tankiewicz-Kwedlo A, Krupa A, Pawlak D. Role of kynurenine pathway in oxidative stress during neurodegenerative disorders. Cells. (2021) 10(7):1603. doi: 10.3390/cells10071603
81. Kang I, Theodoropoulos G, Wangpaichitr M. Targeting the kynurenine pathway: another therapeutic opportunity in the metabolic crosstalk between cancer and immune cells. Front Oncol. (2024) 14:1524651. doi: 10.3389/fonc.2024.1524651
82. Sun S, Hu F, Sang Y, Wang S, Liu X, Shi J, et al. Dysregulated tryptophan metabolism contributes to metabolic syndrome in Chinese community-dwelling older adults. BMC Endocr Disord. (2025) 25(1):7. doi: 10.1186/s12902-024-01826-8
83. Joisten N, Ruas JL, Braidy N, Guillemin GJ, Zimmer P. The kynurenine pathway in chronic diseases: a compensatory mechanism or a driving force? Trends Mol Med. (2021) 27(10):946–54. doi: 10.1016/j.molmed.2021.07.006
84. Hornyák L, Dobos N, Koncz G, Karányi Z, Páll D, Szabó Z, et al. The role of indoleamine-2,3-dioxygenase in cancer development, diagnostics, and therapy. Front Immunol. (2018) 9:151. doi: 10.3389/fimmu.2018.00151
85. Munn DH, Mellor AL. Indoleamine 2,3 dioxygenase and metabolic control of immune responses. Trends Immunol. (2013) 34(3):137–43. doi: 10.1016/j.it.2012.10.001
86. Cormie P, Zopf EM, Zhang X, Schmitz KH. The impact of exercise on cancer mortality, recurrence, and treatment-related adverse effects. Epidemiol Rev. (2017) 39(1):71–92. doi: 10.1093/epirev/mxx007
87. Hojman P. Exercise protects from cancer through regulation of immune function and inflammation. Biochem Soc Trans. (2017) 45(4):905–11. doi: 10.1042/BST20160466
88. Zimmer P, Joisten N, Schenk A, Bloch W. Impact of physical exercise on the kynurenine pathway in patients with cancer: current limitations and future perspectives. Acta Oncol. (2019) 58(8):1116–7. doi: 10.1080/0284186X.2019.1599139
89. Chen JY, Li CF, Kuo CC, Tsai KK, Hou MF, Hung WC. Cancer/stroma interplay via cyclooxygenase-2 and indoleamine 2,3-dioxygenase promotes breast cancer progression. Breast Cancer Res. (2014) 16(4):410. doi: 10.1186/s13058-014-0410-1
90. Schwarcz R, Bruno JP, Muchowski PJ, Wu HQ. Kynurenines in the mammalian brain: when physiology meets pathology. Nat Rev Neurosci. (2012) 13(7):465–77. doi: 10.1038/nrn3257
91. Fallarini S, Magliulo L, Paoletti T, de Lalla C, Lombardi G. Expression of functional GPR35 in human iNKT cells. Biochem Biophys Res Commun. (2010) 398(3):420–5. doi: 10.1016/j.bbrc.2010.06.091
92. Tiszlavicz Z, Németh B, Fülöp F, Vécsei L, Tápai K, Ocsovszky I, et al. Different inhibitory effects of kynurenic acid and a novel kynurenic acid analogue on tumour necrosis factor-α (TNF-α) production by mononuclear cells, HMGB1 production by monocytes and HNP1-3 secretion by neutrophils. Naunyn-Schmiedeberg’s Arch Pharmacol. (2011) 383(5):447–55. doi: 10.1007/s00210-011-0605-2
93. Kupjetz M, Patt N, Joisten N, Ueland PM, McCann A, Gonzenbach R, et al. Baseline inflammation but not exercise modality impacts exercise-induced kynurenine pathway modulation in persons with multiple sclerosis: secondary results from a randomized controlled trial. Int J Tryptophan Res. (2024) 17:11786469241284423. doi: 10.1177/11786469241284423
94. da Cunha LL, Feter N, Alt R, Rombaldi AJ. Effects of exercise training on inflammatory, neurotrophic and immunological markers and neurotransmitters in people with depression: a systematic review and meta-analysis. J Affect Disord. (2023) 326:73–82. doi: 10.1016/j.jad.2023.01.086
95. Lim A, Harijanto C, Vogrin S, Guillemin G, Duque G. Does exercise influence kynurenine/tryptophan metabolism and psychological outcomes in persons with age-related diseases? A systematic review. Int J Tryptophan Res. (2021) 14:1178646921991119. doi: 10.1177/1178646921991119
96. Al-Qahtani Z, Al-Kuraishy HM, Ali NH, Elewa YHA, Batiha GE. Kynurenine pathway in type 2 diabetes: role of metformin. Drug Dev Res. (2024) 85(5):e22243. doi: 10.1002/ddr.22243
97. Song P, Ramprasath T, Wang H, Zou MH. Abnormal kynurenine pathway of tryptophan catabolism in cardiovascular diseases. Cell Mol Life Sci. (2017) 74(16):2899–916. doi: 10.1007/s00018-017-2504-2
98. Kozieł K, Urbanska EM. Kynurenine pathway in diabetes mellitus-novel pharmacological target? Cells. (2023) 12(3):460. doi: 10.3390/cells12030460
99. Yang Y, Liu X, Liu X, Xie C, Shi J. The role of the kynurenine pathway in cardiovascular disease. Front Cardiovasc Med. (2024) 11:1406856. doi: 10.3389/fcvm.2024.1406856
100. Ala M, Eftekhar SP. The footprint of kynurenine pathway in cardiovascular diseases. Int J Tryptophan Res. (2022) 15:11786469221096643. doi: 10.1177/11786469221096643
101. Nori P, Haghshenas R, Aftabi Y, Akbari H. Comparison of moderate-intensity continuous training and high-intensity interval training effects on the Ido1-KYN-ahr axis in the heart tissue of rats with occlusion of the left anterior descending artery. Sci Rep. (2023) 13(1):3721. doi: 10.1038/s41598-023-30847-x
102. Sultana S, Elengickal A, Bensreti H, Belin de Chantemele E, McGee-Lawrence ME, Hamrick MW. The kynurenine pathway in HIV, frailty and inflammaging. Front Immunol. (2023) 14:1244622. doi: 10.3389/fimmu.2023.1244622
103. MacCann R, Landay AL, Mallon PWG. HIV And comorbidities—the importance of gut inflammation and the kynurenine pathway. Curr Opin HIV AIDS. (2023) 18(2):102–10. doi: 10.1097/COH.0000000000000782
104. Almulla AF, Thipakorn Y, Zhou B, Vojdani A, Paunova R, Maes M. The tryptophan catabolite or kynurenine pathway in long COVID disease: a systematic review and meta-analysis. Neuroscience. (2024) 563:268–77. doi: 10.1016/j.neuroscience.2024.10.021
105. Dehhaghi M, Heydari M, Panahi HKS, Lewin SR, Heng B, Brew BJ, et al. The roles of the kynurenine pathway in COVID-19 neuropathogenesis. Infection. (2024) 52(5):2043–59. doi: 10.1007/s15010-024-02293-y
106. Joisten N, Walzik D, Schenk A, Metcalfe AJ, Belen S, Schaaf K, et al. Acute exercise activates the AHR in peripheral blood mononuclear cells in an intensity-dependent manner. Am J Physiol Cell Physiol. (2024) 327(2):C438–C45. doi: 10.1152/ajpcell.00282.2024
107. Gaspar R, Halmi D, Demjan V, Berkecz R, Pipicz M, Csont T. Kynurenine pathway metabolites as potential clinical biomarkers in coronary artery disease. Front Immunol. (2021) 12:768560. doi: 10.3389/fimmu.2021.768560
108. Irimes MB, Tertis M, Bogdan D, Diculescu V, Matei E, Cristea C, et al. Customized flexible platform—starting point for the development of wearable sensor for the direct electrochemical detection of kynurenic acid in biological samples. Talanta. (2024) 280:126684. doi: 10.1016/j.talanta.2024.126684
109. Chen X, Xu D, Yu J, Song XJ, Li X, Cui YL. Tryptophan metabolism disorder-triggered diseases, mechanisms, and therapeutic strategies: a scientometric review. Nutrients. (2024) 16(19):3380. doi: 10.3390/nu16193380
Keywords: disease management, tryptophan, chronic disease, kynurenic acid, metabolism, exercise, kynurenine, health promotion
Citation: Rangel MVdS, Lopes KG, Qin X and Borges JP (2025) Exercise-induced adaptations in the kynurenine pathway: implications for health and disease management. Front. Sports Act. Living 7:1535152. doi: 10.3389/fspor.2025.1535152
Received: 26 November 2024; Accepted: 25 February 2025;
Published: 6 March 2025.
Edited by:
Veronica Perez de la Cruz, Manuel Velasco Suárez National Institute, MexicoReviewed by:
Mark Hamrick, Augusta University, United StatesMasaru Tanaka, University of Szeged, Hungary
Copyright: © 2025 Rangel, Lopes, Qin and Borges. This is an open-access article distributed under the terms of the Creative Commons Attribution License (CC BY). The use, distribution or reproduction in other forums is permitted, provided the original author(s) and the copyright owner(s) are credited and that the original publication in this journal is cited, in accordance with accepted academic practice. No use, distribution or reproduction is permitted which does not comply with these terms.
*Correspondence: Juliana Pereira Borges, anVsaXBib3JnZXNAZ21haWwuY29t