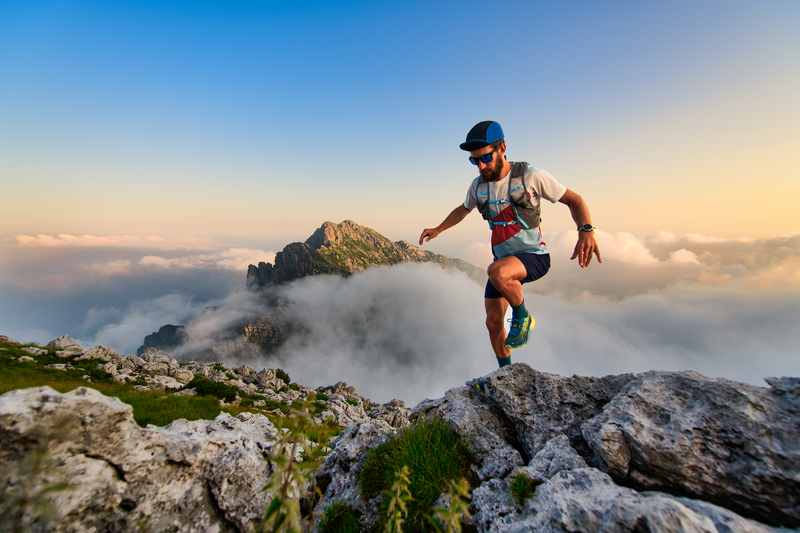
95% of researchers rate our articles as excellent or good
Learn more about the work of our research integrity team to safeguard the quality of each article we publish.
Find out more
ORIGINAL RESEARCH article
Front. Sports Act. Living , 16 November 2022
Sec. Elite Sports and Performance Enhancement
Volume 4 - 2022 | https://doi.org/10.3389/fspor.2022.982548
This article is part of the Research Topic Cycling: Performance Enhancement across the Disciplines View all 6 articles
Purpose: The main purpose of the current study was to investigate the dynamic adjustment of pulmonary oxygen uptake (O2) in response to moderate-intensity cycling on three occasions within 15 months in competitive youth cyclists. Furthermore, the muscle Δdeoxy[heme] on-kinetics and the Δdeoxy[heme]-to-O2 ratio were modeled to examine possible mechanistic basis regulating pulmonary O2 on-kinetics.
Methods: Eleven cyclists (initial age, 14.3 ± 1.6 y; peak O2, 62.2 ± 4.5 mL.min−1.kg−1) with a training history of 2–5 years and a training volume of ~10 h per week participated in this investigation. O2 and Δdeoxy[heme] responses during workrate-transitions to moderate-intensity cycling were measured with breath-by-breath spirometry and near-infrared spectroscopy, respectively, and subsequently modeled with mono-exponential models to derive parameter estimates. Additionally, a normalized Δdeoxy[heme]-to-O2 ratio was calculated for each participant. One-way repeated-measures ANOVA was used to assess effects of time on the dependent variables of the responses.
Results: The O2 time constant remained unchanged between the first (~24 s) and second visit (~22 s, P > 0.05), whereas it was significantly improved through the third visit (~13 s, P = 0.006–0.013). No significant effects of time were revealed for the parameter estimates of the Δdeoxy[heme] response (P > 0.05). A significant Δdeoxy[heme]-to-O2 ratio “overshoot” was evident on the first (1.09 ± 0.10, P = 0.006) and second (1.05 ± 0.09, P = 0.047), though not the third (0.97 ± 0.10, P > 0.05), occasion. These “overshoots” showed strong positive relationships with the O2 time constant during the first (r = 0.66, P = 0.028) and second visit (r = 0.76, P = 0.007). Further, strong positive relationships have been observed between the individual changes of the fundamental phase τp and the Δdeoxy[heme]-to-O2 ratio “overshoot” from occasion one to two (r = 0.70, P = 0.017), and two to three (r = 0.74, P = 0.009).
Conclusion: This suggests that improvements in muscle oxygen provision and utilization capacity both occurred, and each may have contributed to enhancing the dynamic adjustment of the oxidative “machinery” in competitive youth cyclists. Furthermore, it indicates a strong link between an oxygen maldistribution within the tissue of interrogation and the O2 time constant.
The dynamic response of pulmonary oxygen uptake (O2) following a square-wave transition from rest to moderate-intensity [i.e., below the gas exchange threshold (GET)] constant-workrate exercise is characterized by three phases (pulmonary O2 on-kinetics). The first increase in pulmonary O2 during phase I (i.e., cardiodynamic phase) is largely dictated by a fast increase in cardiac output; and hence, pulmonary blood flow during the first 15–20 s of the transition. The subsequent exponential increase during phase II (i.e., fundamental phase) drives the pulmonary O2 toward its projected steady-state (phase III) (1, 2). The fundamental phase is described by the time constant (τp), which (i) reflects the time to achieve 63% of the projected phase II response (3) and (ii) coincides within ~10% with a surrogate of muscular O2 (i.e., kinetics of muscle phosphocreatine breakdown) in children (4). Therefore, the fundamental phase τp can be used as a substitute of muscular O2 on-kinetics and provide useful information regarding the dynamic adjustment of the metabolic processes located in the working myocytes (3).
Pulmonary O2 on-kinetics during moderate-intensity exercise have been extensively studied in healthy and diseased adults, whereas data in (endurance trained) children and adolescents are limited (3, 5). Previous studies revealed no significant differences of the fundamental phase τp between prepubertal children and young adults (6–8), whereas more recent investigations showed smaller τp values (i.e., faster on-kinetics) in prepubertal children vs. young adults (9–12). Cross-sectional comparisons between endurance-trained and untrained youth reported either faster (13, 14) or similar (15) fundamental phase τp in the endurance-trained vs. untrained participants. However, to the best of the authors knowledge, no study has yet investigated longitudinal alterations of pulmonary O2 on-kinetics during moderate-intensity exercise in endurance trained youth. Furthermore, there has been considerable debate on the regulatory factors of the dynamic O2 response following a transition to moderate-intensity exercise between those favoring metabolic limitations and those supporting oxygen (O2) delivery limitations (16, 17). Technologies like portable near-infrared spectroscopy (NIRS) devices applied together with established methods (e.g., breath-by-breath spirometry) have been previously used to investigate muscle O2 delivery/utilization relationships [e.g., Δdeoxy[heme]-to-O2 ratio] in children /adolescents and adults (11, 13, 14, 18–20); and thus, have the potential to further strengthen the understanding of the mechanistic bases regulating (changes of) O2 on-kinetics (16, 21). For example, Marwood et al. (13) showed faster pulmonary O2 and capillary blood flow on-kinetics in trained vs. untrained adolescents, whereas no significant differences in Δdeoxy[heme] on-kinetics have been observed. The authors concluded that proportional enhancements in O2 delivery and utilization capacity determined the faster pulmonary O2 on-kinetics reported in the trained group (13). Further, Murias et al. (19, 20) revealed that training induced improvements of pulmonary O2 on-kinetics in adults are associated with a reduction of the Δdeoxy[heme]-to-O2 ratio; and thus, an improved balance between microvascular O2 distribution and local muscular O2 utilization.
The main purpose of the current study was to investigate changes of pulmonary O2 on-kinetics in response to moderate-intensity exercise in competitive youth cyclists over a period of 15 months, and to model Δdeoxy[heme] on-kinetics and the Δdeoxy[heme]-to-O2 ratio to examine possible mechanisms regulating (changes of) the adjustment of oxidative phosphorylation. We hypothesized a speeding over time of the pulmonary O2 on-kinetic response concomitant with no changes of the Δdeoxy[heme] on-kinetics. Additionally, we expected a reduction of the Δdeoxy[heme]-to-O2 “overshoot” with time and a positive relationship between (changes of) the Δdeoxy[heme]-to-O2 “overshoot” and fundamental phase τp for all occasions.
Eight male and three female youth cyclists with a training history of 2–5 years participated in the current investigation. All cyclists performed a regular endurance-training volume of ~10 h per week throughout the study duration, were members of the junior national team, attended a local sports high school, and regularly competed at national and international level competitions in road cycling, mountain bike XC, and track cycling. The cyclists were part of the same training group, and the whole training process was supervised by one experienced coach who followed a polarized training intensity distribution approach throughout the study duration. Prior to the study, the participants and their legal guardians were informed of the experimental procedures and gave written informed consent to participate. All documents and procedures were submitted to, and approved by, the institutional review board and the study was conducted in accordance with the Declaration of Helsinki.
Participants visited the laboratory twice within 2 weeks on three occasions within 15 months (Occasion 1: 1st month, Occasion 2: 8th month, Occasion 3: 15th month). Body mass and stature were measured with an electronic scale and stadiometer (Seca 813 and 213, Seca, Hamburg, Germany) and adipose tissue thickness (ATT) at the musculus vastus lateralis was determined using a skinfold caliper (Harpenden, Baty International, Burgess Hill, United Kingdom) before a graded ramp exercise test (GXT) was conducted during the first visit. On a subsequent visit, participants performed one square-wave transition from a baseline workrate to a workrate corresponding to the moderate-intensity domain. All tests were conducted on the participants own road bikes mounted to a Cyclus2 Ergometer (RBM Electronics, Leipzig, Germany). Participants were instructed to visit the laboratory in a fully rested state and to refrain from alcohol 24 h and caffeine 3 h prior to testing.
The GXT was conducted to determine peak workrate (Wpeak), peak oxygen consumption (O2peak), peak heart rate (HRpeak), and the GET and the respiratory compensation point (RCP). After a 3 min baseline at 40 W, the workrate increased at a rate of 20 W.min−1 until the limit of tolerance. Participants were asked to maintain a cadence between 90 and 100 rpm during the GXT. They breathed through a low-resistance impeller turbine mounted on a face mask to continuously measure gas exchange and pulmonary ventilation with a portable open circuit spirometry (MetaMax 3B, Cortex Biophysik, Leipzig, Germany). The gas analysers were calibrated with gases of known concentrations [15.99 Vol% oxygen (O2), 4.99 Vol% carbon dioxide (CO2), Cortex Biophysik, Leipzig, Germany] and air flow and volume were calibrated with a 3-L syringe (Type M 9474-C, Cortex Biophysik, Leipzig, Germany). O2peak, HRpeak and peak respiratory exchange ratio (RERpeak) were defined as the highest continuous 30 s average throughout the test. The V-slope method was used to determine the GET (22) which was subsequently visually verified by inspection of an increase of the ventilatory equivalent of O2, without a concomitant change of the ventilatory equivalent of CO2. RCP was determined as the first systematic decrease in end-tidal partial pressure of CO2 with a concomitant increase of the ventilatory equivalent of CO2. It was subsequently visually verified by inspection of the second disproportional increase in minute ventilation (23).
The square-wave transition was conducted to determine pulmonary O2 and local muscular deoxygenation on-kinetics. The required workrate for the square-wave transition was determined after the completion of the GXT as 90% GET. A 3 min baseline at 40 W was followed by a step increase in workrate to moderate-intensity for 6 min and a cool-down of 3 min at 40 W. Participants were asked to maintain a cadence between 90 and 100 rpm during the test. Pulmonary ventilation and gas exchange were continuously measured breath-by-breath as described above. Local muscular deoxygenation of the right m. vastus lateralis was determined using a multi-distance continuous-wave NIRS device (PortaMon, Artinis, Elst, The Netherlands). The NIRS probe was covered in a transparent household plastic film and tightly taped on the cleaned and shaved belly of the muscle, midway between the lateral epicondyle of the femur and the greater trochanter. The probe was further fixed with an elastic bandage and covered with a black hose to minimize movement artifacts and the influence of extraneous light sources, respectively. The NIRS device consisted of three photodiodes emitting light at a wavelength of 762 to 850 nm and a photon detector detecting photons emerging form the interrogated tissue. Light source-detector distances of 30, 35, and 40 mm enabled a penetration depth of 15–20 mm. The device utilized the modified Beer-Lambert law to calculate relative changes of the local tissue deoxygenation status. The Δdeoxy[heme] signal was used for the “physiological calibration” described in the following paragraph.
Following the completion of the square-wave transition protocol, a(n) ischemia/hyperaemia calibration was conducted to normalize the Δdeoxy[heme] signal to its maximal “physiological” range. For this purpose, participants laid down on a massage table in a supine position. A blood pressure cuff (Ulrich medical, Ulm, Germany) attached to a cuff inflator (heidi™ mein Tourniquet, Ulrich medical, Ulm, Germany) was placed proximally of the NIRS probe and inflated to a pressure of ~300 mmHg for 5 min followed by an instantaneous release of the pressure. The Δdeoxy[heme] plateau during the ischemic phase and the Δdeoxy[heme] minimum during the hyperaemic phase of the calibration represents 100 and 0% deoxygenation in the tissue interrogated by the NIRS device. This “physiological calibration” allows the obtainment of “semiquantitative” tissue deoxygenation indices and thus the comparison between participants with differing [heme] and/or adipose tissue thickness (21). As suggested previously, this normalized Δdeoxy[heme] signal was used for further analysis (21).
The pulmonary breath-by-breath O2 data were filtered by removing aberrant breaths that lay outside more than four standard deviations (SD) of the local mean of five data points. The filtered data then were linearly interpolated to receive second-by-second data. These 1-s interpolated data were time-aligned that time zero represents the onset of exercise for each individual. Data of the first 15 s of the square-wave transition were excluded from the analysis to account for the cardiodynamic phase (24, 25), and a mono-exponential model was applied to model the fundamental phase of the pulmonary O2 on-kinetics (Equation. 1).
where O2 (t) represents the pulmonary O2 at a given time t, BL is defined as the mean pulmonary O2 between −60 and −10 s of baseline cycling, Ap is considered as the steady-state increase of pulmonary O2 above BL, TDp is the time delay relative to the onset of exercise and τp represents the pulmonary O2 time constant. The data were modeled from 15 s to the end of the exercise. The parameter estimates were subsequently estimated by least-squares non-linear regression analysis (GraphPad Prism 9.1.2, GraphPad Software Inc., San Diego, USA).
The normalized Δdeoxy[heme] data were averaged to 1-s bins and left-shifted that time zero represents the onset of exercise and subsequently modeled with a mono-exponential model (Equation 2). The start of the exponential increase was identified as the time at which the Δdeoxy[heme] signal started to systematically increase by one SD above baseline (18). Data were fitted up to 140 s, or, where a Δdeoxy[heme] overshoot relative to end-exercise was identified visually, to the peak value of this overshoot (18).
where Δdeoxy[heme] (t), Am, TDm, and τm represent the tissue deoxygenation status at any time t, the asymptotic amplitude, the time delay and the time constant of the Δdeoxy[heme] response, respectively. The MRTm was calculated as the sum of TDm and τm.
In addition to the on-kinetic responses, a normalized Δdeoxy[heme]-to-O2 ratio was derived from the actual data profiles of pulmonary O2 and Δdeoxy[heme] for each individual. A ratio of 1.00 represents a steady-state value between O2 delivery and utilization, whereas an “overshoot” beyond values of 1.00 indicates a slower adjustment of microvascular O2 delivery in proportion to the O2 demand; and hence, is thought to represent a temporary maldistribution of O2 within the working muscles (17, 19, 26, 27). Briefly, the second-by-second pulmonary O2 and Δdeoxy[heme] data were normalized that 0 % corresponds to the baseline values and 100% reflects the steady-state response of pulmonary O2 and Δdeoxy[heme]. To account for the cardiodynamic phase, the normalized pulmonary O2 data were time-aligned that time zero represents the onset of the fundamental phase of the pulmonary O2 response. Subsequently, the data were averaged to 5-s bins and a mean normalized Δdeoxy[heme]-to-O2 ratio was calculated for each individual from 15 to 120 s (26). The start and end point of 15 and 120 s coincide with the start of the ratio “overshoot” and the point at which all participants Δdeoxy[heme] and pulmonary O2 responses reached their amplitude, respectively.
Descriptive data are presented as mean ± SD. Shapiro-Wilk and Mauchly tests were used to examine assumptions of normality and sphericity, respectively. One-way repeated-measures ANOVA were used to determine possible effects of time on the dependent variables of the pulmonary O2 and Δdeoxy[heme] on-kinetic responses and the results of the GXT. Bonferroni correction was used for pairwise comparisons where appropriate. T-tests were applied to assess a significant “overshoot” (i.e., >1.00) of the normalized Δdeoxy[heme]-to-O2 ratio (one sample t-test). Pearson's product moment correlations were used to determine the relationship between the normalized Δdeoxy[heme]-to-O2 ratio and the fundamental phase τp. All statistical and graphical analyses were performed using IBM SPSS Statistics 26 (SPSS Inc., Chicago, IL, USA) and GraphPad Prism 9.1.2 (GraphPad Software Inc., San Diego, CA, USA), respectively. The level of statistical significance was set at P ≤ 0.05.
Participants characteristics and results of the GXT are presented in Table 1. The one-way repeated-measures ANOVA revealed significant effects of time on stature [F(1.14,11.43) = 10.579, P = 0.006], body mass [F(1.14,11.41) = 11.284, P = 0.005], Wpeak [F(1.22,12.24) = 9.119, P = 0.008], the workrate corresponding to 90% GET [F(1.18,11.81) = 6.996, P = 0.018] and RCP [F(1.16,11.56) = 13.685, P = 0.003], absolute O2 at GET [F(1.29,12.91) = 7.122, P = 0.015] and RCP [F(1.22,12.15) = 8.189, P = 0.011], relative O2 at RCP [F(2,20) = 8.398, P = 0.002], and RERpeak [F(2,20) = 4.218, P = 0.030], whereas no significant effect of time was reported on the remaining parameters (P = 0.116–0.724). The pairwise comparisons revealed increases in stature and body mass from the first to the second (P = 0.002 and 0.005 for stature and body mass, respectively) and third occasion (P = 0.011 and 0.008 for stature and body mass, respectively). Further, Wpeak increased from occasion one to two (P = 0.004) and three (P = 0.022), and absolute O2 at GET and RCP increased from occasion two compared to three (P = 0.035 and P = 0.029, respectively). Workrate at RCP increased from occasion one to two (P = 0.006) and three (P = 0.006), and from occasion two to three (P = 0.038), while relative O2 at RCP increased from occasions one/two compared to three (P = 0.045 and P = 0.027, respectively). Furthermore, pairwise comparisons revealed a significant difference between RERpeak at occasion one and two (P = 0.005). However, no significant differences in workrates corresponding to 90% GET have been found between any test occasions (P = 0.054–0.316).
Table 1. Participants characteristics and results of the graded ramp exercise test as mean ± SD (n = 11).
Figure 1 shows representative plots of the pulmonary O2 [panel (A,C,E)] and muscular Δdeoxy[heme] on-kinetics [panel (B,D,F)] from one participant. A significant effect of time was revealed for the fundamental phase τp [F(2,20) = 9.776, P = 0.001] of the pulmonary O2 on-kinetic response (Table 2). Post-hoc tests showed that the fundamental phase τp was significantly smaller (i.e., faster) on occasion three (12.9 ± 4.8 s) compared with occasion one (24.2 ± 6.6 s, P = 0.006) and occasion two (21.7 ± 6.0 s, P = 0.013). The one-way ANOVA revealed no significant effect of time for all parameter estimates describing the muscular Δdeoxy[heme] on-kinetic response to a moderate-intensity square-wave transition (P = 0.111–0.671; Table 2).
Figure 1. Representative plots of pulmonary O2 [open circles on (A,C,E)] and muscular Δdeoxy[heme] on-kinetics [open circles on (B,D,F)] from one participant on occasion one, two, and three. Dotted lines represent baselines where pulmonary O2 and Δdeoxy[heme] is zero. Solid lines and small full circles with connective lines represent the exponential curve fits and residuals, respectively.
Table 2. Pulmonary 2 and Δdeoxy[heme] on-kinetic parameter in response to a square-wave transition to the moderate-intensity domain as mean ± SD (n = 11).
A significant effect of time on the normalized Δdeoxy[heme]-to-O2 ratio was revealed [F(2,20) = 4.717, P = 0.021]. Post-hoc tests showed that the ratio was lower on occasion three compared to one (P = 0.021). The normalized Δdeoxy[heme]-to-O2 ratio was significantly higher than 1.00 on test occasion one (1.09 ± 0.10, P = 0.006) and two (1.05 ± 0.09, P = 0.047), whereas it was not significantly higher on occasion three (0.97 ± 0.10, P = 0.151; Table 2). The Δdeoxy[heme]-to-O2 ratio showed a strong positive relationship with the fundamental phase τp on test occasion one (r = 0.66, P = 0.028) and two (r = 0.76, P = 0.007), though this relationship was not significant on occasion three (r = 0.40, P = 0.220; Figures 2A–C). Further, a strong positive relationship was observed between the change of the fundamental phase τp and the Δdeoxy[heme]-to-O2 ratio from occasion one to two (r = 0.70, P = 0.017), and two to three (r = 0.74, P = 0.009; Figures 2D,E).
Figure 2. Relationship between the normalized Δdeoxy[heme]-to-O2 ratio and fundamental phase τp for all three occasions (A–C), and between changes of the normalized Δdeoxy[heme]-to-O2 ratio and fundamental phase τp over time (D,E). Dotted lines represent 95% confidence bands. O2, pulmonary oxygen uptake; τp, time constant of pulmonary oxygen uptake; r, coefficient of correlation.
The present study examined longitudinal changes in pulmonary O2 and Δdeoxy[heme] on-kinetics, and the Δdeoxy[heme]-to-O2 ratio in response to moderate-intensity exercise in trained youth cyclists over a period of 15 months. The main findings were: (i) Partially in line with our hypothesis, the fundamental phase τp showed no significant change from the first to the second visit, whereas τp decreased significantly from the first/second to the third visit. (ii) In line with our hypothesis, no significant changes of the Δdeoxy[heme] on-kinetic parameter estimates were observed during the current investigation. (iii) A transient Δdeoxy[heme]-to-O2 overshoot relative to the steady-state value of ~1.00 was present on test occasion one and two, whereas this overshoot was abolished on occasion three. (iv) A strong positive relationship between the Δdeoxy[heme]-to-O2 ratio overshoot and the fundamental phase τp was revealed during the first and second visit, though this relationship was attenuated during the third visit. (v) A strong positive correlation was observed between the change of the fundamental phase τp and the Δdeoxy[heme]-to-O2 ratio from occasion one to two, and two to three.
The fundamental phase τp reported on test occasions one and two (~24 and ~22 s, respectively) are in line with previous investigations in endurance-trained adolescents of similar age (~22–26 s) (13, 14). However, the τp reported on test occasion three (~13 s) is well below these values (i.e., faster) and coincides with O2 on-kinetics found in well- to highly-trained adult cyclists, rowers or runners (28–33), and a Belgian Junior cycling champion (3). Due to the lack of a control group in the present investigation, it is difficult to interpret whether the observed speeding of the fundamental phase τp may be attributed to the endurance training performed by the youth cyclists. Previous studies have shown that the fundamental phase τp is either faster (9–12) or similar (6–8) in untrained prepubertal children compared with untrained young adults. Thus, it seems likely to suggest that the herein reported speeding of the O2 on-kinetic response may be largely ascribed to the endurance training performed by the youth cyclists. The notion of a trainable on-kinetic response in youth is further supported by investigations revealing faster pulmonary O2 on-kinetics in trained vs. untrained youth (13, 14).
The time course for the dynamic adjustment of the Δdeoxy[heme] signal (i.e., τm and MRTm) remained constant throughout the study. This in in line with previous cross-sectional studies reporting no significant differences in Δdeoxy[heme] on-kinetics between trained and untrained adolescents (13, 14). In concert with the speeding of the fundamental phase τp, this indicates a proportional enhancement of microvascular O2 provision and O2 utilization capacities (13, 14) between test occasion one/two and three herein. This is supported by studies showing faster heart rate on-kinetics, indicative of an enhanced bulk blood flow, in trained vs. untrained prepubertal children (13), and an increase in muscle oxidative capacity in response to endurance training in youth (34, 35). However, it is noteworthy to mention that an elevated bulk blood flow does not ultimately mean that there was a faster local O2 distribution. Overall, it may be suggested that improvements in local muscular O2 distribution and O2 utilization capacities both occurred, and each may have contributed to improving the pulmonary O2 on-kinetic response observed herein. Again, due to the lack of a control group it is difficult to interpret whether these adaptations may be attributed to exercise training. However, since previous studies reported a higher percentage of type I muscle fibers (36) and faster capillary blood flow kinetics (11) in male children/adolescents compared to adults, and an elevated oxidative enzyme content (37) in male and female adolescents compared to adults, it seems appropriate to associate the above-mentioned adaptations with the exercise training performed by the youth cyclists in the current study.
The Δdeoxy[heme] signal showed a TDm of ~7–10 s during the early phase of the transient which was not affected by time in the present investigation. This is in line with previous investigations showing similar TDm values (~7–9 s) in adolescents which were not affected by training status and/or age (11, 13, 14). The steady Δdeoxy[heme] signal during the early phase of the exercise transition suggests a precise matching of local O2 distribution to utilization in the area of interrogation (16). This notion is in line with studies showing a similar pattern of O2 distribution/utilization indices (i.e., intracellular PO2, arterio-venous O2 difference) in animal myofiber preparations (38–40) and human limbs (41). Since muscle O2 increases immediately after the onset of exercise (41, 42), a concomitant instant increase in local O2 distribution is mandatory to preserve this early “steady-state.” Such a rapid increase in capillary blood flow; and thus, microvascular O2 delivery, has been previously shown early during the transient (43, 44). Together, this indicates intracellular mechanisms other than regional O2 maldistribution to constrain the adjustment of oxidative phosphorylation during the first ~10 s of the transient.
Following this early “homeostasis” between microvascular O2 provision and O2 demand within the working myofibers, Δdeoxy[heme] increased exponentially, and a Δdeoxy[heme]-to-O2 overshoot was evident on test occasion one and two, though not on occasion three. The Δdeoxy[heme]-to-O2 overshoot has been previously interpreted as a greater reliance on O2 extraction in proportion to the O2 demand within the muscle tissue (17, 19, 26, 27). Together, this indicates that on average a temporal mismatch between local O2 distribution and O2 demand following the first ~10 s after exercise onset is evident on occasion one and two, though not three. A mitigated or abrogated Δdeoxy[heme]-to-O2 overshoot indicates a reduced reliance on O2 extraction and thus, a more precise matching between microvascular O2 provision and utilization within the tissue of interrogation (17, 19, 26, 27). This may result in a less pronounced fall in microvascular PO2; and hence, an elevated driving force regulating the capillary-to-myocyte O2 flux resulting in a higher potential for oxidative phosphorylation during the transition (45, 46). This is supported by: (i) The strong positive relationships observed between the extent of the Δdeoxy[heme]-to-O2 overshoot and the fundamental phase τp on occasion one and two (Figures 2A–C), and by the fast τp observed on occasion three where the Δdeoxy[heme]-to-O2 overshoot and hence, an O2 maldistribution, was abrogated. (ii) The strong positive correlation between the change of the Δdeoxy[heme]-to-O2 ratio and the fundamental phase τp from occasion one to two, and two to three (Figures 2D,E).
One limitation resides in the NIRS measurement per-se (e.g., probe placement, small tissue of interrogation). To at least partially counteract these issues, we implemented a standardized operating procedure regarding probe placement to minimize the influence of spatial heterogeneities within the tissue of interest and followed the specific recommendations recently stated by Barstow (21). Limitations related to the modeling of the Δdeoxy[heme]-to-O2 ratio have been discussed extensively elsewhere (17, 21, 26). Briefly, modeling simulations revealed that the currently used method is rather conservative in estimating the “overshoot;” and hence, conclusions would have been unaffected by using another modeling approach (26). The use of only one exercise transition may be considered as a further limitation. Recent studies have shown that multiple transitions increase the confidence in the parameter estimates of the O2 and Δdeoxy[heme] on-kinetics (25, 47) and thus, decrease the smallest change detectable with confidence. However, the herein reported 95% confidence intervals for the fundamental phase τp (~4–5 s) and τm (~2–4 s) are within acceptable boundaries (13, 14), and the mean ~8–11 s decrease in τp between occasion one/two and three is at least similar to the smallest change detectable with confidence in youth by using one exercise transition (47). The lack of a control group may be considered another limitation. However, since previous investigations showed a slowing or no change of the pulmonary O2 on-kinetic response with aging, and a lower potential for oxidative metabolism (e.g., % type I fibers and/or oxidative enzyme content) in adults vs. youth [for review see: (5)] it seems appropriate to attribute the speeding of the pulmonary O2 on-kinetic response reported herein to the endurance-training performed by the youth cyclists.
The data of the current investigation in competitive youth cyclists showed that the fundamental phase τp and hence, muscle O2 on-kinetics, was not affected by time from the first to the second, though from the first/second to the third visit. Concomitant with the unchanged Δdeoxy[heme] on-kinetics, this indicates a proportional improvement in muscle O2 distribution and O2 utilization capacity between the second and third visit, and both may have contributed to improve the pulmonary O2 on-kinetic response observed herein. Furthermore, the data presented herein indicate a strong link between an O2 maldistribution within the tissue of interrogation evident during exercise transitions on occasion one and two, and the fundamental phase τp in trained youth cyclists.
The raw data supporting the conclusions of this article will be made available by the authors, without undue reservation.
The studies involving human participants were reviewed and approved by Review Board, University of Applied Sciences Wiener Neustadt, Wiener Neustadt, Austria. Written informed consent to participate in this study was provided by the participants' legal guardian/next of kin.
AN conceived and designed the research. BP, CR, MH, and MZ conducted the experiments. DS, MH, and MZ analyzed the data. DS and MH interpreted the results of the experiments. AN, BP, and MH drafted the manuscript. All authors were involved in the revision and approval of the final version of the manuscript.
This research was supported by Gesellschaft für Forschungsförderung Niederösterreich m.b.H. (Grant No. SC18-014).
The authors declare that the research was conducted in the absence of any commercial or financial relationships that could be construed as a potential conflict of interest.
All claims expressed in this article are solely those of the authors and do not necessarily represent those of their affiliated organizations, or those of the publisher, the editors and the reviewers. Any product that may be evaluated in this article, or claim that may be made by its manufacturer, is not guaranteed or endorsed by the publisher.
1. Whipp B, Ward S, Lamarra N, Davis J, and Wasserman K. Parameters of ventilatory and gas exchange during exercise. J Appl Physiol. (1982) 52:1506–13. doi: 10.1152/jappl.1982.52.6.1506
2. Whipp BJ, and Wasserman K. Oxygen uptake kinetics for various intensities of constant-load work. J Appl Physiol. (1972) 33:351–6. doi: 10.1152/jappl.1972.33.3.351
3. Poole DC, and Jones AM. Oxygen uptake kinetics. Compr Physiol. (2012) 2:933–96. doi: 10.1002/cphy.c100072
4. Barker AR, Welsman JR, Fulford J, Welford D, Williams CA, and Armstrong N. Muscle phosphocreatine and pulmonary oxygen uptake kinetics in children at the onset and offset of moderate intensity exercise. Eur J Appl Physiol. (2008) 102:727–38. doi: 10.1007/s00421-007-0650-1
5. McNarry MA. Oxygen uptake kinetics in youth: characteristics, interpretation, and application. Pediatr Exerc Sci. (2019) 31:175–83. doi: 10.1123/pes.2018-0177
6. Springer C, Barstwo TJ, Wassermann K, and Cooper DM. Oxygen uptake and heart rate responses during hypoxic exercise in children and adults. Med Sci Sports Exer. (1991) 23:71–9. doi: 10.1249/00005768-199101000-00012
7. Williams CA, Carter H, Jones AM, and Doust JH. Oxygen uptake kinetics during treadmill running in boys and men. J Appl Physiol. (2001) 90:1700–6. doi: 10.1152/jappl.2001.90.5.1700
8. Cooper DM, Berry C, Lamarra N, and Wasserman K. Kinetics of oxygen uptake and heart rate at onset of exercise in children. J Appl Physiol. (1985) 59:211–7. doi: 10.1152/jappl.1985.59.1.211
9. Armon Y, Cooper D, Flores R, Zanconato S, and Barstow T. Oxygen uptake dynamics during high-intensity exercise in children and adults. J Appl Physiol. (1991) 70:841–8. doi: 10.1152/jappl.1991.70.2.841
10. Fawkner SG, Armstrong N, Potter CR, and Welsman JR. Oxygen uptake kinetics in children and adults after the onset of moderate-intensity exercise. J Sports Sci. (2002) 20:319–26. doi: 10.1080/026404102753576099
11. Leclair E, Berthoin S, Borel B, Thevenet D, Carter H, Baquet G, et al. Faster pulmonary oxygen uptake kinetics in children vs. adults due to enhancements in oxygen delivery and extraction. Scand J Med Sci Sports. (2013) 23:705–12. doi: 10.1111/j.1600-0838.2012.01446.x
12. Breese BC, Barker AR, Armstrong N, Jones AM, and Williams CA. The effect of baseline metabolic rate on pulmonary O2 uptake kinetics during very heavy intensity exercise in boys and men. Respir Physiol Neurobiol. (2012) 180:223–9. doi: 10.1016/j.resp.2011.11.013
13. Marwood S, Roche D, Rowland T, Garrard M, and Unnithan VB. Faster pulmonary oxygen uptake kinetics in trained vs. untrained male adolescents. Med Sci Sports Exer. (2010) 42:127–34. doi: 10.1249/MSS.0b013e3181af20d0
14. Unnithan VB, Roche DM, Garrard M, Holloway K, and Marwood S. Oxygen uptake kinetics in trained adolescent females. Eur J Appl Physiol. (2015) 115:213–20. doi: 10.1007/s00421-014-3005-8
15. Cleuziou C, Lecoq AM, Candau R, Courteix D, Guenon P, and Obert P. Kinetics of oxygen uptake at the onset of moderate and heavy exercise in trained and untrained prepubertal children. Sci Sports. (2002) 17:291–6. doi: 10.1016/S0765-1597(02)00169-7
16. Poole DC, Barstow TJ, McDonough P, and Jones AM. Control of oxygen uptake during exercise. Med Sci Sports Exerc. (2008) 40:462–74. doi: 10.1249/MSS.0b013e31815ef29b
17. Murias JM, Spencer MD, and Paterson DH. The critical role of O2 provision in the dynamic adjustment of oxidative phosphorylation. Exerc Sport Sci Rev. (2014) 42:4–11. doi: 10.1249/JES.0000000000000005
18. Breese BC, Saynor ZL, Barker AR, Armstrong N, and Williams CA. Relationship between (non)linear phase ii pulmonary oxygen uptake kinetics with skeletal muscle oxygenation and age in 11-15 year olds. Exp Physiol. (2019) 104:1929–41. doi: 10.1113/EP087979
19. Murias JM, Kowalchuk JM, and Paterson DH. Speeding of Vo2 kinetics with endurance training in old and young men is associated with improved matching of local O2 delivery to muscle O2 utilization. J Appl Physiol. (2010) 108:913–22. doi: 10.1152/japplphysiol.01355.2009
20. Murias JM, Kowalchuk JM, and Paterson DH. Speeding of Vo2 kinetics in response to endurance-training in older and young women. Eur J Appl Physiol. (2011) 111:235–43. doi: 10.1007/s00421-010-1649-6
21. Barstow TJ. Understanding near infrared spectroscopy and its application to skeletal muscle research. J Appl Physiol. (2019) 126:1360–76. doi: 10.1152/japplphysiol.00166.2018
22. Beaver WL, Wasserman K, and Whipp BJ. A new method for detecting anaerobic threshold by gas exchange. J Appl Physiol. (1986) 60:2020–7. doi: 10.1152/jappl.1986.60.6.2020
23. Ahmaidi S, Hardy JM, Varray A, Collomp K, Mercier J, and Préfaut C. Respiratory gas exchange indices used to detect the blood lactate accumulation threshold during an incremental exercise test in young athletes. Eur J Appl Physiol Occup Physiol. (1993) 66:31–6. doi: 10.1007/BF00863396
24. Hebestreit H, Hughson R, and Bar-Or O. Kinetics of oxygen uptake at the onset of exercise in boys and men. J Appl Physiol. (1998) 85:1833–41. doi: 10.1152/jappl.1998.85.5.1833
25. Benson AP, Bowen TS, Ferguson C, Murgatroyd SR, and Rossiter HB. Data collection, handling, and fitting strategies to optimize accuracy and precision of oxygen uptake kinetics estimation from breath-by-breath measurements. J Appl Physiol. (2017) 123:227–42. doi: 10.1152/japplphysiol.00988.2016
26. Murias JM, Spencer MD, Kowalchuk JM, and Paterson DH. Muscle deoxygenation to vo2 relationship differs in young subjects with varying Tvo2. Eur J Appl Physiol. (2011) 111:3107–18. doi: 10.1007/s00421-011-1937-9
27. Murias JM, Spencer MD, Delorey DS, Gurd BJ, Kowalchuk JM, and Paterson DH. Speeding of Vo2 kinetics during moderate-intensity exercise subsequent to heavy-intensity exercise is associated with improved local O2 distribution. J Appl Physiol. (2011) 111:1410–5. doi: 10.1152/japplphysiol.00607.2011
28. Jones AM, Kirby BS, Clark IE, Rice HM, Fulkerson E, Wylie LJ, et al. Physiological demands of running at 2-hour marathon race pace. J Appl Physiol. (2021) 130:369–79. doi: 10.1152/japplphysiol.00647.2020
29. Koppo K, Bouckaert J, and Jones AM. Effects of training status and exercise intensity on phase II Vo2 kinetics. Med Sci Sports Exerc. (2004) 36:225–32. doi: 10.1249/01.MSS.0000113473.48220.20
30. Ingham SA, Carter H, Whyte GP, and Doust JH. Comparison of the oxygen uptake kinetics of club and olympic champion rowers. Med Sci Sports Exerc. (2007) 39:865–71. doi: 10.1249/mss.0b013e31803350c7
31. Berger NJ, and Jones AM. Pulmonary O2 uptake on-kinetics in sprint- and endurance-trained athletes. Appl Physiol Nutr Metabol. (2007) 32:383–93. doi: 10.1139/H06-109
32. Inglis EC, Iannetta D, and Murias J. Association between Vo2 kinetics and Vo2max in groups differing in fitness status. Eur J Appl Physiol. (2021) 21:6. doi: 10.1007/s00421-021-04623-6
33. Kilding AE, Winter EM, and Fysh M. A comparison of pulmonary oxygen uptake kinetics in middle- and long-distance runners. Int J Sports Med. (2006) 27:419–26. doi: 10.1055/s-2005-865778
34. Fournier M, Ricci J, Taylor AW, Ferguson RJ, Montpetit RR, and Chaitman BR. Skeletal muscle adaptation in adolescent boys: sprint and endurance training and detraining. Med Sci Sports Exerc. (1982) 14:453–6. doi: 10.1249/00005768-198206000-00008
35. Eriksson BO, Gollnick PD, and Saltin B. Muscle metabolism and enzyme activities after training in boys 11–13 years old. Acta Physiol Scand. (1973) 87:485–97. doi: 10.1111/j.1748-1716.1973.tb05415.x
36. Glenmark B, Hedberg G, and Jansson E. Changes in muscle fibre type from adolescence to adulthood in women and men. Acta Physiol Scand. (1992) 146:251–9. doi: 10.1111/j.1748-1716.1992.tb09414.x
37. Haralambie G. Enzyme activities in skeletal muscle of 13-15 years old adolescents. Bull Eur Physiopathol Respir. (1982) 18:65–74.
38. Behnke BJ, Kindig CA, Musch TI, Koga S, and Poole DC. Dynamics of microvascular oxygen pressure across the rest-exercise transition in rat skeletal muscle. Respir Physiol. (2001) 126:53–63. doi: 10.1016/S0034-5687(01)00195-5
39. Kindig CA, Howlett RA, and Hogan MC. Effect of extracellular Po2 on the fall in intracellular Po2 in contracting single myocytes. J Appl Physiol. (2003) 94:1964–70. doi: 10.1152/japplphysiol.00893.2002
40. Grassi B, Hogan M, Greenhaff P, Hamann J, Kelley K, Aschenbach W, et al. Oxygen uptake on-kinetics in dog gastrocnemius in situ following activation of pyruvate dehydrogenase by dichloroacetate. J Physiol. (2002) 538:195–207. doi: 10.1113/jphysiol.2001.012984
41. Grassi B, Poole DC, Richardson RS, Knight DR, Erickson BK, and Wagner PD. Muscle O2 uptake kinetics in humans: implications for metabolic control. J Appl Physiol. (1996) 80:988–98. doi: 10.1152/jappl.1996.80.3.988
42. Krustrup P, Jones AM, Wilkerson DP, Calbet JAL, and Bangsbo J. Muscular and pulmonary O2 uptake kinetics during moderate- and high-intensity sub-maximal knee-extensor exercise in humans. J Physiol. (2009) 587:1843–56. doi: 10.1113/jphysiol.2008.166397
43. Ferreira LF, Townsend DK, Lutjemeier BJ, and Barstow TJ. Muscle capillary blood flow kinetics estimated from pulmonary O2 uptake and near-infrared spectroscopy. J Appl Physiol. (2005) 98:1820–8. doi: 10.1152/japplphysiol.00907.2004
44. Harper AJ, Ferreira LF, Lutjemeier BJ, Townsend DK, and Barstow TJ. Human femoral artery and estimated muscle capillary blood flow kinetics following the onset of exercise. Exp Physiol. (2006) 91:661–71. doi: 10.1113/expphysiol.2005.032904
45. Manoir GR, DeLorey DS, Kowalchuk JM, and Paterson DH. Kinetics of Vo2 limb blood flow and regional muscle deoxygenation in young adults during moderate intensity, knee-extension exercise. Eur J Appl Physiol. (2010) 108:607–17. doi: 10.1007/s00421-009-1263-7
46. Koga S, Okushima D, Barstow TJ, Rossiter HB, Kondo N, and Poole DC. Near-infrared spectroscopy of superficial and deep rectus femoris reveals markedly different exercise response to superficial vastus lateralis. Physiol Rep. (2017) 5:13402. doi: 10.14814/phy2.13402
Keywords: near-infrared spectroscopy, pulmonary kinetics, youth cyclists, longitudinal, oxidative phosphorylation, microvascular blood flow, oxygen uptake, muscular oxygen utilization
Citation: Hovorka M, Prinz B, Simon D, Zöger M, Rumpl C and Nimmerichter A (2022) Longitudinal alterations of pulmonary O2 on-kinetics during moderate-intensity exercise in competitive youth cyclists are related to alterations in the balance between microvascular O2 distribution and muscular O2 utilization. Front. Sports Act. Living 4:982548. doi: 10.3389/fspor.2022.982548
Received: 30 June 2022; Accepted: 31 October 2022;
Published: 16 November 2022.
Edited by:
Everton Crivoi Carmo, Senac University Center, BrazilReviewed by:
Danilo Marcelo Leite Do Prado, University Hospital of São Paulo, BrazilCopyright © 2022 Hovorka, Prinz, Simon, Zöger, Rumpl and Nimmerichter. This is an open-access article distributed under the terms of the Creative Commons Attribution License (CC BY). The use, distribution or reproduction in other forums is permitted, provided the original author(s) and the copyright owner(s) are credited and that the original publication in this journal is cited, in accordance with accepted academic practice. No use, distribution or reproduction is permitted which does not comply with these terms.
*Correspondence: Alfred Nimmerichter, YWxmcmVkLm5pbW1lcmljaHRlckBmaHduLmFjLmF0
†ORCID: Alfred Nimmerichter orcid.org/0000-0001-8275-2166
Disclaimer: All claims expressed in this article are solely those of the authors and do not necessarily represent those of their affiliated organizations, or those of the publisher, the editors and the reviewers. Any product that may be evaluated in this article or claim that may be made by its manufacturer is not guaranteed or endorsed by the publisher.
Research integrity at Frontiers
Learn more about the work of our research integrity team to safeguard the quality of each article we publish.