- Département de kinésiologie, Université Laval, Quebec City, QC, Canada
This study investigated the impact of hyperoxic gas breathing (HYP) on repeated-sprint ability (RSA) and on the associated training load (TL). Thirteen team- and racquet-sport athletes performed 6-s all-out sprints with 24-s recovery until exhaustion (power decrement ≥ 15% for two consecutive sprints) under normoxic (NOR: FIO2 0.21) and hyperoxic (HYP: FIO2 0.40) conditions in a randomized, single-blind and crossover design. The following variables were recorded throughout the tests: mechanical indices, arterial O2 saturation (SpO2), oxygenation of the vastus lateralis muscle with near-infrared spectroscopy, and electromyographic activity of the vastus lateralis, rectus femoris, and gastrocnemius lateralis muscles. Session TL (work × rate of perceived exertion) and neuromuscular efficiency (work/EMG [Electromyography]) were calculated. Compared with NOR, HYP increased SpO2 (2.7 ± 0.8%, Cohen's effect size ES 0.55), the number of sprints (14.5 ± 8.6%, ES 0.28), the total mechanical work (13.6 ± 6.8%, ES 0.30), and the session TL (19.4 ± 7.0%, ES 0.33). Concomitantly, HYP increased the amplitude of muscle oxygenation changes during sprints (25.2 ± 11.7%, ES 0.36) and recovery periods (26.1 ± 11.4%, ES 0.37), as well as muscle recruitment (9.9 ± 12.1%, ES 0.74), and neuromuscular efficiency (6.9 ± 9.0%, ES 0.24). It was concluded that breathing a hyperoxic mixture enriched to 40% O2 improves the total work performed and the associated training load during an open-loop RSA session in trained athletes. This ergogenic impact may be mediated by metabolic and neuromuscular alterations.
Introduction
The nature and the magnitude of a training effect are dictated by the frequency, duration, and intensity of the exercise; the so-called training load (TL). Those parameters are modulated multiple times during a training cycle to manage fatigue accumulation and ensure progressive, specific physiological adaptations. Enhancement in athletic performance is well-known to be attributable to the controlled fluctuation of the TL throughout the year (Fitz-Clarke et al., 1991). Therefore, the understanding and quantification of the impact of varied stimuli on TL represent a key element to sport performance optimization (Foster et al., 2001).
Among the multiple stimuli used by athletes and coaches to increase TL and promote physiological adaptations, oxygen supplementation (i.e., hyperoxia) appears very attractive due to cardiopulmonary, neuromuscular, and metabolic functions relying on this gas (Sperlich et al., 2017). With growing popularity since its approbation by the World Anti-Doping Agency (WADA) in 2001, hyperoxia can be obtained by increasing the inspired oxygen fraction (FIO2, normobaric hyperoxia) and/or the barometric pressure (hyperbaric hyperoxia). Performance during aerobic exercise such as time trials, time to exhaustion, graded exercise tests, and dynamic muscle function tests is, not surprisingly, acutely improved under hyperoxic conditions (Peltonen et al., 2001; Tucker et al., 2007; Manselin et al., 2017). Those improvements are mainly derived from the increase in arterial hemoglobin saturation (SaO2), arterial content of oxygen (CaO2), and therefore, the systemic O2 delivery to organs and skeletal muscles (Powers et al., 1993; Peltonen et al., 1995). These improvements attenuate exercise-induced hypoxemia (Nummela et al., 2002) and alter energy metabolism (Linossier et al., 2000). Hyperoxia has also been used during repeated high-intensity exercise and results are promising. As such, higher peak and mean power output have been documented when breathing 100% O2, compared to room air, during two 30-s cycle sprints separated by 4 min (Kay et al., 2008), during five sets of 40 high-intensity breast strokes (~50 s) (Sperlich et al., 2011), and during ten 15-s cycling sprints interspersed with 45 s of recovery (Porter et al., 2020). Overall, these data indicate that hyperoxia may be beneficial to exercise performance in varied sport settings, although the activity patterns of intermittent sports such as rackets and team sports have received limited attention so far.
During most team and racket sports, athletes repeat brief maximal or near-maximal efforts (i.e., sprints, changes of direction, jumps) with short recoveries at low to moderate intensity throughout the duration of the competition. This physical quality, called repeated-sprint ability (RSA), is critical to success, and coaches and support staff use different training methods to enhance RSA during training (Billaut and Bishop, 2009; Bishop et al., 2011; Girard et al., 2011). Many metabolic (e.g., phosphocreatine resynthesis, aerobic and anaerobic glycolysis, metabolite accumulation) and neuromuscular factors (e.g., muscle activation strategies) determine RSA, which have been reviewed in details elsewhere (Billaut and Bishop, 2009; Bishop et al., 2011; Girard et al., 2011). Short maximal efforts rely heavily on anaerobic metabolic systems to produce ATP (Gaitanos et al., 1993). However, research using muscle biopsy has also well-demonstrated that the contribution of the anaerobic glycolysis to ATP resynthesis diminishes and the aerobic metabolism becomes more critical when sprints are repeated (Bogdanis et al., 1996). This is confirmed by studies using hypoxia to reduce tissue oxygen availability. It was demonstrated with near-infrared spectroscopy (NIRS) that reducing arterial O2 saturation led to a decline in muscle oxygenation which impaired RSA in trained athletes (Billaut and Buchheit, 2013; Willis et al., 2017). Furthermore, the recovery of performance between sprints is purely fueled via aerobic energy sources, and muscle tissue reoxygenation has been shown to be another determinant of RSA (Buchheit and Ufland, 2011; Billaut and Buchheit, 2013). With such metabolic changes occurring during a repeated-sprint training session, one may expect a hyperoxic mixture to enhance some the aerobic components of RSA and, thereby, better maintain performance. A higher exercise intensity or the possibility to perform more sprint repetitions during a given training session would lead to a higher TL, and theoretically, greater training adaptations over time. However, to our best knowledge, no study has assessed the influence of HYP on RSA and the associated TL.
Before promoting such practice in training, one may first assess the efficacy of adding hyperoxia to a repeated-sprint training session with athletes. Therefore, this investigation examined the effects of acute normobaric hyperoxia on RSA, key psycho-physiological parameters, and TL in trained athletes. It was hypothesized that hyperoxia would enhance muscle reoxygenation and better maintain muscle activation, which would lead to enhanced RSA and TL.
Materials and Methods
Ethics Approval
The study was approved by the Ethics Committee of University Laval, and adhered to the principles established in the Declaration of Helsinki. Participants provided written informed consent after being informed of experimental procedures, associated risks, and potential benefits.
Participants
Thirteen athletes (including 5 women) volunteered for this study (mean ± SE; age, 23.0 ± 2.7 year; body mass, 70.7 ± 14.9 kg; stature, 175.5 ± 7.3 cm; percent body fat, 14.00 ± 4.38%). All participants were non-smokers, free of health problems, and did not use any medication or any other tobacco/nicotine products. Participants were training 8 (range 6–12) hours per week (in a sport that included high-intensity short intervals (e.g., basketball, hockey, and badminton). Athletes avoided vigorous exercise, drug and nutritional supplements 48 h before test, and refrained from alcohol and caffeine for 12 h. Athletes were also asked to consume a regular meal at least 2 h before every session and to keep a constant training load during the course of the study.
Experimental Design
Participants visited the laboratory for one preliminary visit and two experimental trials during which all testing procedures were performed by the same investigator. Sessions were separated by a minimum of 2 days to avoid fatigue and a maximum of 7 days.
During the first visit, stature, body mass, and percentage body fat (Tanita TBF-310; Tanita Corp. of America Inc., Arlington Heights, IL) were recorded. Resting heart rate and blood pressure (inclusion criteria, <140/100 mm Hg) were taken before trial in a seated position. The handlebars and seat were adjusted vertically and horizontally to each participants preference, and feet were secured to the pedals using toe clips. These settings were also replicated for all subsequent trials. Participants completed a 10-min standardized, specific RSA warm-up on a computer-controlled electrically braked cycle ergometer (Velotron Elite; RacerMate, Seattle, WA). After 3 min of passive rest, participants performed a force-velocity (FV) test to determine the optimal cycling resistance (see Force-Velocity Test procedures below). Athletes had 5 min of passive recovery and were then familiarized with the RSA test procedures. Athletes executed a shortened version of the RSA test (see Repeated-Sprint Ability Test procedures below) which included six 6-s sprints while wearing the breathing mask only.
Following the preliminary visit, athletes were randomized in a single-blind, cross-over design and asked to perform a RSA test in normoxia (NOR, FIO2 0.21) and in normobaric hyperoxia (HYP, FIO2 0.40). Normoxic and hyperoxic gas mixtures were delivered by a continuous-flow O2 concentrator (New Life Intensity 10, AirSep Corporation, Buffalo, NY) and administered via a rig of 3 × 170-liter Douglas bags connected to a facemask (AltitudeTech Inc., Kingston, ON, Canada). After the standardized warm-up, participants were instrumented with the NIRS device, electromyography electrodes, and pulse oximeter. For both experimental trials, the breathing apparatus was installed 1 min before the beginning of the RSA test and was removed after the last sprint. A 5-minute cool-down was observed after every trial.
Testing Procedures
Standardized Warm-Up
A standardized warm-up was realized by the athletes before the force-velocity and the RSA tests. It included a total of 3 min of moderate intensity cycling, two 15 s accelerations with 15 s active recovery, 1 min 30 s of moderate intensity and then three 6 s sprints at 80, 90, and 100% of their maximal effort with 24 s of recovery between each of them. The last 3 min of the warm-up were passive rest where participants could stayed on the bike without pedaling or stand-up.
Force-Velocity Test
Athletes performed five maximal 6-s cycle sprints against increasing resistances and interspersed with 4 min of passive recovery. Sprints were initiated with 5-s of cycling at a standardized cadence (between 80 and 90 rpm) with the same resistance of the following sprint. Athletes were allowed to stand-up for the first two pedal strokes and then remained seated for the duration of the sprint and were strongly encouraged to reach maximal power output as quickly as possible (same instructions were given for the RSA test). The individual power–velocity relationships were obtained, and the optimal cycling resistance was defined as the gear for which maximal cycling power output was reached.
Repeated-Sprint Ability Test
After the standardized warm-up, participants performed consecutive maximal 6-s cycle sprints, separated by 24 s of rest (19 s of passive rest and 5 s of progressive re-acceleration of the flywheel) until exhaustion on an electronically braked cycle ergometer (Velotron Elite, RacerMate, Seattle, WA). An electronic tablet with a visual and audible signal was placed directly in front of the participants for precise count-down. Participants were asked to stop pedaling immediately at the end of the sprint, and the flywheel was stopped by the experimenter with the ergometer's brake. The resistance determined during the force-velocity test was set at the beginning of the RSA test and did not change afterwards. Following the test, all instrumentation was removed, and participants performed a self-paced cool down of at least 3 min.
To reduce the inter-subject variability in performance decrement and to enable a more standardized level of fatigue between the participants, both RSA tests were stopped when participants completed two consecutive sprints with a percent decrement score (SDEC) higher than 15% or if they reached 20 sprints (Morin et al., 2011). The SDEC across the sprints was determined as the percent difference between total and ideal performance (Glaister et al., 2008), as follows: SDEC = [100 × (total performance/ideal performance)]−100, where total performance is the sum of the peak power output (PPO) from all sprints performed, and ideal performance is the highest PPO recorded multiplied by the number of sprints performed.
The Velotron ergometer provides instantaneous, average and peak values for power output, pedal frequency, and speed at 23 Hz. The mechanical work (kJ) performed during every sprint was calculated by integrating the instantaneous power curve over the 6 s of the sprint. The number of sprints, the PPO, and the total mechanical work (Wtot, the sum of the work from all sprints) were measured and calculated. The sum of the mechanical work values for the common sprints (i.e., the sprints performed by every participant in the two conditions, Wcom) were also calculated. For example, if a participant performed 12 sprints in NOR and 14 sprints in HYP, only the first 12 sprints of each condition were taken for the Wcom calculation.
Exercise Physiological Responses
Rate of Perceived Exhaustion (RPE) and TL
Perceived exhaustion (RPEtot), perceived limb discomfort (RPElim), and perceived difficulty breathing (RPEbre) were recorded from a modified Borg CR10 scale after every sprint. Participants were asked to point the number associated with their perceived effort on a printed scale.
The TL was calculated as the product of the total mechanical work and the average of RPEtot scores during the RSA test. This calculation considers both the external load and the internal load, which is a valid and practical tool to monitor athletes training load (Gabbett et al., 2017). Moreover, it is used by many sport performance professionals in the fields of strength and conditioning and sport physiology. Calculation: TL = (Wtot × average rates of perceived effort).
Arterial O2 Saturation (SpO2) and Heart Rate (HR)
The SpO2 was estimated via pulse oximetry (Nellcor Bedside; Nellcor Inc., Hayward, CA) with adhesive optodes placed on the forehead. The reproducibility and validity (intraclass correlation coefficient = 0.99) for this method of measurement for saturations above 80% has been shown to be in good agreement with hemoglobin O2 when compared with arterial blood gas measurements (Romer et al., 2007). The SpO2 and the HR were recorded at rest, and immediately after every sprint, and every recovery period. The lowest value of SpO2 recorded during the RSA test was considered as SpO2min.
Near-Infrared Spectroscopy (NIRS)
A portable spatially-resolved, dual wavelength NIRS apparatus (PortaMon, Artinis Medical Systems BV, The Netherlands) was installed on the distal part of the dominant lower limb vastus lateralis muscle belly (~15 cm above the proximal border of the patella), parallel to muscle fibers, to quantify changes in the absorption of near-infrared light by oxyhemoglobin/myoglobin (O2Hb/Mb) and deoxyhemoglobin/myoglobin (HHb/Mb). Skinfold thickness was measured at the site of the application of the NIRS (9.0 ± 4.6 mm) using a Harpenden skinfold caliper (British Indicators Ltd, West Sussex, Great Britain) during the familiarization session and was less than half the distance between the emitter and the detector (i.e., 20 mm). This thickness is adequate to let near-infrared light through muscle tissue (McCully and Hamaoka, 2000). The device was packed in transparent plastic wrap to protect it from sweat and fixed with tape. Black bandages were used to cover the device from interfering background light. The position of the apparatus was marked with an indelible pen for repositioning in the subsequent visit.
A modified form of the Beer–Lambert law, using two continuous wavelengths (760 and 850 nm) and a differential optical path length factor of 4.95, was used to calculate micromolar concentrations in tissue O2Hb/Mb, HHb/Mb and total hemoglobin/myoglobin ([THb/Mb] = [O2Hb/Mb] + [HHb/Mb]). The tissue saturation index (TSI = [O2Hb/Mb]/[THb/Mb]) was also calculated. Exercise data was normalized to a 1-min baseline recorded while resting on the bike before the warm-up of visit 2 and 3 (defined as 0 μM). The NIRS data were acquired continuously at 10 Hz and then filtered using a tenth-order Butterworth low-pass filter with a 4 Hz cut-off frequency. Analysis of muscle oxygenation was limited to [HHb/Mb] because this variable is less sensitive than [O2Hb/Mb] to perfusion variations during contraction and recovery and is closely related to the venous O2 content (De Blasi et al., 1993; Ferrari et al., 2004), and to TSI because this parameter is independent of near-infrared photon path length in tissue and reflects the dynamic balance between O2 supply and O2 consumption in the microcirculation (Van Beekvelt et al., 2001). From the filtered signal, the maximal and minimal [HHb/Mb], [THb/Mb], and TSI were identified for every sprint/recovery cycle from a 5-s moving average to identify the maximal upper and lower limits of the signals (Rodriguez et al., 2018). In addition, the deoxygenation amplitude during sprints (Δ[HHb/Mb]exercise and ΔTSIexercise) was also calculated as the average difference between maximal and minimal [HHb/Mb] and TSI values for every sprint. Similarly, the reoxygenation amplitude during post-sprint recovery periods (Δ[HHb/Mb]recovery and ΔTSIrecovery) were calculated as the difference between maximal and minimal [HHb/Mb] and TSI values for every recovery period.
Normalized NIRS values were also represented as percentage of RSA test completion. As the fewer number of sprints realized by a participant was five, data was divided into five segments to obtain beginning (0%), 25, 50, 75, and 100% of test completion, and calculated the averages of all parameters for every segment for both sprint and recovery periods.
Electromyography (EMG)
The EMG signals of the vastus lateralis, rectus femoris, and gastrocnemius lateralis were recorded from the dominant lower limb via surface electrodes (Delsys, Trigno Wireless, Boston, MA) during every sprint. A careful preparation of the skin (shaving, light abrasion, and cleaning with an alcohol swab) on the electrodes sites was made before every test. Electrodes were fixed longitudinally (aligned parallel to the underlying muscle fiber direction) over the muscle belly. The EMG signal was pre-amplified and filtered (bandwidth 12–500 Hz, gain = 1,000, sampling frequency 2 kHz) and recorded with Delsys hardware (Bagnoli EMG System; Delsys, Inc., USA). During post-processing, the onset and offset of activation of all EMG bursts in all muscles and every sprint were detected manually. The activity of each muscle was determined by calculating the integral of the EMG (iEMG) and the median power frequency (MPF) between the onset and the offset of the last 6 subsequent bursts of the sprint. Values of iEMG of every muscle were totaled and a new sum-iEMG parameter was used to represent the global muscle activity during sprints (Billaut and Smith, 2009; Smith and Billaut, 2010; Billaut et al., 2013). The sum-iEMG for all the sprints (sum-iEMGtot) and for the common sprints (sum-iEMGcom) were also calculated.
Neuromuscular efficiency (NME) was calculated for every sprint as the ratio of work to sum-iEMG (NME = work/sum-iEMG) and expressed for all the sprints (NMEtot) and for the common sprints (NMEcom). A decrease in NME would indicate perturbations in muscle contractility (Bigland-Ritchie, 1981). Mechanical and EMG parameters are reported as raw data and as percent of the first sprint value. Normalization has been used widely when the effects of fatigue on force production and EMG activity are studied (Bigland-Ritchie, 1981; Hunter et al., 2004).
Statistical Analysis
All data are reported as means ± SD, percentage of normalized values or percentage of change from NOR. The NOR and HYP differences were analyzed using Cohen's effect size (ES) ± 90% confidence limits and compared to the smallest worthwhile change (0.2 multiplied by the between-participant SD), as well as the first sprint PPO and work values (Batterham and Hopkins, 2005; Hopkins et al., 2009). Effect sizes were classified as small (>0.2), moderate (>0.5) and large (>0.8), and only likely effects (>75%) are reported (Batterham and Hopkins, 2005; Hopkins et al., 2009).
Results
Mechanical and Perceptual Measurements
Performance variables are presented in Table 1 and Figure 1. The PPO reached was similar in both conditions, but HYP extended the number of sprints performed before exhaustion compared to NOR (difference between groups 14.5 ± 8.6%, ES 0.28). The total work performed over the entire series also increased in HYP (13.6 ± 6.8%, ES 0.30), but when the same number of sprints was considered in both NOR and HYP, the work in these common sprints did not change meaningfully (2.0 ± 1.7%, ES 0.05). Similar effects were observed when analyzing work to body mass ratio (J/kg) on Wtot (13.6 ± 6.8%, ES 0.27) and Wcom (2.0 ± 1.7%, ES 0.04).
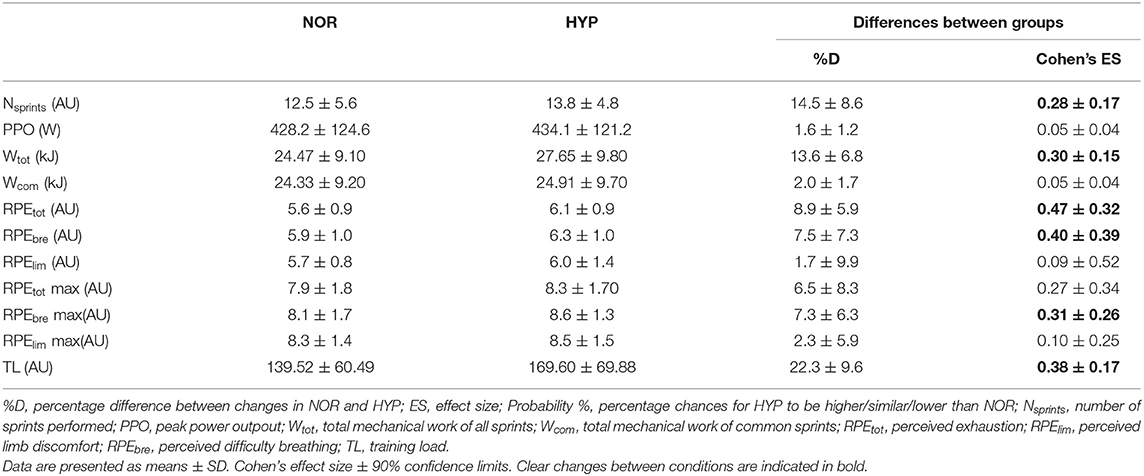
Table 1. Mean changes in performance and perceptual exercise responses in the repeated-sprint ability test in normoxia (NOR, FIO2: 0.21) or hyperoxia (HYP, FIO2: 0.40).
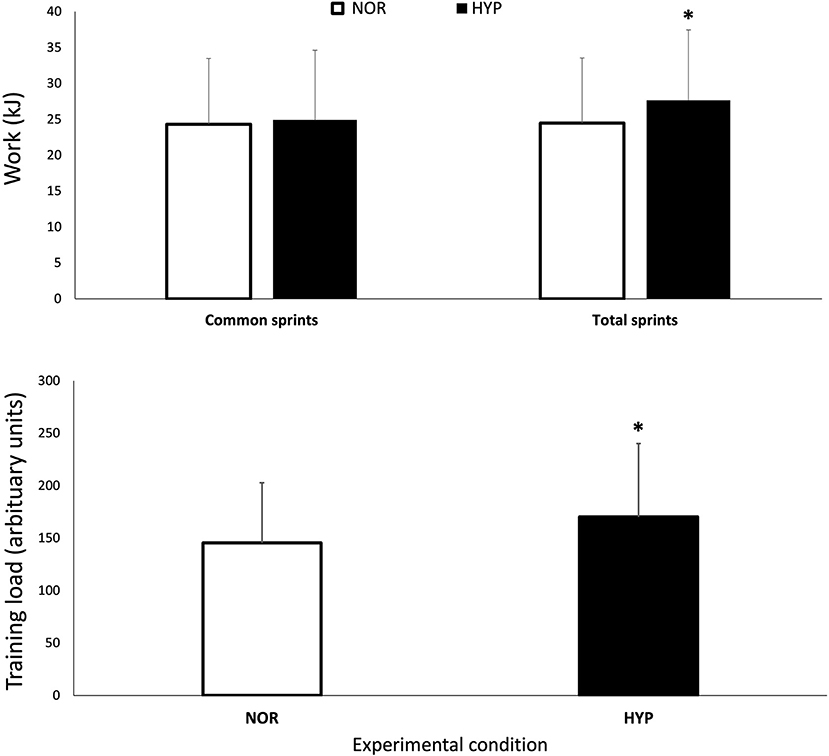
Figure 1. Mechanical work performed over the entire series of sprints (Wtot) and for the same number of sprints performed in both conditions (Wcom) session training load over the repeated-sprint ability test in normoxia and hyperoxia (FIO2 0.40). Data are presented as means ± SD. *Small effect between conditions. Wtot values were 24.47 ± 9.1 kJ in NOR and 27.65 ± 9.8 kJ in NYP. Wcom values were 24.33 ± 9.2 kJ in NOR and 24.91 ± 9.7 kJ in HYP. TL was increased from 139.52 ± 60.49 AU in NOR to 169.60 ± 69.88 in HYP.
Importantly, HYP also increased the session TL by 19.4 ± 7.0% (ES 0.33). HYP also increased RPEtot (8.9 ± 5.9%, ES 0.47) and RPEbre (7.5 ± 7.3%, ES 0.40). There was no clear difference between conditions for RPElim.
Arterial Oxygenation and Heart Rate
Breathing the hyperoxic mixture increased the averaged SpO2 recorded during the entire series of sprints (2.7 ± 0.8, ES 0.55) as well as the lowest SpO2 (HYP 99.2 ± 0.8 vs. NOR 95.1 ± 2.1, difference 4.4 ± 1.0, ES 1.85). Furthermore, HR was lower in HYP (HYP 163.1 ± 14.2 bpm vs. NOR 164.8 ± 12.6 bpm, difference −1.1 ± 1.9, ES −0.24).
Muscle Oxygenation
Figure 2 displays the changes in NIRS variables over the RSA test in both NOR and HYP. During the sprints, the maximal metabolic alterations ([HHb/Mb]max and TSImin) were not different between conditions, however it was observed that greater average muscle deoxygenation amplitude occurred when sprints were performed in HYP compared with NOR (Δ[HHb/Mb]exercise: 25.2 ± 11.7%, ES 0.36 and ΔTSIexercise: 14.0 ± 13.3%, ES 0.62).
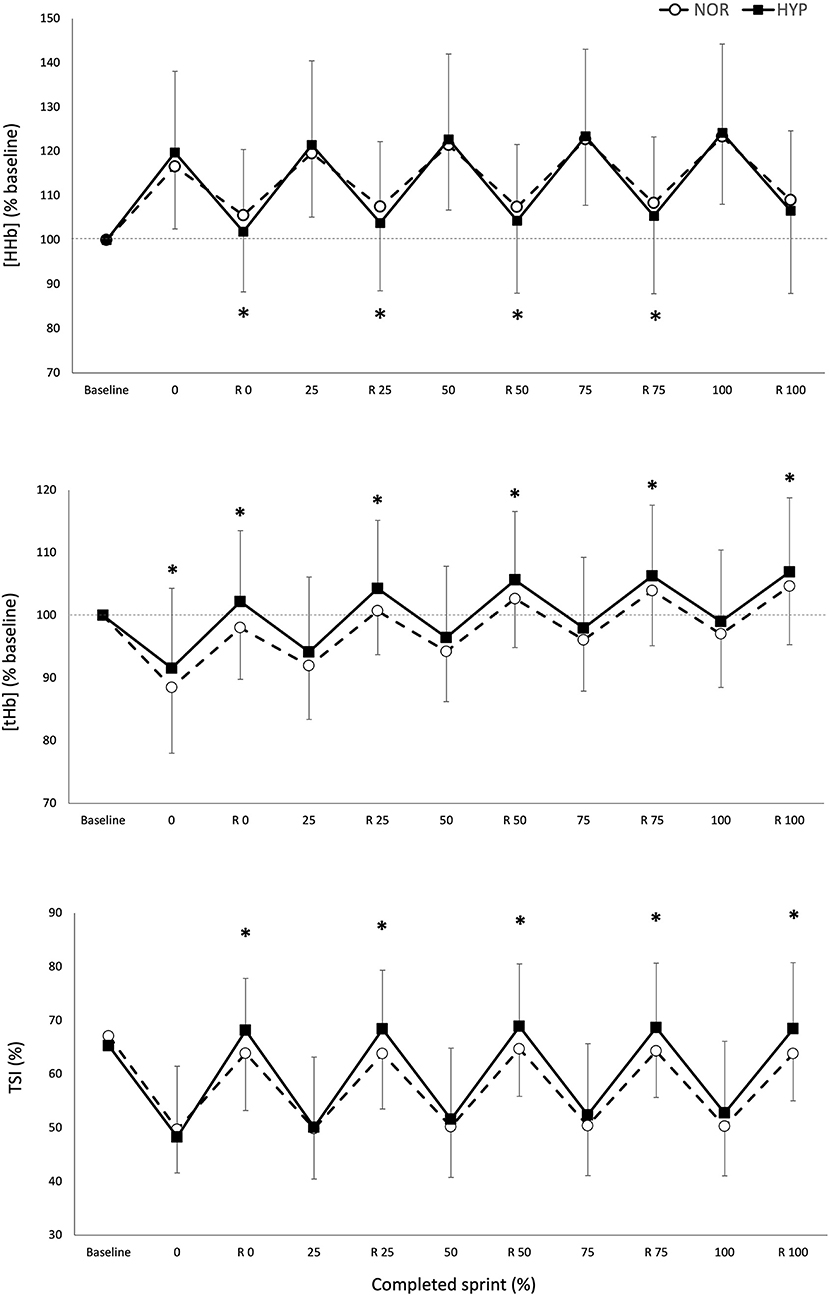
Figure 2. Maximal and minimal values of normalized deoxyhemoglobin/myoglobin concentration [HHb/Mb], total hemoglobin/myoglobin concentration [tHb/Mb], and tissue saturation index [TSI], over the number of sprints and recovery (R) periods for the five relative percentages of test completion in normoxia and hyperoxia (Fi IO2 0.40). Data are presented as means ± SD, expressed as a percent of the baseline. *Small effect between conditions.
The metabolic status of the recovery periods between sprints was also altered by the condition. Muscle reoxygenation was greater in HYP (Δ[HHb/Mb]recovery: 26.1 ± 11.4%, ES 0.37 and ΔTSIrecovery: 27.8 ± 15.7%, ES 0.53). This led to lower values of [HHb/Mb]min in HYP at the beginning (−3.5 ± 4.1%, ES −0.25), 25% (−3.5 ± 5.2%, ES −0.24), 50% (−3.2 ± 5.1%, ES −0.22) and 75% (−3.0 ± 5.5%, ES −0.21) of test completion. Furthermore, TSImax was higher in HYP at the beginning (7.0 ± 6.7%, ES 0.36), 25% (7.0 ± 6.9%, ES 0.36), 50% (5.8 ± 7.8%, ES 0.30), 75% (5.9 ± 7.8%, ES 0.31), and 100% (6.3 ± 7.9%, ES 0.33) of test completion. An increase in [THb/Mb]max was also observed at the beginning (4.3 ± 4.7%, ES 0.45), 25% (3.3 ± 3.9%, ES 0.35), 50% (2.7 ± 3.5%, ES 0.29), 75% (2.1 ± 3.4%, ES 0.22), and 100% (1.9 ± 3.5%, ES 0.21).
Electromyographic Activity
HYP increased sum-iEMGtot by 9.9 ± 12.1% (ES 0.74) and sum-iEMGcom by 10.2 ± 12.0% (ES 0.77). When looking at muscles individually, HYP increased the average value of the vastus lateralis sum-iEMGtot by 10.3 ± 11.8% (ES 0.71) and that of the rectus femoris sum-iEMGtot by 14.8 ± 16.7% (ES 0.93), while no change was observed in the gastrocnemius lateralis.
NME values are presented in Figure 3. Mean value of NMEtot increased in HYP (6.9 ± 9.0%, ES 0.24) as well as mean value of NMEcom (7.8 ± 8.7%, ES 0.27). Finally, MPF decreased from 82.3 ± 13.9 Hz to 77.85 ± 10.81 Hz in NOR and from 81.75 ± 13.22 to 78.14 ± 10.61 Hz in HYP, without showing any clear difference between conditions.
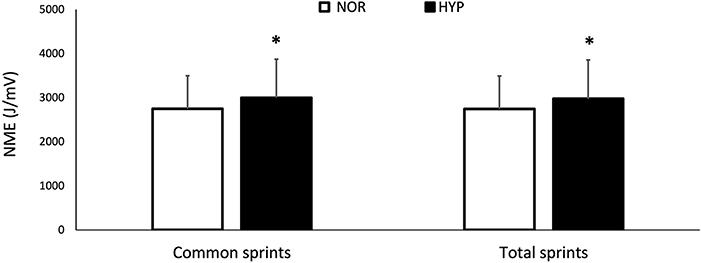
Figure 3. Changes for neuromuscular efficiency (NME) over the entire series of sprints (NMEtot) and for the same number of sprints performed in both conditions (NMEcom) over the repeated-sprint ability test in normoxia and hyperoxia (FIO2 0.40). Data are presented as means ± SD. *Small effect between conditions. NMEtot values were 2747.12 ± 746.54 AU in NOR and 2975.51 ± 879.80 AU in HYP. NMEcom values were 2749.86 ± 746.07 AU in NOR and 3000.14 ± 872.70 AU in HYP.
Discussion
The present study was performed to assess the acute effects of breathing a hyperoxic mixture on psycho-physiological responses and performance during a repeated-sprint ability test in athletes. The main results were that hyperoxia enhanced sprint endurance during short cycling sprints and the training load of the session. This improvement in mechanical performance may have been due to the concomitant increase in muscle oxygenation fluxes during sprints and recovery periods subsequent to the enhancement in arterial O2 saturation and in neuromuscular activity.
Hyperoxia and Repeated-Sprint Session Training Load
The present study demonstrated that the initial sprint mechanical indices (work and PPO) were not altered by HYP. This comes with no surprise since the ability to produce maximal power during short efforts is mainly derived from anaerobic energy sources (Calbet et al., 2003). This is in line with the robust finding that lowering FIO2 (i.e., hypoxia) also does not impair maximal power output (Balsom et al., 1994; Smith and Billaut, 2010; Billaut and Buchheit, 2013). Taken together, this data suggests that short-term maximal performance is well-preserved in face of a wide range of FIO2. The initial sprint performance is related to the mechanical decrement occurring in subsequent sprints (Bishop et al., 2003), thus the influence of this methodological factor on RSA can be excluded in the present study. When sprints are repeated, the accumulation of metabolic end-products linked to high rates of anaerobic glycolysis induces muscle fatigue. In this regard, breathing more O2 may attenuate the loss of power and prolong exercise. Indeed, participants produced ~14% more work over the entire series in HYP compared to room air, and this enhanced performance likely came from a greater aerobic ATP production (Calbet et al., 2020). Such ergogenic effect has also been reported during sprints of 15–30-s duration with 100% O2 (Kay et al., 2008; Porter et al., 2020). The present data therefore adds to the literature that mild hyperoxia (40% O2) can also enhance RSA during very short efforts.
In addition to the greater performance, HYP exacerbated the breathing and total effort perception. This may be surprising since the physical difficulty an athlete experiences has been shown to stay the same or be slightly lowered by hyperoxic breathing during maximal exercise, especially with FIO2 lower than 1.00 (Sperlich et al., 2017). Importantly however, such finding comes mostly from exercise of given duration in which the higher O2 saturation improves cardiopulmonary and metabolic functions and the sense of effort. In the current study, participants exercised longer, which could explain why they perceived the RSA test more exhausting. More research will need to disentangle what mechanisms (e.g., ease of breathing, neurotransmitter release) play a pivotal role in modifying the sense of effort.
Overall, HYP increased the session TL by ~19%, and this occurred in 12 participants out of 13. A further interesting result, with practical applications for training, was that HYP enhanced performance by increasing the number of repetitions without altering the work performed during the sprints that were common to both conditions. This represents a differing ergogenic impact of hyperoxia during short vs. long sprints, in which power output appears to increase during the sprint (Kay et al., 2008; Porter et al., 2020). This is likely caused by the different muscle energetics during efforts of varying duration (Calbet et al., 2020). Since aerobic processes become more predominant as 6-s sprints are repeated (Gaitanos et al., 1993), it is not surprising to observe the ergogenic impact of hyperoxia in the latter stages of a RSA protocol. This difference may also be caused by the mild hyperoxia used in the present study compared with a 1.0 FIO2 in former studies. Nonetheless, for the coach or sport scientist aiming to increase TL during RSA sessions with team- and racquet-sport athletes, it may be more relevant to use open-loop exercises in which athletes can perform more repetitions before ending a session. According to the overload principle, performing more repetitions of an exercise will trigger mechanical and/or physiological changes that, over time, should enhance the neuromuscular function and exercise capacity. One may thus argue that performing a few more efforts during every sprint session during a mesocycle could lead to superior adaptations. Such training approach will have to be tested in long-term, periodized, longitudinal studies.
Due to the cumbersomeness of generators, the easiest setting to use HYP during RSA sessions would be indoors, when athletes train on ergometers with a facemask attached to a generator. In fact, in many sports athletes do train in gymnasiums, strength and conditioning spaces or laboratories to complement the specific outdoor activities and increase their TL above that achievable in room air. In such environments, another opportunity would be to use HYP generators to fill a space with hyperoxic mixtures and have athletes exercise more freely within. To date, the main challenge to using HYP in the field is really the lack of light and practical portable generators for use during intense bouts of exercise. While some athletes may try and breathe HYP through a facemask during recovery between sets of high-intensity exercise, this is not practical, and the development of technological advances will certainly improve this limitation to use environmental exposure for specific physiological adaptations.
Hyperoxia and Arterial and Muscle Oxygenation
Althoug hypoxemia is rarely seen during RSA protocols at sea level, it may still develop during longer exercise protocols and hinder performance (Billaut and Smith, 2010). Considering the criteria defined by Dempsey and Wagner (Dempsey and Wagner, 1999), the present results show that seven participants experienced mild hypoxemia (>3% SpO2 fall from resting levels) and one participant experienced moderate hypoxemia (SpO2 <93%) during the test. Oxygen availability plays an important role during RSA tests. In fact, arterial hypoxemia imposed by hypoxia induces greater metabolic perturbations and reduces performance during repeated sprints with brief recovery (Billaut and Smith, 2010; Smith and Billaut, 2012; Willis et al., 2017). Here, HYP had a clear positive impact to prevent the fall in SpO2 and maintained it at 99–100% throughout the test. Interestingly, the five athletes displaying no significant fall in SpO2 in NOR were those exhibiting the smallest difference in mechanical work between NOR and HYP.
To our best knowledge, the present study is the first to report changes in muscle oxygenation during repeated sprints under hyperoxia. The performance gains in HYP were concomitant with greater muscle oxygenation fluxes. Typically, muscle deoxygenation increases rapidly in the first sprint and then plateaus in the subsequent sprints, which indicates maximal skeletal muscle O2 extraction early in the exercise (Buchheit et al., 2009; Smith and Billaut, 2010; Buchheit and Ufland, 2011; Billaut and Buchheit, 2013). HYP could not enhance this mechanism further since both [HHb/Mb]max and TSImin remained similar to those observed in NOR. However, Δ[HHb/Mb]exercise and ΔTSIexercise increased in HYP, indicative of greater peripheral muscle O2 extraction when more O2 is available before reaching maximal limit. Thus, one may argue that the relative contribution of the oxidative metabolism vs. anaerobic glycolysis was greater during the sprints in HYP, thereby contributing to prolong exercise.
This greater aerobic contribution during exercise was probably possible through changes during the recovery. The lower [HHb/Mb]min, and greater TSImax, Δ[HHb/Mb]recovery, and ΔTSIrecovery suggest that HYP also enhanced oxygenation status of the muscle during recovery periods. Muscular activity is dependent on the balance between O2 delivery and O2 consumption by the skeletal muscle fibers, and convective delivery of O2 is regulated by blood flow supplying the working muscles and the O2 content of the blood. HYP enhanced SpO2 and also [THb/Mb]max suggesting a greater regional volume of O2-rich blood. Although this study did not measure blood flow per se, it is still likely that O2 delivery and utilization were enhanced during the recovery between sprints. Muscle reoxygenation plays a critical role on RSA (Buchheit et al., 2009; Billaut and Buchheit, 2013). The present results confirm these findings and together highlight muscle reoxygenation capacity as an important factor of the ability to reproduce mechanical performance in subsequent sprint repetitions. The recovery of phosphocreatine and muscle reoxygenation after exercise presents similar kinetics (Chance et al., 1992; McCully et al., 1994; Kime et al., 2003), and it has been reported that hyperoxia accelerates phosphocreatine resynthesis (Haseler et al., 1998; Hogan et al., 1999). It is therefore probable that a better muscle reoxygenation in HYP would have translated into a better PCr resynthesis during the recovery periods and a higher PCr availability for subsequent sprints. Other factors altered by systemic O2 availability and muscle blood flow such as accumulation of glucose-6-phosphate, hydrogen ions (and subsequent drop in pH) and inorganic phosphates, and reliance on fat metabolism for energy not measured in this study but robustly detailed elsewhere (Sperlich et al., 2017) may have also contributed to extend RSA.
Although the maximal peripheral O2 uptake during the sprints did not appear to differ between the environments, probably due to the very high intensity of the efforts and stress put on the oxidative pathway, HYP still generated marked amplitudes of tissue oxygenation during both sprint and recovery. Repeated fluctuations of tissue partial pressure of O2 are necessary for muscular oxidative capacity adaptations (Daussin et al., 2008; Casey and Joyner, 2012). Thus, in addition to increasing a session TL per se, hyperoxia may also be an effective environmental stress for inducing peripheral adaptations by imposing repeated muscle oxygenation fluxes. For instance, Kon et al. (2019) reported an improvement in lactate curve at submaximal exercise after 3 weeks of sprint-interval (30-s sprint) training with 60% O2 in college athletes, which could be related to an improvement in mitochondrial oxidative capacity. On the other hand, 6-week interval training between 80 and 100% of maximal aerobic power with 30% O2 in trained cyclists did not result in any improvement in mitochondrial phosphorylation capacity nor markers of mitochondrial content (Cardinale et al., 2019). The current and previous findings are difficult to reconcile owing the disparity in inspired O2 fractions used, type of training, and probably also athletes' fitness and histochemical and metabolic profile. Repeated-sprint training in hyperoxia should be investigated as RSA training provides a very different stimulus than high-intensity interval training (Billaut and Bishop, 2009; Bishop et al., 2011; Girard et al., 2011).
Hyperoxia and Muscle Activation
Under substantial fatigue (evaluated at >10% fatigue index or sprint decrement), a concomitant attenuation of EMG activity and mechanical performance is usually observed across sprint repetitions (Racinais et al., 2007; Mendez-Villanueva et al., 2008; Billaut and Buchheit, 2013). This suggests that the ability to fully activate the working muscles may be incriminated in the loss of mechanical output. Motor neuron activity is greatly influenced by O2 availability (Bigland-Ritchie et al., 1986; Szubski et al., 2006; Amann et al., 2007). In the RSA literature, the reduction in SpO2 has been correlated with the attenuation in surface EMG activity (Billaut and Smith, 2010), and has also been shown to accentuate the decline in central motor command assessed from peripheral muscle stimulation (Billaut et al., 2013). The present results therefore suggest that O2 supplementation during sprints helps maintain a higher muscle activation (as evidenced by a ~10% higher sum-iEMG indices in HYP). The most likely explanation would be that the greater tissue perfusion and oxygenation improved the metabolic milieu of contracting muscles and attenuated the reflex inhibition originating from group III and IV afferents, thereby maintaining neural drive to skeletal muscles (Amann and Dempsey, 2008).
There is also the possibility that changes in EMG indices related at least in part to sarcolemma excitability and changes in conduction velocity of action potentials. Membrane excitability is impaired during intense fatiguing exercise as a result of a lower activity of the sodium(Na+)/potassium(K+)-adenosine triphosphatase activity to maintain transmembrane ionic gradient caused by the decline in pH and accumulation of inorganic phosphates. Greater muscle perfusion and oxygenation during sprints could have altered energy metabolism and the subsequent accumulation of by-products and acidosis that resulted in lesser sarcolemma dysfunction (Linossier et al., 2000). Future studies should more robustly investigate the potential attenuation of centrally mediated decline in neural drive and alterations in M-wave amplitude.
Conclusion
This study demonstrated that breathing a hyperoxic mixture with 40% O2 improved the total work performed and the associated training load during an open-loop test of RSA in trained team- and racquet-sport athletes. This ergogenic effect occurred concurrent with an increase in muscle oxygenation fluxes during sprints and recovery periods and in neuromuscular activity. Although this study showed promising results about the effectiveness of mild hyperoxia to enhance TL during RSA sessions, important factors such as manipulation of FIO2 and optimum exercise-to-rest ratios remain to be explored for efficient applications.
Data Availability Statement
The raw data supporting the conclusions of this article will be made available by the authors, without undue reservation.
Ethics Statement
The studies involving human participants were reviewed and approved by Ethics Committee of University Laval. The patients/participants provided their written informed consent to participate in this study.
Author Contributions
SC-K and FB conceptualized and designed the research project. SC-K acquired the data and conducted the statistical analysis. SC-K interpreted results with assistance from FB. SC-K wrote the manuscript with revisions from FB. Both authors reviewed and agreed upon the final manuscript.
Conflict of Interest
The authors declare that the research was conducted in the absence of any commercial or financial relationships that could be construed as a potential conflict of interest.
Publisher's Note
All claims expressed in this article are solely those of the authors and do not necessarily represent those of their affiliated organizations, or those of the publisher, the editors and the reviewers. Any product that may be evaluated in this article, or claim that may be made by its manufacturer, is not guaranteed or endorsed by the publisher.
Acknowledgments
The authors thank the athletes for their participation in this study. We also sincerely thank all graduate and undergraduate students from our research group for their valuable help, as well as TMC Distribution for the generous loan of the oxygen concentrator.
References
Amann, M., and Dempsey, J. A. (2008). Locomotor muscle fatigue modifies central motor drive in healthy humans and imposes a limitation to exercise performance. J. Physiol. 586, 161–173. doi: 10.1113/jphysiol.2007.141838
Amann, M., Romer, L. M., Subudhi, A. W., Pegelow, D. F., and Dempsey, J. A. (2007). Severity of arterial hypoxaemia affects the relative contributions of peripheral muscle fatigue to exercise performance in healthy humans. J. Physiol. 581, 389–403. doi: 10.1113/jphysiol.2007.129700
Balsom, P. D., Ekblom, B., and Sjödin, B. (1994). Enhanced oxygen availability during high intensity intermittent exercise decreases anaerobic metabolite concentrations in blood. Acta Physiol. Scand. 150, 455–456. doi: 10.1111/j.1748-1716.1994.tb09711.x
Batterham, A. M., and Hopkins, W. G. (2005). Making meaningful inferences about magnitudes. Sportscience 9, 6–14. doi: 10.1123/ijspp.1.1.50
Bigland-Ritchie, B (1981). Emg/Force relations and fatigue of human voluntary contractions. Exerc. Sport Sci. Rev. 9, 75–117. doi: 10.1249/00003677-198101000-00002
Bigland-Ritchie, B. R., Dawson, N. J., Johansson, R. S., and Lippold, O. C. (1986). Reflex origin for the slowing of motoneurone firing rates in fatigue of human voluntary contractions. J. Physiol. 379, 451–459. doi: 10.1113/jphysiol.1986.sp016263
Billaut, F., and Bishop, D. (2009). Muscle fatigue in males and females during multiple-sprint exercise. Sports Med. 39, 257–278. doi: 10.2165/00007256-200939040-00001
Billaut, F., and Buchheit, M. (2013). Repeated-sprint performance and vastus lateralis oxygenation: effect of limited O2 availability. Scand. J. Med. Sci. Sports 23:e185–e193. doi: 10.1111/sms.12052
Billaut, F., Kerris, J. P., Rodriguez, R. F., Martin, D. T., Gore, C. J., and Bishop, D. J. (2013). Interaction of central and peripheral factors during repeated sprints at different levels of arterial O2 saturation. PLoS ONE 8:e77297. doi: 10.1371/journal.pone.0077297
Billaut, F., and Smith, K. (2009). Sex alters impact of repeated bouts of sprint exercise on neuromuscular activity in trained athletes. Appl. Physiol. Nutr. Metab. 34:689–99. doi: 10.1139/H09-058
Billaut, F., and Smith, K. (2010). Prolonged repeated-sprint ability is related to arterial O2 desaturation in men. Int. J. Sports Physiol. Perform. 5, 197–209. doi: 10.1123/ijspp.5.2.197
Bishop, D., Girard, O., and Mendez-Villanueva, A. (2011). Repeated-sprint ability — Part II. Sports Med. 41, 741–756. doi: 10.2165/11590560-000000000-00000
Bishop, D., Lawrence, S., and Spencer, M. (2003). Predictors of repeated-sprint ability in elite female hockey players. J. Sci. Med. Sport 6, 199–209. doi: 10.1016/S1440-2440(03)80255-4
Bogdanis, G. C., Nevill, M. E., Boobis, L. H., and Lakomy, H. K. (1996). Contribution of phosphocreatine and aerobic metabolism to energy supply during repeated sprint exercise. J. Appl. Physiol. 80, 876–884. doi: 10.1152/jappl.1996.80.3.876
Buchheit, M., Cormie, P., Abbiss, C. R., Ahmaidi, S., Nosaka, K. K., and Laursen, P. B. (2009). Muscle deoxygenation during repeated sprint running: effect of active vs. passive recovery. Int. J. Sports Med. 30, 418–425. doi: 10.1055/s-0028-1105933
Buchheit, M., and Ufland, P. (2011). Effect of endurance training on performance and muscle reoxygenation rate during repeated-sprint running. Eur. J. Appl. Physiol. 111, 293–301. doi: 10.1007/s00421-010-1654-9
Calbet, J. A. L., De Paz, J. A., Garatachea, N., de Vaca, S. C., and Chavarren, J. (2003). Anaerobic energy provision does not limit Wingate exercise performance in endurance-trained cyclists. J. Appl. Physiol. 94:668–76. doi: 10.1152/japplphysiol.00128.2002
Calbet, J. A. L., Martín-Rodríguez, S., Martin-Rincon, M., and Morales-Alamo, D. (2020). An integrative approach to the regulation of mitochondrial respiration during exercise: focus on high-intensity exercise. Redox Biol. 35:101478. doi: 10.1016/j.redox.2020.101478
Cardinale, D. A., Larsen, F. J., Lännerström, J., Manselin, T., Södergård, O., Mijwel, S., et al. (2019). Influence of hyperoxic-supplemented high-intensity interval training on hemotological and muscle mitochondrial adaptations in trained cyclists. Front. Physiol. 10:730. doi: 10.3389/fphys.2019.00730
Casey, D. P., and Joyner, M. J. (2012). Compensatory vasodilatation during hypoxic exercise: mechanisms responsible for matching oxygen supply to demand. J. Physiol. 590, 6321–6326. doi: 10.1113/jphysiol.2012.242396
Chance, B., Dait, M. T., Zhang, C., Hamaoka, T., and Hagerman, F. (1992). Recovery from exercise-induced desaturation in the quadriceps muscles of elite competitive rowers. Am. J. Physiol. 262, C766–C775. doi: 10.1152/ajpcell.1992.262.3.C766
Daussin, F. N., Zoll, J., Ponsot, E., Dufour, S. P., Doutreleau, S., Lonsdorfer, E., et al. (2008). Training at high exercise intensity promotes qualitative adaptations of mitochondrial function in human skeletal muscle. J. Appl. Physiol. 104, 1436–1441. doi: 10.1152/japplphysiol.01135.2007
De Blasi, R. A., Cope, M., Elwell, C., Safoue, F., and Ferrari, M. (1993). Noninvasive measurement of human forearm oxygen consumption by near infrared spectroscopy. Eur. J. Appl. Physiol. 67, 20–25. doi: 10.1007/BF00377698
Dempsey, J. A., and Wagner, P. D. (1999). Exercise-induced arterial hypoxemia. J. Appl. Physiol. 87, 1997–2006. doi: 10.1152/jappl.1999.87.6.1997
Ferrari, M., Mottola, L., and Quaresima, V. (2004). Principles, techniques, and limitations of near infrared spectroscopy. Can. J. Appl. Physiol. 29, 463–487. doi: 10.1139/h04-031
Fitz-Clarke, J. R., Morton, R. H., and Banister, E. W. (1991). Optimizing athletic performance by influence curves. J. Appl. Physiol. 71, 1151–1158. doi: 10.1152/jappl.1991.71.3.1151
Foster, C. G., Florhaug, J. A., Franklin, J. E., Gottschall, L., Hrovatin, L. A., Parker, S., et al. (2001). A new approach to monitoring exercise training. J. Strength Cond. Res. 15, 109–15. doi: 10.1519/00124278-200102000-00019
Gabbett, T. J., Nassis, G. P., Oetter, E., Pretorius, J., Johnston, N., Medina, D., et al. (2017). The athlete monitoring cycle: a practical guide to interpreting and applying training monitoring data. Br. J. Sports Med. 51, 1451–1452. doi: 10.1136/bjsports-2016-097298
Gaitanos, G. C., Williams, C., Boobis, L. H., and Brooks, S. (1993). Human muscle metabolism during intermittent maximal exercise. J. Appl. Physiol. 75, 712–719. doi: 10.1152/jappl.1993.75.2.712
Girard, O., Mendez-Villanueva, A., and Bishop, D. (2011). Repeated-Sprint ability — Part I. Sports Med. 41, 673–694. doi: 10.2165/11590550-000000000-00000
Glaister, M., Howatson, G., Pattison, J. R., and McInnes, G. (2008). The reliability and validity of fatigue measures during multiple-sprint work: an issue revisited. J. Strength Cond. Res. 22, 1597–1601. doi: 10.1519/JSC.0b013e318181ab80
Haseler, L. J., Richardson, R. S., Videen, J. S., and Hogan, M. C. (1998). Phosphocreatine hydrolysis during submaximal exercise: the effect of F I O 2. J. Appl. Physiol. 85:1457–63. doi: 10.1152/jappl.1998.85.4.1457
Hogan, M. C., Richardson, R. S., and Haseler, L. J. (1999). Human muscle performance and PCr hydrolysis with varied inspired oxygen fractions: a 31P-MRS study. J. Appl. Physiol. 86, 1367–1373. doi: 10.1152/jappl.1999.86.4.1367
Hopkins, W. G., Marshall, S. W., Batterham, A. M., and Hanin, J. (2009). Progressive statistics for studies in sports medicine and exercise science. Med. Sci. Sports Exerc. 41, 3–13. doi: 10.1249/MSS.0b013e31818cb278
Hunter, S. K., Critchlow, A., Shin, I.-S., and Enoka, R. M. (2004). Men are more fatigable than strength-matched women when performing intermittent submaximal contractions. J. Appl. Physiol. 96, 2125–2132. doi: 10.1152/japplphysiol.01342.2003
Kay, B., Stannard, S. R., and Morton, R. H. (2008). Hyperoxia during recovery improves peak power during repeated wingate cycle performance. Braz. J. Biomotrocity. 2, 92–100.
Kime, R., Karlsen, T., Nioka, S., Lech, G., Madsen, Ø., Sæterdal, R., et al. (2003). Discrepancy between cardiorespiratory system and skeletal muscle in elite cyclists after hypoxic training. Dyn. Med. 2:4. doi: 10.1186/1476-5918-2-4
Kon, M., Nakagaki, K., and Ebi, Y. (2019). Effects of all-out sprint interval training under hyperoxia on exercise performance. Physiol. Rep. 7:14194. doi: 10.14814/phy2.14194
Linossier, M. T., Dormois, D., Arsac, L., Denis, C., and Lacour, J. R. (2000). Effect of hyperoxia on aerobic and anaerobic performances and muscle metabolism during maximal cycling exercise. Acta Physiol. Scand. 168, 403–411. doi: 10.1046/j.1365-201x.2000.00648.x
Manselin, T. A., Södergård, O., Larsen, F. J., and Lindholm, P. (2017). Aerobic efficiency is associated with the improvement in maximal power output during acute hyperoxia. Physiol. Rep. 5:e13119. doi: 10.14814/phy2.13119
McCully, K. K., and Hamaoka, T. (2000). Near-infrared spectroscopy: what can it tell us about oxygen saturation in skeletal muscle? Exerc. Sport Sci. Rev. 28, 123–127.
McCully, K. K., Iotti, S., Kendrick, K., Wang, Z., Posner, J. D., Leigh, J., et al. (1994). Simultaneous in vivo measurements of HbO2 saturation and PCr kinetics after exercise in normal humans. J. Appl. Physiol. 77, 5–10. doi: 10.1152/jappl.1994.77.1.5
Mendez-Villanueva, A., Hamer, P., and Bishop, D. (2008). Fatigue in repeated-sprint exercise is related to muscle power factors and reduced neuromuscular activity. Eur. J. Appl. Physiol. 103, 411–419. doi: 10.1007/s00421-008-0723-9
Morin, J.-B., Dupuy, J., and Samozino, P. (2011). Performance and fatigue during repeated sprints: what is the appropriate sprint dose? J. Strength Cond. Res. 25, 1918–1924. doi: 10.1519/JSC.0b013e3181e075a3
Nummela, A., Hämäläinen, I., and Rusko, H. (2002). Effect of hyperoxia on metabolic responses and recovery in intermittent exercise. Scand. J. Med. Sci. Sports 12, 309–315. doi: 10.1034/j.1600-0838.2002.10157.x
Peltonen, J. E., Rantamäki, J., Niittymäki, S. P., Sweins, K., Viitasalo, J. T., and Rusko, H. K. (1995). Effects of oxygen fraction in inspired air on rowing performance. Med. Sci. Sports Exerc. 27, 573–579. doi: 10.1249/00005768-199504000-00016
Peltonen, J. E., Tikkanen, H. O., and Rusko, H. K. (2001). Cardiorespiratory responses to exercise in acute hypoxia, hyperoxia and normoxia. Eur. J. Appl. Physiol. 85, 82–88. doi: 10.1007/s004210100411
Porter, M. S., Reed, K., and Jones, B. (2020). The use of acute oxygen supplementation upon muscle tissue saturation during repeat sprint cycling. J. Human Sport Exerc. 17. doi: 10.14198/jhse.2022.171.10
Powers, S. K., Martin, D., and Dodd, S. (1993). Exercise-induced hypoxaemia in elite endurance athletes. Incidence, causes and impact on VO2max. Sports Med. 16, 14–22. doi: 10.2165/00007256-199316010-00003
Racinais, S., Bishop, D., Denis, R., Lattier, G., Mendez-Villaneuva, A., and Perrey, S. (2007). Muscle deoxygenation and neural drive to the muscle during repeated sprint cycling. Med. Sci. Sports Exerc. 39, 268–274. doi: 10.1249/01.mss.0000251775.46460.cb
Rodriguez, R. F., Townsend, N. E., Aughey, R. J., and Billaut, F. (2018). Influence of averaging method on muscle deoxygenation interpretation during repeated-sprint exercise. Scand. J. Med. Sci. Sports 28, 2263–2271. doi: 10.1111/sms.13238
Romer, L. M., Haverkamp, H. C., Amann, M., Lovering, A. T., Pegelow, D. F., and Dempsey, J. A. (2007). Effect of acute severe hypoxia on peripheral fatigue and endurance capacity in healthy humans. Am. J. Physiol. Regul. Integr. Comp. Physiol. 292, R598–R606. doi: 10.1152/ajpregu.00269.2006
Smith, K. J., and Billaut, F. (2010). Influence of cerebral and muscle oxygenation on repeated-sprint ability. Eur. J. Appl. Physiol. 109, 989–999. doi: 10.1007/s00421-010-1444-4
Smith, K. J., and Billaut, F. (2012). Tissue oxygenation in men and women during repeated-sprint exercise. Int. J. Sports Physiol. Perform. 7, 59–67. doi: 10.1123/ijspp.7.1.59
Sperlich, B., Zinner, C., Hauser, A., Holmberg, H.-C., and Wegrzyk, J. (2017). The impact of hyperoxia on human performance and recovery. Sports Med. 47, 429–438. doi: 10.1007/s40279-016-0590-1
Sperlich, B., Zinner, C., Krueger, M., Wegrzyk, J., Mester, J., and Holmberg, H. C. (2011). Ergogenic effect of hyperoxic recovery in elite swimmers performing high-intensity intervals. Scand. J. Med. Sci. Sports 21, e421–e429. doi: 10.1111/j.1600-0838.2011.01349.x
Szubski, C., Burtscher, M., and Löscher, W. N. (2006). The effects of short-term hypoxia on motor cortex excitability and neuromuscular activation. J. Appl. Physiol. 101, 1673–1677. doi: 10.1152/japplphysiol.00617.2006
Tucker, R., Kayser, B., Rae, E., Rauch, L., Bosch, A., and Noakes, T. (2007). Hyperoxia improves 20 km cycling time trial performance by increasing muscle activation levels while perceived exertion stays the same. Eur. J. Appl. Physiol. 101, 771–781. doi: 10.1007/s00421-007-0458-z
Van Beekvelt, M. C. P., Colier, W. N. J. M., Wevers, R. A., and Van Engelen, B. G. M. (2001). Performance of near-infrared spectroscopy in measuring local O2 consumption and blood flow in skeletal muscle. J. Appl. Physiol. 90, 511–519. doi: 10.1152/jappl.2001.90.2.511
Keywords: oxygen supplementation, training load, multiple sprints, team sports, muscle oxygenation, hyperoxic training, neuromuscular activation
Citation: Cyr-Kirk S and Billaut F (2022) Hyperoxia Improves Repeated-Sprint Ability and the Associated Training Load in Athletes. Front. Sports Act. Living 4:817280. doi: 10.3389/fspor.2022.817280
Received: 17 November 2021; Accepted: 09 February 2022;
Published: 11 March 2022.
Edited by:
Michael John Hamlin, Lincoln University, New ZealandReviewed by:
Gregory C. Bogdanis, National and Kapodistrian University of Athens, GreeceSarah J. Willis, University of Lausanne, Switzerland
Copyright © 2022 Cyr-Kirk and Billaut. This is an open-access article distributed under the terms of the Creative Commons Attribution License (CC BY). The use, distribution or reproduction in other forums is permitted, provided the original author(s) and the copyright owner(s) are credited and that the original publication in this journal is cited, in accordance with accepted academic practice. No use, distribution or reproduction is permitted which does not comply with these terms.
*Correspondence: François Billaut, ZnJhbmNvaXMuYmlsbGF1dCYjeDAwMDQwO2tpbi51bGF2YWwuY2E=