- 1Human Potential Translational Research Programme, Yong Loo Lin School of Medicine, National University of Singapore, Singapore, Singapore
- 2Research and Scientific Support, Aspetar Orthopaedic and Sports Medicine Hospital, Doha, Qatar
- 3Centre for Exercise and Sports Science Research, School of Medical and Health Sciences, Edith Cowan University, Perth, WA, Australia
- 4School of Sport and Health Sciences, University of Central Lancashire, Preston, United Kingdom
In the last decade, cold water immersion (CWI) has emerged as one of the most popular post-exercise recovery strategies utilized amongst athletes during training and competition. Following earlier research on the effects of CWI on the recovery of exercise performance and associated mechanisms, the recent focus has been on how CWI might influence adaptations to exercise. This line of enquiry stems from classical work demonstrating improved endurance and mitochondrial development in rodents exposed to repeated cold exposures. Moreover, there was strong rationale that CWI might enhance adaptations to exercise, given the discovery, and central role of peroxisome proliferator-activated receptor gamma coactivator-1α (PGC-1α) in both cold- and exercise-induced oxidative adaptations. Research on adaptations to post-exercise CWI have generally indicated a mode-dependant effect, where resistance training adaptations were diminished, whilst aerobic exercise performance seems unaffected but demonstrates premise for enhancement. However, the general suitability of CWI as a recovery modality has been the focus of considerable debate, primarily given the dampening effect on hypertrophy gains. In this mini-review, we highlight the key mechanisms surrounding CWI and endurance exercise adaptations, reiterating the potential for CWI to enhance endurance performance, with support from classical and contemporary works. This review also discusses the implications and insights (with regards to endurance and strength adaptations) gathered from recent studies examining the longer-term effects of CWI on training performance and recovery. Lastly, a periodized approach to recovery is proposed, where the use of CWI may be incorporated during competition or intensified training, whilst strategically avoiding periods following training focused on improving muscle strength or hypertrophy.
Introduction
Cold water immersion (CWI) is a strategy aimed at enhancing recovery from strenuous exercise, typically involving the submersion up to the waist or mid-torso for ~5–20 min in temperatures between ~8 and 15°C (Versey et al., 2013; Ihsan et al., 2016; Machado et al., 2016). Following contemporary work by Vaile et al. (2008a,b,c); Vaile et al. (2011) and Peiffer et al. (2010a,b) in late-2000s investigating the effect of CWI on the recovery of physical performance, research in this area has extended to investigate a plethora of recovery outcomes including thermoregulatory response (Stephens et al., 2018), hemodynamics (Mawhinney et al., 2013; Choo et al., 2016), hormonal balance (Halson et al., 2008), skeletal muscle damage (Goodall and Howatson, 2008), and autonomic nervous function (Bastos et al., 2012; Choo et al., 2018). Complementing this growth in research, CWI has become one of the most popular post-exercise recovery strategies utilized amongst athletes during training and competition (Périard et al., 2016; Crowther et al., 2017; Murray et al., 2018; Cross et al., 2019).
Meta-analyses and experimental research in general show that CWI can beneficially influence the recovery of physical performance (Montgomery et al., 2008; Bleakley and Davison, 2010; Rowsell et al., 2011; Poppendieck et al., 2013; Tabben et al., 2018). Nevertheless, many consider the efficacy of CWI to be equivocal. The inconsistent findings of CWI hence must be acknowledged, and this is likely driven by factors such as the nature of exercise modality preceding CWI, nature of recovery variables assessed, timing between recovery assessment and completion of CWI, and the CWI protocol itself. While such complexities need to be resolved to appropriately compare study findings and interpret with context, recent discussions have revolved extensively around how the regular use of CWI for recovery might in parallel influence adaptations to exercise (Broatch et al., 2018; Malta et al., 2021). Yet, the first study to address this extends considerably back to 2006 (Yamane et al., 2006). Following a hiatus, a series of studies examining the influence of CWI on adaptation to endurance exercise emerged from independent laboratories (Ihsan et al., 2014b, 2015, 2020b; Aguiar et al., 2016; Joo et al., 2016; Allan et al., 2017, 2019, 2020; Broatch et al., 2017), which was followed up by others examining the influence of CWI on resistance training adaptations (Frohlich et al., 2014; Roberts et al., 2015; Figueiredo et al., 2016; D'Souza et al., 2018; Fyfe et al., 2019; Fuchs et al., 2020; Peake et al., 2020; Poppendieck et al., 2020). A recent meta-analytical review showed that CWI effects on exercise adaptations are mode-dependant, where resistance training adaptations were diminished, whilst aerobic exercise performance seemed unaffected (Malta et al., 2021). Alongside these publications, detailed narrative reviews on the adaptative response following regular CWI have been published within this research topic series (Petersen and Fyfe, 2021), and elsewhere (Broatch et al., 2018). Additionally, editorials, point-counterpoints, and opinion pieces (Allan and Mawhinney, 2017; McPhee and Lightfoot, 2017; Méline et al., 2017; Peake, 2017, 2020; White and Caterini, 2017; Cheng, 2018; Ihsan et al., 2020a) have been published discussing the suitability of CWI as a post-exercise recovery tool given its dampening effect on hypertrophy gains. However, potential adaptive benefits that can be harnessed from CWI following endurance exercise, or from a recovery objective are often overlooked.
In this mini-review, we have adopted an introspective approach in discussing the molecular mechanisms and rationale surrounding CWI and endurance exercise adaptations. Moreover, we review and discuss key studies which provide information on the applied scenarios where CWI can be utilized to promote physical recovery and adaptation whilst avoiding potential negative effects on hypertrophy and strength gains.
Cold Exposure and Endurance Exercise Adaptation
Cold stimulus is a physiological stressor capable of triggering primary signals and downstream cascades implicated in exercise-induced improvements in muscle oxidative function (Ihsan et al., 2014a). Evidence for cold-induced changes in a/the mammalian oxidative profile can be derived from as early as 1960, where Hannon (1960) demonstrated robust increases in the enzyme activities of several electron transport chain components of rat gastrocnemius muscle following 3–4 weeks of cold exposure. Subsequent studies extended these initial findings by demonstrating comparable increases in muscle oxidative enzymes between cold acclimation and exercise (Hamilton and Ferguson, 1972; Harri and Valtola, 1975). More recent studies have since shown improved fatigue resistance and exercise capacity following cold adaptation, in line with molecular signatures implicating increased mitochondrial content (Schaeffer et al., 2003; Bruton et al., 2010). In summary, research within rodent models highlight cold exposure as a viable modality to enhance muscle oxidative adaptations and endurance.
Seminal work by Spielgeman's group in the late 90's generated major breakthroughs in the mechanisms underpinning mitochondrial biogenesis (Puigserver et al., 1998; Wu et al., 1999), which incidentally further supported the use of cold therapeutic strategies such as CWI. Their work investigating the mechanisms of adaptive thermogenesis led to the discovery of the transcriptional coactivator peroxisome proliferator-activated receptor gamma coactivator-1α (PGC-1α), which was found to robustly increase in response to cold exposure (3 and 12 h exposure @ 4°C) in mice brown fat and skeletal muscle, concomitant with an increase in numerous mitochondrial markers (Puigserver et al., 1998; Wu et al., 1999). Subsequent work highlighted a regulatory role for PGC-1α in mitochondrial biogenesis (Lira et al., 2010), and other oxidative adaptations such as angiogenesis through the vascular endothelial growth factor (VEGF) (Chinsomboon et al., 2009), muscle fiber type transformation (Lin et al., 2002), glucose (Wende et al., 2005), and fat metabolism (Vega et al., 2000) in cell culture and murine models. Following evidence within human exercise models demonstrating a regulatory role for PGC-1α in skeletal muscle aerobic adaptations (Pilegaard et al., 2003; Russell et al., 2003; Perry et al., 2010), there was understandably speculation that CWI might additively enhance adaptations to exercise through common mechanisms involving PGC-1α (Ihsan et al., 2014a).
Endurance Exercise Adaptation to Post-Exercise CWI: Discrepancy Between Molecular Signaling and Exercise Performance
Whilst CWI was not conceived as a strategy specifically meant to supplement exercise adaptations, there was substantial interest in examining how recovery-based CWI might influence skeletal muscle adaptations to endurance exercise (Ihsan et al., 2014b, 2015, 2020b; Aguiar et al., 2016; Joo et al., 2016; Allan et al., 2017, 2019, 2020; Broatch et al., 2017). This line of enquiry is likely motivated by work in cell cultures and rodents demonstrating robust increases in mitochondrial markers following exercise and cold exposure with common mechanisms involving PGC-1α. Moreover, recent work in humans extends further support, where markers of mitochondrial development have been shown to be enhanced in the skeletal muscle after acute aerobic exercise in the cold compared with room temperature (Shute et al., 2018), or when post-exercise recovery was undertaken in a cold environment (Slivka et al., 2013). In agreement, CWI (10–15 min @ 8–10°C) administered independently, or following an acute bout of endurance exercise was shown to increase the mRNA of PGC-1α and VEGF (Ihsan et al., 2014b; Joo et al., 2016). Additionally, regular CWI (15 min @ 10°C) application during 4 weeks of endurance training (30 s to 8 min interval bouts @ 80–110% of peak power/velocity) has been shown to increase a variety of mitochondrial markers (mRNA and protein abundance) and upstream regulatory kinases (Ihsan et al., 2015; Aguiar et al., 2016). However, an increase in PGC-1α protein content was not consistently observed in these studies (Ihsan et al., 2015; Aguiar et al., 2016) with only Ihsan et al. (2015) reporting an CWI-mediated increase following training. Regardless, complimenting the aforementioned studies showing an increase in VEGF mRNA (Ihsan et al., 2014b; Joo et al., 2016), regular CWI (10–15 min @ 10°C) incorporated during exercise training lasting 4–12 weeks has been shown to enhance skeletal muscle microvascular function (Ihsan et al., 2020b) and increase skeletal muscle capillarity (D'Souza et al., 2018). Conversely, Broatch et al. (2017) found no effect of CWI (15 min @ 10°C) on molecular markers indicative of mitochondrial development (i.e., mRNA responses, phosphorylation status, and protein abundance) following a single sprint interval training session or following 6 weeks of sprint interval training. Factors such as subcutaneous fat, muscle mass, body surface area, and acclimation status may influence the adaptations that are driven by the magnitude of tissue temperature change, and hence partly account for such disparity in findings. Alternatively, high-intensity exercise/training as undertaken by Broatch et al. (2017) can robustly increase mitochondrial markers (Granata et al., 2016) creating a ceiling effect, resulting in diminished potential for CWI augment further adaptations. Collectively, these findings indicate that the effects of post-exercise CWI may be less pronounced following high-intensity exercises, but is able to influence molecular and structural adaptations befitting muscle oxidative function following lower intensity endurance exercise.
Although such molecular responses would expectedly improve endurance performance in the longer term, Yamane et al. (2006) reported attenuated improvements in maximal oxygen uptake and cycling time to exhaustion following regular CWI (2 × 20 min at 5°C) during 4 weeks of endurance training. The authors suggested that the decrease in muscle temperature and metabolism following cooling might have suppressed regenerative mechanisms mediated through inflammatory and heat shock proteins (HSP). This mechanism is unlikely prevalent during recovery-based CWI protocols resulting in mild to moderate decreases in tissue temperature. Indeed, typical post-exercise CWI involves 10–15°C immersion for 10–15 min, and such protocols have been shown to not influence skeletal muscle inflammatory response, HSP expression, or trafficking (Aguiar et al., 2016; Peake et al., 2017). Interestingly, HSF-1, a transcription factor for multiple HSPs, has been shown to be upregulated following regular CWI administered throughout 4 weeks of endurance training (Aguiar et al., 2016). As such, the findings demonstrated by Yamane et al. (2006) likely involve other mechanisms such as increased muscle proteolysis, increased oxidative stress or lowered tissue metabolism and consequently remodeling (Fu et al., 1997; Manfredi et al., 2013; Broatch et al., 2018) that are associated with aggressive cooling and extreme decreases in tissue temperature.
In contrast to Yamane et al. (2006) initial report, studies investigating the longer-term effects of recovery-based CWI do not support concerns of this modality being detrimental to endurance training adaptations. For instance, regular CWI administered during 4–6 weeks of sprint- or aerobic-interval training similarly improved maximal oxygen uptake, peak aerobic power, and time-trial performance compared to control conditions (Aguiar et al., 2016; Broatch et al., 2017). Likewise, CWI administered to competitive cyclists undergoing 3-4 weeks of intensified training reported similar improvements in most cycling performance parameters, although some parameters were reported to improve to a greater extent following CWI (Halson et al., 2014).
While these findings refute suggestions that CWI might counteract endurance adaptations, it nevertheless questions whether post-exercise CWI is an effective strategy to promote muscle adaptations resulting in improved exercise performance. Indeed, changes in acute signaling response (Ihsan et al., 2014b; Joo et al., 2016; Broatch et al., 2017), training-induced protein accretion (Ihsan et al., 2015; Aguiar et al., 2016), and vascular adaptations (D'Souza et al., 2018; Ihsan et al., 2020b) do not seem to translate into improved exercise performance following regular CWI. Some have reasoned that endurance performances are largely governed by central factors (e.g., cardiovascular, hematological adaptations), and changes in muscle aerobic function following CWI may only marginally contribute (Malta et al., 2021). Alternatively, frequent CWI might have de-sensitized transcriptional responses. For instance, the magnitude of PGC-1α mRNA increases have been shown to progressively diminish in response to repeated exercise stimulus (Perry et al., 2010). Similarly, PGC-1α mRNA has been shown to robustly increase following exercise in a cold environment, but demonstrated a blunted PGC-1α mRNA response to an identical stimulus following 3 weeks of endurance training in the cold (Shute et al., 2020). However, it remains to be ascertained if this attenuated response is due to habituation to cold, exercise or a combination of both stimuli. Regardless, it must be re-iterated that CWI does not appear to impair aerobic training adaptations, and can be confidently incorporated as a recovery modality following endurance training sessions.
Effect of CWI On Endurance and Resistance Exercise Adaptations: Divergent Effects or Coordinated Regulation?
While some studies have shown that CWI can enhance physical recovery following resistance exercise (Vaile et al., 2008c; Roberts et al., 2014), practitioners should avoid scheduling this modality at least during the immediate recovery period. Indeed, regular CWI has been shown to attenuate the magnitude of anabolic signaling (Roberts et al., 2015) and protein synthesis (Fuchs et al., 2020), leading to reduced magnitude of strength and muscle mass gain following resistance training (Frohlich et al., 2014; Roberts et al., 2015; Fyfe et al., 2019; Poppendieck et al., 2020). Readers are directed to excellent reviews elsewhere (Broatch et al., 2018; Malta et al., 2021) and within this research topic (Petersen and Fyfe, 2021) elaborating on the mechanisms surrounding CWI and resistance training.
Complimenting these mechanisms, we suggest that the attenuated increase in muscle mass observed following CWI and resistance training may be part of a macro-level mechanism protecting the oxidative profile of the muscle. This is supported by D'Souza et al. (2018) demonstrating increased muscle capillarity following 12 weeks of resistance training with regular CWI application with concomitant decreases in muscle mass reported in other companion papers (Roberts et al., 2015; Peake et al., 2017). Reductions in muscle blood flow and metabolism during CWI may reduce O2 supply and utilization (Ihsan et al., 2013; Mawhinney et al., 2013, 2020; Choo et al., 2016), triggering compensatory adaptation involving decreased muscle mass and microvascular expansion to maintain perfusion capacity. Further support for such a phenotypic response can be derived from rodent and human models of cold-acclimation. Cross-sectional areas of gastrocnemius and soleus muscle fibers were found to be 15–21% smaller in cold-acclimated rats, with concomitant increases in capillarity (Suzuki et al., 1997). Similarly, Bae et al. (2003) showed that cold-acclimated breath-hold divers possessed higher skeletal muscle capillary density, a lower oxygen diffusion distance and a smaller muscle fiber CSA, whist no such adaptations were evident in breath-hold divers who dived at moderate water temperatures (29–30°C) (Park et al., 2005).
While we rationalize that the dampened increase in muscle mass observed following CWI is a compensatory mechanism improving oxidative function, further research is needed to understand how this might influence athletic function and performance. For instance, it is currently unknown if CWI influences the regulation of muscle mass following aerobic exercise, and whether this hypothetical trade-off involving the attenuated increase in muscle mass and strength might be beneficial to endurance performance. On the other hand, co-assessment of muscle aerobic function within these resistance training studies (Frohlich et al., 2014; Roberts et al., 2015; Fyfe et al., 2019; Poppendieck et al., 2020) would have furthered our understanding of the functional consequence of the dampened increase in mass coupled with increased capillarity.
CWI and Resistance Training: Insights From Applied Research
Athletes embark on a variety of training sessions such as cardiovascular conditioning, strength/resistance training, technical, and tactical work. In sports science practice, CWI is likely to be incorporated at various instances to promote recovery, particularly when recovery time between sessions is limited. Caution should be warranted against the regular use of CWI particularly following resistance exercise sessions.
Recent work (Table 1) examining the longer-term effects of CWI on training performance and recovery amongst professional and semi-professional athletes provides invaluable insights regarding CWI programming and recovery-adaptation interaction throughout training/competition phases (Lindsay et al., 2016; Tavares et al., 2019, 2020; Seco-Calvo et al., 2020). These studies collectively demonstrate no impairments in strength gains despite administering frequent post-exercise CWI over 2.5 weeks to 8 months. In contrast to the current literature (Frohlich et al., 2014; Roberts et al., 2015; Fyfe et al., 2019; Poppendieck et al., 2020), most of these studies (Table 1) report a trend for improved strength gains over the training/study period. Such divergent findings are hard to reconcile. One possibility, as Broatch et al. (2018) highlighted is that laboratory-based experimental studies are designed with 2–3 sessions per week permitting adequate recovery between sessions, and by extension not capitalizing on the recovery effects of CWI.
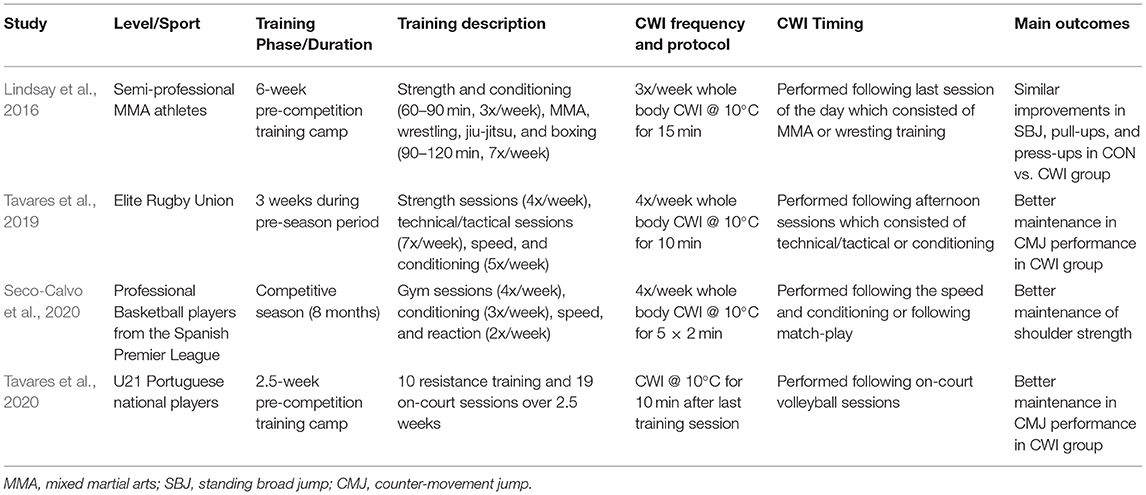
Table 1. Summary of studies examining the longer-term effect of CWI on the recovery of exercise performance.
Training frequency reported within these applied studies surmounts to at least 10 sessions per week (Table 1). Perhaps, in scenarios where recovery between training sessions may be limited, CWI can improve training performances and consequently the stimulus for adaptation. In support, post-exercise CWI has been shown to enhance the ability to perform more volitional work during subsequent squat exercise (Roberts et al., 2014), or better maintain day-to-day exercise performance during intensified periods of endurance training (Vaile et al., 2008b; Stanley et al., 2013). Given that adaptations to exercise stimulus are volume and intensity dependant, it seems reasonable to consider that the recovery benefits of CWI (and resultant increase in training quality) might outweigh its dampening effects on hypertrophy response. Conversely, it can be argued that anabolic adaptations are better enhanced if CWI is avoided, albeit this might deter the quality of subsequent training sessions. It is currently unknown which approach would better influence athletes' recovery-adaptation interaction. Longer term applied studies similar to those highlighted in this review (Table 1) will significantly contribute to our understanding in this area.
Another key feature of these studies (Table 1), and perhaps within sport science practice is that CWI is often not administered immediately following a resistance training session, but instead following technical/tactical or conditioning sessions. These findings show promise that beneficial recovery outcomes can be harnessed whilst avoiding negative effects of CWI on strength gains by simply avoiding the use of this recovery modality in the proximity of resistance training sessions. Regardless, we acknowledge that these studies were not specifically designed to address this notion. Moreover, the majority of these studies were relatively short-term (2.5–6 weeks). Specific, longer-term studies are therefore required to address the effect of CWI timing on strength adaptation.
Summary and Perspectives
Cold water immersion is widely utilized by athletes during training and competition. Given that both a cold stimulus and exercise are independent stressors capable of enhancing muscle oxidative function, there remains substantial interest in examining how this modality might influence adaptations to exercise. Although post-exercise CWI up-regulates mitochondrial-related signaling, longer-term changes in protein content and result in vascular adaptations, these changes do not seem to translate to improved endurance performance. As such, further research is required to elucidate how endurance performance can be improved through its positive molecular signaling outcomes for CWI to be incorporated to enhance exercise-induced oxidative adaptations. It must be re-iterated that CWI does not impair aerobic training adaptations, and can be incorporated as a recovery modality following endurance training if needed. In contrast, regular CWI recovery incorporated into a resistance training program will dampen strength adaptations, and therefore the use of this modality following resistance exercise sessions should be discouraged. However, there is emerging data showing no impairments in strength gains in athletes incorporating regular use of CWI during intensified training periods; this either indicates that the recovery benefits conferred by CWI may outweigh its dampening effects on hypertrophy response, or the negative effects of CWI on strength may be circumvented by programing CWI following technical or aerobic conditioning sessions. In this regard, “recovery periodization” may be an important approach, where the use of CWI may be incorporated during competition or intensified training, whilst strategically avoided following training focused on improving muscle strength or hypertrophy.
Author Contributions
All authors listed have made a substantial, direct and intellectual contribution to the work, and approved it for publication.
Conflict of Interest
The authors declare that the research was conducted in the absence of any commercial or financial relationships that could be construed as a potential conflict of interest.
References
Aguiar, P. F., Magalhães, S. M., Fonseca, I. A. T., da Costa Santos, V. B., de Matos, M. A., Peixoto, M. F. D., et al. (2016). Post-exercise cold water immersion does not alter high intensity interval training-induced exercise performance and Hsp72 responses, but enhances mitochondrial markers. Cell Stress Chaperones 21, 793–804. doi: 10.1007/s12192-016-0704-6
Allan, R., and Mawhinney, C. (2017). Is the ice bath finally melting? Cold water immersion is no greater than active recovery upon local and systemic inflammatory cellular stress in humans. J. Physiol. 595, 1857–1858. doi: 10.1113/JP273796
Allan, R., Morton, J. P., Close, G. L., Drust, B., Gregson, W., and Sharples, A. P. (2020). PGC-1α alternative promoter (Exon 1b) controls augmentation of total PGC-1α gene expression in response to cold water immersion and low glycogen availability. Eur. J. Appl. Physiol. 120, 2487–2493. doi: 10.1007/s00421-020-04467-6
Allan, R., Sharples, A. P., Close, G. L., Drust, B., Shepherd, S. O., Dutton, J., et al. (2017). Postexercise cold water immersion modulates skeletal muscle PGC-1alpha mRNA expression in immersed and nonimmersed limbs: evidence of systemic regulation. J. Appl. Physiol. 123, 451–459. doi: 10.1152/japplphysiol.00096.2017
Allan, R., Sharples, A. P., Cocks, M., Drust, B., Dutton, J., Dugdale, H. F., et al. (2019). Low pre-exercise muscle glycogen availability offsets the effect of post-exercise cold water immersion in augmenting PGC-1α gene expression. Physiol. Rep. 7:e14082. doi: 10.14814/phy2.14082
Bae, K., An, N., Kwon, Y., Kim, C., Yoon, C., Park, S., et al. (2003). Muscle fibre size and capillarity in Korean diving women. Acta Physiol. Scand. 179, 167–172. doi: 10.1046/j.1365-201X.2003.01185.x
Bastos, F. N., Vanderlei, L. C., Nakamura, F. Y., Bertollo, M., Godoy, M. F., Hoshi, R. A., et al. (2012). Effects of cold water immersion and active recovery on post-exercise heart rate variability. Int. J. Sports Med. 33, 873–879. doi: 10.1055/s-0032-1301905
Bleakley, C. M., and Davison, G. W. (2010). What is the biochemical and physiological rationale for using cold-water immersion in sports recovery? A systematic review. Br. J. Sports Med. 44, 179–187. doi: 10.1136/bjsm.2009.065565
Broatch, J. R., Petersen, A., and Bishop, D. J. (2017). Cold-water immersion following sprint interval training does not alter endurance signaling pathways or training adaptations in human skeletal muscle. Am. J. Physiol. Regul. Integr. Comp. Physiol. 313, R372–R384. doi: 10.1152/ajpregu.00434.2016
Broatch, J. R., Petersen, A., and Bishop, D. J. (2018). The influence of post-exercise cold-water immersion on adaptive responses to exercise: a review of the literature. Sports Med. 48, 1369–1387. doi: 10.1007/s40279-018-0910-8
Bruton, J. D., Aydin, J., Yamada, T., Shabalina, I. G., Ivarsson, N., Zhang, S. J., et al. (2010). Increased fatigue resistance linked to Ca2+-stimulated mitochondrial biogenesis in muscle fibres of cold-acclimated mice. J. Physiol. 588(Pt 21), 4275–4288. doi: 10.1113/jphysiol.2010.198598
Cheng, A. J. (2018). Cooling down the use of cryotherapy for post-exercise skeletal muscle recovery. Temperature 5, 103–105. doi: 10.1080/23328940.2017.1413284
Chinsomboon, J., Ruas, J., Gupta, R. K., Thom, R., Shoag, J., Rowe, G. C., et al. (2009). The transcriptional coactivator PGC-1alpha mediates exercise-induced angiogenesis in skeletal muscle. Proc. Natl. Acad. Sci. U.S.A. 106, 21401–21406. doi: 10.1073/pnas.0909131106
Choo, H. C., Nosaka, K., Peiffer, J. J., Ihsan, M., Yeo, C. C., and Abbiss, C. R. (2016). Peripheral blood flow changes in response to postexercise cold water immersion. Clin. Physiol. Funct. Imaging 38, 46–55. doi: 10.1111/cpf.12380
Choo, H. C., Nosaka, K., Peiffer, J. J., Ihsan, M., Yeo, C. C., and Abbiss, C. R. (2018). Effect of water immersion temperature on heart rate variability following exercise in the heat. Kinesiology 50, 67–74.
Cross, R., Siegler, J., Marshall, P., and Lovell, R. (2019). Scheduling of training and recovery during the in-season weekly micro-cycle: Insights from team sport practitioners. Eur. J. Sport Sci. 19, 1287–1296. doi: 10.1080/17461391.2019.1595740
Crowther, F., Sealey, R., Crowe, M., Edwards, A., and Halson, S. (2017). Team sport athletes' perceptions and use of recovery strategies: a mixed-methods survey study. BMC Sports Sci. Med. Rehabil. 24:6. doi: 10.1186/s13102-017-0071-3
D'Souza, R. F., Zeng, N., Markworth, J. F., Figueiredo, V. C., Roberts, L. A., Raastad, T., et al. (2018). Divergent effects of cold water immersion versus active recovery on skeletal muscle fiber type and angiogenesis in young men. Am. J. Physiol. Regul. Integr. Comp. Physiol. 314, R824–R833. doi: 10.1152/ajpregu.00421.2017
Figueiredo, V. C., Roberts, L. A., Markworth, J. F., Barnett, M. P., Coombes, J. S., Raastad, T., et al. (2016). Impact of resistance exercise on ribosome biogenesis is acutely regulated by post-exercise recovery strategies. Physiol. Rep. 4:e12670. doi: 10.14814/phy2.12670
Frohlich, M., Faude, O., Klein, M., Pieter, A., Emrich, E., and Meyer, T. (2014). Strength training adaptations after cold water immersion. J. Strength Cond. Res. 28, 2628–2633. doi: 10.1519/JSC.0000000000000434
Fu, F. H., Cen, H.-W., and Eston, R. G. (1997). The effects of cryotherapy on muscle damage in rats subjected to endurance training. Scand. J. Med. Sci. Sports 7, 358–362. doi: 10.1111/j.1600-0838.1997.tb00167.x
Fuchs, C. J., Kouw, I. W. K., Churchward-Venne, T. A., Smeets, J. S. J., Senden, J. M., Lichtenbelt, W., et al. (2020). Postexercise cooling impairs muscle protein synthesis rates in recreational athletes. J. Physiol. 598, 755–772. doi: 10.1113/JP278996
Fyfe, J. J., Broatch, J. R., Trewin, A. J., Hanson, E. D., Argus, C. K., Garnham, A. P., et al. (2019). Cold water immersion attenuates anabolic signaling and skeletal muscle fiber hypertrophy, but not strength gain, following whole-body resistance training. J. Appl. Physiol. 127, 1403–1418. doi: 10.1152/japplphysiol.00127.2019
Goodall, S., and Howatson, G. (2008). The effects of multiple cold water immersions on indices of muscle damage. J. Sports Sci. Med. 7, 235–241.
Granata, C., Oliveira, R. S., Little, J. P., Renner, K., and Bishop, D. J. (2016). Training intensity modulates changes in PGC-1α and p53 protein content and mitochondrial respiration, but not markers of mitochondrial content in human skeletal muscle. FASEB J. 30, 959–970. doi: 10.1096/fj.15-276907
Halson, S. L., Bartram, J., West, N., Stephens, J., Argus, C. K., Driller, M. W., et al. (2014). Does hydrotherapy help or hinder adaptation to training in competitive cyclists? Med. Sci. Sports Exerc. 46, 1631–1639. doi: 10.1249/MSS.0000000000000268
Halson, S. L., Quod, M. J., Martin, D. T., Gardner, A. S., Ebert, T. R., and Laursen, P. B. (2008). Physiological responses to cold water immersion following cycling in the heat. Int. J. Sports Physiol. Perform. 3, 331–346. doi: 10.1123/ijspp.3.3.331
Hamilton, M. J., and Ferguson, J. H. (1972). Effects of exercise and cold acclimation on the ventricular and skeletal muscles of white mice (Mus musculus). I. Succinic dehydrogenase activity. Comp. Biochem. Physiol. A Comp. Physiol. 43, 815–824. doi: 10.1016/0300-9629(72)90152-1
Hannon, J. P. (1960). Effect of prolonged cold exposure on components of the electron transport system. Am. J. Physiol. 198, 740–744. doi: 10.1152/ajplegacy.1960.198.4.740
Harri, M. N., and Valtola, J. (1975). Comparison of the effects of physical exercise, cold acclimation and repeated injections of isoprenaline on rat muscle enzymes. Acta Physiol. Scand. 95, 391–399. doi: 10.1111/j.1748-1716.1975.tb10066.x
Ihsan, M., Abbiss, C. R., Gregson, W., and Allan, R. (2020a). Warming to the ice bath: Don't go cool on cold water immersion just yet!: Temperature 7, 223–225. doi: 10.1080/23328940.2020.1727085
Ihsan, M., Markworth, J. F., Watson, G., Choo, H. C., Govus, A., Pham, T., et al. (2015). Regular Post-exercise cooling enhances mitochondrial biogenesis through AMPK and p38 MAPK in human skeletal muscle. Am. J. Physiol. Regul. Integr. Comp. Physiol. 309, R286–R294. doi: 10.1152/ajpregu.00031.2015
Ihsan, M., Watson, G., and Abbiss, C. (2014a). PGC-1α mediated muscle aerobic adaptations to exercise, heat and cold exposure. Cell Mol. Exerc. Physiol. 3:e7. doi: 10.7457/cmep.v3i1.e7
Ihsan, M., Watson, G., and Abbiss, C. R. (2016). What are the physiological mechanisms for post-exercise cold water immersion in the recovery from prolonged endurance and intermittent exercise? Sports Med. 46, 1095–1109. doi: 10.1007/s40279-016-0483-3
Ihsan, M., Watson, G., Choo, H. C., Govus, A., Cocking, S., Stanley, J., et al. (2020b). Skeletal muscle microvascular adaptations following regular cold water immersion. Int. J. Sports Med. 41, 98–105. doi: 10.1055/a-1044-2397
Ihsan, M., Watson, G., Choo, H. C., Lewandowski, P., Papazzo, A., Cameron-Smith, D., et al. (2014b). Postexercise muscle cooling enhances gene expression of PGC-1alpha. Med. Sci. Sports Exerc. 46, 1900–1907. doi: 10.1249/MSS.0000000000000308
Ihsan, M., Watson, G., Lipski, M., and Abbiss, C. R. (2013). Influence of postexercise cooling on muscle oxygenation and blood volume changes. Med. Sci. Sports Exerc. 45, 876–882. doi: 10.1249/MSS.0b013e31827e13a2
Joo, C. H., Allan, R., Drust, B., Close, G. L., Jeong, T. S., Bartlett, J. D., et al. (2016). Passive and post-exercise cold-water immersion augments PGC-1alpha and VEGF expression in human skeletal muscle. Eur. J. Appl. Physiol. 116, 2315–2326. doi: 10.1007/s00421-016-3480-1
Lin, J., Wu, H., Tarr, P. T., Zhang, C. Y., Wu, Z., Boss, O., et al. (2002). Transcriptional co-activator PGC-1 alpha drives the formation of slow-twitch muscle fibres. Nature 418, 797–801. doi: 10.1038/nature00904
Lindsay, A., Othman, M. I., Prebble, H., Davies, S., and Gieseg, S. P. (2016). Repetitive cryotherapy attenuates the in vitro and in vivo mononuclear cell activation response. Exp. Physiol. 101, 851–865. doi: 10.1113/EP085795
Lira, V. A., Benton, C. R., Yan, Z., and Bonen, A. (2010). PGC-1{alpha} regulation by exercise training and its influences on muscle function and insulin sensitivity. Am. J. Physiol. Endocrinol. Metab. 299, E145–161. doi: 10.1152/ajpendo.00755.2009
Machado, A. F., Ferreira, P. H., Micheletti, J. K., de Almeida, A. C., Lemes, Í. R., Vanderlei, F. M., et al. (2016). Can water temperature and immersion time influence the effect of cold water immersion on muscle soreness? A systematic review and meta-analysis. Sports Med. 46, 503–514. doi: 10.1007/s40279-015-0431-7
Malta, E. S., Dutra, Y. M., Broatch, J. R., Bishop, D. J., and Zagatto, A. M. (2021). The effects of regular cold-water immersion use on training-induced changes in strength and endurance performance: a systematic review with meta-analysis. Sports Med. 51, 161–174. doi: 10.1007/s40279-020-01362-0
Manfredi, L. H., Zanon, N. M., Garofalo, M. A., Navegantes, L. C., and Kettelhut, I. C. (2013). Effect of short-term cold exposure on skeletal muscle protein breakdown in rats. J Appl. Physiol. 115, 1496–1505. doi: 10.1152/japplphysiol.00474.2013
Mawhinney, C., Heinonen, I., Low, D. A., Han, C., Jones, H., Kalliokoski, K. K., et al. (2020). Changes in quadriceps femoris muscle perfusion following different degrees of cold-water immersion. J. Appl. Physiol. 128, 1392–1401. doi: 10.1152/japplphysiol.00833.2019
Mawhinney, C., Jones, H., Joo, C. H., Low, D. A., Green, D. J., and Gregson, W. (2013). Influence of cold-water immersion on limb and cutaneous blood flow after exercise. Med. Sci. Sports Exerc. 45, 2277–2285. doi: 10.1249/MSS.0b013e31829d8e2e
McPhee, J. S., and Lightfoot, A. P. (2017). Post-exercise recovery regimes: blowing hot and cold. J. Physiol. 595, 627–628. doi: 10.1113/JP273503
Méline, T., Watier, T., and Sanchez, A. M. (2017). Cold water immersion after exercise: recent data and perspectives on “kaumatherapy”. J. Physiol. 595, 2783–2784. doi: 10.1113/JP274169
Montgomery, P. G., Pyne, D. B., Hopkins, W. G., Dorman, J. C., Cook, K., and Minahan, C. L. (2008). The effect of recovery strategies on physical performance and cumulative fatigue in competitive basketball. J. Sports Sci. 26, 1135–1145. doi: 10.1080/02640410802104912
Murray, A., Fullagar, H., Turner, A. P., and Sproule, J. (2018). Recovery practices in division 1 collegiate athletes in North America. Phys. Ther. Sport 32, 67–73. doi: 10.1016/j.ptsp.2018.05.004
Park, J. B., Kim, H. J., Kim, J. C., Lutan, R., and Kim, C. K. (2005). Muscle characteristics in career breath-hold divers: effect of water temperature. Aviat. Space Environ. Med. 76, 1123–1127.
Peake, J. M. (2017). Cryotherapy: are we freezing the benefits of exercise? Temperature 595, 1–3. doi: 10.1080/23328940.2017.1304194
Peake, J. M. (2020). Independent, corroborating evidence continues to accumulate that post-exercise cooling diminishes muscle adaptations to strength training. J. Physiol. 598, 625–626. doi: 10.1113/JP279343
Peake, J. M., Markworth, J. F., Cumming, K. T., Aas, S. N., Roberts, L. A., Raastad, T., et al. (2020). The effects of cold water immersion and active recovery on molecular factors that regulate growth and remodeling of skeletal muscle after resistance exercise. Front. Physiol. 11:737. doi: 10.3389/fphys.2020.00737
Peake, J. M., Roberts, L. A., Figueiredo, V. C., Egner, I., Krog, S., Aas, S. N., et al. (2017). The effects of cold water immersion and active recovery on inflammation and cell stress responses in human skeletal muscle after resistance exercise. J. Physiol. 595, 695–711. doi: 10.1113/JP272881
Peiffer, J. J., Abbiss, C. R., Watson, G., Nosaka, K., and Laursen, P. B. (2010a). Effect of a 5-min cold-water immersion recovery on exercise performance in the heat. Br. J. Sports Med. 44, 461–465. doi: 10.1136/bjsm.2008.048173
Peiffer, J. J., Abbiss, C. R., Watson, G., Nosaka, K., and Laursen, P. B. (2010b). Effect of cold water immersion on repeated 1-km cycling performance in the heat. J. Sci. Med. Sport 13, 112–116. doi: 10.1016/j.jsams.2008.08.003
Périard, J. D., Racinais, S., Timpka, T., Dahlström, Ö., Spreco, A., Jacobsson, J., et al. (2016). Strategies and factors associated with preparing for competing in the heat: a cohort study at the 2015 IAAF World Athletics Championships. Br. J. Sports Med. 51, 264–270. doi: 10.1136/bjsports-2016-096579
Perry, C. G., Lally, J., Holloway, G. P., Heigenhauser, G. J., Bonen, A., and Spriet, L. L. (2010). Repeated transient mRNA bursts precede increases in transcriptional and mitochondrial proteins during training in human skeletal muscle. J. Physiol. 588(Pt 23), 4795–4810. doi: 10.1113/jphysiol.2010.199448
Petersen, A. C., and Fyfe, J. J. (2021). Post-exercise cold water immersion effects on physiological adaptations to resistance training and the underlying mechanisms in skeletal muscle: a narrative review. Front. Sports Act Living 3:660291. doi: 10.3389/fspor.2021.660291
Pilegaard, H., Saltin, B., and Neufer, P. D. (2003). Exercise induces transient transcriptional activation of the PGC-1α gene in human skeletal muscle. J. Physiol. 546, 851–858. doi: 10.1113/jphysiol.2002.034850
Poppendieck, W., Faude, O., Wegmann, M., and Meyer, T. (2013). Cooling and performance recovery of trained athletes: a meta-analytical review. Int. J. Sports Physiol. Perform. 8, 227–242. doi: 10.1123/ijspp.8.3.227
Poppendieck, W., Wegmann, M., Hecksteden, A., Darup, A., Schimpchen, J., Skorski, S., et al. (2020). Does cold-water immersion after strength training attenuate training adaptation? Int. J. Sports Physiol. Perform. 8, 227–242. doi: 10.1123/ijspp.2019-0965
Puigserver, P., Wu, Z., Park, C. W., Graves, R., Wright, M., and Spiegelman, B. M. (1998). A cold-inducible coactivator of nuclear receptors linked to adaptive thermogenesis. Cell 92, 829–839. doi: 10.1016/S0092-8674(00)81410-5
Roberts, L. A., Nosaka, K., Coombes, J. S., and Peake, J. M. (2014). Cold water immersion enhances recovery of submaximal muscle function after resistance exercise. Am. J. Physiol. Regul. Integr. Comp. Physiol. 307, R998–R1008. doi: 10.1152/ajpregu.00180.2014
Roberts, L. A., Raastad, T., Markworth, J. F., Figueiredo, V. C., Egner, I. M., Shield, A., et al. (2015). Post-exercise cold water immersion attenuates acute anabolic signalling and long-term adaptations in muscle to strength training. J. Physiol. 593, 4285–4301. doi: 10.1113/JP270570
Rowsell, G. J., Coutts, A. J., Reaburn, P., and Hill-Haas, S. (2011). Effect of post-match cold-water immersion on subsequent match running performance in junior soccer players during tournament play. J. Sports Sci. 29, 1–6. doi: 10.1080/02640414.2010.512640
Russell, A. P., Feilchenfeldt, J., Schreiber, S., Praz, M., Crettenand, A., Gobelet, C., et al. (2003). Endurance training in humans leads to fiber type-specific increases in levels of peroxisome proliferator-activated receptor-gamma coactivator-1 and peroxisome proliferator-activated receptor-alpha in skeletal muscle. Diabetes 52, 2874–2881. doi: 10.2337/diabetes.52.12.2874
Schaeffer, P. J., Villarin, J. J., and Lindstedt, S. L. (2003). Chronic cold exposure increases skeletal muscle oxidative structure and function in Monodelphis domestica, a marsupial lacking brown adipose tissue. Physiol. Biochem. Zool. 76, 877–887. doi: 10.1086/378916
Seco-Calvo, J., Mielgo-Ayuso, J., Calvo-Lobo, C., and Córdova, A. (2020). Cold water immersion as a strategy for muscle recovery in professional basketball players during the competitive season. J. Sport Rehabil. 29, 301–309. doi: 10.1123/jsr.2018-0301
Shute, R., Marshall, K., Opichka, M., Schnitzler, H., Ruby, B., and Slivka, D. (2020). Effects of 7°C environmental temperature acclimation during a 3-week training period. J. Appl. Physiol. 128, 768–777. doi: 10.1152/japplphysiol.00500.2019
Shute, R. J., Heesch, M. W., Zak, R. B., Kreiling, J. L., and Slivka, D. R. (2018). Effects of exercise in a cold environment on transcriptional control of PGC-1alpha. Am. J. Physiol. Regul. Integr. Comp. Physiol. 314, R850–R857. doi: 10.1152/ajpregu.00425.2017
Slivka, D., Heesch, M., Dumke, C., Cuddy, J., Hailes, W., and Ruby, B. (2013). Effects of post-exercise recovery in a cold environment on muscle glycogen, PGC-1alpha, and downstream transcription factors. Cryobiology 66, 250–255. doi: 10.1016/j.cryobiol.2013.02.005
Stanley, J., Peake, J. M., and Buchheit, M. (2013). Consecutive days of cold water immersion: effects on cycling performance and heart rate variability. Eur. J. Appl. Physiol. 113, 371–384. doi: 10.1007/s00421-012-2445-2
Stephens, J. M., Sharpe, K., Gore, C., Miller, J., Slater, G. J., Versey, N., et al. (2018). Core temperature responses to cold-water immersion recovery: a pooled-data analysis. Int. J. Sports Physiol. Perform. 13, 917–925. doi: 10.1123/ijspp.2017-0661
Suzuki, J., Gao, M., Ohinata, H., Kuroshima, A., and Koyama, T. (1997). Chronic cold exposure stimulates microvascular remodeling preferentially in oxidative muscles in rats. Jpn. J. Physiol. 47, 513–520. doi: 10.2170/jjphysiol.47.513
Tabben, M., Ihsan, M., Ghoul, N., Coquart, J. B., Chaouachi, A., Chaabene, H., et al. (2018). Cold water immersion enhanced athletes'wellness and 10-m short sprint performance 24-h after a simulated mixed martial arts combat. Front. Physiol. 9:1542. doi: 10.3389/fphys.2018.01542
Tavares, F., Beaven, M., Teles, J., Baker, D., Healey, P., Smith, T. B., et al. (2019). Effects of chronic cold-water immersion in elite rugby players. Int. J. Sports Physiol. Perform. 14, 156–162. doi: 10.1123/ijspp.2018-0313
Tavares, F., Simoes, M., Matos, B., Smith, T. B., and Driller, M. (2020). The acute and longer-term effects of cold water immersion in highly-trained volleyball athletes during an intense training block. Front Sports Act Living 2:568420. doi: 10.3389/fspor.2020.568420
Vaile, J., Halson, S., Gill, N., and Dawson, B. (2008a). Effect of cold water immersion on repeat cycling performance and thermoregulation in the heat. J. Sports Sci. 26, 431–440. doi: 10.1080/02640410701567425
Vaile, J., Halson, S., Gill, N., and Dawson, B. (2008b). Effect of hydrotherapy on recovery from fatigue. Int. J. Sports Med. 29, 539–544. doi: 10.1055/s-2007-989267
Vaile, J., Halson, S., Gill, N., and Dawson, B. (2008c). Effect of hydrotherapy on the signs and symptoms of delayed onset muscle soreness. Eur. J. Appl. Physiol. 102, 447–455. doi: 10.1007/s00421-007-0605-6
Vaile, J., O'Hagan, C., Stefanovic, B., Walker, M., Gill, N., and Askew, C. D. (2011). Effect of cold water immersion on repeated cycling performance and limb blood flow. Br. J. Sports Med. 45, 825–829. doi: 10.1136/bjsm.2009.067272
Vega, R. B., Huss, J. M., and Kelly, D. P. (2000). The coactivator PGC-1 cooperates with peroxisome proliferator-activated receptor alpha in transcriptional control of nuclear genes encoding mitochondrial fatty acid oxidation enzymes. Mol. Cell. Biol. 20, 1868–1876. doi: 10.1128/MCB.20.5.1868-1876.2000
Versey, N. G., Halson, S. L., and Dawson, B. T. (2013). Water immersion recovery for athletes: effect on exercise performance and practical recommendations. Sports Med. 43, 1101–1130. doi: 10.1007/s40279-013-0063-8
Wende, A. R., Huss, J. M., Schaeffer, P. J., Giguere, V., and Kelly, D. P. (2005). PGC-1alpha coactivates PDK4 gene expression via the orphan nuclear receptor ERRalpha: a mechanism for transcriptional control of muscle glucose metabolism. Mol. Cell. Biol. 25, 10684–10694. doi: 10.1128/MCB.25.24.10684-10694.2005
White, G., and Caterini, J. E. (2017). Cold water immersion mechanisms for recovery following exercise: cellular stress and inflammation require closer examination. J. Physiol. 595, 631–632. doi: 10.1113/JP273659
Wu, Z., Puigserver, P., Andersson, U., Zhang, C., Adelmant, G., Mootha, V., et al. (1999). Mechanisms controlling mitochondrial biogenesis and respiration through the thermogenic coactivator PGC-1. Cell 98, 115–124. doi: 10.1016/S0092-8674(00)80611-X
Yamane, M., Teruya, H., Nakano, M., Ogai, R., Ohnishi, N., and Kosaka, M. (2006). Post-exercise leg and forearm flexor muscle cooling in humans attenuates endurance and resistance training effects on muscle performance and on circulatory adaptation. Eur. J. Appl. Physiol. 96, 572–580. doi: 10.1007/s00421-005-0095-3
Keywords: recovery, cryotherapy, hypertrophy, mitochondrial biogenesis, muscle adaptations
Citation: Ihsan M, Abbiss CR and Allan R (2021) Adaptations to Post-exercise Cold Water Immersion: Friend, Foe, or Futile? Front. Sports Act. Living 3:714148. doi: 10.3389/fspor.2021.714148
Received: 24 May 2021; Accepted: 22 June 2021;
Published: 16 July 2021.
Edited by:
Oliver Faude, University of Basel, SwitzerlandReviewed by:
James Robert Broatch, Victoria University, AustraliaErich Hohenauer, THIM University of Applied Sciences for Physiotherapy, Switzerland
Copyright © 2021 Ihsan, Abbiss and Allan. This is an open-access article distributed under the terms of the Creative Commons Attribution License (CC BY). The use, distribution or reproduction in other forums is permitted, provided the original author(s) and the copyright owner(s) are credited and that the original publication in this journal is cited, in accordance with accepted academic practice. No use, distribution or reproduction is permitted which does not comply with these terms.
*Correspondence: Mohammed Ihsan, SWhzYW4ubSYjeDAwMDQwO251cy5lZHUuc2c=