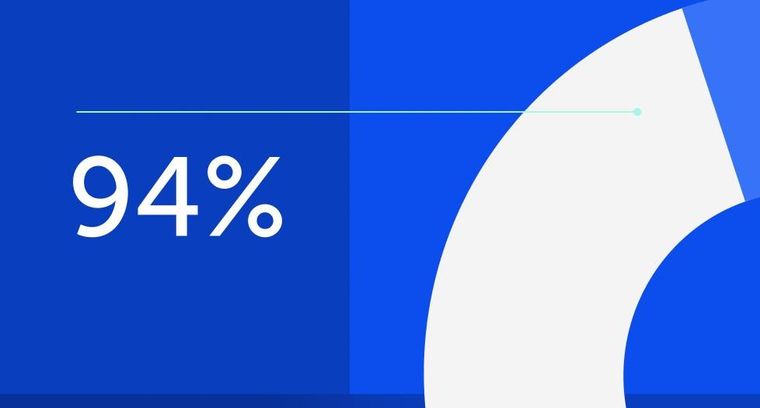
94% of researchers rate our articles as excellent or good
Learn more about the work of our research integrity team to safeguard the quality of each article we publish.
Find out more
ORIGINAL RESEARCH article
Front. Space Technol., 01 April 2025
Sec. Microgravity
Volume 6 - 2025 | https://doi.org/10.3389/frspt.2025.1552919
Introduction: Microgravity science involves studies of physical phenomena in which the Earth’s-gravity is significantly decreased, and these have given uniquely-new advancements towards understanding cellular metabolic processes. Under this status, plants have developed cellular readjustment mechanisms that allows adaptation to these external physical factors. Peanut (Arachis hypogaea L.), corn (Zea mays) and tomato (Solanum lycopersicum) are essential economic crops; with nutritional, medicinal and economic-values of the seeds, germinated roots and seedlings.Methods: In this study, the effects of simulated microgravity (using Clinostat) on peanut, corn and tomato germinated roots were investigated on the resistance of abiotic salinity [NaCl; 30 mM–100 mM] and heavy metal [Pb(NO3)2; 30 mM–100 mM] through antioxidants assay, and activities of root germination and elongation enzymes. The simulated microgravity equipment used in this project is a two-dimensional (2D) Clinostat. The antioxidant-potentials were done using DPPH (2,2-diphenylpicrylhydrazyl), FRAP (Fluorescence Recovery After Photobleaching) and ABTS (2,2-azinobis-(3-ethylbenzothiazoline-6- sulfonic acid) assays. The root germination enzymes (alpha-amylase and lipase) and root elongation enzyme (beta-glucanase) activities were determined and read with ELISA (Enzyme-Linked-Immunosorbent Serologic Assay) spectrophotometer.Results: Results revealed that simulated-microgravity root samples had significantly better antioxidant-potentials than control for all the selected crops. Also, all the unstressed and stressed simulated microgravity samples had significant higher enzyme activities than the gravity (unstressed and stressed) samples.Discussion: The collection and analysis of these root samples provided a valuable resource of improved biochemical properties of the simulated microgravity samples.
Man has always explored, exploited, colonized and controlled their environment by expanding their field of activity. From the very first hominids, technological progress has exposed species to extreme situations and hostile environments. Although mankind’s expansion into space seems irreversible, it is still a major challenge for humans as gravity has fashioned the plant and animal kingdom for more than millions of years, and human has spent much of his live resisting it.
Gravity has supplied a constant input throughout the evolution of life on Earth, providing a directional cue by which organisms organize cells, tissues, and organs; and they elaborate their systemic interactions. Microgravity science entails the investigation of physical occurrence in which the normal gravitational force is greatly reduced. Microgravity research can provide unique new approaches to further basic understanding of development and metabolic processes of cells and organisms, and to further the application (Oluwafemi, 2017). Microgravity research includes a wide range of disciplines such as biological, fluid dynamics, materials and combustion systems. More so, the international endeavour to investigate the space region has given opportunities to study plant growth response under microgravity situation of the low-Earth orbit aboard the Mir, Spacelab, the International Space Station (ISS), U.S. Space Shuttle missions, and various satellite-based lab environments (Pletser and Russomano, 2020).
Biologically, various means by which the force of acceleration due to gravity is perceived, transduced, and transmitted throughout the body of the organism such as plant, remains an active and important research enterprise, drawing upon the latest tools in cell biology, biochemistry, molecular genetics, signal transduction, and physiology to advance the understanding of this complex response (Enemali et al., 2020). The use of microgravity simulators and models allow the assessment of microgravity induced deconditioning effects, and reveal gravitational mechanisms in the body’s physiological systems, as well as mechanisms involved in adaptation of the body to microgravity.
Gravity has constantly influenced both physical and biological phenomena through Earth’s history (Oluwafemi, 2018). Plants grow in gravity presence, and develop molecular and cellular machinery to regulate their growth harmoniously to physical forces on the Earth (Bradbury et al., 2020). By gravity the growth of plant organs is harmonized, making plants to use the spaces below and above the Earth’s surface. It was shown that relocalization of statoliths (starch-filled plastids located in columella cells) and changes in auxin distribution play important roles in gravity signal transduction.
Basic growth and development processes of plants subjected to microgravity are usually investigated (Oluwafemi, 2021), while some of the biochemistry experiments done under microgravity are still awaiting clarification at the biochemical and genetic levels (Mukhopadhyay and Bagh, 2020). One of core changes in cells exposed to microgravity is profoundly effected on the physical changes on reduced gravity dependent hydrodynamic sedimentation, cell-cell and cell-substrate adhesions, tissue formation and multicellular aggregations (Pletser and Russomano, 2020; Bradbury et al., 2020). As a consequence of these transformations, many genetic, proteins and enzymatic pathways change resulting in several alterations in cells/tissues shapes and functions (Pletser and Russomano, 2020).
Although some reference/constitutive expressed genes are found stable under microgravity exposure, the biochemical and molecular biological experiment showed that Orbitally Manifested Gene 1 (OMG1) expression of Arabidopsis thaliana root ecotype Wassilewskija (WS) has a 2-4 folds change in space flight compared to ground controls, in the Advanced Plant Experiment 01 (APEX01) experiments, and it regulates the expression of reactive oxygen species (ROS)-associated genes GRX480 and MYB77 which are known to be induced in spaceflight and upon wounding (Li Sng, 2017). A study has found that an alteration of ROS signalling in response to the spaceflight environment could affect plant growth and development indirectly through changes in hormonal influx within the plants (Li Sng, 2017). A study of gravity effects on the root growth and the amino acids of the plants of corn, Panicumamericanum (pearl millet), Pisumsativum (pea), jasmine rice and rice variety Anber 33 was done and it was found that the clinorotated samples showed significantly different characteristics of amino acids compared to those of the 1g control (Li Sng, 2017).
In vitro and in vivo experiments have revealed that antioxidants aid in the prevention of free radical harm that is connected with heart disease, cancer and degenerative diseases (Dhalaria et al., 2020; Oluwafemi et al., 2024). Antioxidants can be found in roots, root bark, fruits, vegetables and legumes, but also culinary herbs and medicinal herbs can contain high levels of antioxidants (Amsalu and Asfaw, 2020).
The peanut (Arachis hypogaea L.) or groundnut is a multi-purpose oil seed plant which has been adapted to soils in tropical and temperate zones around the world. Peanut is a legume that is has in it various bioactive compounds and is also rich in antioxidant as many fruits (Arnarson, 2019). Peanut contains many flavonoids, phenolic acids and stilbenes (e.g., resveratrol) that have several health benefits. These are all antioxidative phytochemicals (Mahatma et al., 2016). Corn (Zea mays) is a cereal that contains most antioxidant than other cereals. Corn as it contains certain vitamins that are very good for eyesight (Oluwafemi and Olubiyi, 2019). Tomato (Solanum lycopersicum) is classified as both a fruit and a vegetable, and the major constituent of this is vitamins and minerals that are of antioxidant values. The nutrition, medicinal and economic-values of seeds and germinated roots cannot be overemphasized (Omotayo and Aremu, 2021; Oluwafemi et al., 2018, 2022).
The life cycle of every plant begins with a seed, of which every seed have a miniature plant known as embryo. This makes seeds to be highly important. Plant growth is directed by different processes that involves organic material synthesis by green aerial parts and the root uptake of nutrients and moisture. Plant’s root is the part of the plant that attaches the plant to the ground for support conveying water, nourishment or nutrient to the rest of the plant through the branches and fibres (Oluwafemi and Oyewole, 2022). These processes interact in that carbonIVoxide assimilation is necessary for root growth and uptake by the roots for shoot growth. If fixation of carbonIVoxide is poor, roots grow badly. Reciprocally, when the roots take-up only small quantity of nutrients and moisture, the aerial growth portion of the plant is impaired even if aerial conditions are favourable. Symptoms observed above the ground may be caused by soil factors that affect the development of the root system and consequently the absorptive capacity of the root system.
Agricultural crops usually need a well-developed root system in order to exploit deeper layers more fully. They are therefore more resistant to stress periods that occur often during growth and are also more likely to still yield well. In root ecology, certain morphological characteristics are important. They can be studied on the field or in containers (Freschet et al., 2021; Oluwafemi et al., 2023).
Therefore, because of the importance of plant’s root; this study is important to be carried out on the roots of peanut, corn and tomato to know root’s implications to the varying factors (gravity or simulated microgravity; abiotic stresses of high salinity - sodium chloride and heavy metal–lead(II)nitrate; and plant physiological characterizations of root germination and elongation enzymes, and antioxidant potential (through free radical scavenging activities of the inherent phytochemicals) of the roots specific to this study. In plants, the root is the major site for access of heavy metals. Toxic heavy metals (HMs) presence is a vital factor that can cause harm to plants by altering the plant’s physiological and metabolic processes.
The excess of heavy metals affects root functions on various levels and causes the accumulation of ABA. Haven observed that different metal has specific effects on plant; lead effect as a chemical stress for the plant was determined in this study, specifically to determine the possibility of simulated microgravity to reverse its effect (withstand this chemical stress). Lead(II)nitrate (Pb(NO3)2) was used as a result of its solubility in water for access into the cells of the plants.
High salinity adversely affects plant growth and development. The buildup of salt ions in plants can lead to osmotic stress and ionic toxicity which then induce nutritional deficiencies. In addition to these physiological disturbances, abiotic factors such as excess of the salts can trigger secondary stresses such as oxidative stress due to accumulation of reactive oxygen under normal condition (Taibi et al., 2016). High salinity and drought generate similar impact. In this study, sodium chloride was used to stress the plant to determine the possibility of simulated microgravity to reduce the negative effects, with the determination through biochemical characterizations using antioxidant assays and activities of the root germination and elongation enzymes.
Seed germination is a process whereby the embryo inside the seed develops into plumule and radicle. Seeds absorb water and afterwards swells, the inactive tissues become active, and the cells begin to divide. The radicle arises from the micropyle and goes downwards into the soil. The radicles change into roots that supply nutrients and water to the plants. Seeds are storage reserves with heterogeneous compounds such as soluble carbohydrates, starch, proteins, phosphate, and lipids. Enzymes play important role in seed germination, root elongation, growth and development. Enzymes in seed like amylase, protease and lipase are responsible for solubilizing spare food substances in the form of starch, protein and lipid respectively; to deliver energy and other crucial food substances to the germinating embryo. The proteins in the seeds are catalyzed by protease enzymes, to amino acids and peptides which are utilized by the growing embryo. The amino acids are further used for the biosynthesis of pyrimidines and purines bases, proteins, enzymes, and hormones. The starch is catalyzed by amylase enzyme that provides food substances for the growth and development of the embryo. Likewise, enzyme lipases are accountable for the metabolism of the triacylglycerols to fatty acids and glycerol. This also serves as the source of energy for the growing embryo (Joshi, 2018).
The process of seed germination consists of numerous sequential phases, including the activation of enzymes through water absorption, the growth of the radicle into a root for water uptake from the soil, and the development of the plumule into a shoot for photosynthesis in the plant. During germination, the seed absorbs water and produces various enzymes that aid in the decomposition of stored food into simpler molecules. The food molecules are delivered to the budding seedling for growth and maturation. Multiple minerals, enzymes, and proteins play essential roles at different stages of seed germination. During the process of seed germination, there is a significant increase in the levels of minerals, vitamins, proteins, and enzymes, ranging from around 25 to 4,000 percent (Joshi, 2018). According to Zhang et al. (2012), the elongation of seedlings is necessary for shoots to develop. This process is accompanied by an increase in beta-glucanase activity, which promotes root elongation.
Plant alpha-amylase has the Enzyme Commission (EC) number 3.2.1.1. Without enzymes germination process cannot continue. Seed’s cotyledons have the food substances that gives energy for growth and development. The reserved food is mostly found as starch (insoluble sugars) that are required to be converted into soluble form before it can be used for embryo’s growth and development. Amylase enzymes are produced in the seeds for the catalysis of starch (amylase substrate) in seeds after the uptake of water from soil. Amylase breaks down starch into maltose which then facilitates germination process (Murtaza and Asghar, 2012; Joshi, 2018). Alpha-amylase is measured by using 4,6-O-benzylidene-α-4-nitrophenyl-maltoheptaoside in the presence of α-glucosidase a thermostable.
The plant lipase enzyme is classified with the EC number 3.1.1.1. Peanuts, as oil seeds, are composed of the primary component called the kernel and a protective covering known as husk or tegument. The kernel comprises the endosperm and the embryo. The enzyme lipase’s actions are more extensively researched during seed germination, particularly when the oil concentrations reach exceptionally high levels. Oleosomes contain triacylglycerols, which make up approximately 20%–50% of their dry weight. During germination, the process of breaking down or hydrolyzing triacylglycerols occurs. This breakdown produces energy that is essential for the manufacture of sugars, amino acids (such as asparagine, aspartate, glutamine, and glutamate), and carbon chains that play a vital role in the growth and development of the embryo. The key hydrolytic enzymes involved in lipid catalysis during seed germination are lipases. These enzymes catalyse the hydrolysis of ester carboxylate bonds, resulting in the release of organic alcohols and fatty acids. Additionally, lipases can also perform esterification (reverse reaction) and catalyse various trans-esterification reactions (Joshi, 2018). Therefore, p-nitrophenyl palmitate (p-NPP) serves as a substrate for lipase. The determination of lipase activity can be achieved by utilising a pair of enzyme reactions that yield a colorimetric product in direct proportion to the level of enzymatic activity.
Plant beta-glucanase enzyme has EC number 3.2.1.39. Beta-glucanase has no power to break the seeds for germination. Beta-1,3-Glucanase is an enzyme that catalyzes beta-1,3-Glucan; hence it is the substrate of Beta-1,3-Glucanase. Beta-1,3-Glucan is a thick layer that covers seed. This layer is later degraded during germination by Beta-1,3-Glucanase activities. Beta-1,3-Glucanase also play a very crucial role during seed dormancy, regulation and germination, as the envelope of beta-1,3-Glucan delays the radicle emergence; it is activity is associated with root elongation (Zhang et al., 2012). A method of the analysis of this enzyme is based on measuring the glucose released by the substrate glucan. The released glucose in an alkaline salt solution reduces 3,5-dinitrosalicyclic acid (DNSA) to 3-amino-5-nitrosalicylic acid. The sensitivity of the reaction is increased by adding phenol; while the addition of sodium potassium tartarate or sodium bisulphite could be added to stabilize the red colour (DG-OIV, 2022).
In this study, the effects of simulated microgravity (using Clinostat) on the selected crops’ (peanut, corn and tomato) germinated and elongated roots, were determined via biochemical characterizations. Antioxidant assays and the activities of the root germination and elongation enzymes were the biochemical characterizations carried out. This is to investigate to what extent the simulated microgravity will resist or reverse the abiotic (chemical) stresses [high salinity (sodium chloride) and heavy metal (lead(II)nitrate]; the results were compared to the unstressed and stressed germinated root samples under normal Earth’s gravity (control). The simulated microgravity equipment used in this project is a two-dimensional (2D) Clinostat (Oluwafemi and Neduncheran, 2022). The collection and analysis of these samples provided a valuable resource.
The seed variety Samnut 23 of peanut; Samas 39 variety of corn; and UC 82 B variety of tomato were all bought at the International Institute of Tropical Agriculture (IITA), Kubwa, Abuja, Nigeria. The choice of the selected crops was also dependent on their usability on the 2D Clinostat (Oluwafemi et al., 2020). These plants seeds are small, easy to handle with fast-growing germination time not longer than 3–7 days.
The simulated microgravity was created using 2D Clinostat. It was manufactured by Advanced Engineering Services., Co. Ltd. Model UN-KTM2 REV. NC. 2012.11. Figure 1 shows seedlings rotation on the 2D Clinostat.
Figure 1. Germinated Peanut Mounted on Uniaxial Clinostat and its Control Box, with Rotation Position Horizontal (i.e., rotational axis angle of 90°).
Seeds of each species (peanut, corn and tomato) were separately planted into 2 petri dishes using plant substrate, agar-agar, following the standard preparation method described in Oluwafemi (2021). Subsequently, the seeds were sown in the substrate and nurtured in a moist chamber (wet chamber) in a vertical orientation. 2 days after planting, the seeds were germinated with short roots. The 2 petri dishes were obtained and marked as “1g” and “Cli.” The 1g sample, which served as the control for root growth-rate, was kept in a vertical position. The Clinorotated sample was positioned at the centre of the Clinostat using double-sided tape. The observation was conducted for a duration of 24 h, adhering to the following specified parameters. The humidity was at 85%, the temperature was 25°C, and the light-dark cycle consisted of 12 h of light with an intensity of 75 lux, followed by 12 h of darkness. The Clinorotated sample exhibited the following conditions in addition: a rotation-speed of 85 rpm, a rotational-axis angle of 90°, with a clockwise direction of rotation. Following the observation period, the roots of the plants from the 1g and Cli samples were individually severed and placed into vials for subsequent biochemical studies. Of keynote is that this procedure was carried out as many times as possible to get the required quantity of the germinated roots for the biochemical characterizations (Oluwafemi et al., 2019).
For the stress application: For each crop (peanut, corn and tomato), another two petri dishes were prepared by adding 100 mmolL-1 of Pb(NO3)2 to the agar prepared [one as gravity control sample (1gPN)] and the other as simulated microgravity experimental sample (CliPN). This set-up was prepared in triplicates. After germination inside the wet chamber, the experimental samples were subjected to simulated microgravity for 24 h. Afterwards, the specified biochemical characterizations was carried out on the roots of the germinated seeds to determine if there are any significant effects of Pb(NO3)2 stress on the samples. The same method was used to apply 100 mmolL−1 NaCl, and the samples were labelled as “1gNC” and “CliNC.” For the tomato the applied concentration of Pb(NO3)2 and NaCl was 30 mmolL−1.
The germinated root samples (Figure 2) were dried in an oven, and afterwards grinded into dry powder. Sample powder was stored for subsequent biochemical analyses. These were the experimental samples: gravity; simulated microgravity; gravity + lead(II)nitrate; simulated microgravity + lead(II)nitrate; gravity + sodium chloride; simulated microgravity + sodium chloride.
The methanolic extracts of the sample were analysed for their total-phenolic content (TPC) using the Folin-Ciocalteu assay, following the protocol published by Babu et al. (2012). The 0.25 mL extract was transferred into a 25 mL volumetric flask, followed by the addition of 5 mL of distilled water. The Folin-Ciocalteu’s phenol reagent (1.25 mL) was introduced and thoroughly coalesce. 2 min later, 3.75 mL of a sodium carbonate solution with a concentration of 20% (w/v) was introduced. Subsequently, the components were thoroughly coalesced and supplemented with distilled water to achieve the desired volume, followed by another round of mixing. After the addition of sodium carbonate, the mixture was allowed to stand for a duration of 2 h. Subsequently, the absorbance of the mixture was measured at a wavelength of 760 nm using a Lambda EZ150 spectrophotometer. The standard utilised in this experiment was tannic acid, and the results were reported as mg of tannic acid equivalents per Gram.
The extract’s overall flavonoid content was measured using a colorimeter assay, following the method outlined by Golla and Sharabu (2017) with a minor alteration. The methanolic extract of the sample, measuring 0.5 mL, was combined with 0.5 mL of methanol, 50 μL of 10% aluminium chloride (AlCl3), 50 μL of 1 molL−1 potassium acetate, and 1.4 mL of water. The mixture was then incubated at room temperature for 30 min. Subsequently, the absorbance of the reaction mixture was quantified at a wavelength of 415 nm using a Lambda EZ150 spectrophotometer. The total flavonoid content (TFC) was evaluated through a seven-point standard curve (ranging from 0 to 100 μg/mL) using quercetin as the standard. The samples were analysed in triplicates, and the results were represented as mg of quercetin equivalents per Gram of the sample.
The reducing property of the sample was determined by evaluating its capacity to reduce a solution of iron(III) chloride (FeCl3). The methanolic extract of the sample (2.5 mL) was combined with 2.5 mL of 200 mM sodium phosphate buffer (pH 6.6) and 2.5 mL of 1% potassium ferricyanide. The solution was subjected to incubation at a temperature of 50°C for a duration of 20 min. Subsequently, 2.5 mL of a solution containing 10% trichloroacetic acid was introduced. The combination underwent centrifuged with a force of 353 x g for a duration of 10 min 5 mL of the generated supernatant was combined with an equal volume of water and 1 mL of 0.1% ferric chloride. Lambda EZ150 spectrophotometer was used to measure the absorbance at a wavelength of 700 nm. A comparable procedure was carried out on the standard ascorbic acid solution. The ferric reducing antioxidant power (FRAP) was quantified as mg of ascorbic acid equivalent (AAE) per Gram of the sample.
The sample’s capacity to scavenge free radicals against DPPH (1, 1-diphenyl-2-picryhydrazyl) was assessed using the method published by Lakshamanashetty et al. (2010) with minor adjustments. The stock solution was made by dissolving 24 mg of 2,2-diphenylpicrylhydrazyl (DPPH) in 100 mL of methanol and subsequently stored at a temperature of −20°C until it was required. The working solution was achieved by combining 10 mL of the stock solution with 45 mL of methanol, resulting in an absorbance of 1.1 units at a wavelength of 515 nm as measured by the spectrophotometer. The methanolic extract (300 mL) was mixed with 2,700 mL of the DPPH solution and left to react for 6 h in the absence of light. Subsequently, the measurement of absorbance was conducted at a wavelength of 515 nm using the Lambda EZ150 spectrophotometer. The results are quantified in terms of MTE (Milligram of Test Element) per Gram of sample. The extract was substituted with distilled water in the blank, and the percent inhibition was determined using the subsequent formula:
Where Absblank and Abssample stands for absorption of the blank samples and absorption of tested extract solution respectively. Ascorbic acid was the standard used with the median inhibitory concentration (IC50) plotted concentration with percent inhibition.
The ABTS test was employed to assess the antioxidant activity of the samples. A solution of 8 mM ABTS and 3 mM potassium persulphate (K2S2O8) was made by combining them to make the ABTS stock solution. The solution was combined in a volumetric flask, which was covered with foil to prevent light exposure. The mixture was left to react for at least 12 h in a dark location. A volume of 5 mL from the stock solution was combined with 145 mL of phosphate buffer (pH 7.4) to create the working solution. There was preparation in acidified methanol. A series of trolox (6-hydroxy-2,5,7,8-tetramethylchroman-carboxylic acid) standard solutions with concentrations ranging from 100 to 1,000 μM was prepared. The solution containing 2.9 mL of the working solution was combined with 0.1 mL of the methanolic extracts and 0.1 mL of the trolox standard in a test tubes. The mixture was then mixed vigorously and left undisturbed for a duration of 30 min. The standards and samples were analysed for absorbance at a wavelength of 734 nm using a Lambda EZ150 spectrophotometer. The results are expressed in μM trolox equivalents per Gram of sample, based on the dry weight.
Preparation of reagents
Buffer preparation
0.1 M buffer (pH 5.5) of sodium acetate salt was prepared.
3,5-dinitrosalicylic acid (DNSA) solution
Reagent was prepared by dissolving 1.0 g of 3,5-DNSA, and 0.5 g of sodium sulphite in 100 mL of 0.64 M NaOH solution. The DNSA reagent was stored into a dark coloured bottle.
Potassium sodium tartrate (Rochelle salt)
40 g of potassium sodium tartrate was dissolved in 100 mL of distilled water (freshly prepared) for colour stabilization.
Root Samples
2 g of root sample was homogenized with 20 mL of 0.1 M of sodium acetate buffer, crushed by mortar and pestle, and left for 15 min. Centrifugation was done at 10,000 rpm for 15 min. The obtained supernatant represented the crude extract and was assayed for the specified enzyme. It was stored at 4°C.
Note: These are stop assays, hence only one absorbance reading was taken. This enzyme assay was carried out in triplicates.
Alpha-amylase activity was determined by the slight modification of the method of Kafilzadeh and Dehdari (2015). 0.5 mL of the alpha-amylase enzyme extract (sample) was measured into test tube already containing 0.5 mL of sodium acetate buffer (0.1 M, pH 5.5) and 0.5 mL of 1% soluble starch solution (prepared by dissolving 1 g of starch into 100 mL of distilled water). This mixture was incubated at 50°C for 30 min. Afterwards, 1.0 mL of 3,5-dinitrosalicyclic acid (DNSA) reagent was added (to stop the reaction), these constituent was boiled for 10 min. Thereafter 1 mL of 40% sodium potassium tartrate was added to stabilize the red colour formed. The mixture was then allowed to cool, and the absorbance was read at 540 nm using ELISA spectrophotometer MOBI. The concentration of enzyme was gotten by extrapolating the absorbance values from the standard curve of glucose prepared by different concentrations of glucose from 10 μg/mL to 40 μg/mL (0.0000556 mM–0.000222 mM); and afterwards calculated using the straight line equation formula (y = mx + c), where y is the absorbance, and x is the enzyme concentration.
The substrate blank contained 0.5 mL of distilled water instead of starch solution, 0.5 mL crude enzyme, and 0.5 mL buffer.The enzyme/sample blank contained 0.5 mL of distilled water instead of enzyme, 0.5 mL starch, and 0.5 mL buffer.
Lipase activity was determined by the colorimetric method with a slight modification of the method of Alami et al. (2017). This method is based on the cleavage of p-nitrophenyl palmitate (p-NPP). 0.5 mL of lipase enzyme extract (sample) was pipetted into 1 mL of sodium acetate buffer solution (0.1 M, pH 5.5), and rapidly mixed, then 0.5 mL of 2 mM p-NPP was added and incubated at 50°C for 30 min. Thereafter, the reaction was stopped with 4 mL of 1 M NaOH and absorbance was read at 410 nm using ELISA spectrophotometer MOBI. The blank reaction used contained the same assay mixture without the enzyme extract. The concentration of enzyme was gotten by extrapolating the absorbance values from the standard curve of p-NPP prepared by different concentrations of glucose from 438,180 μg/mL to 1,752,720 μg/mL (2 mM–8 mM); and afterwards calculated using the straight line equation formula (y = mx + c), where y is the absorbance, and x is the enzyme concentration.
Beta-glucanase activity was determined by the slight modification of the method of Konig et al. (2002). 0.5 mL of the beta-glucanase enzyme extract (sample) was measured into test tube already containing 0.5 mL of sodium acetate buffer (0.1 M, pH 5.5) and 0.5 mL of 1% glucan. This mixture was incubated at 50°C for 30 min. Afterwards, 1.0 mL of 3,5-dinitrosalicyclic acid (DNSA) reagent was added (to stop the reaction), these constituent was boiled for 10 min. Thereafter 1 mL of 40% sodium potassium tartrate was added to stabilize the red colour formed. The mixture was then allowed to cool, and the absorbance was read at 540 nm using ELISA spectrophotometer MOBI. The concentration of enzyme was gotten by extrapolating the absorbance values from the standard curve of glucose prepared by different concentrations of glucose from 10 μg/mL to 40 μg/mL (0.0000556 mM–0.000222 mM); and afterwards calculated using the straight line equation formula (y = mx + c), where y is the absorbance, and x is the enzyme concentration.
The substrate blank contained 0.5 mL of distilled water instead of glucan, 0.5 mL crude enzyme, and 0.5 mL buffer.
The enzyme/sample blank contained 0.5 mL of distilled water instead of enzyme, 0.5 mL glucan, and 0.5 mL buffer.
All analyses were run in triplicates. Relevant statistical method was applied [one-way or two-way Analysis of Variance (ANOVA) with Duncan’s Multiple Range Test (DMRT)]. The average value and the standard deviation (STDEV) were determined using the Microsoft Excel software (Microsoft Corporation, Redmond, WA), Statistical Package for Social Sciences version 22.0, 23.0 and 25.0 (SPSS Inc., Chicago, IL, United States). Values were found to be statistically significant when test and controlled experimental data were compared at p value (confidence level) ≤0.05.
The alphabet in the histogram indicates that there is a significant difference between the two groups at p ≤ 0.05.
Comparison:
a = mean difference is significant when compared with Gravity (1g)
b = mean difference is significant when compared with Simulated Microgravity (Cli)
c = mean difference is significant when compared with Gravity + Lead(II)nitrate (1gPN)
d = mean difference is significant when compared with Simulated Microgravity + Lead(II)nitrate (CliPN)
e = mean difference is significant when compared with Gravity + Sodium Chloride (1gNC)
f = mean difference is significant when compared with Simulated Microgravity + Sodium Chloride (CliNC)
The germination of the stressed samples were not the same duration with the unstressed samples. For peanut, the unstressed sample germinated after 2 days, the NaCl stressed sample germinated after 4 days, while the Pb(NO3)2 stressed sample germinated after 6 days (Table 1; Figure 3). For corn, the unstressed sample germinated after 3 days, the NaCl stressed sample germinated after 5 days, while the Pb(NO3)2 stressed sample germinated after 8 days. For tomato, the unstressed sample germinated after 6 days, the NaCl stressed sample germinated after 8 days, while the Pb(NO3)2 stressed sample germinated after 10 days.
The root antioxidant potentials of the 6 experimental treatments of peanut, corn and tomato species are shown in Table 2 and Figure 4.
Table 3 and Figure 5 shows the root enzymes activity of the 6 experimental treatments of peanut, corn and tomato species. Figure 6 shows the glucose standard curve that was used for the extrapolation of the concentration of alpha-amylase and beta-glucanase, while Figure 7 shows the p-NitroPhenyl Palmitate (p-NPP) standard curve used for the extrapolation of the concentration of lipase.
For the peanut roots, there were significantly higher antioxidant values of the simulated microgravity samples than the gravity samples for: TPC, TFC, FRAP lead heavy metal stressed roots; and TPC, TFC, FRAP, DPPH, ABTS sodium chloride salinity stressed roots. For the corn roots, there were significantly higher antioxidant values of the simulated microgravity samples than the gravity samples for: TPC, TFC, FRAP, DPPH, ABTS unstressed roots; TPC, FRAP, DPPH, ABTS lead heavy metal stressed roots; and TPC, TFC, FRAP, ABTS sodium chloride salinity stressed roots. For the tomato roots, there were significantly higher antioxidant values of the simulated microgravity samples than the gravity samples for: TFC, FRAP, DPPH, ABTS unstressed roots; TPC, TFC, FRAP, DPPH, ABTS lead heavy metal stressed roots; and TPC, FRAP, ABTS sodium chloride salinity stressed roots.
Hence: the peanut stressed simulated microgravity root samples for TPC, TFC and FRAP could significantly withstand or resist the free radical stresses than the gravity samples; all the corn simulated microgravity root samples for TPC, FRAP and ABTS could significantly withstand or resist the free radical stresses than the gravity samples; and all the tomato simulated microgravity root samples for TFC, FRAP and ABTS could significantly withstand or resist the free radical stresses than the gravity samples (Table 2; Figure 4). Therefore, for the three species, the simulated microgravity root samples for FRAP could significantly withstand or resist the free radical stresses than the gravity samples. It can be deduced that simulated microgravity confers protection of root cells from free radicals (oxidative stress) for legumes, cereals and vegetables (Nguyen et al., 2021; Oluwafemi et al., 2024).
It was observed that alpha-amylase, lipase and beta-glucanase activity were significantly higher in all the species unstressed simulated microgravity samples than the unstressed gravity samples. The work of Nakajima et al. (2021) as well confirmed that seedlings under clinorotation exhibited a significantly higher amylase activity. This could be linked to the faster germination and elongation recorded for the simulated microgravity samples of all the species during the nursery periods (Kiss et al., 2019). Alpha-amylase breaks down the starch inside the seed (after uptake of water) into maltose which facilitates germination process (Murtaza and Asghar, 2012; Joshi, 2018); hence the significantly increased activity in peanut and corn than tomato, can be seen in its expression via the number of the germination days of 2, 3 and 6 respectively for peanut, corn and tomato. The activity of alpha-amylase enzyme in the germinated roots were also deduced to be significantly low in the unstressed samples (gravity and microgravity) of the three species. Alpha-amylase activity was observed to be significantly high in all the heavy metal stressed samples. This is synonymous to the discussion of Joshi (2018) that various environmental stresses increase the activity of alpha-amylases. Alpha-amylase activity was found significantly highest in the peanut species; with the highest activity value in the heavy metal stressed (microgravity), next to the salinity stressed (microgravity), and then the unstressed (microgravity). Alpha-amylase activity was found next higher in corn species after peanut; with the highest activity value in the heavy metal stressed (gravity), next to the salinity stressed (microgravity), and then to the unstressed (microgravity). The least activity of alpha-amylase was found in the tomato species; the highest activity value in the tomato species was also in the heavy metal stressed (microgravity), next to the salinity stressed (gravity), and then to the unstressed (microgravity). This observed result of alpha-amylase activity in these species is in correlation with what was observed during the nursery periods of these plants (germination periods); as the germination of peanut was more rapid than corn; and the very least germination was observed in tomato species (Table 3; Figure 5A).
Lipases are accountable for the metabolism of the triacylglycerols to fatty acids and glycerol (Joshi, 2018); hence the highest activity was observed in peanut germinated roots. This may be due to the fact that peanut is rich in triacylglycerols than corn and tomato (Arya et al., 2016). Lipase enzyme activity was found significantly highest in all the species’ unstressed simulated microgravity samples than in their unstressed gravity samples; and the least significant activity found in all the species’ heavy metal samples. Peanut species had the significant highest lipase activity; corn species’ lipase activity had the next significant higher; while tomato species had the lowest significant lipase activity (Table 3; Figure 5B).
Since shoots cannot come up without seedlings elongation, hence beta-glucanase activity as is associated with root elongation (Zhang et al., 2012) was significantly highest in the germinated root of the unstressed samples (microgravity). Beta-glucanase’s activity was found significantly highest in the tomato species; with the highest activity value in the unstressed (microgravity), next to the heavy metal stressed (microgravity), and then the salinity stressed (microgravity). Beta-glucanase’s activity was found next significantly higher in corn species after tomato; with the highest activity value in the unstressed (microgravity), next to the salinity stressed (microgravity), and then to the heavy metal stressed (gravity). The least significant activity of beta-glucanase was found in the peanut species; the highest activity value in the peanut species was in the unstressed (microgravity), next to the heavy metal stressed (gravity), and then to the salinity stressed (microgravity). This observed result of beta-glucanase in these species is in correlation with what was observed during the nursery periods of these plants (elongation periods); as the elongation of tomato was more rapid than the other species. Next to tomato as observed was the corn elongation, and the least elongated species was peanut (Table 3; Figure 5C).
In comparison, recent study reported the seed germination of Pinus pinea L. under altered gravity, and found a higher seed germination rate under microgravity that was due to a higher activity of enzymes related to seed germination (Baba et al., 2022). This result, hence, is in support of this experiment.
The currently pressing issues in the world include food insecurity, sustaining the environment and the economy. Global population growth, limited production quantity and quality, and the exposure of plants to environmental stressors (such as salt and heavy metal) are some of the causes. Hence, there is an urgent need to produce more food while reducing the impact on the environment and ecosystem. As food security is having access to good quantity and quality of food as required; and from the knowledge obtained from microgravity simulations of crops, it is discovered that it bio-fortifies. Therefore in this study, the use of space technology application product called Clinostat (an equipment that uses rotation to negate the gravitational pull effects on plant growth and development) simulated microgravity model was employed on the germinated roots of peanut, corn and tomato as economic crops to ascertain its propensity on the roots’ nutritional qualities, yield and their abilities to withstand or resist environmental stresses (chemical–salinity and heavy metal) over the conventional (control) plants. This model was also employed to generate clear and beneficial differences with the roots’ and their controls by the determination of specific biochemical characterization and processes [antioxidant potentials and activities of root germination and elongation enzymes]. The study therefore has social economic benefits through the improved biochemical characteristics of the roots of the simulated microgravity samples that is proposed to improve the agronomic characteristics of the crops. The data generated contributes to meaningful knowledge that is needed to be acknowledged.
The original contributions presented in the study are included in the article/supplementary material, further inquiries can be directed to the corresponding author.
FO: Conceptualization, Formal Analysis, Investigation, Methodology, Resources, Writing–original draft, Writing–review and editing. OI: Conceptualization, Methodology, Project administration, Supervision, Writing–review and editing. AO: Formal Analysis, Writing–review and editing.
The author(s) declare that no financial support was received for the research and/or publication of this article.
Authors appreciate Federal University Oye-Ekiti (FUOYE), Nigeria for academic support.
The authors declare that the research was conducted in the absence of any commercial or financial relationships that could be construed as a potential conflict of interest.
The author(s) declare that no Generative AI was used in the creation of this manuscript.
All claims expressed in this article are solely those of the authors and do not necessarily represent those of their affiliated organizations, or those of the publisher, the editors and the reviewers. Any product that may be evaluated in this article, or claim that may be made by its manufacturer, is not guaranteed or endorsed by the publisher.
Alami, N. H., Nasihah, L., Umar, R. L. A., Kuswytasari, N. D., Zulaika, E., and Shovitri, M. (2017). Lipase production in lipolytic yeast from Wonorejo mangrove area. AIP Conf. Proc. 1854, 020001. doi:10.1063/1.4985392
Amsalu, N., and Asfaw, Z. (2020). Review of the antioxidant properties of wild edible plants in Ethiopia. Afr. J. Med. Health Sci. 19 (6), 84–102. Article number: 06ADAE264795. ISSN: 2384-5589. doi:10.5897/AJMHS2019.0082
Arnarson, A. (2019). Peanuts 101: nutrition facts and health benefits. Healthline. Available online at: https://www.healthline.com/nutrition/foods/peanuts#:∼:text=Peanuts%20contain%20various%20bioactive%20plant,peanuts%20are%20raw%20(%2015%20 (Accessed March 11, 2023).
Arya, S. S., Salve, A. R., and Chauhan, S. (2016). Peanuts as functional food: a review. J. Food Sci. Technol. 53 (1), 31–41. doi:10.1007/s13197-015-2007-9
Baba, A. I., Mir, M. Y., Riyazuddin, R., Cséplő, A., Rigó, G., and Fehér, A. (2022). Plants in microgravity: molecular and technological perspectives. Int. J. Mol. Sci. 23 (18), 10548. doi:10.3390/ijms231810548
Babu, M. A., Suriyakala, M. A., and Gothandam, K. M. (2012). Varietal impact on phytochemical contents and antioxidant properties of Musa acuminate (banana). J. Pharmaceu. Sci. Res. 4 (10), 1950–1955.
Bradbury, P., Wu, H., Choi, J. U., Rowan, A. E., Zhang, H., Poole, K., et al. (2020). Modeling the impact of microgravity at the cellular level: implications for Human Disease. Front. Cell Dev. Biol. 8, 96. doi:10.3389/fcell.2020.00096
DG-OIV (2022). The Director General-OIV. COEI-1-ACTGLU determination of beta-glucanase (1-3,1-6) activity in enzyme preparations. Secr. General Assembly, Pau Roca. Available online at: https://www.oiv.int/public/medias/5164/e-coei-1-actglu.pdf
Dhalaria, R., Verma, R., Kumar, D., Puri, S., Tapwal, A., Kumar, V., et al. (2020). Bioactive compounds of edible fruits with their anti-aging properties: a comprehensive review to prolong human life. Antioxidants 9 (11), 1123. doi:10.3390/antiox9111123
Enemali, J. O., Oluwafemi, F. A., Hussaini, S. J., Daniel, I. B., Ameh, O., Adamu, A., et al. (2020). Microgravity: a tool for protein drug development. Int. J. Pharm. Sci. Rev. Res. 64 (2), 82–86. doi:10.47583/ijpsrr.2020.v64i02.014
Freschet,, G. T., Pages, L., Iversen, C. M., Comas, L. H., Rewald, B., Roumet, C., et al. (2021). A starting guide to root ecology: Strengthening ecological concepts and standardizing root classification, sampling, processing an dtrait measurements. New. Phytologist. 232, 3. P.973–1122. doi:10.1111/nph.17572
Golla, V. S., and Sharabu, R. (2017). Preliminary phytochemical, UV-visible. FTir and in vitro antioxidant studies of aqueous methanolic extract of Canthium parviflorum stem bark (Rubiaceae). Euro. J. Biome. Pharmaceu. Sci. 4 (12), 862–866.
Joshi, R. (2018). Role of enzymes in seed germination. Int. J. Creative Res. Thoughts (IJCRT) 6 (2), 1481–1485. Available online at: https://www.ijcrt.org/papers/IJCRT1812620.pdf
Kafilzadeh, F., and Dehdari, F. (2015). Amylase activity of aquatic actinomycetes isolated from the sediments of mangrove forests in south of Iran. Egypt. J. Aquatic Res. 41, 197–201. doi:10.1016/j.ejar.2015.04.003
Kiss, J. Z., Wolverton, C., Wyatt, S. E., Hasenstein, K. H., and van Loon, J. J. W. A. (2019). Comparison of microgravity analogs to spaceflight in studies of plant growth and development. Front. Plant Sci. 10, 1577. doi:10.3389/fpls.2019.01577
Konig, J., Grasser, R., Pikor, H., and Vogel, K. (2002). Determination of xylanase, β-glucanase, and cellulase activity. Anal. Bioanal. Chem. 374 (1), 80–87. doi:10.1007/s00216-002-1379-7
Lakshamanashetty, R. H., Nagaraj, V. B., Hiremath, M. G., and Kumar, V. (2010). In vitro antioxidant activity of Vitex negundo L. leaf extracts. Chiang Mai Journal of Science 1:37(3), 489–497.
Li Sng, N. J. (2017). Characterizing a novel reactive oxygen species gene identified from spaceflight experiments. University of Florida, Gainesville, Florida, United States University of Florida Dissertation. Available online at: https://Ufdc.Ufl.Edu/Ufe0051719/00001.
Mahatma, M. K., Thawait, L. K., Bishi, S. K., Khatediya, N., Rathnakumar, A. L., Lalwani, H. B., et al. (2016). Nutritional composition and antioxidant activity of Spanish and Virginia groundnuts (Arachis hypogaea L.): a comparative study. J. Food Sci. Technol. 53 (5), 2279–2286. doi:10.1007/s13197-016-2187-y
Mukhopadhyay, S., and Bagh, S. (2020). A microgravity responsive synthetic genetic device in Escherichia coli. Biosens. Bioelectron. 167, 112462. doi:10.1016/j.bios.2020.112462
Murtaza, G., and Asghar, R. (2012). Alpha-amylase activities during seed development and germination in pea (Pisum sativum L.) treated with salicylic acid. Pak. J. Bot. 44 (6), 1823–1829. Available online at: https://www.semanticscholar.org/paper/%CE%B1-AMYLASE-ACTIVITIES-DURING-SEED-DEVELOPMENT-AND-IN-Murtaza-Asghar/3140d344abad40789311434538588b76b3d33500
Nakajima, S., Nagata, M., and Ikehata, A. (2021). Mechanism for enhancing the growth of mung bean seedlings under simulated microgravity. NPJ Microgravity 7 (1), 26. doi:10.1038/s41526-021-00156-6
Nguyen, H. P., Tran, P. H., Kim, K.-S., and Yang, S.-G. (2021). The effects of real and simulated microgravity on cellular mitochondrial function. Npj Microgravity 7 (44), 44. doi:10.1038/s41526-021-00171-7
Oluwafemi, F. (2021). “Gravity variation on the growth of maize shoots,” in Proceedings of 1st electronic conference on universe (ECU) (virtual): general relativity and gravitation section (MDPI, Basel, Switzerland: Multidisciplinary Digital Publishing Institute MDPI). doi:10.3390/ECU2021-10184
Oluwafemi, F. A. (2018). Space science and technology in economic prosperity. Lap Lambert Academic Publishing.
Oluwafemi, F. A. (2017). Microgravity research and applications: Roles in economic prosperity and poverty reduction. Conference proceedings of United Nations/United Arab Emirates high level forum: Space as a driver for socio-economic sustainable development. Dubai, United Arab Emirates.
Oluwafemi, F. A., De La Torre, A., Afolayan, E. M., Olalekan-Ajayi, B. M., Dhital, B., Mora-Almanza, J. G., et al. (2018). Space food and nutrition in a long-term manned mission. Adv. Astronautics Sci. Technol. 1, 1–21. doi:10.1007/s42423-018-0016-2
Oluwafemi, F. A., Gedebo, A., Gobena, A., Tesema, , Ibraheem, O., Olubiyi, R., et al. (2023). “Climate-change mitigation: a case study of soil biochar influence on morpho-physiology of crop species and genotypes,” in Proceedings of the Second Biennial Africa Climate-Smart Agriculture (CSA) Stakeholders Conference, Accra, Ghana, 14-16 September, 2022. Forum for Agricultural Research in Africa (FARA) Research Report, 777–782. Available online at: https://library.faraafrica.org/2023/04/27/climate-change-mitigation-a-case-study-of-soil-biochar-influence-on-morpho-physiology-of-crop-species-and-genotypes/.
Oluwafemi, F. A., Ibraheem, O., and Fatoki, T. H. (2020). Clinostat microgravity impact on root morphology of selected nutritional and economic crops. Plant Cell Biotechnol. Mol. Biol. 21 (43&44), 92–104. Available online at: https://ikprress.org/index.php/PCBMB/article/view/5478
Oluwafemi, F. A., and Neduncheran, A. (2022). Analog and simulated microgravity platforms for life sciences research: their individual capacities, benefits and limitations. Adv. Space Res. 69, 2921–2929. doi:10.1016/j.asr.2022.01.007
Oluwafemi, F. A., Neduncheran, A., Andrews, S., and Wu, D. (2019). “Methods of seeds planting in space: soilless or not. IAC-19,A1,8,12,x48743,” in 70th International Astronautical Congress (IAC), Washington, D.C, USA, 21–25 October, 2019.
Oluwafemi, F. A., Olalekan-Ajayi, B. M., Rabiu, B. A., Ibraheem, O., and Lawal-Akinlami, H. A. (2022). Microgravity research in biology and biochemistry of plants: a Review. J. Voice Women Dev. Agric. (VOWDA) 1 (1), 76–86. Available online at: https://www.researchgate.net/publication/366989846_Measurement_of_Growth_Rate_of_Rice_Oryza_sativa_Seedlings_under_Gravity_and_Simulated_Microgravity_Conditions.
Oluwafemi, F. A., and Olubiyi, R. A. (2019). “Investigation of corn seeds growth under simulated microgravity,” in Arid zone journal of engineering, technology and environment (AZOJETE). Centre for satellite technology development special issue: space science and technology for sustainable development, 15, 110–115.
Oluwafemi, F. A., Oyetunde, F. N., Okhuelegbe, E. O., Isokpan, D. E., Hanson-Akpan, R. I., Ameh, O., et al. (2024). Space life sciences a critical aspect of space research: a case study of the antioxidant activity of gravity and simulated microgravity seedlings root of cowpea. NASRDA Int. J. Space Technol. Earth Sci. 1 (1), 30–38. Available online at: https://www.researchgate.net/publication/387175372_Space_Life_Sciences_a_Critical_Aspect_of_Space_Research_A_Case_Study_of_the_Antioxidant_Activity_of_Gravity_and_Simulated_Microgravity_Seedlings_Root_of_Cowpea
Oluwafemi, F. A., and Oyewole, O. O. (2022). Measurement of growth rate of rice (Oryza sativa) seedlings under gravity and simulated microgravity conditions. J. Voice Women Dev. Agric. (VOWDA) 1 (2), 52–59. Available online at: https://www.researchgate.net/publication/360055391_Microgravity_Research_in_Biology_and_Biochemistry_of_Plants_A_Review.
Omotayo, A. O., and Aremu, A. O. (2021). Marama bean [Tylosema esculentum (Burch.) A. Schreib.]: an indigenous plant with potential for food, nutrition, and economic sustainability. Food Funct. 12 (6), 2389–2403. doi:10.1039/D0FO01937B
Pletser, V., and Russomano, T. (2020). Research in microgravity in physical and life sciences: an introduction to means and methods, preparation of space experiments. IntechOpen. doi:10.5772/intechopen.93463
Taibi, K., Taibi, F., Abderrahim, L. A., Ennajah, A., Belkhodja, M., and Mulet, J. M. (2016). Effect of salt stress on growth, chlorophyllcontent, lipid peroxidation and antioxidant defence systems in Phaseolus vulgaris L. South Afr. J. Bot. 105, 306–312. doi:10.1016/j.sajb.2016.03.011
Keywords: microgravity, antioxidant, root germination enzymes, root elongation enzymes, heavy metal, salinity
Citation: Oluwafemi FA, Ibraheem O and Olubiyi AR (2025) Improved biochemical properties of roots of selected economic crops after simulated microgravity impact. Front. Space Technol. 6:1552919. doi: 10.3389/frspt.2025.1552919
Received: 29 December 2024; Accepted: 11 March 2025;
Published: 01 April 2025.
Edited by:
Timothy Grant Hammond, Duke University, United StatesReviewed by:
Holly Hyde Birdsall, Baylor College of Medicine, United StatesCopyright © 2025 Oluwafemi, Ibraheem and Olubiyi. This is an open-access article distributed under the terms of the Creative Commons Attribution License (CC BY). The use, distribution or reproduction in other forums is permitted, provided the original author(s) and the copyright owner(s) are credited and that the original publication in this journal is cited, in accordance with accepted academic practice. No use, distribution or reproduction is permitted which does not comply with these terms.
*Correspondence: Funmilola A. Oluwafemi, b2x1d2FmZW1pZnVubWlsb2xhQGdtYWlsLmNvbQ==
Disclaimer: All claims expressed in this article are solely those of the authors and do not necessarily represent those of their affiliated organizations, or those of the publisher, the editors and the reviewers. Any product that may be evaluated in this article or claim that may be made by its manufacturer is not guaranteed or endorsed by the publisher.
Research integrity at Frontiers
Learn more about the work of our research integrity team to safeguard the quality of each article we publish.