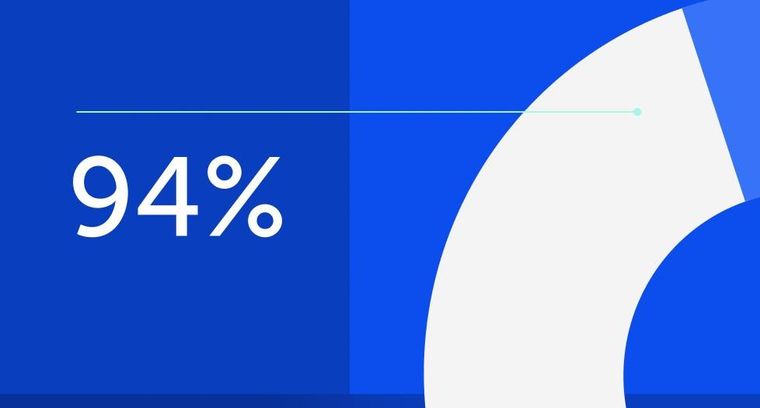
94% of researchers rate our articles as excellent or good
Learn more about the work of our research integrity team to safeguard the quality of each article we publish.
Find out more
MINI REVIEW article
Front. Space Technol., 16 December 2024
Sec. Space Exploration
Volume 5 - 2024 | https://doi.org/10.3389/frspt.2024.1471001
The idea of synthetic torpor, an artificial state resembling the hypo-metabolic state of torpor/hibernation, has recently gained significant scientific attention. Building on pioneering research demonstrating the protective effects of torpor against radiation in mammals, this mini-review aims to evaluate its scientific validity. We will explore the theoretical foundations, practical applications, and potential challenges linked to synthetic torpor. By critically examining the current knowledge, we aim to determine if synthetic torpor is a scientifically viable and achievable concept with broad implications for fields such as space exploration and healthcare.
In recent years, research into the mechanism of torpor and hibernation has gained momentum (Hitrec et al., 2019; Hrvatin et al., 2020; Takahashi et al., 2020). Torpor is a state used by some mammals to survive harsh conditions, and avoid predation, and is characterized by a significant decrease in metabolic rate and body temperature (Geiser, 2013; Ruf and Bieber, 2023). A sequence of torpor bouts separated by brief interbout arousals identifies hibernation. During torpor/hibernation, all physiological systems have to adapt to the new condition of low metabolism, and many of these adaptations offer interesting opportunities for medical (Cerri, 2017) and space technology (Choukér et al., 2021; Cerri et al., 2021).
In the last decade, a few procedures have been shown to be effective in mimicking torpor in non-hibernating mammals—a state referred to as synthetic torpor—garnering significant attention in the realms of space and medical research (Cerri et al., 2013; Takahashi et al., 2020; Tupone et al., 2013; Yang et al., 2023; Zakharova et al., 2019; Squire et al., 2020). As the scientific community investigates the fundamental mechanisms of torpor, questions about the potential applications of synthetic torpor, its scientific validity and its broader applicability become increasingly relevant.
Our goal is to scrutinize the scientific underpinnings, practical implications, and potential challenges associated with synthetic torpor. By evaluating its potential across diverse fields such as space exploration and healthcare, we aim to determine whether this concept holds genuine scientific promise or remains an aspirational idea with substantial obstacles yet to overcome. In doing so, we aim to contribute to the ongoing discussion on the validity and feasibility of synthetic torpor in the context of future scientific advancements.
Torpor has garnered global attention due to its recently suggested applications for space exploration and medical interventions (Petit et al., 2018). Despite its promise, the true power of torpor remains largely untapped, primarily because the underlying mechanisms of this behavior have yet to be fully understood. While scientists have made efforts to comprehend the natural hibernation processes of animals, the current surge in interest centers around the induction of synthetic torpor through pharmaceuticals (Schafer, 2023). Replicating the natural hibernation process using chemicals and drugs presents a promising avenue for pharmacological development.
Overall, two main uses of synthetic torpor can be envisioned: the first one is to replicate the entire behavior, exploiting the full set of physiological adaptations; the second one is to understand the mechanism of single system/organ adaptations to develop specific medical treatments or countermeasures for space exploration. Among these, a particularly interesting case is the resistance to radiation damage induced by torpor (Cerri et al., 2016; Puspitasari et al., 2021). Metabolic rate was first hypothesized to modulate radiation resistance when patients treated with the available thyroid hormone reported higher sensitivity to radiation (Smith and Smith, 1951). If higher metabolism was sensitized to radiation damage, hypometabolism might have been protective. Since torpor and hibernation are the natural models with the largest decrease in metabolic rate, squirrels and other hibernators were tested for their response to radiation exposure (Musacchia and Barr, 1968). The results indicated that torpor was indeed protective against the damage induced by radiation. When procedures for synthetic torpor were later developed, it was only natural to test if this pharmacologically induced state shared such features with natural torpor (Puspitasari et al., 2022).
Research on various hibernating animals, such as ground squirrels, bears, and certain bat species, has contributed to our understanding of the physiological and biochemical mechanisms of adaptation. These studies have provided a first frame to understand the physiology of torpor, as described in (Figure 1) (Andrews, 2007; Neff, 2018). This mechanism enables small mammals not only to endure periods of low resource availability or high energy demand but also to tolerate increased competition with other species for limited resources (Levy et al., 2011; Heldmaier and Steinlechner, 1981). Numerous physiological changes have been observed during torpor and hibernation, including very little muscle loss despite prolonged periods of immobility, alterations in immune cells reactivity (including those in the gut), changes in the physiology of the gastrointestinal tract (GI tract) (Sisa et al., 2017; Regan et al., 2022; Carey and Assadi-Porter, 2017; Greene et al., 2022), neuroinflammation (Arendt and Bullmann, 2013; Chiocchetti et al., 2021; Hitrec et al., 2021; Luppi et al., 2019; Squarcio et al., 2023), and a decrease in protein synthesis (Wei et al., 2019; Morin and Storey, 2009; Kurtz et al., 2021). Torpor duration can vary significantly: from less than 24 h in species displaying what is called “daily torpor” to several weeks in species undergoing seasonal hibernation, in which sequential torpor episodes are separated by 24 h interbout arousals.
Figure 1. Overview of the mechanisms in torpor. Cells within the hibernating brain undergo reversible morphological changes in microglia, exhibit reduced inflammatory responses, suppressed metabolic pathways, and inhibition of the cell cycle. Additionally, gene activity and other molecular pathways slow down at the molecular level, alongside the reduction in body temperature (Giroud et al., 2021; Logan and Storey, 2021). This figure has been generated using Biorender.com.
Recently, studies using mostly animal models for daily torpor, such as mice, have unraveled the neural network responsible for natural torpor, in the attempt to better understand the process and build the knowledge required to replicate it in non-hibernators (Ambler et al., 2022; Hitrec et al., 2019; Hrvatin et al., 2020; Takahashi et al., 2020; Yamaguchi et al., 2023). These studies show that VGLUT2-or Adcyap1-positive neurons within the avMLPA appear to be responsible for the induction of torpor (Hrvatin et al., 2020; Takahashi et al., 2020). The involvement of the hypothalamus is also supported by the findings that there is an upregulation of thioredoxin-interacting protein (TXNIP) in the hypothalamus, all influenced by factors such as food and water consumption (Hand et al., 2013).
Several procedures, such as the use of pharmacology and ultrasound, have been shown to be effective in inducing a state of synthetic torpor in rats, which are used as non-hibernating animal model (Takahashi et al., 2020; Cerri et al., 2013; Tupone et al., 2013; Yang et al., 2023; Zakharova et al., 2019; Zakharova et al., 2021). Previous work highlights the role of hydrogen sulfide (
The first effective procedure to induce synthetic torpor in non-hibernating animals were first shown by repeated injections of the GABA-A agonist muscimol into the Raphe pallidus (RPa) region. RPa is a key thermoregulatory region within the brainstem; RPa neurons stimulate thermogenesis when activated and thermal dissipation when inhibited (Cerri et al., 2010; Morrison and Nakamura, 2019). The results showed that prolonged RPa inhibition resulted in deep hypothermia, accompanied by a decreased heart rate and EEG activity, which are similar to natural torpor animals (Cerri et al., 2013).
Other research on rats has suggested that synthetic torpor can be induced through the injection of adenosine agonist such as 5′-Adenosine monophosphate (5′-AMP), which activates central adenosine (A1) receptors (A1ARs) (Tupone et al., 2013; Iliff and Swoap, 2012; Shimaoka et al., 2018; Jinka et al., 2011), a pharmacological intervention effective also on mice (Ghosh et al., 2017; Silvani et al., 2018). Activation of A1ARs in rats led to a torpor-like state and reduced body temperature, possibly associated with the inhibition of brown adipose tissue (Tupone et al., 2013). Moreover, 5′-AMP has shown to protect both mice and rats against total body irradiation (TBI) from X-rays and has also found on rats following TBI with heavy ions (Ghosh et al., 2017; Puspitasari et al., 2022; Tinganelli et al., 2019). While radiation resistance seems to be present in both natural and synthetic torpor, other physiological functions, such as mitochondrial function have been shown to differ (Sgarbi et al., 2022).
Another promising approach to induce a torpor-like state is through remote transcranial ultrasound stimulation targeted at the hypothalamic preoptic area (POA). This noninvasive, precise, and safe method achieves a long-lasting (
The induction of torpor in animals offers potential benefits, as demonstrated by Weissman et al., who provided proof-of-concept evidence for its benefits in long-duration space missions (Weissman et al., 2024). Torpor induces a hypothermic, hypoactive, and hypo-metabolic state that activates multiple biological countermeasures to the conditions encountered in space missions (Choukér et al., 2021; Pavez et al., 2021; Cerri, 2017). Nevertheless, translating this to humans presents significant challenges (Griko and Regan, 2018). Some studies and technologies already exist for implementing hypothermia in cardiac arrest patients, such as in the study referenced (Dankiewicz et al., 2021). However, the process of inducing hypothermia differs from torpor, which not only requires hypothermia but also coordination with other physiological processes and functions (Geiser et al., 2014). Moreover, these studies focus specifically on patients who have experienced cardiac arrest and not on healthy subject.
The idea of implementing torpor in space missions could yield cost-efficiency benefits, with astronauts consuming less oxygen, food, and water, and protecting against cosmic radiation (Shi et al., 2021). Additionally, it may help mitigate muscle tissue loss, a common issue in long-space flights, and provide protection from harmful cosmic radiation damage (Cerri, 2017; Puspitasari et al., 2021). Torpor’s ability to reduce the impact of environmental stressors, such as confinement, and it could help preserve neuroendocrine and immune systems during missions (Nordeen and Martin, 2019; Crucian et al., 2014). Apart for Beyond Low Earth Orbit (BLEO) space exploration, torpor is thought to be a promise in cancer treatment by slowing tumor progression through metabolic depression (Schafer, 2023). Studies on hamsters with sarcomas has shown that tumor growth can be halted during hibernation (Lyman and Fawcett, 1954).
The induction of synthetic hibernation for space travel in astronauts is a fascinating concept, yet it remains under discussion, as torpor induction studies in mammals are still in early stages. NASA’s Phase I study showed that subjects could be kept in a mild hypometabolic state for up to 14 days without fatal outcomes, suggesting the potential of induced hypothermia in humans (Nordeen and Martin, 2019). Hypothermia one of the primary physiological changes in torpor, is commonly employed for cardiac arrest patients. Studies have demonstrated that humans can achieve mild hypothermia (35°C–36.3°C) for approximately 90 min through intravenous dexmedetomidine administration (Callaway et al., 2015). However, this duration is inadequate for long-term space travel, and extended hypothermia beyond 24 h raises risks of immune and gastrointestinal issues (Shi et al., 2021). Ongoing research is exploring the role of the CNS in regulating torpor and the mechanisms for inducing this state in humans. Moreover, as mentioned earlier in this review, hibernation might help mitigate the adverse effects of radiation exposure in space (Shi et al., 2021). In the context of cancer treatment, radiotherapy, either used alone or in combination with chemotherapy, is limited by the maximum tolerable dose for each organ (Shi et al., 2021). Exploring how synthetic hibernation influences radioresistance in both normal and tumor tissues could be intriguing, as it may play a significant role in halting tumor growth.
While there’s been some success in inducing torpor artificially, scientists still have concerns about its safety, how well induction methods work, controlling how long it lasts, whether it is truly like natural torpor, whether it can be reversed, potential risks, if it applies to humans, and its long-term effects on health and behavior. These concerns revolve around the effectiveness of torpor induction methods, the ability to replicate all the features of natural torpor states, and the potential risks and long-term consequences. Therefore, scientists must continue refining the process to improve its quality and safety. Key challenges include:
It has been suggested that metabolic depression is similar to torpor. Achieving shallow metabolic depression (20% reduction of metabolic rate relative to basal levels) is a feasible first step towards synthetic torpor during human spaceflight. This could potentially benefit crew health, reduce spacecraft demands, and serve as a testbed for deeper metabolic depression technologies. The benefits of shallow metabolic depression would include increasing sleep time to 7–8 h per night, which might reduce life support system requirements and decrease caloric needs (Regan et al., 2020).
Moreover, the study in rats has shown that synthetic torpor triggers a neuroprotective effect on the brain, reversing brain Tau hyperphosphorylation. This might open new paths to study the treatment of Alzheimer’s and other associated diseases (Squarcio et al., 2023).
This review provides a concise analysis of synthetic torpor, emphasizing its significance in both clinical and space research, while also illuminating the challenges that may arise. In nature, hibernation is a seasonal adaptation associated with low body temperature and driven by environmental changes. Cerri et al. first introduced the term ‘synthetic torpor,’ defining it as a state of artificially induced reversible metabolic depression (Cerri, 2017). Synthetic torpor could be induced by clinical drugs such as 5′-AMP, leading to a decrease in body temperature and metabolic rate. Since torpor is associated with many physiological changes, it could also increase the survival probability of patients in life-threatening conditions by slowing metabolism and disease progression (Hypothermia after Cardiac Arrest Study Group, 2002).
Previously, we have proposed the idea of possible synthetic torpor for space travel (Cerri et al., 2016), (Puspitasari et al., 2021). In this review, we consolidate recent findings from animal models to establish a foundation for future studies and identify key areas for further research to bridge the gap toward human application. Despite the challenges associated with torpor, there is increasing evidence that its use could be advantageous from both clinical and scientific perspectives, especially for long-duration space missions. Interestingly, torpor provides space-protective effects to the body, including the preservation of muscle and bone mineralization, as well as radiation resistance. However, studies on synthetic torpor’s ability to reduce radiation effects have so far been limited to rodent models, and significant challenges remain before this approach can be applied to humans.
In the area of synthetic torpor, much remains to be explored, and torpor-inspired technologies are still in their infancy. Nevertheless, with adequate support from government agencies, private biotech firms, and biomedical companies, the development of highly beneficial torpor technology could be within reach for this generation.
AK: Writing–original draft, Writing - review and editing. RC: Writing–review and editing. KS: Writing–review and editing. MC: Writing–review and editing. WT: Writing–review and editing.
The author(s) declare that no financial support was received for the research, authorship, and/or publication of this article.
The authors declare that the research was conducted in the absence of any commercial or financial relationships that could be construed as a potential conflict of interest.
All claims expressed in this article are solely those of the authors and do not necessarily represent those of their affiliated organizations, or those of the publisher, the editors and the reviewers. Any product that may be evaluated in this article, or claim that may be made by its manufacturer, is not guaranteed or endorsed by the publisher.
Ambler, M., Hitrec, T., Wilson, A., Cerri, M., and Pickering, A. (2022). Neurons in the dorsomedial hypothalamus promote, prolong, and deepen torpor in the mouse. J. Neurosci. 42, 4267–4277. doi:10.1523/JNEUROSCI.2102-21.2022
Andrews, M. T. (2007). Advances in molecular biology of hibernation in mammals. BioEssays 29, 431–440. doi:10.1002/bies.20560
Arendt, T., and Bullmann, T. (2013). Neuronal plasticity in hibernation and the proposed role of the microtubule-associated protein tau as a “master switch” regulating synaptic gain in neuronal networks. Am. J. Physiology. Regul. Integr. Comp. Physiology 305, R478–R489. doi:10.1152/ajpregu.00117.2013
Callaway, C. W., Elmer, J., Guyette, F. X., Molyneaux, B. J., Anderson, K. B., Empey, P. E., et al. (2015). Dexmedetomidine reduces shivering during mild hypothermia in waking subjects. PLoS One 10, e0129709. doi:10.1371/journal.pone.0129709
Carey, H., and Assadi-Porter, F. (2017). The hibernator microbiome: host-bacterial interactions in an extreme nutritional symbiosis. Annu. Rev. Nutr. 37, 477–500. doi:10.1146/annurev-nutr-071816-064740
Cerri, M. (2017). The central control of energy expenditure: exploiting torpor for medical applications. Annu. Rev. Physiology 79, 167–186. doi:10.1146/annurev-physiol-022516-034133
Cerri, M., Hitrec, T., Luppi, M., and Amici, R. (2021). Be cool to be far: exploiting hibernation for space exploration. Neurosci. Biobehav. Rev. 128, 218–232. doi:10.1016/j.neubiorev.2021.03.037
Cerri, M., Mastrotto, M., Tupone, D., Martelli, D., Luppi, M., Perez, E., et al. (2013). The inhibition of neurons in the central nervous pathways for thermoregulatory cold defense induces a suspended animation state in the rat. J. Neurosci. 33, 2984–2993. doi:10.1523/JNEUROSCI.3596-12.2013
Cerri, M., Tinganelli, W., Negrini, M., Helm, A., Scifoni, E., Tommasino, F., et al. (2016). Hibernation for space travel: impact on radioprotection. Life Sci. Space Res. 11, 1–9. doi:10.1016/j.lssr.2016.09.001
Cerri, M., Zamboni, G., Tupone, D., Dentico, D., Luppi, M., Martelli, D., et al. (2010). Cutaneous vasodilation elicited by disinhibition of the caudal portion of the rostral ventromedial medulla of the free-behaving rat. Neuroscience 165, 984–995. doi:10.1016/j.neuroscience.2009.10.068
Chiocchetti, R., Hitrec, T., Giancola, F., Sadeghinezhad, J., Squarcio, F., Galiazzo, G., et al. (2021). Phosphorylated tau protein in the myenteric plexus of the ileum and colon of normothermic rats and during synthetic torpor. Cell. Tissue Res. 384, 287–299. doi:10.1007/s00441-020-03328-0
Choukér, A., Ngo-Anh, T. J., Biesbroek, R., Heldmaier, G., Heppener, M., and Bereiter-Hahn, J. (2021). European space agency’s hibernation (torpor) strategy for deep space missions: linking biology to engineering. Neurosci. Biobehav. Rev. 131, 618–626. doi:10.1016/j.neubiorev.2021.09.054
Crucian, B., Simpson, R. J., Mehta, S., Stowe, R., Chouker, A., Hwang, S. A., et al. (2014). Terrestrial stress analogs for spaceflight-associated immune system dysregulation. Brain, Behav. Immun. 39, 23–32. doi:10.1016/j.bbi.2014.01.011
Dankiewicz, J., Cronberg, T., Lilja, G., Jakobsen, J. C., Levin, H., Ullén, S., et al. (2021). Hypothermia versus normothermia after out-of-hospital cardiac arrest. N. Engl. J. Med. 384, 2283–2294. doi:10.1056/NEJMoa2100591
Drabek, T., Kochanek, P. M., Stezoski, J., Wu, X., Bayr, H., Morhard, R. C., et al. (2011). Intravenous hydrogen sulfide does not induce hypothermia or improve survival from hemorrhagic shock in pigs. Shock 35, 67–73. doi:10.1097/SHK.0b013e3181e86f49
Geiser, F., Currie, S. E., O’Shea, K. A., and Hiebert, S. M. (2014). Torpor and hypothermia: reversed hysteresis of metabolic rate and body temperature. Am. J. Physiology-Regulatory, Integr. Comp. Physiology 307, R1324–R1329. doi:10.1152/ajpregu.00214.2014
Ghosh, S., Indracanti, N., Joshi, J., Ray, J., and Indraganti, P. K. (2017). Pharmacologically induced reversible hypometabolic state mitigates radiation induced lethality in mice. Sci. Rep. 7, 14900. doi:10.1038/s41598-017-15002-7
Giroud, S., Habold, C., Nespolo, R. F., Mejías, C., Terrien, J., Logan, S. M., et al. (2021). The torpid state: recent advances in metabolic adaptations and protective mechanisms. Front. Physiology 11, 623665. doi:10.3389/fphys.2020.623665
Greene, L. K., Andriambeloson, J. B., Rasoanaivo, H. A., Yoder, A. D., and Blanco, M. B. (2022). Variation in gut microbiome structure across the annual hibernation cycle in a wild primate. FEMS Microbiol. Ecol. 98, fiac070. doi:10.1093/femsec/fiac070
Griko, Y., and Regan, M. D. (2018). Synthetic torpor: a method for safely and practically transporting experimental animals aboard spaceflight missions to deep space. Life Sci. Space Res. 16, 101–107. doi:10.1016/j.lssr.2018.01.002
Hand, L. E., Saer, B. R. C., Hui, S. T., Jinnah, H. A., Steinlechner, S., Loudon, A. S. I., et al. (2013). Induction of the metabolic regulator txnip in fasting-induced and natural torpor. Endocrinology 154, 2081–2091. doi:10.1210/en.2012-2051
Haouzi, P., Notet, V., Chenuel, B., Chalon, B., Sponne, I., Ogier, V., et al. (2008). H2s induced hypometabolism in mice is missing in sedated sheep. Respir. Physiol. Neurobiol. 160, 109–115. doi:10.1016/j.resp.2007.09.001
Heldmaier, G., and Steinlechner, S. (1981). Seasonal pattern and energetics of short daily torpor in the djungarian hamster, phodopus sungorus. Oecologia 48, 265–270. doi:10.1007/BF00347975
Hitrec, T., Luppi, M., Bastianini, S., Squarcio, F., Berteotti, C., Lo, M. V., et al. (2019). Neural control of fasting-induced torpor in mice. Sci. Rep. 9, 15462. doi:10.1038/s41598-019-51841-2
Hitrec, T., Squarcio, F., Cerri, M., Martelli, D., Occhinegro, A., Piscitiello, E., et al. (2021). Reversible tau phosphorylation induced by synthetic torpor in the spinal cord of the rat. Front. Neuroanat. 15, 592288. doi:10.3389/fnana.2021.592288
Hrvatin, S., Sun, S., Wilcox, O. F., Yao, H., Lavin-Peter, A. J., Cicconet, M., et al. (2020). Neurons that regulate mouse torpor. Nature 583, 115–121. doi:10.1038/s41586-020-2387-5
Hypothermia after Cardiac Arrest Study Group (2002). Mild therapeutic hypothermia to improve the neurologic outcome after cardiac arrest. N. Engl. J. Med. 346, 549–556. doi:10.1056/NEJMoa012689
Iliff, B. W., and Swoap, S. J. (2012). Central adenosine receptor signaling is necessary for daily torpor in mice. Am. J. Physiology-Regulatory, Integr. Comp. Physiology 303, R477–R484. doi:10.1152/ajpregu.00081.2012
Jensen, B. S., and Fago, A. (2021). Sulfide metabolism and the mechanism of torpor. J. Exp. Biol. 224, jeb215764. doi:10.1242/jeb.215764
Jinka, T. R., Toien, O., and Drew, K. L. (2011). Season primes the brain in an arctic hibernator to facilitate entrance into torpor mediated by adenosine a1 receptors. J. Neurosci. 31, 10752–10758. doi:10.1523/JNEUROSCI.1240-11.2011
Kurtz, C. C., Otis, J. P., Regan, M. D., and Carey, H. V. (2021). How the gut and liver hibernate. Comp. Biochem. Physiology Part A Mol. Integr. Physiology 253, 110875. doi:10.1016/j.cbpa.2020.110875
Levy, O., Dayan, T., and Kronfeld-Schor, N. (2011). Interspecific competition and torpor in golden spiny mice: two sides of the energy-acquisition coin. Integr. Comp. Biol. 51, 441–448. doi:10.1093/icb/icr071
Logan, S. M., and Storey, K. B. (2021). Microrna expression patterns in the brown fat of hibernating 13-lined ground squirrels. Genomics 113, 769–781. doi:10.1016/j.ygeno.2021.01.017
Luppi, M., Hitrec, T., Cristoforo, A. D., Squarcio, F., Stanzani, A., Occhinegro, A., et al. (2019). Phosphorylation and dephosphorylation of tau protein during synthetic torpor. Front. Neuroanat. 13, 57. doi:10.3389/fnana.2019.00057
Lyman, C., and Fawcett, D. (1954). The effect of hibernation on the growth of sarcoma in the hamster. Cancer Res. 25–28.
Morin, P., and Storey, K. B. (2009). Mammalian hibernation: differential gene expression and novel application of epigenetic controls. Int. J. Dev. Biol. 53, 433–442. doi:10.1387/ijdb.082643pm
Morrison, S. F., and Nakamura, K. (2019). Central mechanisms for thermoregulation. Annu. Rev. Physiology 81, 285–308. doi:10.1146/annurev-physiol-020518-114546
Musacchia, X. J., and Barr, R. E. (1968). Survival of whole-body-irradiated hibernating and active ground squirrels; citellus tridecemlineatus. Radiat. Res. 33, 348–356. doi:10.2307/3572485
Nordeen, C. A., and Martin, S. L. (2019). Engineering human stasis for long-duration spaceflight. Physiology 34, 101–111. doi:10.1152/physiol.00046.2018
Pavez, L. E., Baatout, S., Choukér, A., Buchheim, J. I., Baselet, B., Dello Russo, C., et al. (2021). The future of personalized medicine in space: from observations to countermeasures. Front. Bioeng. Biotechnol. 9, 1–20. doi:10.3389/fbioe.2021.739747
Petit, G., Koller, D., Summerer, L., Heldmaier, G., Vyazovskiy, V. V., Cerri, M., et al. (2018). Hibernation and torpor: prospects for human spaceflight. Handbook of life support systems for spacecraft and extraterrestrial habitats. Springer, 1–15. doi:10.1007/978-3-319-09575-2_100-1
Puspitasari, A., Cerri, M., Takahashi, A., Yoshida, Y., Hanamura, K., and Tinganelli, W. (2021). Hibernation as a tool for radiation protection in space exploration. Life Basel, Switz. 11, 54. doi:10.3390/life11010054
Puspitasari, A., Squarcio, F., Quartieri, M., Totis, C., Hitrec, T., Takahashi, A., et al. (2022). Synthetic torpor protects rats from exposure to accelerated heavy ions. Sci. Rep. 12, 16405. doi:10.1038/s41598-022-20382-6
Regan, M., Chiang, E., Liu, Y., Tonelli, M., Verdoorn, K., Gugel, S., et al. (2022). Nitrogen recycling via gut symbionts increases in ground squirrels over the hibernation season. Science 375, 460–463. doi:10.1126/science.abh2950
Regan, M. D., Flynn-Evans, E. E., Griko, Y. V., Kilduff, T. S., Rittenberger, J. C., Ruskin, K. J., et al. (2020). Shallow metabolic depression and human spaceflight: a feasible first step. J. Appl. Physiology 128, 637–647. doi:10.1152/japplphysiol.00725.2019
Ruf, T., and Bieber, C. (2023). Why hibernate? predator avoidance in the edible dormouse. Mammal Res. 68, 1–11. doi:10.1007/s13364-022-00652-4
Schafer, J. (2023). Timeout with torpor: history, biology, and future medical applications of a survival strategy in Hibernation writing project. UC Davis.
Sgarbi, G., Hitrec, T., Amici, R., Baracca, A., Cristoforo, A. D., Liuzzi, F., et al. (2022). Mitochondrial respiration in rats during hypothermia resulting from central drug administration. J. Comp. Physiology B 192, 349–360. doi:10.1007/s00360-021-01421-6
Shi, Z., Qin, M., Huang, L., Xu, T., Chen, Y., Hu, Q., et al. (2021). Human torpor: translating insights from nature into manned deep space expedition. Biol. Rev. 96, 642–672. doi:10.1111/brv.12671
Shimaoka, H., Kawaguchi, T., Morikawa, K., Sano, Y., Naitou, K., Nakamori, H., et al. (2018). Induction of hibernation-like hypothermia by central activation of the a1 adenosine receptor in a non-hibernator, the rat. J. Physiological Sci. 68, 425–430. doi:10.1007/s12576-017-0543-y
Silvani, A., Cerri, M., Zoccoli, G., and Swoap, S. J. (2018). Is adenosine action common ground for nrem sleep, torpor, and other hypometabolic states? Physiol. (Bethesda) 33, 182–196. doi:10.1152/physiol.00007.2018
Sisa, C., Turroni, S., Amici, R., Brigidi, P., Candela, M., and Cerri, M. (2017). Potential role of the gut microbiota in synthetic torpor and therapeutic hypothermia. World J. Gastroenterology 23, 406–413. doi:10.3748/wjg.v23.i3.406
Smith, W. W., and Smith, F. (1951). Effect of thyroid hormone on radiation lethality. Am. J. Physiology 165, 639–650. doi:10.1152/ajplegacy.1951.165.3.639
Squarcio, F., Hitrec, T., Piscitiello, E., Cerri, M., Giovannini, C., Martelli, D., et al. (2023). Synthetic torpor triggers a regulated mechanism in the rat brain, favoring the reversibility of tau protein hyperphosphorylation. Front. Physiology 14, 1129278. doi:10.3389/fphys.2023.1129278
Squire, T., Ryan, A., and Bernard, S. (2020). Radioprotective effects of induced astronaut torpor and advanced propulsion systems during deep space travel. Life Sci. Space Res. 26, 105–113. doi:10.1016/j.lssr.2020.05.005
Takahashi, T. M., Sunagawa, G. A., Soya, S., Abe, M., Sakurai, K., Ishikawa, K., et al. (2020). A discrete neuronal circuit induces a hibernation-like state in rodents. Nature 583, 109–114. doi:10.1038/s41586-020-2163-6
Tinganelli, W., Hitrec, T., Romani, F., Simoniello, P., Squarcio, F., Stanzani, A., et al. (2019). Hibernation and radioprotection: gene expression in the liver and testicle of rats irradiated under synthetic torpor. Int. J. Mol. Sci. 20, 352. doi:10.3390/ijms20020352
Tupone, D., Madden, C. J., and Morrison, S. F. (2013). Central activation of the a1 adenosine receptor (a1ar) induces a hypothermic, torpor-like state in the rat. J. Neurosci. 33, 14512–14525. doi:10.1523/JNEUROSCI.1980-13.2013
Wei, Y., Zhang, J., Yan, X., Peng, X., Xu, S., Chang, H., et al. (2019). Remarkable protective effects of nrf2-mediated antioxidant enzymes and tissue specificity in different skeletal muscles of durian ground squirrels over the torpor-arousal cycle. Front. physiology 10, 1449. doi:10.3389/fphys.2019.01449
Weissman, A. J., Flickinger, K. L., Wu, V., DeMaio, R., Jonsson, A., Prescott, P., et al. (2024). Quasi-torpor for long-duration space missions. Front. Space Technol. 5, 1457487. doi:10.3389/frspt.2024.1457487
Yamaguchi, H., Murphy, K. R., Fukatsu, N., Sato, K., Yamanaka, A., and d, L. L. (2023). Brain-wide mapping of neuronal architecture controlling torpor. arXiv. doi:10.1101/2023.03.03.531064
Yang, Y., Yuan, J., Field, R., Ye, D., Hu, Z., Xu, K., et al. (2023). Induction of a torpor-like hypothermic and hypometabolic state in rodents by ultrasound. Nat. Metab. 5, 789–803. doi:10.1038/s42255-023-00804-z
Zakharova, N. M., Tarahovsky, Y. S., Fadeeva, I. S., Komelina, N. P., Khrenov, M. O., Glushkova, O. V., et al. (2019). A pharmacological composition for induction of a reversible torpor-like state and hypothermia in rats. Life Sci. 219, 190–198. doi:10.1016/j.lfs.2019.01.023
Keywords: synthetic torpor, radiation, space radiation, hibernation, torpor, hypothermia
Citation: Kokko AP, Chowdhury R, Sherman K, Cerri M and Tinganelli W (2024) Understanding the scientific validity of synthetic torpor for radiation research and extended space missions. Front. Space Technol. 5:1471001. doi: 10.3389/frspt.2024.1471001
Received: 26 July 2024; Accepted: 29 November 2024;
Published: 16 December 2024.
Edited by:
Shannon Noella Tessier, Massachusetts General Hospital and Harvard Medical School, United StatesReviewed by:
Jack J. W. A. van Loon, VU Amsterdam, NetherlandsCopyright © 2024 Kokko, Chowdhury, Sherman, Cerri and Tinganelli. This is an open-access article distributed under the terms of the Creative Commons Attribution License (CC BY). The use, distribution or reproduction in other forums is permitted, provided the original author(s) and the copyright owner(s) are credited and that the original publication in this journal is cited, in accordance with accepted academic practice. No use, distribution or reproduction is permitted which does not comply with these terms.
*Correspondence: Walter Tinganelli, w.tinganelli@gsi.de
†These authors have contributed equally to this work
Disclaimer: All claims expressed in this article are solely those of the authors and do not necessarily represent those of their affiliated organizations, or those of the publisher, the editors and the reviewers. Any product that may be evaluated in this article or claim that may be made by its manufacturer is not guaranteed or endorsed by the publisher.
Research integrity at Frontiers
Learn more about the work of our research integrity team to safeguard the quality of each article we publish.