- Instituto de Fisiología, Benemérita Universidad Autónoma de Puebla, Puebla, Mexico
This work discusses the challenges of space exploration, focusing on microgravity-induced physiological changes, particularly those affecting the vestibular system, which significantly alters human performance in space, necessitating effective countermeasures. In microgravity, astronauts experience disorientation and space motion sickness due to changes in vestibular input, leading to symptoms like vertigo and headache. Postflight, astronauts show various neurological changes, similar to symptoms in individuals with vestibular disorders experiencing significant cognitive and perceptual difficulties. Studies have also shown that microgravity affects cortical and sensory responses, altering perception, motor function, and brain connectivity. Galvanic Vestibular Stimulation (GVS) is explored as a countermeasure, using modulated electrical currents to evoke neuronal activity in vestibular end-organs, potentially stabilizing posture and gaze in microgravity. The work proposes that GVS could serve as a non-invasive intervention to help adapt to space environments by enhancing vestibular function and possibly aiding cognitive functions and underscores the need for continued research into the vestibular system’s role in human health and performance during space missions. It highlights the potential of GVS as a promising countermeasure for the challenges posed by microgravity.
1 Introduction
Space exploration entails numerous technical challenges, including propulsion systems, human space health, and medicine, the cost of space technologies, ambient aspects related to production and launching, the active removal of debris in space, and in-orbit servicing, among others (Aglietti, 2020). As mission duration increases, the requirements for effective medical countermeasures become more complex. Countermeasures or combinations of them must be developed because mission success could be compromised if astronauts cannot carry out essential functions. Failures in sensorimotor performance during piloting, extravehicular activity, or guidance tasks would pose significant risks to missions. The weightlessness problems must be addressed before seriously considering long-duration human missions (Clément et al., 2015).
In this work, we focus on the use of devices for Galvanic Vestibular Stimulation (GVS) as a countermeasure for microgravity-induced physiological changes, particularly those associated with the vestibular system and the neurosensory conflict, both of which may lead to significant alterations in human performance in space (Kornilova et al., 2017).
1.1 Vestibule and space adaptation syndrome
The Vestibular System is constituted by a set of biomechanical sensors located in the part of the inner ear. In a healthy person, the vestibular system produces the appropriate reflexes and reactions to achieve and maintain a stable body position, stabilize the gaze, and spatial orientation (Young, 1974). This biomechanical system comprises three semicircular canals (lateral, posterior, and anterior), oriented almost orthogonally, and two otolithic organs (saccule and utricle). The semicircular canals (SCC) allow for the detection of angular movements of the head, while the otolithic organs provide information on linear displacements (such as normal gravitational). The vestibular system generates a set of vestibule-spinal and vestibule-colic reflexes that contribute to maintaining stable posture, vestibule-ocular reflexes related to the maintenance of visual stability, and vestibule-autonomic reflexes related to vaso-vagal stability (Fife, 2010; Cullen, 2012).
The proper function of the vestibular apparatus is crucial for sensory integration and spatial location (Cullen, 2019). However, alterations in vestibular input can occur, particularly in microgravity environments. Disorientation in space and space motion sickness are commonly experienced by astronauts during the transition between gravitational environments (Moore et al., 2011). Exposure to microgravity triggers space adaptation syndrome and, in some cases, spatial motion sickness. Symptoms of this syndrome include vertigo, general malaise, lethargy, and headache (Crampton, 1990).
Alterations in vestibular end-organ function contribute to the development of this disabling condition. These alterations may include otolith deafferentation, channel–otolith conflict, interlabyrinth asymmetry, intersensory mismatch, and changes in labyrinth fluid dynamics due to fluid redistribution in the cranial direction (Kornilova and Kozlovskaya, 2003).
During space travel, there are transitions between periods of predominant adaptation and sub-adaptation (decompensation) (Kornilova and Kozlovskaya, 2003; Kornilova et al., 2017). Space adaptation process involves significant changes in the relative contributions of sensory inputs to cognitive and motor planning for the entire body and intersensory interactions in the new sensory environment. Consequently, this leads to significant rewiring of central integrative systems to ensure a new functional level appropriate for the altered environment.
Functional analyses of somatosensory responses have revealed that microgravity significantly influences cortical somatosensory and motor planning activity. Specifically, cortical responses to proprioceptive stimulation, such as leg muscle vibration, were more significant when subjects were planning a step. However, this preparatory activity is suppressed in microgravity conditions (Saradjian et al., 2014), indicating that the altered gravitational environment of space can impact how the brain processes somatosensory information and plans motor actions. Understanding these effects is crucial for developing effective countermeasures to mitigate the challenges astronauts face during space missions.
Significant postflight alterations have been observed in various aspects of perception and motor function, including changes in the perception of position, stabilization of gaze, gait, and balance (Buckey, 2006). Postflight analysis of brain connectivity using functional magnetic resonance imaging (fMRI) has revealed decreases in connectivity in specific brain regions, including the right insula, the ventral posterior cingulate cortex, and the intercortical connectivity of motor areas (Demertzi et al., 2016). Additionally, a decrease in gray matter around the frontal and temporal lobes has been reported in individuals who underwent 70 days of head-down training and astronauts who spent from 2 weeks to 6 months in orbit (Koppelmans et al., 2016). fMRI scanning in a group of 11 cosmonauts before and after completing a long-term mission to the International Space Station between 2014 and 2017 showed a postflight increase in the stimulation-specific connectivity of the right posterior supramarginal gyrus with the rest of the brain, a strengthening of connections between the left and right insulae, decreased connectivity of the vestibular nuclei, right inferior parietal cortex (BA40) and cerebellum with areas associated with motor, visual, vestibular, and proprioception functions, and decreased coupling of the cerebellum with the visual cortex and the right inferior parietal cortex (Pechenkova et al., 2019).
These findings underscore the complex and multifaceted impact of space travel on the human brain and motor system, highlighting the importance of further research in understanding and mitigating the effects of long-duration space missions on astronaut health and performance.
On Earth, individuals with vestibular disorders often experience symptoms that bear resemblance to those found in microgravity environments. Individuals with vestibular disorders commonly exhibit dysfunction related to spatial cognition, particularly at the cognitive level, including alterations in memory, navigation, mental rotation, and space representation (Bigelow and Agrawal, 2015; Smith et al., 2024). These cognitive impairments can significantly impact an individual’s ability to perform daily activities and may lead to difficulties in spatial orientation and movement. Therefore, understanding and managing vestibular disorders is essential to improve the quality of life for affected individuals. Distorted vestibular signals can lead to an erroneous perception of self-motion and body position in space, in a form analogous to errors in patients with vestibular disorders when an internal egocentric-based image is required. Vestibular cognitive dysfunction may also contribute to cognitive decline in other domains, such as arithmetic, object memory, and self-identification (Smith et al., 2024). These findings emphasize the importance of understanding vestibular dysfunction in microgravity.
2 GVS
GVS is a technique that uses modulated electrical currents to evoke neuronal activity in vestibular end-organs without head motion, and it has been explored for various medical applications and space-related activities (Alexandrov et al., 2004). Since the vestibular end organs reside in the temporal bone underneath the mastoid process. To perform the GVS, at least one electrode, the anode or the cathode, must be placed over the mastoid process (behind the ears). Applying electrical currents leads to activation or inhibition of the afferent nerve activity from the vestibular organs (For deep Reviews, see Utz et al., 2010; Lopez and Cullen, 2024) (Figure 1).
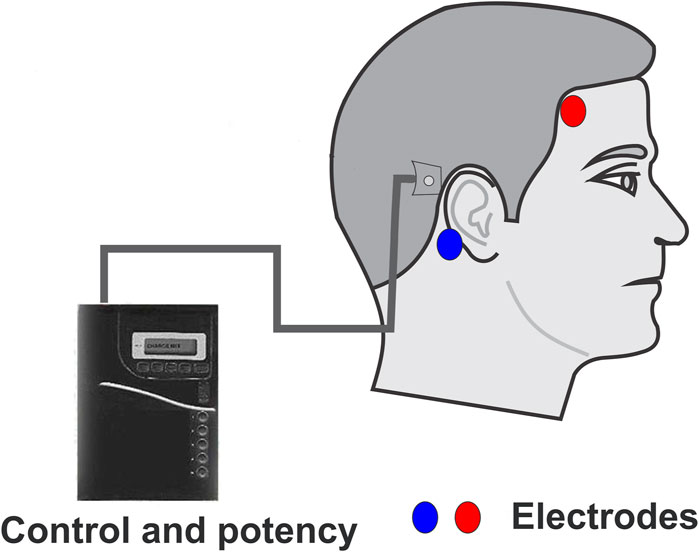
Figure 1. Simplified schematic representation of a GVS device. Typical GVS devices comprise a control and potency unit, a signal conditioning unit, and electrodes. The control and potency unit can be carried by the subject or manipulated externally. The output of the device can modulate the stimulating waveform, its frequency, and amplitude, to inject the current into the electrodes. One electrode should always be located on the mastoid process, while the other electrode can be placed in various locations: on the frontoparietal region (either ipsilateral, as shown, or contralateral), on the other mastoid process (transmastoid), or at the nape region.
GVS activates both the otolithic and semicircular canal afferent neurons, producing oculomotor and postural responses resulting from semicircular canal and otolithic signal convergence (Dlugaiczyk et al., 2019). Stimulation of the vestibular afferent nerve with electrical current in the squirrel monkey showed that irregular vestibular afferent neurons were the most sensitive to galvanic current stimulation, leading to the idea that GVS primarily activates irregular afferent neurons (Goldberg et al., 1984). Preliminary evidence showed that GVS modulates transmitter release by hair cells in the frog semicircular canals (Norris et al., 1998). Later work, in the isolated semicircular canals of the frog, using a semi-intact preparation of Xenopus laevis tadpoles, block of the hair cell to afferent neuron synapse significantly reduced the response to current stimuli, indicating that hair cell activation participates in the GVS effects. Notably, low-intensity stimulation mainly recruits hair cells, whereas larger currents directly activate the afferent neurons (Gensberger et al., 2016).
GVS modulates equilibrium and gaze stability in humans, becoming a tool for prosthetic devices to modulate balance. GVS is a form of transcranial current stimulation known to be safe and targets specifically the vestibular system non-invasively (Utz et al., 2010; Antal et al., 2017). In anesthetized male Long–Evans rats, repeated activation of the vestibulosympathetic reflex with ± 2 and ± 3 mA, 0.025 Hz sinusoidal GVS caused incremental changes in blood pressure and heart rate that blocked further generation of vasovagal responses. Thus, repeated low-frequency activation of the vestibulosympathetic reflex resulted in a reduction and loss of susceptibility to vasovagal responses in rats (Cohen et al., 2017). In a double-blind, sham-controlled study in humans resting in a supine position and then tilted up by 70°, it was found that noisy GVS produced non-significant changes in mean arterial blood pressure and heart rate variability (Matsugi et al., 2021). Studies of cardio-circulatory variables in normal subjects of a broad age range, in both standing and seated positions, and with unilateral or transmastoid direct current GVS, produced a marginal transient decrease in heart rate (Pliego et al., 2021). These studies showed that GVS is safe for use in humans. (Pliego et al., 2021). GVS elicits a sensation of displacement and reflex responses, either vestibulo-ocular or vestibulo-spinal. This phenomenon occurs because transmastoid GVS increases the discharge frequency of ipsilateral afferent neurons to the cathode. As a result, there is a compensatory inclination towards the anode side to maintain balance (Fitzpatrick and Day, 2004). Research has demonstrated that GVS can effectively stabilize subjects when their balance and posture are compromised (Scinicariello et al., 2001).
Moreover, changing the head position can modify the balance and orientation responses to GVS. Proper head alignments during locomotion allow the galvanic stimulus to direct the path or disturb balance as needed (St George et al., 2011). Overall, these findings underscore the potential utility of GVS in addressing balance-related challenges and improving postural stability.
The use of GVS has raised attention due to the possibility of developing a device to correct posture and gaze in vestibular dysfunction subjects or extreme conditions such as microgravity or astronaut training (Alexandrov et al., 2004; Moore et al., 2011; Alexandrov et al., 2014; Vega et al., 2016; Sadovnichii et al., 2019; Vega et al., 2023). Previous modeling work shows that GVS can modulate the vestibular afferent neuron dynamics. The vestibular afferent neurons behave as a bistable system, exhibiting a direct transition between a stable focus (no spiking activity) and a cycle (repetitive spiking activity). An amplitude characterized by the positive Hausdorff distance was obtained for a fixed galvanic current. Consequently, a transition occurs from the region of the point attractor to the area of attainability of a cycle (Alexandrov et al., 2021). Our model results also demonstrated that vestibular afferent neurons may have a stochastic resonance, which is relevant in its responses to different stimulus types and the role of random noise in the stimulating signals (Alexandrov et al., 2014).
2.1 GVS and cortical activation
The output of vestibular hair cells drives the dynamics of vestibular afferent neurons, which synapse with second-order vestibular neurons in the vestibular nuclear complex. These second-order neurons then project to the anterior dorsal thalamus and ventral posterior lateral nucleus through two ascending vestibular thalamocortical pathways (Dale and Cullen, 2019). From there, the pathways continue toward the primary sensory cortex (Fredrickson et al., 1966; Ödkvist et al., 1974) and other cortical and subcortical areas (De Waele et al., 2001; Hitier et al., 2014). These projections collectively shape the perception of balance and head position relative to space (Cullen, 2019).
The vestibular cortex encompasses various multisensory zones distributed across several brain regions, including the somatosensory cortex, temporoparietal junction, posterior insula, posterior parietal cortex, frontal medial cortex, and anterior insula (Lopez and Blanke, 2011; Lopez et al., 2012; Hitier et al., 2014). These regions have been characterized as “a set of neural networks located in the cerebral cortex whose neurons activate by some stimulus of vestibular origin” (Aedo Sánchez et al., 2016). Functional imaging studies have unveiled a network of vestibular cortical connectivity, demonstrating extensive interconnectivity between various vestibular cortical areas. This network mainly centers around the parietoinsular vestibular cortex (PIVC) (Smith et al., 2024).
fMRI studies have revealed that during GVS, the oxygenation level-dependent blood flow signal significantly increases in specific brain regions. Specifically, heightened activity is observed in the parietal operculum, central operculum, and opercular part of the inferior frontal gyrus (Mitsutake et al., 2020). Furthermore, using a tissue perfusion tracer during the magnetic resonance imaging (MRI), transcranial anodal direct current stimulation (tDCS) over the C4 (using the 10–20 EEG system) and the reference electrode over the left supra-orbital region leads to a notable increase in regional cerebral blood flow. In contrast, cathodic stimulation induces a comparatively lower increase (Zheng et al., 2011). These findings shed light on the differential effects of GVS on cerebral blood flow across different stimulation conditions, providing valuable insights into the neural mechanisms underlying vestibular stimulation.
Initial studies have yielded promising findings concerning the feasibility of employing functional near-infrared spectroscopy (fNIRS) to evaluate vestibular cortical responses. One study observed bilateral activation in the Superior Temporal Gyrus and Supramarginal Gyrus during dynamic posturography (Karim H. et al., 2013). Additionally, warm caloric irrigation elicited bilateral activation, while cold caloric irrigation resulted in contralateral activation of the temporoparietal zone. Moreover, older subjects exhibited more significant bilateral effects than younger individuals (Karim H. T. et al., 2013). In a study utilizing noisy GVS and recording neuronal metabolic responses by fNIRS, researchers reported a significant response in the amount of hemoglobin (Hb) in the supramarginal area. However, no significant increase was observed in oxygenated hemoglobin (HbO2) levels (Valdés et al., 2021), indicating that noisy GVS may selectively affect certain aspects of cerebral hemodynamics without necessarily altering oxygenation levels in the targeted brain region.
Our research demonstrates that both galvanic vestibular stimulation (GVS) and passive movement induce activity in the temporal and parietal regions. Our findings confirm that GVS activates the bilateral parietal cortex and the ipsilateral temporal cortex relative to the cathode position, mirroring the activation pattern observed during back-and-forth movements (Hernández-Román et al., 2023). These results underscore the potential of functional near-infrared spectroscopy (fNIRS) as a non-invasive technique for examining in spaceflight the cortical responses to movement and to GVS.
2.2 GVS and sleep
A consistent finding in space research is that total sleep duration, rapid eye movement (REM) sleep latency, and REM sleep duration are shorter than those on Earth. The assumption that the absence of gravity plays a role in sleep explains a significant portion of these results. Improving sleep quality is essential for achieving the expected performance of astronauts.
The organization of sleep and wakefulness regulation and control implies the participation of several neural circuits. It has been traditionally known that the rocking motion of babies is a successful strategy to induce sleep in children. Rocking motion by hammocks and rocking chairs facilitates relaxation and sleep. Sleeping in a rocking bed (at 0.25 Hz) reduced sleep onset and increased the stage N2 of sleep and the mean spindle density. Rocking also increased the EEG power of slow wave activity. Authors concluded that “rocking induces a speeded transition to an unambiguous sleep state and may enhance sleep by boosting slow oscillations and spindle activity” (Bayer et al., 2011). More recently, it was found that compared to a stationary night, continuous rocking (at 0.25 Hz) shortened the latency to non-REM (NREM) sleep and strengthened sleep maintenance by increasing the duration of NREM stage 3 (N3) and fewer arousals. Memory consolidation was also enhanced during rocking night, and fast spindles were enhanced (Perrault et al., 2019). However, it should be recognized that in some studies, no significant effect of rocking on sleep quality in normal subjects was found (Omlin et al., 2018). In mice, rhythmic rocking promotes sleep and accelerates sleep onset. Specifically, rocking mice at 1.0 Hz increased time spent in non-REM sleep by shortening wake episodes. In mice of the otoconia-deficient tilted mouse line, which cannot encode linear accelerations, were insensitive to rocking, demonstrating that functional otolithic organs were essential for mediating this effect of mechanical accelerations (Kompotis et al., 2019). These studies demonstrate that, among various physiological processes, vestibular input may influence the sleep-wake state, probably, by informing the brain about the daily quantity of motion (Besnard et al., 2018).
GVS has been investigated for its potential effects on sleep, although research in this area is still relatively limited compared to other applications of vestibular stimulation. There are reports that in humans, GVS promotes sleepiness, which is significant in at least 12% of normal subjects (Zago Mazzocco, 2018; Barranco, 2020). Electrical stimulation of the vestibular nerves seems to shorten sleep onset latency (Krystal et al., 2010). Regular GVS for over 4 weeks (30 min daily, 1 h before rest) resulted in a significant decrease in Insomnia Severity Index (ISI) scores and improved general wellbeing in young adults with insomnia, indicating that GVS may have potential as a non-invasive therapy to improve sleep (Goothy and McKeown, 2021).
The neural interactions between the vestibular system and sleep-wake system have recently been shown to imply a set of GABAergic neurons of the medial vestibular nucleus whose activity has been shown to stabilize wakefulness and to gate the transition from non-REM sleep to REM sleep (Nakatsuka et al., 2024). The neurons have projections to hypothalamic and brainstem sleep-wake regulatory areas such as lateral and posterior hypothalamic areas (LH and PH), mammillary nuclei, interpeduncular nuclei (IPN), ventrolateral PAG (vlPAG), median raphe nucleus (MnR), dorsomedial tegmental area (DMTg), parabrachial area (PB), LDTg, and pons central gray matter (PCG). Notably, it also projections to the medial septal nucleus (MS), which generates the theta rhythm in the EEG. The suppression of LMVN-GABAergic neuron activity increased the NRM sleep at the expense of wakefulness either during darkness or during the light phase of the mice cycle. Interestingly, REM sleep during rest time (light exposure for mice) significantly decreased by suppressing LMVN GABAergic neurons (Nakatsuka et al., 2024).
In clinical studies, poor sleep quality has been linked to vestibular hypofunction. In fact, patients experiencing chronic vertigo for 2 years or more reported significantly poorer global sleep quality, as assessed by the Pittsburgh Sleep Quality Index. Moreover, the association between longer duration of vertigo and poor sleep quality persisted even after multivariate adjustment (Katzenberger et al., 2023). Also, a negative correlation has been reported between sleep quality scores and sensory organization tests that examine vestibular balance function. However, low sleep quality affected both the visual and vestibular systems and the somatosensory system in a lower proportion (Sendesen et al., 2024). The effect of transcranial Alternating Current Stimulation (tACS) targeting the forehead and mastoid areas over a 4-week period was studied in chronic insomnia patients. Patients underwent 20 daily sessions of tACS (duration: 40 min, frequency: 77.5 Hz, and intensity: 15 mA) or sham tACS. The stimulated group significantly improved sleep quality and increased total sleep time. In older subjects, there was additionally a significant insomnia reduction with attenuated depressive and anxiety symptoms post-tACS treatment (Zhu et al., 2024).
Improving sleep quality is essential for achieving astronauts’ expected performance. Studies about the relationship between vestibular activation and sleep lead us to propose that GVS could also be a valuable countermeasure to sleep disorders in long-duration space flights.
2.3 GVS in space exploration
In space research, GVS has been used to create a cognitive simulation of movement for astronauts in flight simulators and potentially during a flight in microgravity (Vega et al., 2020).
GVS has been used as an analog of the sensorimotor effects of microgravity, affecting pilot performance during simulated Shuttle landings; a review of the first 100 Shuttle missions found that touchdown speed was above the specified limits in 20% of landings (Moore et al., 2011). Thence pseudorandom bilateral bipolar GVS was used as an analog of the sensorimotor effects of microgravity during Shuttle landing simulations. It was found that touchdown speed increased significantly during GVS exposure, and unsuccessful landings increased from 2.3% to 9%. It is concluded that GVS was an analog of decrements in postflight Shuttle pilot performance (Moore et al., 2011). GVS was also used to model locomotor and gaze dysfunction observed in astronauts following spaceflight, suggesting that an ambulatory system could be a valuable adjunct to pre-flight astronaut training regimens. GVS has also been used in flight simulators to mimic vestibular spatial disorientation illusions, such as the “post-roll illusion,” which is relevant for pilot training (Houben et al., 2024).
In contrast, integrating GVS with virtual reality (VR) flight training simulators have shown a potential to reduce visual-vestibular sensory conflict, improve flight performance, and reduce simulator sickness. The study demonstrates that coherent and precise synchronization of GVS with the visual stimuli in VR flight training reduces visual-vestibular sensory conflict and improves fidelity and performance (Pradhan et al., 2022). GVS mitigates cybersickness in virtual reality applications by reconciling the sensory mismatch between visually induced impressions of ego-motion and physical rest (Groth et al., 2022), thus enhancing the immersive experience in virtual training environments that could simulate spaceflight conditions. GVS has the potential to counteract spatial disorientation illusions like leans, which can confuse pilots about the position of the aircraft during flight. This illusion could lead to serious adverse effects and even flight accidents. A study of 20 Air Force pilots showed that step-GVS and ramped-GVS yielded lower bank angle errors and subjective spatial disorientation than without GVS (Kim et al., 2023).
Our research group developed a prosthetic device leveraging GVS to countermeasure the effects of a microgravity environment on posture and gaze stabilization. The design of this device relies on inertial sensors, including gyroscopes and accelerometers, which convert acceleration—either linear or angular—into voltage variations (Vega et al., 2020; Vega et al., 2023). The output from these sensors, specifically the acceleration-voltage relationship, can be translated into current pulses or steady state current, whose frequency or amplitude is mathematically related to the input. The output frequency oscillates within a range aligned with the natural burst frequency observed in afferent neurons of the vestibular system. Additionally, an offset voltage variation can determine the proportion of the accelerometer signal that the frequency-modulated output signal will accurately represent. This approach facilitates the translation of inertial sensor data into GVS signals, enabling posture and gaze stabilization in microgravity environments.
This type of stimulation aims to generate augmented reality for pilots during training or in-flight operations, which could be particularly useful in environments where vestibular cues are altered, such as in microgravity (Vega et al., 2020). The GVS stimulation should be coherent with the subject motion. Therefore, sensors should have an exquisite sensitivity to operate in microgravity. In this regard, experiments in mission Tatyana-2 tested the quality of micro gyroscopes functioning in space, and readings obtained from them compared with those obtained from precision angular velocity sensors, which are a part of the standard satellite positioning system (Aleksandrov et al., 2011; Sadovnichii et al., 2011). Also, in the Satellite MVS-330, mission IMISS showed that micro accelerometers allow for the detection of small linear accelerations consistently, and functional characteristics of the microdevices during the operation in space were comparable to their values on Earth (Sadovnichii et al., 2018). Results from these experiments confirm the possibility of using micro accelerometers and micro gyroscopes to sense the head motion of astronauts.
The device has demonstrated its capability to modulate vestibular responses, leading to its potential application in auxiliary devices for vestibular pathological hypofunction (Soto et al., 2014; Vega et al., 2016) or extreme conditions such as flight and microgravity (Alexandrov et al., 2014; Sadovnichii et al., 2019; Vega et al., 2023). Notably, the device has improved gaze fixation in volunteer subjects when applied alongside dynamic flight simulations. Eye tracking analysis revealed that GVS effectively modulates the vestibulo-ocular reflex, thereby reducing the displacement of the eyes from a target during platform movement (Vega et al., 2020). Gaze deviation with respect to the horizon (0°) was right-GVS < control and left-GVS < control, showing that the GVS application stabilizes the gaze on the target.
To address the challenges of microgravity adaptation during space missions, we envisioned two GVS protocols: In the first protocol, GVS is preprogrammed to be applied for 20–30 min daily before crew members go to rest. The amplitude of the stimulating current is maintained at a constant level of 1 mA, initiated by a 60-s ramp-up and terminated by a 60-s ramp-down. This protocol aims to improve sleep quality and to provide a vestibular cortical input. In the second protocol, the current injection characteristics are based on head movements in response to the output of the sensor (triaxial micro accelerometers and micro gyroscopes), modulating the output current above or below 1 mA. This protocol is intended to be used in extravehicular operations and in circumstances in which the highest level of performance is required. The preprogrammed protocol provides consistent and scheduled GVS sessions, while the sensor-based protocol offers adaptive stimulation tailored to crew members’ movements and environmental conditions. Implementing these protocols could contribute to enhancing crew members’ adaptability, performance, and wellbeing during space missions (Figure 2).
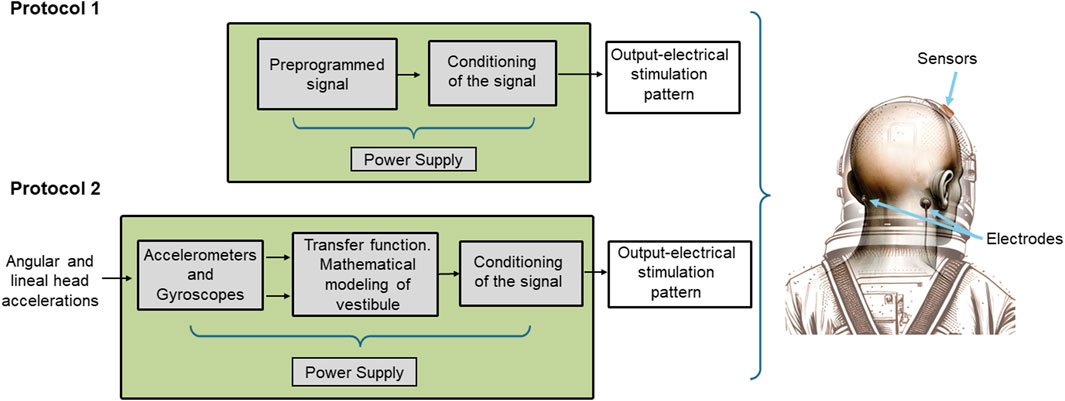
Figure 2. Scheme of the functional elements of the device. In protocol 1 mode of operation, the waveform and intensity of the GVS are preprogrammed and injected into the electrodes without any further processing. In protocol 2 mode of operation, the sensors (3D gyroscope and 3D accelerometer) are active. Their output is processed according to a transfer function based on a mathematical model of the human vestibular system, and the signal output is modulated accordingly. On the right is a schematic depiction of the electrodes and sensors located in the helmet and on the mastoid processes of an astronaut.
3 Discussion
GVS is a neuromodulation technique that has drawn attention for its potential applications in aerospace medicine, particularly in managing spatial orientation and motion perception during space flight. In the microgravity environment of space, astronauts experience vestibular disorientation due to the lack of gravitational cues normally present on Earth. This disorientation can lead to space motion sickness, impaired locomotion, and difficulties in spatial orientation and manual control tasks. GVS is being explored as a tool to artificially provide these missing gravitational cues by stimulating the vestibular system, potentially helping astronauts to adapt to the unique conditions of space flight. GVS may be an effective countermeasure for sensorimotor and neurovestibular disturbances, encompassing an effect that may contribute to countering the cortical changes observed in astronauts and also to improve the sleep quality of the subjects in space.
The finding that GVS activates cortical regions like movement, as demonstrated by Hernández-Román et al. (2023), is intriguing. Building upon these results, we propose that GVS, modulated by motion or preprogrammed, could serve as a potential countermeasure to stabilize the subject position and enhance gaze stability in microgravity. Additionally, GVS may mitigate cognitive dysfunction resulting from vestibular input alterations and potentially contribute to preventing cortical alterations induced by microgravity. It may also contribute significantly to improving the sleep quality of astronauts during space flight. By leveraging the capability of GVS to activate cortical regions, it could be integrated into space missions as a non-invasive intervention to help adapt to the challenges of the space environment. Further research is warranted to explore the feasibility and efficacy of GVS as a countermeasure in spaceflight settings.
Artificial gravity is another option to counter the weightlessness-induced effects on the human body (Clément et el., 2015). However, methods to induce artificial gravity are based on the rotation of a segment or whole of a spacecraft, with complex effects on sensed accelerations outside the exact plane of rotation. Long-duration head-down tilt bedrest (HDT) has been used as a spaceflight analog to model physiological changes that occur in microgravity (Tays et al., 2024). This procedure removes the somatosensory inputs to the foot sole, and vestibular cues are up weighted or relied upon more heavily (Mulavara et al., 2018; Hupfeld et al., 2022). The use of artificial gravity (1 G) applied along the long axis of the body via centrifugation during an experiment of 60 days of HDT shows that artificial gravity may serve as an effective countermeasure for balance and mobility deficit caused by HDT. Furthermore, during centrifugation, subjects could outperform cognitive tasks compared to those resting in bed (Tays et al., 2024). However, use of artificial gravity devices in space implies a significant investment of resources as compared to the use of GVS.
Previous work analyzing the effect of GVS addition on pilots’ virtual reality training has shown that GVS, coherent with visually simulated movement, significantly reduced motion sickness frequently produced by using the virtual reality system. It was shown that placing GVS electrodes in various locations induces a preferential sensation of roll, pitch, or yaw (Cevette et al., 2012).
It is worth noting that most of the research performed about GVS potentiality for use its use in spaceflight research has been done on Earth and in normal subjects. The balance and cognitive functions of astronauts, during and after the spaceflight, are similar to those of subjects with bilateral vestibulopathy (Clément et al., 2023). Furthermore, there is evidence that GVS can induce beneficial neural plasticity in the central pathways of patients with vestibular loss (Lopez and Cullen, 2024). Consequently, most probably, studies intended to demonstrate the effect of GVS on physiological variables in normal subjects and in normal gravity are not transposable to effects expected in astronauts; it may be something like using auditory devices in normal hearing subjects.
It cannot be discarded that GVS is producing its effect through cognitive responses. It should also be taken into consideration that effects due to transcranial stimulation will also affect cortical activity inducing cognitive responses, and by a placebo-like effect of GVS setup (Dilda et al., 2012), these actions cannot be discarded and would be considered a confounding factor.
A negative aspect of GVS devices intended for use in space is their relative lack of specificity, which could be partially solved using complex stimulation patterns and a set of electrodes to focus transcranial current stimulation (Pixa et al., 2017). Further experiments will contribute to clarifying the effects of other stimulation waveforms, such as noisy and sinusoidal stimulating currents, and their influence on both reflex and voluntary eye movements.
There are some potential limitations of GVS for space applications: it has been shown to adversely affect the performance of astronaut pilots during simulated Shuttle landings, leading to increased unsuccessful and hard landings (Moore et al., 2011). However, these studies were carried out in a flight simulator and normal subjects. The effectiveness of GVS can vary between individuals, which may limit its utility as a one-size-fits-all solution for training or counteracting spatial disorientation (Utz et al., 2010); this will imply tailoring GVS for each subject. While GVS is generally considered safe, there is always a concern for potential adverse effects, especially with long-term or intense use (Utz et al., 2010). GVS can induce sensorimotor impairment, which may impact the subject ability to perform critical tasks during transitions between gravity environments (Allred et al., 2024). Implementing GVS in a space environment may present technical challenges, such as ensuring the reliability and safety of the equipment under conditions of microgravity and radiation exposure. Finally, we must acknowledge that there is limited research on the long-term effects of GVS on subjects in spaceflight, which is necessary to understand its implications for space missions fully (Nguyen et al., 2021).
However, we envision a future where the astronauts’ helmets are equipped with micro gyroscopes and micro accelerometers, among other sensors, and are capable of applying GVS to stabilize the gaze and posture and contribute to astronauts’ orientation to counteract the effects of microgravity. Additionally, a non-helmet system may be used daily as an effective countermeasure against cortical alterations and to improve the sleep and wellbeing of crew members.
Author contributions
ES: Conceptualization, Formal Analysis, Investigation, Methodology, Project administration, Resources, Software, Supervision, Validation, Visualization, Writing–original draft, Writing–review and editing, Data curation, Funding acquisition. RV: Methodology, Project administration, Resources, Validation, Writing–original draft, Writing–review and editing, Investigation.
Funding
The author(s) declare that no financial support was received for the research, authorship, and/or publication of this article.
Acknowledgments
Agencia Espacial Mexicana (AEM) financed part of the research leading to this work, grant AEM-2016-1-275058 to RV (2016). Proof reading of the English manuscript was performed using ChatPT and Grammatic.
Conflict of interest
The authors declare that the research was conducted in the absence of any commercial or financial relationships that could be construed as a potential conflict of interest.
The author(s) declared that they were an editorial board member of Frontiers, at the time of submission. This had no impact on the peer review process and the final decision.
Publisher’s note
All claims expressed in this article are solely those of the authors and do not necessarily represent those of their affiliated organizations, or those of the publisher, the editors and the reviewers. Any product that may be evaluated in this article, or claim that may be made by its manufacturer, is not guaranteed or endorsed by the publisher.
Abbreviations
ASL, Arterial Spin Labeling; DMTg, Dorsomedial tegmental area; EEG, Electro encephalogram; fMRI, Functional magnetic resonance imaging; fNIRS, functional near-infrared spectroscopy; GVS, Galvanic Vestibular Stimulation; HDT, head-down tilt bedrest; Hb, hemoglobin; ISI, Insomnia Severity Index; LDTg, Lateral dorsal Tegmental area; PCG, LDTg, and pons central gray; HDT, Long duration head-down tilt bedrest; IPN, Interpeduncular nuclei; MS, Medial septal nucleus; MnR, Median raphe nucleus; NREM, non-Rapid Eye Movement sleep; HbO2, oxygenated hemoglobin; PB, Parabrachial area; PIVC, Parietoinsular vestibular cortex; LH and PH, Posterior hypothalamic areas; REM, Rapid eye movement; tDCS, Transcranial direct current stimulation; tACS, transcranial Alternating Current Stimulation; VR, Ventrolateral PAG (vlPAG), virtual reality.
References
Aedo Sánchez, C., Collao, J. P., and Délano Reyes, P. (2016). Anatomía, fisiología y rol clínico de la corteza vestibular. Rev. Otorrinolaringol. Cir. Cabeza Cuello. 76 (3), 337–346. doi:10.4067/S0718-48162016000300014
Aglietti, G. S. (2020). Current challenges and opportunities for space technologies. Front. Space Technol. 1, 1. doi:10.3389/frspt.2020.00001
Aleksandrov, V. V., Belen’kii, A. D., Bugrov, D. I., Lebedev, A. V., Lemak, S. S., and Guerrero Sanchez, W. F. (2011). Telemetry-based estimate of orientation accuracy for the tat’yana-2 satellite. Mosc. Univ. Mech. Bull. 66 (3), 72–75. doi:10.3103/S0027133011030058
Alexandrov, V., Lemak, S., Shkel, A., and Soto, E. (2004). Corrections of a vestibular function under extreme conditions of the aerospace flight. Proc. 16th IFAC Symposium Automatic Control Aerosp. 37 (6), 269–274. doi:10.1016/S1474-6670(17)32185-7
Alexandrov, V. V., Alexandrova, T. B., Bugrov, D. I., Lebedev, A. V., Lemak, S. S., Tikhonova, K. V., et al. (2014). Mathematical modeling of output signal for the correction of the vestibular system inertial biosensors. Int. Symposium Inert. Sensors Syst. IEEE Proc., 109–112. doi:10.1109/ISISS.2014.6782526
Alexandrov, V. V., Alexandrova, T. B., Vega, R., Sadovnichii, V. A., Sidorenko, Y. G., Soto, E., et al. (2021). Mathematical modeling of the information process in the angular acceleration biosensor. J. Math. Sci. 253 (6), 756–767. doi:10.1007/s10958-021-05267-9
Allred, A. R., Weiss, H., Clark, T. K., and Stirling, L. (2024). An augmented reality hand-eye sensorimotor impairment assessment for spaceflight operations. Aerosp. Med. Hum. Perform. 95 (2), 69–78. doi:10.3357/AMHP.6313.2024
Antal, A., Alekseichuk, I., Bikson, M., Brockmöller, J., Brunoni, A. R., Chen, R., et al. (2017). Low intensity transcranial electric stimulation: safety, ethical, legal regulatory and application guidelines. Clin. Neurophysiol. 128 (9), 1774–1809. doi:10.1016/j.clinph.2017.06.001
Barranco, N. (2020). Efecto de la estimulación galvánica vestibular (EGV) sobre los movimientos oculares. Tesis Maest. Ciencias Fisiológicas. Inst. Fisiol. BUAP. 25 noviembre2020.
Bayer, L., Constantinescu, I., Perrig, S., Vienne, J., Vidal, P. P., Mühlethaler, M., et al. (2011). Rocking synchronizes brain waves during a short nap. Curr. Biol. 21, R461–R462. doi:10.1016/j.cub.2011.05.012
Besnard, S., Tighilet, B., Chabbert, C., Hitier, M., Toulouse, J., Gall, L., et al. (2018). The balance of sleep: role of the vestibular sensory system. Sleep. Med. Rev. 42, 220–228. doi:10.1016/j.smrv.2018.09.001
Bigelow, R. T., and Agrawal, Y. (2015). Vestibular involvement in cognition: visuospatial ability, attention, executive function, and memory. J. Vestib. Res. 25, 73–89. doi:10.3233/VES-150544
Cevette, M. J., Stepanek, J., Cocco, D., Galea, A. M., Pradhan, G. N., Wagner, L. S., et al. (2012). Oculo-vestibular recoupling using galvanic vestibular stimulation to mitigate simulator sickness. Aviat. Space Environ. Med. 83 (6), 549–555. doi:10.3357/asem.3239.2012
Clément, G., Kuldavletova, O., Macaulay, T. R., Wood, S. J., Navarro Morales, D. C., Toupet, M., et al. (2023). Cognitive and balance functions of astronauts after spaceflight are comparable to those of individuals with bilateral vestibulopathy. Front. Neurol. 14, 1284029. doi:10.3389/fneur.2023.1284029
Clément, G. R., Bukley, A. P., and Paloski, W. H. (2015). Artificial gravity as a countermeasure for mitigating physiological deconditioning during long-duration space missions. Front. Syst. Neurosci. 9, 92. doi:10.3389/fnsys.2015.00092
Cohen, B., Martinelli, G. P., Xiang, Y., and RaphanYakushin, T. S. B. (2017). Vestibular activation habituates the vasovagal response in the rat. Front. Neurol. 8, 83. doi:10.3389/fneur.2017.00083
Crampton, G. H. (1990). “Neurophysiology of motion sickness,” in Motion and space sickness. Editor G. H. Crampton (Boca Raton, FL: CRC Press), 29–42.
Cullen, K. E. (2012). The vestibular system: multimodal integration and encoding of self-motion for motor control. Trends Neurosci. 35 (3), 185–196. doi:10.1016/j.tins.2011.12.001
Cullen, K. E. (2019). Vestibular processing during natural self-motion: implications for perception and action. Nat. Rev. Neurosci. 20, 346–363. doi:10.1038/s41583-019-0153-1
Dale, A., and Cullen, K. E. (2019). The ventral posterior lateral thalamus preferentially encodes externally applied versus active movement: implications for self-motion perception. Cereb. Cortex 29 (1), 305–318. doi:10.1093/cercor/bhx325
Demertzi, A., Van Ombergen, A., Tomilovskaya, E., Jeurissen, B., Pechenkova, E., Di Perri, C., et al. (2016). Cortical reorganization in an astronaut's brain after long-duration spaceflight. Brain Struct. Funct. 221 (5), 2873–2876. doi:10.1007/s00429-015-1054-3
De Waele, C., Baudonnière, P., Lepecq, J., Tran Ba Huy, P., and Vidal, P. P. (2001). Vestibular projections in the human cortex. Exp. Brain Res. 141, 541–551. doi:10.1007/s00221-001-0894-7
Dilda, V., MacDougall, H. G., Curthoys, I. S., and Moore, S. T. (2012). Effects of Galvanic vestibular stimulation on cognitive function. Exp. Brain Res. 216 (2), 275–285. doi:10.1007/s00221-011-2929-z
Dlugaiczyk, J., Gensberger, K. D., and Straka, H. (2019). Galvanic vestibular stimulation: from basic concepts to clinical applications. J. Neurophysiol. 121, 2237–2255. doi:10.1152/jn.00035.2019
Fife, T. D. (2010). “Overview of anatomy and physiology of the vestibular system,” in Vertigo and imbalance: clinical neurophysiology of the vestibular system. Handbook of clinical neurophysiology. Editors S. D. Z. Eggers, and S. Z. David (EUA: Elsevier).
Fitzpatrick, R. C., and Day, B. L. (2004). Probing the human vestibular system with galvanic stimulation. J. Appl. Physiol. 96 (6), 2301–2316. doi:10.1152/japplphysiol.00008.2004
Fredrickson, J. M., Scheid, P., Figge, U., and Kornhuber, H. H. (1966). Vestibular nerve projection to the cerebral cortex of the rhesus monkey. Exp. Brain Res. 2 (4), 318–327. doi:10.1007/BF00234777
Gensberger, K. D., Kaufmann, A. K., Dietrich, H., Branoner, F., Banchi, R., Chagnaud, B. P., et al. (2016). Galvanic vestibular stimulation: cellular substrates and response patterns of neurons in the vestibulo-ocular network. J. Neurosci. 36, 9097–9110. doi:10.1523/JNEUROSCI.4239-15.2016
Goldberg, J. M., Smith, C. E., and Fernández, C. (1984). Relation between discharge regularity and responses to externally applied galvanic currents in vestibular nerve afferents of the squirrel monkey. J. Neurophysiol. 51, 1236–1256. doi:10.1152/jn.1984.51.6.1236
Goothy, S. S. K., and McKeown, J. (2021). Modulation of sleep using electrical vestibular nerve stimulation prior to sleep onset: a pilot study. J. Basic Clin. Physiol. Pharmacol. 32 (2), 19–23. doi:10.1515/jbcpp-2020-0019
Groth, C., Tauscher, J. P., Heesen, N., Hattenbach, M., Castillo, S., and Magnor, M. (2022). Omnidirectional galvanic vestibular stimulation in virtual reality. IEEE Trans. Vis. Comput. Graph. 28 (5), 2234–2244. doi:10.1109/TVCG.2022.3150506
Hernández-Román, J., Montero-Hernández, S., Vega, R., Orihuela-Espina, F., and Soto, E. (2023). Galvanic vestibular stimulation activates the parietal and temporal cortex in humans: a functional near-infrared spectroscopy (fNIRS) study. Eur. J. Neurosci. 58 (1), 2267–2277. doi:10.1111/ejn.16041
Hitier, M., Besnard, S., and Smith, P. F. (2014). Vestibular pathways involved in cognition. Front. Integr. Neurosci. 8, 59. doi:10.3389/fnint.2014.00059
Houben, M. M. J., Stuldreher, I. V., Forbes, P. A., and Groen, E. L. (2024). Using galvanic vestibular stimulation to induce post-roll illusion in a fixed-base flight simulator. Aerosp. Med. Hum. Perform. 95 (2), 84–92. doi:10.3357/AMHP.6325.2024
Hupfeld, K. E., McGregor, H. R., Koppelmans, V., Beltran, N. E., Kofman, I. S., De Dios, Y. E., et al. (2022). Brain and behavioral evidence for reweighting of vestibular inputs with long-duration spaceflight. Cereb. Cortex 32 (4), 755–769. doi:10.1093/cercor/bhab239
Karim, H., Fuhrman, S. I., Sparto, P., Furman, J., and Huppert, T. (2013a). Functional brain imaging of multi-sensory vestibular processing during computerized dynamic posturography using near-infrared spectroscopy. Neuroimage 74, 318–325. doi:10.1016/j.neuroimage.2013.02.010
Karim, H. T., Fuhrman, S. I., Furman, J. M., and Huppert, T. J. (2013b). Neuroimaging to detect cortical projection of vestibular response to caloric stimulation in young and older adults using functional near-infrared spectroscopy (fNIRS). NeuroImage 76, 1–10. doi:10.1016/j.neuroimage.2013.02.061
Katzenberger, B., Brosch, F., Besnard, S., and Grill, E. (2023). Chronic vestibular hypofunction is associated with impaired sleep: results from the dizzyreg patient registry. J. Clin. Med. 12, 5903. doi:10.3390/jcm12185903
Kim, S., Lazaro, M. J., and Kang, Y. (2023). Galvanic vestibular stimulation to counteract leans illusion: comparing step and ramped waveforms. Ergonomics 66 (4), 432–442. doi:10.1080/00140139.2022.2093403
Kompotis, K., Hubbard, J., Emmenegger, Y., Perrault, A., Mühlethaler, M., Schwartz, S., et al. (2019). Rocking promotes sleep in mice through rhythmic stimulation of the vestibular system. Curr. Biol. 29, 392–401 e4. doi:10.1016/j.cub.2018.12.007
Koppelmans, V., Bloomberg, J. J., Mulavara, A. P., and Seidler, R. D. (2016). Brain structural plasticity with spaceflight. NPJ Microgravity 2, 2. Erratum in: NPJ Microgravity. 2017 Nov 27; 3: 30. doi:10.1038/s41526-016-0001-9
Kornilova, L. N., and Kozlovskaya, I. B. (2003). Neurosensory mechanisms of space adaptation syndrome. Hum. Physiol. 29 (5), 527–538. Translated from Fiziologiya Cheloveka, 29(5), 17–28. doi:10.1023/a:1025899413655
Kornilova, L. N., Naumov, I. A., Glukhikh, D. O., Ekimoskviy, A. S., Pavlova, V. V., Khabarova, V. V., et al. (2017). Vestibular function and space motion sickness. Hum. Physiol. 43, 557–568. doi:10.1134/S0362119717050085
Krystal, A. D., Zammit, G. K., Wyatt, J. K., Quan, S. F., Edinger, J. D., White, D. P., et al. (2010). The effect of vestibular stimulation in a four-hour sleep phase advance model of transient insomnia. J. Clin. Sleep. Med. 6 (4), 315–321. doi:10.5664/jcsm.27871
Lopez, C., and Blanke, O. (2011). The thalamocortical vestibular system in animals and humans. Brain Res. Rev. 67 (1-2), 119–146. doi:10.1016/j.brainresrev.2010.12.002
Lopez, C., Blanke, O., and Mast, F. W. (2012). The human vestibular cortex revealed by coordinate-based activation likelihood estimation meta-analysis. Neuroscience 212, 159–179. doi:10.1016/j.neuroscience.2012.03.028
Lopez, C., and Cullen, K. E. (2024). Electrical stimulation of the peripheral and central vestibular system. Curr. Opin. Neurol. 37 (1), 40–51. doi:10.1097/WCO.0000000000001228
Matsugi, A., Nagino, K., Shiozaki, T., Okada, Y., Mori, N., Nakamura, J., et al. (2021). No impact of stochastic galvanic vestibular stimulation on arterial pressure and heart rate variability in the elderly population. Front. Hum. Neurosci. 15, 646127. doi:10.3389/fnhum.2021.646127
Mitsutake, T., Sakamoto, M., Kawaguchi, A., Tamari, M., and Horikawa, E. (2020). Greater functional activation during galvanic vestibular stimulation is associated with improved postural stability: a GVS-fMRI study. Somatosens. Mot. Res. 37 (4), 257–261. doi:10.1080/08990220.2020.1803256
Moore, S. T., Dilda, V., Hamish, G., and MacDougal, H. G. (2011). Galvanic vestibular stimulation as an analogue of spatial disorientation after spaceflight. Aviat. Space Environ. Med. 82 (5), 535–542. doi:10.3357/asem.2942.2011
Mulavara, A. P., Peters, B. T., Miller, C. A., Kofman, I. S., Reschke, M. F., Taylor, L. C., et al. (2018). Physiological and functional alterations after spaceflight and bed rest. Med. Sci. Sports Exerc 50 (9), 1961–1980. doi:10.1249/MSS.0000000000001615
Nakatsuka, D., Kanda, T., Sato, M., Ishikawa, Y., Cherasse, Y., and Yanagisawa, M. (2024). A novel GABAergic population in the medial vestibular nucleus maintains wakefulness and gates rapid eye movement sleep. iScience 27, 109289. doi:10.1016/j.isci.2024.109289
Nguyen, T. T., Nam, G.-S., Kang, J.-J., Han, G. C., Kim, J.-S., Dieterich, M., et al. (2021). Galvanic vestibular stimulation improves spatial cognition after unilateral labyrinthectomy in mice. Front. Neurol. 12, 716795. doi:10.3389/fneur.2021.716795
Norris, C. H., Miller, A. J., Perin, P., Holt, J. C., and Guth, P. S. (1998). Mechanisms and effects of transepithelial polarization in the isolated semicircular canal. Hear Res. 123, 31–40. doi:10.1016/S0378-5955(98)00096-3
Ödkvist, L. M., Schwarz, D. W. F., Fredrickson, J. M., and Hassler, R. (1974). Projection of the vestibular nerve to the area 3a arm field in the squirrel monkey (Saimiri Sciureus). Exp. Brain Res. 21, 97–105. doi:10.1007/BF00234260
Omlin, X., Crivelli, F., Näf, M., Heinicke, L., Skorucak, J., Malafeev, A., et al. (2018). The effect of a slowly rocking bed on sleep. Sci. Rep. 8 (1), 2156. doi:10.1038/s41598-018-19880-3
Pechenkova, E., Nosikova, I., Rumshiskaya, A., Litvinova, L., Rukavishnikov, I., Mershina, E., et al. (2019). Alterations of functional brain connectivity after long-duration spaceflight as revealed by fMRI. Front. Physiol. 10, 761. doi:10.3389/fphys.2019.00761
Perrault, A. A., Khani, A., Quairiaux, C., Kompotis, K., Franken, P., Muhlethaler, M., et al. (2019). Whole-night continuous rocking entrains spontaneous neural oscillations with benefits for sleep and memory. Curr. Biol. 29, 402–411.e3. doi:10.1016/j.cub.2018.12.028
Pixa, N. H., Steinberg, F., and Doppelmayr, M. (2017). Effects of high-definition anodal transcranial direct current stimulation applied simultaneously to both primary motor cortices on bimanual sensorimotor performance. Front. Behav. Neurosci. 11, 130. doi:10.3389/fnbeh.2017.00130
Pliego, A., Vega, R., Gómez, R., Reyes-Lagos, J., and Soto, E. (2021). A transient decrease in heart rate with unilateral and bilateral galvanic vestibular stimulation in healthy humans. Eur. J. Neurosci. 54, 4670–4681. doi:10.1111/ejn.15338
Pradhan, G. N., Galvan-Garza, R., Perez, A. M., Bogle, J., and Cevette, M. J. (2022). Generating flight illusions using galvanic vestibular stimulation in virtual reality flight simulations. Front. Neuroergon. 3, 883962. doi:10.3389/fnrgo.2022.883962
Sadovnichii, V. A., Aleksandrov, V. V., Aleksandrova, O. V., Vega, R., Konovalenko, I. S., Soto, E., et al. (2019). Galvanic correction of pilots' vestibular activity during visual flight control. Mosc. Univ. Mech. Bull. 74, 1–8. doi:10.3103/S0027133019010011
Sadovnichii, V. A., Alexandrov, V. V., Bugrov, D. I., Lemak, S. S., Pakhomov, V. B., Panasyuk, M. I., et al. (2018). The IMISS-1 experiment for recording and analysis of accelerations in orbital flight. Space Sci. Rev. 214, 51. doi:10.1007/s11214-018-0470-0
Sadovnichii, V. A., Panasyuk, M. I., Yashin, I. V., Barinova, V. O., Veden'kin, N. N., Vlasova, N. A. M., et al. (2011). Investigations of the space environment aboard the Universitetsky-Tat'yana and Universitetsky-Tat'yana-2 microsatellites. Sol. Sys. Res. 45, 3–29. doi:10.1134/S0038094611010096
Saradjian, A. H., Paleressompoulle, D., Louber, D., Coyle, T., Blouin, J., and Mouchnino, L. (2014). Do gravity-related sensory information enable the enhancement of cortical proprioceptive inputs when planning a step-in microgravity? PLoS One 9 (9), e108636. doi:10.1371/journal.pone.0108636
Scinicariello, A., Eaton, K., Inglis, J., and Collins, J. J. (2001). Enhancing human balance control with galvanic vestibular stimulation. Biol. Cybern. 84, 475–480. doi:10.1007/PL00007991
Sendesen, E., Kocabay, A. P., and Yiğit, Ö. (2024). Does sleep quality affect balance? The perspective from the somatosensory, vestibular, and visual systems. Am. J. Otolaryngol. 45 (3), 104230. doi:10.1016/j.amjoto.2024.104230
Smith, L. J., Wilkinson, D., Bodani, M., and Surenthiran, S. S. (2024). Cognition in vestibular disorders: state of the field, challenges, and priorities for the future. Front. Neurol. 15, 1159174. doi:10.3389/fneur.2024.1159174
Soto, E., Vega, R., Alexandrova, T., Alexandrov, V., Reyes, M., Pliego, A., et al. (2014). Vestibular prosthesis. U. S. Pat. no. 8 855, 774. B2.
St George, R. J., Day, B. L., and Fitzpatrick, R. C. (2011). Adaptation of vestibular signals for self-motion perception. J. Physiol. 589 (Pt 4), 843–853. doi:10.1113/jphysiol.2010.197053
Tays, G. D., Hupfeld, K. E., McGregor, H. R., Beltran, N. E., De Dios, Y. E., Mulder, E., et al. (2024). Daily artificial gravity partially mitigates vestibular processing changes associated with head-down tilt bedrest. NPJ Microgravity. 10 (1), 27. doi:10.1038/s41526-024-00367-7
Utz, K. S., Dimova, V., Oppenländer, K., and Kerkhoff, G. (2010). Electrified minds: transcranial direct current stimulation (tDCS) and galvanic vestibular stimulation (GVS) as methods of non-invasive brain stimulation in neuropsychology--a review of current data and future implications. Neuropsychologia 48 (10), 2789–2810. doi:10.1016/j.neuropsychologia.2010.06.002
Valdés, B. A., Lajoie, K., Marigold, D. S., and Menon, C. (2021). Cortical effects of noisy galvanic vestibular stimulation using functional near-infrared spectroscopy. Sensors 21 (4), 1476. doi:10.3390/s21041476
Vega, R., GordilloAlexandrovAlexandrova, J. V. V. T., Soto, E., and Soto, E. (2020). Use of vestibular galvanic stimulation for correction of the position and of the gaze in a flight simulator. BioRxiv. doi:10.1101/2020.05.22.111146
Vega, R., Soto, E., Gordillo, J., Pliego, A. C., Alexandrov, V., Alexandrova, T., et al. (2023). Device for the use of vestibular galvanic stimulation for pilot training and the correction of the position and sight fixation in microgravity. U. S. Pat. US11721233B2.
Vega, R., Soto, E., Pliego, A., Alexandrov, A., and Alexandrova, A. (2016). Dispositivo para la estabilización de la postura en microgravedad (Cap. 9). Medicina Espacial. Coordinadores: Raúl Carrillo Esper, Díaz Ponce Medrano J A. Padrón S. Juan. Acad. Nac. Med., 121–137.
Young, L. R. (1974). “Role of the vestibular system in posture and movement,” in Medical physiology. 13th ed. (Mosby Company), 704–720.
Zago Mazzocco, B. (2018). Desarrollo de un dispositivo para estimulación galvánica vestibular y efecto de la estimulación magnética en el reflejo vestíbulo-ocular en sujetos voluntarios. Tesis Maest. Ciencias Fisiológicas. Inst. Fisiol. BUAP.
Zheng, X., Alsop, D. C., and Schlaug, G. (2011). Effects of transcranial direct current stimulation (tDCS) on human regional cerebral blood flow. NeuroImage 58 (1), 26–33. doi:10.1016/j.neuroimage.2011.06.018
Zhu, X., Ren, Y., Tan, S., and Ma, X. (2024). Efficacy of transcranial alternating current stimulation in treating chronic insomnia and the impact of age on its effectiveness: a multisite randomized, double-blind, parallel-group, placebo-controlled study. J. Psychiatr. Res. 170, 253–261. doi:10.1016/j.jpsychires.2023.12.037
Keywords: human spaceflight, space medicine, microgravity countermeasure, GVS, vestibular prosthesis, space adaptation syndrome
Citation: Soto E and Vega R (2024) Use of galvanic vestibular stimulation device as a countermeasure for microgravity effects in spaceflight. Front. Space Technol. 5:1422868. doi: 10.3389/frspt.2024.1422868
Received: 24 April 2024; Accepted: 14 June 2024;
Published: 05 July 2024.
Edited by:
Saswati Das, Atal Bihari Vajpayee Institute of Medical Sciences and Dr. Ram Manohar Lohia Hospital, IndiaReviewed by:
Funmilola Oluwafemi, National Space Research and Development Agency (NASRDA), NigeriaT. John Tharakan, Indian Space Research Organisation, India
Copyright © 2024 Soto and Vega. This is an open-access article distributed under the terms of the Creative Commons Attribution License (CC BY). The use, distribution or reproduction in other forums is permitted, provided the original author(s) and the copyright owner(s) are credited and that the original publication in this journal is cited, in accordance with accepted academic practice. No use, distribution or reproduction is permitted which does not comply with these terms.
*Correspondence: Enrique Soto, ZXNvdG8yNEBnbWFpbC5jb20=