- 1NASA Glenn Research Center, Cleveland, OH, United States
- 2KBR, Houston, TX, United States
- 3ZIN Technologies, Inc., Cleveland, OH, United States
- 4NASA Johnson Space Center, Houston, TX, United States
Throughout the history of human spaceflight, spacefarers have experienced and reported the occurrence of medical conditions, including various illnesses and injuries. Therefore, future spaceflight missions to the Moon and Mars will require the capabilities necessary for maintaining the health of these new space travelers. Mass, power, and volume available in the space vehicles used for these missions will be severely constrained. The ability to resupply or evacuate to Earth will be limited or non-existent, and ground-based support will no longer be immediate due to communication latencies and blackouts. These vehicle and mission constraints will necessitate healthcare be provided from an efficiently planned medical system. To provide the necessary care, these medical systems will need to include at a minimum, several different types of medical devices, consumable resources, centralized data management, procedural guidance, and decision support technologies. Medical devices needed for diagnosing and treating medical conditions that are expected to occur during future spaceflight missions may include real-time health monitoring, medical imaging capabilities, as well as blood and urine analysis. Novel methods for interacting with onboard patient medical records will be necessary, as will resource tracking. Terrestrial medicine shares many of these same needs, therefore a multitude of these required medical capabilities can likely be satisfied by currently available, Commercial-Off-The-Shelf (COTS) devices and methodologies; however, in some cases the unique space environment and increased mission durations will drive the need for modifications or customization of standard technologies and treatment procedures. This article will provide a review of medical devices and technologies that have been considered for inclusion within future spaceflight medical systems. It will also include a discussion about the modifications and customized development that have been performed, as well as descriptions of the technology demonstrations that have been conducted in analog and spaceflight environments.
1 Introduction
Medical illnesses, injuries, medical responses to environmental factors, and significant medical contingencies have occurred throughout the history of human spaceflight. On the International Space Station (ISS), astronauts have experienced symptoms associated with bacterial or viral infections, clinical viral reactivation, hypersensitive skin, and increased incidences of allergies (Crucian, Babiak-Vazquez, et al., 2016). Musculoskeletal injuries and trauma occur in spaceflight, including abrasions, contusions, strains, lacerations, sprains, and dislocations (Scheuring, et al., 2009; Ramachandran, et al., 2018). The most common location for these injuries is the hand, but they also occur in other locations such as the back, shoulder, foot, arms, and legs (Scheuring, et al., 2009; Ramachandran, et al., 2018). Astronauts are particularly vulnerable to musculoskeletal injury during Extravehicular Activity (EVA) activities (Scheuring, et al., 2009; Ramachandran, et al., 2018), and complicating the understanding of musculoskeletal injury within the back is the phenomenon of space adaptation back pain, a medical condition that is unique to exposure and adaptation to the space environment (Kerstman, et al., 2012). There have also been notable spaceflight medical contingencies such as deep vein thrombosis on the ISS (Auñón-Chancellor, et al., 2020) and a severe urinary tract infection on Apollo 13 (Johnston et al., 1975). Although medical events occur in space, the robust medical systems incorporated within spaceflight missions to date have been able to obviate the need for a mission-ending medical evacuation in the United States spaceflight program (Nowadly, et al., 2019). Medical events similar to those experienced during Low Earth Orbit (LEO) missions and short-term Apollo missions will be a concern during future exploration missions to the Moon and Mars, along with medical events unique to exploration mission environments. Therefore, the medical systems provided during exploration missions will need to contain the necessary components to manage the medical events that occur.
There will be unique challenges associated with the exploration missions compared to LEO mission and the exploration medical systems will need to be designed to overcome these challenges. During the Lunar Artemis and Martian missions, mission architectures will incorporate the use of several different types of space vehicles, orbiting stations, and surface habitats (Creech et al., 2022; Choate, et al., 2023; Goodliff, et al., 2023). Although the spacecrafts will have different shapes and sizes, they will all likely have much less habitable volume and more severe constraints on allocated mass and power consumption than is currently available on the ISS. As a result, exploration medical system design challenges include maximizing the ability to manage medical events while minimizing the amount of mass, volume, and power needed for the medical system (Levin, et al., 2023). The ability to manage medical events includes providing resources and capabilities sufficient for preventing, diagnosing, treating, and rehabilitating medical conditions, while organizing them efficiently and effectively for use in microgravity and/or partial gravity environments. It will therefore be important for the medical system to have multiple components distributed within the various spacecrafts, to have key elements of the system be modular and portable to reduce redundancy across vehicles, and to have all components be seamlessly integrated to function together as one cohesive medical system.
Additionally, humans will fly farther from Earth than they ever have before on these future exploration missions. Challenges associated with these far distances when designing exploration medical systems include communication latencies, resupply limitations, and evacuations that vary from difficult to impossible (Figure 1) (Creech et al., 2022; Choate, et al., 2023; Goodliff, et al., 2023; Thompson, et al., 2023). Currently during ISS missions, a cadre of ground-based medical specialists provide daily real-time support to the crew both during routinely scheduled medical exams and when unplanned medical attention is needed (Doarn, 2011; Doarn, Polk, et al., 2021; Richardson and Piper, 2022). Additionally, relatively quick medical evacuation from the ISS to the Earth is available for severe medical conditions (Doarn, 2011; Nowadly, et al., 2019; Doarn, Polk, et al., 2021; Richardson and Piper, 2022). A paradigm shift is needed from these LEO practices for the future exploration missions. The far distances traveled will cause communication latencies to be experienced between the space vehicle and ground support, up to 24 min each direction between the Earth and Mars, and soon after setting out on an interplanetary trajectory, evacuation is no longer a viable option (Levin, et al., 2023). To accommodate these challenges, the medical systems and exploration mission crew will need to be increasingly self-sufficient. The crew will need to have the skills necessary to manage medical events and be supported by Just-In-Time (JIT) in-flight training, procedural guidance, and decision support tools. With less opportunities for resupply during these missions compared to ISS missions, medical system design will need to optimize critical medical resources and capabilities, while taking into consideration provisions for medical equipment maintenance and (Levin, et al., 2023). Additionally, some of the consumable medical system resources currently used on Earth and on ISS are not designed to have long duration shelf lives or to withstand harsh spaceflight environments. Because of this, degradation of these resources could be experienced over the course of a long-duration deep space mission (Nelson and Arnon, 2010; Mehta and Bhayani, 2017; Watkins, et al., 2022).
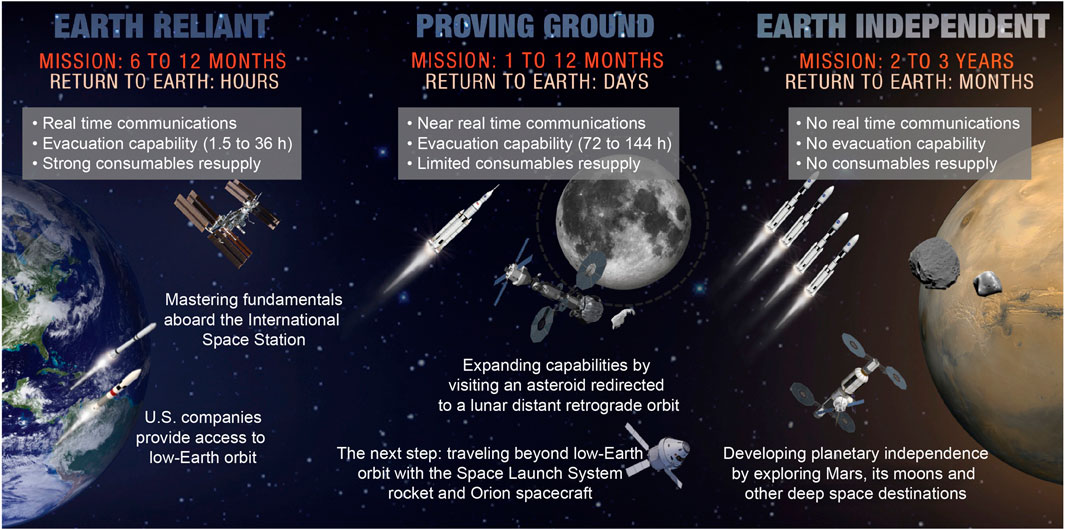
Figure 1. Graphical representation of the challenges associated with increasingly longer and farther from Earth space missions, taken directly from (Thompson, et al., 2023).
The National Aeronautics and Space Administration (NASA) Human System Risk Board (HSRB) defines and tracks exploration mission challenges through the maintenance of risk postures for the adverse outcomes to human health and performance that could occur during spaceflight. Risk posture is defined by the likelihood of occurrence and the consequence of occurrence to astronaut health and mission success (Antonsen, Connell, et al., 2023). The HSRB Medical Risk, stated as “the risk of adverse health outcomes and decrements in performance due to medical conditions that occur in mission, as well as long term health outcomes due to mission exposures”, encompasses the medical outcomes of individual risks as well as all other medical outcomes that may occur during spaceflight (Antonsen, Myers, et al., 2022). The identification of the HSRB Medical Risk drives the need to have medical care available for the astronauts during spaceflight. To date, this is a critical risk for long duration Lunar and Martian missions, and key information and technological advancements for the spaceflight medical systems are still needed for mitigation of the potential consequences. One specific mitigation step for the HSRB Medical Risk is to increase inflight medical capabilities and identify new capabilities that a) maximize benefit and/or b) reduce resource costs associated with the human system, mission, or vehicle (Antonsen, Myers, et al., 2022).
Part of NASA’s preparation for missions that will return humans to the Moon includes an effort where gaps in technological capabilities necessary for sustainability on the Lunar surface are identified, mapped to spaceflight architectural elements, and categorized into broad technology taxonomy areas (Burg, et al., 2021). The Human Health, Life Support, and Habitation Systems taxonomy area encompasses the Environmental Control and Life Support System (ECLSS) and Habitation Systems, Environmental Monitoring, Safety and Emergency Response, EVA Systems, Human Health and Performance, and Radiation. This paper will focus on the Human Health and Performance area and a subset of the Human System Capability Gaps derived within this area to identify capabilities necessary to maintain crew health and performance on future space missions (Abercromby, et al., 2022). Each of the Human System Capability Gaps are mapped to a human system risk to help inform and prioritize technology development and research investments across NASA (Abercromby, et al., 2022). The gaps that will be addressed within this paper and the roadmap of tasks planned for closing the gaps include: 1) Medical Imaging, Diagnostics, and Treatment Technologies (Figure 2); 2) In-situ Sample Storage, Processing and Analysis (Figure 3); and 3) Operational Medical Decision Support Software and Informatics (Figure 4) (Thompson, et al., 2023). These are gaps that map to the HSRB Medical Risk and define the technical challenges that need to be resolved prior to being able to design and realize an exploration medical system which will effectively provide management of the medical events that may occur during future space exploration missions. Excluded from discussion within this paper are the following gaps: Medical Concepts of Operations, Crew Health and Performance (CHP) Integrated Data Architecture, Integrated Medical Simulation Technologies, Medical Risk Model and Trade Space Analysis Tools, Semi-autonomous Behavioral Health and Performance, and Safe and Effective Pharmaceuticals (Thompson, et al., 2023). The Exploration Medical Capability (ExMC) Element of NASA’s Human Research Program (HRP) and the Exploration Medical Integrated Product Team (XMIPT) of NASA’s Mars Campaign Office engage in technology projects defined on the Human System Capability Gaps roadmaps, which have the potential to mitigate medical risk and to fulfill technology gaps.
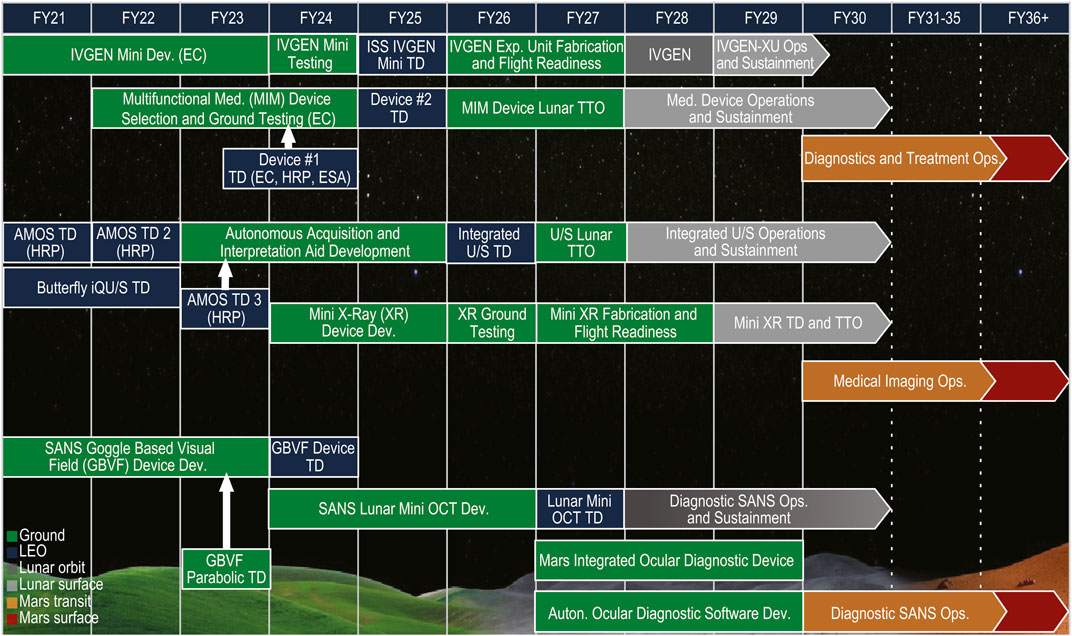
Figure 2. The roadmap of tasks that are planned for closing the Medical Imaging, Diagnosis, and Treatment NASA Human System Capability Gap, taken directly from (Thompson, et al., 2023) (LEO = Low Earth Orbit; IVGEN = Intravenous Fluid Generation; Dev. = Development; EC = Exploration Capabilities; ISS = International Space Station; TD = Technology Demonstration; Exp. = Exploration; IVGEN-XU = IVGEN Exploration Unit; Ops = Operations; Med. = Medical; MIM = Multi-functional Integrated Medical; TTO = Transition to Operations; HRP = Human Research Program; ESA = European Space Agency; Diag. = Diagnosis; AMOS = Autonomous Medical Officer Support; U/S = ultrasound; XR = X-Ray; SANS = Spaceflight Associated Neuro-ocular Syndrome; GBVF = Goggle Based Visual Field; OCT = Optical Coherence Tomography; Auton. = Autonomous).
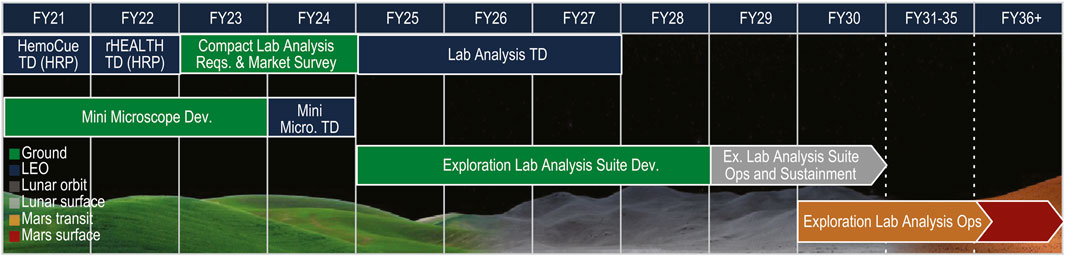
Figure 3. The roadmap of tasks that are planned for closing the In-situ Sample Storage, Processing and Analysis NASA Human System Capability Gap, taken directly from (Thompson, et al., 2023) (LEO = Low Earth Orbit; TD = Technology Demonstration; HRP = Human Research Program; Dev. = Development; Micro. = Microscope; Ex. = Exploration; Ops = Operations).
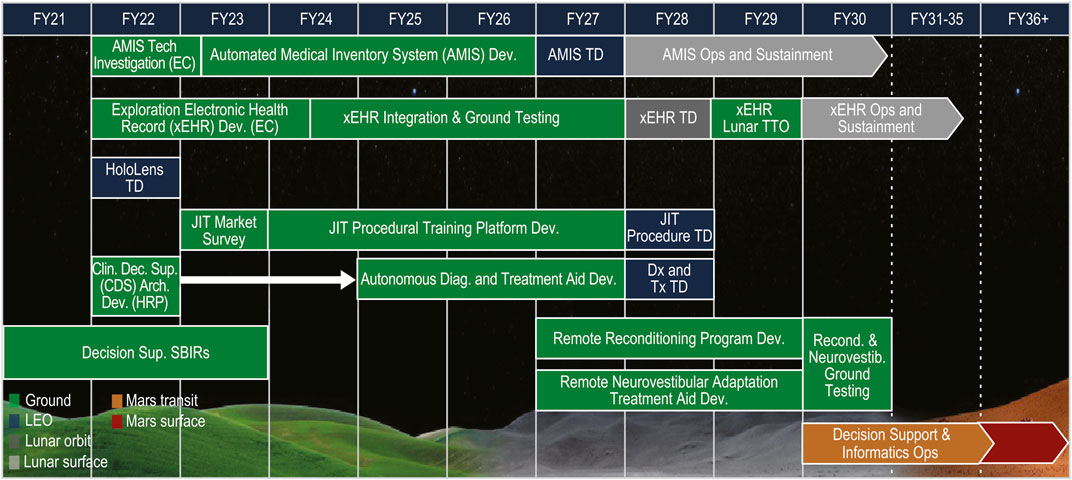
Figure 4. The roadmap of tasks that are planned for closing the Operational Medical Decision Support Software and Informatics NASA Human System Capability Gap, taken directly from (Thompson, et al., 2023) (LEO = Low Earth Orbit; AMIS = Automated Medical Inventory System; Tech = Technology; EC = Exploration Capabilities; Dev. = Development; TD = Technology Demonstration; Ops = Operations; xEHR = Exploration Electronic Health Record; TTO = Transition to Operations; JIT = Just in Time; Proced = Procedural; Clin = Clinical; Dec. = Decision; Sup. = Support; Arch. = Architecture; HRP = Human Research Program; Diag. = Diagnosis; Dx = Diagnosis; Tx = Treatment; SBIRs = Small Business Innovation Research projects; Recond. = Reconditioning; Neurovestib. = Neurovestibular).
The following subsections within this paper will provide information about the medical conditions expected to occur or which could occur on exploration missions. A description of the ISS capabilities currently used to treat medical events in LEO will be given. Information will be provided about medical capabilities and technologies that have the potential to mitigate the HSRB Medical Risk and to fulfill the Human System Capability Gaps, as well as descriptions of the Earth-based and spaceflight technology demonstrations that have been performed to gather evidence for or against the inclusion these technologies within future exploration medical systems. Finally, a discussion will be provided about modifications and customizations that often need to be made to ensure medical devices operate properly in the space environment, including explanations as to why certain devices may function adequately on Earth but may not perform accurately within a microgravity environment.
2 Medical conditions likely to occur during space exploration missions and technologies needed to manage the conditions
NASA has created several lists of spaceflight medical conditions for various reasons. The medical conditions within the lists are conditions that have occurred during previous spaceflights or have the potential to occur during a future spaceflight. NASA standards outline the requirement that astronauts be provided with healthcare throughout and after their career as an astronaut (NASA-STD-3001 2023). Table E.2-2 within (NASA-STD-3001 2023) contains a list of medical conditions that can occur in spaceflight, categorized into different types of conditions, with the highest likelihood and highest consequence conditions indicated. A medical condition list, termed the Accepted Medical Condition List, was developed to aid prioritization of spaceflight medical conditions based upon their HSRB designated likelihood and consequence rating (Blue et al., 2019). A list of medical conditions that have or could occur in LEO was devised for use within the Integrated Medical Model (IMM), which is a probabilistic model that uses simulation to predict ISS medical risk (Keenan, et al., 2015; Walton and Kerstman, 2020). The IMM uses the incidence rate of occurrence of ISS medical events as the input to its simulations. The incidence rates for frequently observed conditions were derived from available astronaut clinical data (Walton and Kerstman, 2020). For less frequently occurring conditions, Bayesian updates to incidence rates from analog populations were used to derive the spaceflight incidence rates (Gilkey, et al., 2012), and computational modeling was use to derive incidence rates for rare occurrence spaceflight medical events (Nelson, Lewandowski, et al., 2009). Most recently, the LEO medical condition list has been updated for exploration missions and for use within a new probabilistic analysis tool, Informing Mission Planning via Analysis of Complex Tradespaces (IMPACT), designed for performing risk assessment for exploration-class missions (Blue et al., 2019; Kreykes, et al., 2023).
The medical conditions mentioned above have some probability of occurrence on any type of space mission. However, there are some types of conditions that have a higher probability of occurrence and/or have a higher consequence if they occur during exploration missions to the Moon and Mars. For example, any missions with planetary extravehicular activity will be expected to have an increase in incidence rate of musculoskeletal related medical conditions such as sprains and strains, dislocations, fractures, contusions, blunt trauma to the head, chest or abdomen, and vertebral disk injury (Ramachandran, et al., 2018). Exposure to planetary dust will be unique to exploration missions and therefore the likelihood of experiencing conditions associated with toxic exposure to dust will need to be considered (Pohlen, et al., 2022). Exploration missions will require multiple gravity transitions throughout the mission, including transitions from Earth’s gravity to microgravity, transitions from microgravity to partial gravity, transitions from partial gravity back to microgravity and finally transitions from microgravity back to Earth’s gravity. These multiple transitions increase the likelihood of occurrences of gravity transition neurovestibular disturbances, space motion sickness and other space adaptation medical conditions.
Currently on the ISS, the medical capabilities available to prevent, diagnose, treat, and rehabilitate the medical conditions that occur include advanced and basic life support capabilities, Intravenous (IV) fluid administration supplies, the ability to treat decompression sickness, provisions for dental care, in-flight biomedical monitoring of various vital signs, and telemedicine capabilities. There are several medication packs available containing oral, topical, and injectable medications for treating a variety of routine and emergent symptoms. The medical supply and treatment packs contain bandages, gauze, and other supplies for treating minor injuries (NASA-JSC-CN-24908, 2011; NASA-STD-3001 2023). There are procedures in place addressing stabilization and transport, ambulatory medical care, how to make decisions on treatment vs. evacuation, evacuation procedures and procedures for death of a crew member (NASA OCHMO-TB-033, 2023; NASA-STD-3001 2023). Most of the medical capabilities currently provided on the ISS will also be necessary within exploration medical systems along with additional or enhanced capabilities. For example, the ability to perform medical imaging and biochemistry analysis will be desirable on exploration missions and these capabilities will need to be enhanced over what is currently available on the ISS. Improvements to IV fluid and medical oxygen administration technologies are needed for exploration missions. Efficiencies and advancements in the technologies used to collect vital sign measurements, perform inventory tracking, and medical record keeping are also necessary. The next sections provide descriptions of different types of medical technology that has the potential to satisfy exploration spaceflight medical system requirements.
3 Medical device technology identification, development and demonstration in preparation for exploration medical systems
Medical devices and technologies that have potential use within exploration medical systems are introduced within the following paragraphs. These are technologies that NASA has developed, evaluated, modified, and/or demonstrated in various environmental and gravitational platforms. The key aspects of the technology as it relates to its use in a spaceflight medical system, the challenges necessary to mature the technology to a spaceflight readiness level, and descriptions of demonstrations of the devices and technology that have been performed to date are provided. The devices and technologies introduced do not constitute a comprehensive review of what is currently available or what may be available in the future, but rather represent products that were determined by NASA’s ExMC and XMIPT to be beneficial for reducing the HSRB spaceflight medical risk and for fulfilling the current gaps in knowledge and technological need as outlined in the Human System Capability Gaps. NASA’s ExMC and XMIPT select candidate technologies using various means, such as market surveys, independent subject matter expert opinion, technical interchange meetings, and directed studies. The actual project technologies are generally selected after internal NASA review which weighs both cost and benefit factors.
The technology examples include a mixture of standard COTS products, COTS products modified for a specific spaceflight use, and technologies that are custom designed and built specifically for spaceflight applications. Part of the technology maturation process includes performance of technology demonstrations, which are often used by NASA as a method to aid the development of system requirements, to support design decision making, and to verify and validate operational performance within the environment the technology will be used (Seyedmadani, et al., 2023). NASA utilizes a Technology Readiness Level (TRL) scale to track advancements in the maturity state of spaceflight technology that is developed, modified, or demonstrated. The NASA TRL scale starts at TRL 1, which indicates basic research. Feasibility of technology is established at TRL 3, typically through ground-based demonstration in a laboratory setting. Technology development continues through TRL 6, when demonstrations of functionality are often performed in environments similar to those experienced during operations, such as spaceflight analogs (Cromwell, et al., 2021). A technology can reach TRL 7 when first demonstrated in the space environment, will advance to a TRL 8 when flight qualified and will reach TRL 9 once flight proven through successful mission operations (NASA SP-2016-6105 2016; NASA SP-20205003605 2020). Reduced gravity flights, sub-orbital, and commercial partner orbital missions are examples of platforms where technology can be tested over short periods of time in microgravity and are often used as a stepping-stone to experiments and testing performed on the ISS. The descriptions in the following sections provide information on how some of the required spaceflight medical device technology is being advanced towards the TRL 9 level in preparation for its use in future exploration medical systems.
3.1 Medical imaging, diagnostics, and treatment technologies
Flight-tested medical imaging, diagnostic, and treatment technologies are necessary to effectively manage medical conditions relevant to exploration missions, which meet vehicle constraints (e.g., mass, volume, power, data), integrate with medical decision-support tools, and enable increasingly Earth-independent operations.
3.1.1 Imaging technologies
Medical radiography increases the range of conditions that can be diagnosed and treated on exploration missions, buying down health and operational risk. Current clinical x-ray devices have a large mass, volume, and power footprint. Given that mass, volume, and power constraints will be more restrictive for Mars missions as compared to the ISS, an x-ray device with low resource requirements that can be accommodated by the vehicle will be needed for medical x-ray imaging in space. Autonomous acquisition and interpretation aides are also of importance to decrease reliance on ground teams and preflight training, and to expedite care.
NASA’s XMIPT performed a market survey in 2023 to identify existing and in-development medical x-ray devices that have the mass, volume, and power characteristics that may allow for use on long duration spaceflight missions. The imaging capabilities specified in the survey included dental, extremities, spine, chest, abdomen, pelvic, head, neck, and breast. Other desired capabilities included autonomous support including JIT training, procedural guidance, interpretation aids, Food and Drug Administration (FDA) approval, and robustness against electromagnetic interference, vibration, radiation, and other types of environmental exposures.
The devices identified in the market survey weighed between 1.8–7.7 kg and had volumes between 4.7–11 L. These measurements do not include an additional 35.6 × 43 × 1.3 cm, 2.7 kg panel detector accessory used with the x-ray devices. An example of the power requirements for one of the x-ray generators explored in the market survey has a voltage supply of 100–240 VAC, at a frequency of 50–60 Hz, drawing 1.5 A of current. The high-ranking devices were capable of imaging all specified areas except for the breast. These devices are FDA-approved and include aids within their software for improving imaging results and for image interpretation. The largest differences between the top devices were successful demonstrations of device functionality in austere environments and dental x-ray capabilities. Some devices are marketed for at-home use while others are ruggedized for use in remote areas. Furthermore, as dental x-rays are commonly taken with a separate device, most of the manufacturers have either not tested their device for dental use or currently have little data for that application. However, all companies expect dental x-rays to be possible with their devices. The next step for XMIPT is to select a device for a technology demonstration on the ISS leveraging the results of the decision matrix informed by the market survey. The goal of the x-ray ISS technology demonstration will be to assess the feasibility of the use of the x-ray devices in space, including determining the ability to flight certify the devices, the quality of the images, and the sensitivity of the device electronics to interferences.
3.1.2 Multi-functional integrated medical (MIM) devices
Currently on ISS there is a collection of discrete medical devices used to periodically assess crew health status. NASA is currently in the process of evaluating if utilization of a single vital sign monitoring system integrated with other medical capabilities on future exploration missions may improve communications, reduce training requirements and be less resource intensive. The Tempus Pro™ (Remote Diagnostic Technologies, Ltd., Philips Corp., Farnborough, UK) is a Multi-functional Integrated Medical (MIM) device that includes vital signs monitoring, on-board procedure support (iAssist), telemedicine communication features, and medical imaging (Figure 5). Usability testing of the Tempus Pro™ was completed within the NASA Human Exploration System Testbed for Integration and Advancement (HESTIA), which is a hypobaric chamber located at the NASA Johnson Space Center (JSC) (Marmolejo and Ewert, 2016), during a 2023 Exploration Atmosphere and Pre-breathe Validation study (Garbino, et al., 2024). The atmosphere of a spacecraft may be kept at a higher oxygen concentration and lower partial pressure than experienced at sea level on Earth and therefore it is often important to determine the functionality of equipment and the physiological response to that atmosphere. As part of this effort, multiple participants, while living in the HESTIA chamber stepped through medical procedures with the Tempus Pro™, from which usability survey results, observations, and lessons learned were then obtained. Vital signs measurements (e.g., electrocardiogram, non-invasive blood pressure, pulse oximetry, and temperature) and medical imaging (e.g., mid-range camera, laryngoscope, and ultrasound), which are all integrated into the device, were demonstrated as part of the study. The Tempus Pro™ output was compared to known signals produced with a biomedical signal simulator (Pronk Technologies Inc., Sun Valley, CA, United States of America) before and after exposure to the alternate atmosphere within the chamber, with nominal results in each case. The participants’ observations made during the training, procedures, and scenarios of this demonstration were collected with user experience surveys and will be used in future demonstration planning and ultimately to aid exploration medical system design. Additionally, a future ISS demonstration of the Tempus Pro™ is currently being planned collaboratively between the European Space Agency (ESA) and NASA.
The LifeBot 10® (LifeBot Health, Chicago, IL, United States of America) is a MIM device with similar capabilities and functions as the Tempus Pro™, with less product maturity but with a more open platform, which presents opportunities for customization, frequent updates, and extending capabilities (Figure 6). The LifeBot 10® was also tested within the HESTIA hypobaric chamber (Marmolejo and Ewert, 2016), where the following measurement functionalities were demonstrated: electrocardiography, non-invasive blood pressure, pulse oximetry, digital stethoscope, dermatoscope, and ultrasound imaging. The demonstrations performed in the isolated atmospheric chamber provided insight into how effectively the device performs in this operational environment (e.g., The demonstration answered questions such as, is it easy to use? Does it cause the patient discomfort? Can adequate procedural support be provided?).
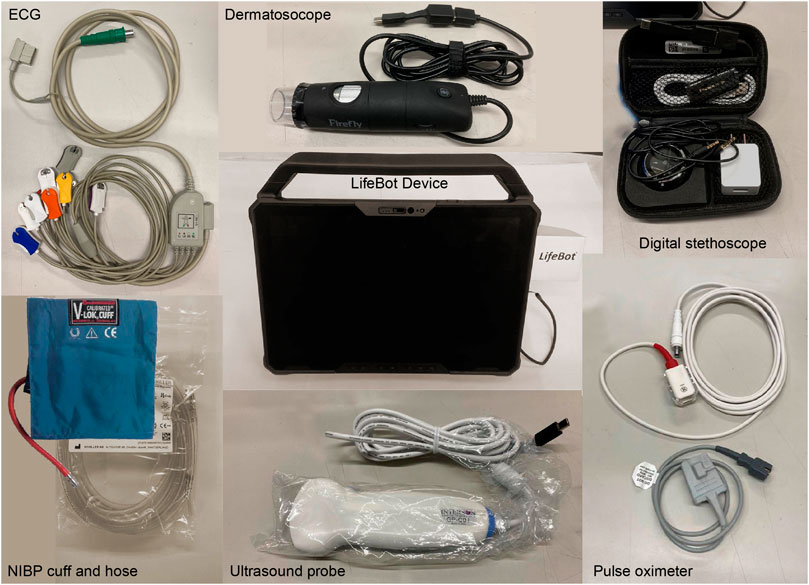
Figure 6. LifeBot 10® Hardware used in demonstrations within the JSC HESTIA Hypobaric Chamber and in preparation for future ISS demonstrations.
NASA is currently in the process of comparing and contrasting the Tempus Pro™ and LifeBot 10® devices within a ground-based study, with a goal of providing requirements and recommendations for MIM devices in the context of future exploration medical systems. This study will also introduce communications latencies and determine whether a MIM device can aid the efficiency of medical diagnosis and treatment under varying communication latency scenarios. The ability of these devices to integrate with electronic medical records, such as to the Exploration Electronic Health Record (xEHR), or to an integrated data architecture will also be evaluated.
3.1.3 Intravenous fluid administration
For medical care in LEO, pre-filled bags of IV fluid are supplied and stocked in the ISS medical kit. During missions with increased distances from Earth, medical operations cannot rely only on pre-supplied IV fluid, since terrestrial IV fluids have a shelf life of approximately 16 months from the date of manufacture, likely resulting in expired fluids before the mission is complete. In 2010, the ExMC Element of NASA’s HRP funded ZIN Technologies, Inc. (Cleveland, OH, United States of America), to develop the Intravenous Fluid Generation (IVGen) system. IV fluids were successfully generated with IVGen, using the potable water supply on ISS, during a demonstration on ISS Expedition 23 (Figure 7). The original IVGen system was comprised of an accumulator, a purification assembly which included the deionizing resin cartridge, air removal filters, and the instrumentation, and the mixing assembly which contained the final collection bag. The on-orbit system performance was monitored by cameras, pressure transducers, thermocouples, conductivity sensors, and a flow meter.
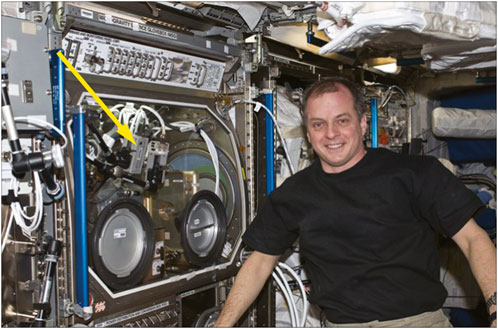
Figure 7. IVGen hardware on ISS in the Microgravity Science Glovebox (MSG) with astronaut Timothy Creamer during Expedition 23. The arrow points to the IVGen hardware (Photo credit: NASA).
The overall objective of the IVGen experiment was to verify production of sterile injectable fluids, using the ISS Water Processing Assembly’s potable water (Straub et al., 2011), mixed with United States Pharmacopeia (USP) standard grade Sodium Chloride (NaCl), to produce a 0.9% normal saline solution. Two 1.5-L bags of sterile IV fluids were generated on-orbit and returned to Earth and tested to USP standards by a certified laboratory. Eight tests were conducted on the IVGen-generated IV fluid: concentration of sterile NaCl for injection, endotoxin concentration, heavy metal concentration, identification of sodium and chloride, concentration of iron, particulate matter analysis, sterility, and pH testing. The test results indicated that all success criteria were met with the exception of the salt concentration measurement. This was due to the presence of a large air bubble in one bag and an inadequate amount of pre-measured salt in another bag. The test results included less than 0.005 endotoxin units per milliliter (mL), not more than 0.001% heavy metals, not more than 2 parts per million of iron, less than or equal to 25 particles per mL of particles larger than 10 μm (μm), and less than or equal to 3 particles per mL of particles larger than 25 μm. Sterility was assessed through the demonstration of no growth, the samples responded to tests for sodium and chloride, and had a pH of 5.4 (McQuillen, et al., 2011).
NASA’s XMIPT is currently in the process of developing a miniaturized version of the original IVGen hardware and plans to demonstrate its operation aboard ISS to increase the system’s overall TRL (Figure 8). IV fluids support the treatment of around 30% of the most likely to occur in-flight medical conditions (Ray, et al., 2022), and an in-situ capability of providing medical grade IV fluids reduces the overall mass and volume of an exploration medical system, ensuring the crew will have continued access to fluids within their shelf life. Currently, the system mixes pre-measured NaCl with the sterile water that is generated. If there were a need for other solutions such as Lactated Ringer’s or other electrolytes, IV fluid bags with those substances could also be part of the pre-supply and mixed with the generated sterile water when needed in-mission. Any in-flight attempts to add the mixing substances into the IV fluid bags would require additional controls for minimizing the introduction of air and non-sterile contaminants. Compared to the original IVGen design, current development efforts are focused on reducing system mass and volume, eliminating the need for gaseous nitrogen, moving from an active mixing mechanism to passive mixing, and refining the filter design to reduce the risk of air entering the IV bag. By increasing fluid production rates and adding process automations, the new design also optimizes operability and should reduce crew time and interaction. Additional future development efforts to mature the system include increasing portability by enabling the ability to operate on battery power and radiation hardening of components. The IVGen Mini project plans to test to the same USP standards and will also include testing for total organic carbon.
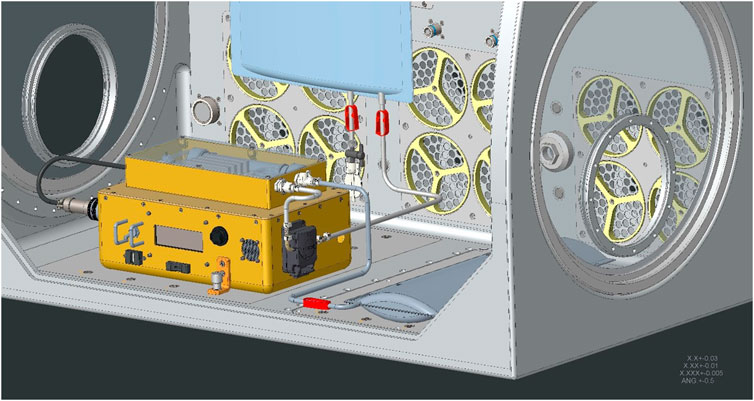
Figure 8. Concept drawing of the IVGen Mini prototype, graphically shown within the ISS Microgravity Science Glovebox.
3.1.4 Medical oxygen concentrator and administration
Delivery of supplemental medical oxygen is a desirable treatment capability for several medical conditions which could occur during exploration missions. Using an oxygen concentrator to pull oxygen out of the ambient environment instead of adding oxygen from reserve tanks can improve resource optimization and reduce the fire hazard associated with high oxygen concentration within the space vehicle. The goal of the Oxygen Concentrator Module (OCM) development effort was to provide supplemental oxygen in exploration vehicles while decreasing the burden on vehicle resources and the fire risk to crew members. Medical Oxygen Patient Interface (MOPI) was an extension of the OCM project, which focused on defining an oxygen interface for various medical treatments. Ground testing of hardware developed by TDA Research Inc. (Wheat Ridge, CO, United States of America) was completed from November 2018 thru May 2019 (Figure 9). The ground testing focused on requirement evaluation, atmosphere testing, flow testing, operational time testing, and power testing. Overall, the testing found the technology to have merit for spaceflight applications; however, the system version tested at the time did not have a self-calibrating oxygen sensor, causing significant drift in the measurements which needs to be addressed in future designs. Technologies that do not enrich the cabin concentration of oxygen through management of oxygen molecules already present in the cabin atmosphere remain a desirable trait for small, enclosed spacecraft. NASA has continued oxygen concentrator technology development through SBIR contracts and evaluation of COTS oxygen concentrator devices for potential uses within future missions. However, oxygen tanks will be used as the solution in near term Artemis missions.
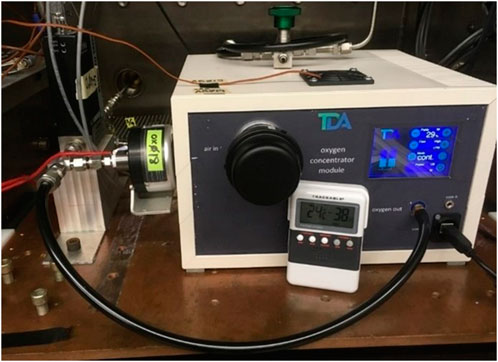
Figure 9. Test setup in a NASA Glenn Research Center Test Chamber of the TDA Research, Inc. (Wheat Ridge, CO, United States of America) low power oxygen concentrator module.
3.2 In-situ sample storage, processing, and analysis
Technologies to store, process, and analyze a variety of biological and non-biological samples in-situ are needed to enable Earth independent human research and medical operations during exploration missions with limited sample return capability.
3.2.1 Blood analysis
NASA’s Portable Clinical Blood Analyzer (PCBA) project tested a commercially available blood analyzer, the i-STAT™ (Abbott Laboratories, Abbott Park, IL, United States of America), during shuttle missions in the mid-1990s (Smith, et al., 1997), and after successful demonstrations, it was transitioned into medical operations on the ISS (Figure 10). The i-STAT™ is a hand-held blood analyzer which utilizes a cartridge-based design to measure a variety of analytes. Sample collection is performed using a capillary, or fingerstick, blood draw and then an i-STAT™ EC6+ Cartridge (Abbott Laboratories, Abbott Park, IL, United States of America) is used to analyze sodium, potassium, glucose, ionized calcium, pH, and hematocrit levels. The analyzer can provide results in less than 2 min.
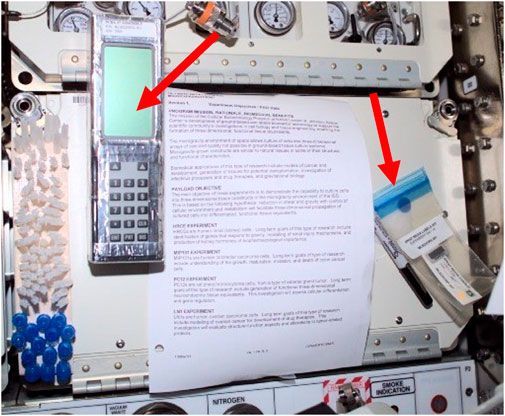
Figure 10. View of a Fixative Label Kit and the Portable Clinical Blood Analyzer (PCBA) equipment in the U.S. Laboratory/Destiny Module taken during ISS Expedition 3. The arrows point to the PCBA and the label kit (Photo credit: NASA).
The PCBA has many desirable characteristics that meet the requirements for an exploration compact laboratory analysis device. It requires a very small biological sample volume, the comprehensive suite of cartridges can perform a large variety of panels (clinical chemistry, hematology, blood gasses, etc.), it has a relatively small mass and volume, it is portable, and operates on battery power. However, this device in its current state does have some limitations and does not meet all the needs for exploration missions. For example, the cartridge shelf-life is limited to 6-months and requires refrigerated storage to maintain accuracy. While the device performs many of the required medical analyses, it does not perform all that are desired, for example, it does not have the capability to measure liver or thyroid analytes. The device also requires regular software updates and a validation access code which could be challenging in communication limited scenarios, and if not installed could render the device unusable.
White Blood Cell (WBC) count with 5-part differentials are desirable analytes to have available on future space exploration missions, as they are among the most common clinical biomarkers. The management of several in-flight medical conditions, such as bacterial and viral infections and acute radiation syndrome, becomes possible through the ability to assess condition severity and to track treatment responses based on changes in WBC counts. The HemoCue® WBC DIFF analyzer (HemoCue, Brea, CA, United States of America) is a COTS device that was selected to measure the five WBC types during ISS technology demonstrations in December 2020 and January 2021 (Figure 11). Three control solutions containing known levels of low, normal, and high concentrations of WBCs were wicked into the cavity of a cuvette and inserted into the analyzer, which used optical measurements to count the cells. The goal of this demonstration was to compare test results obtained on the ground to tests performed on ISS. The result comparisons provided evidence that the HemoCue® WBC DIFF analyzer is able to produce accurate results when operated in a microgravity environment. This HemoCue® WBC DIFF analyzer technology demonstration marked the first time that hematology measurements have been performed real-time in microgravity (Crucian, Valentine, et al., 2021).
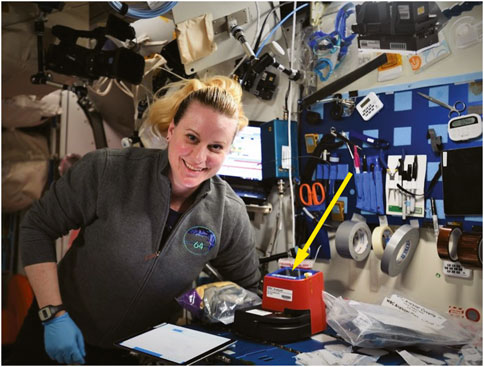
Figure 11. ISS Increment 64 Flight Engineer Kate Rubins is shown with the HemoCue® device in the Maintenance Work Area of ISS Harmony Module in January 2021. The arrow points to the HemoCue® device (Photo Credit: NASA).
Laboratory analysis uses wet and dry chemicals susceptible to mixing and bubble formation problems in microgravity, as well as potential color changes and oxidation (especially in reduced pressure environments where oxygen concentration is increased). HemoCue® is a solid assembly, robustly aligned and needed little in the way of modification to make it spaceflight ready. However, it did rely on optical imaging of a cuvette space where a dry chemical had to be mixed with the blood sample to lyse and dye the cells. Advanced quality control programming was utilized to reject images not matching color, bubble, distribution, and cell shape criteria. Whether the solutions in the cuvette would mix properly, and the programming would be robust enough to recognize cells under gravity changes was an unknown until it was successfully proven on ISS (Crucian, Valentine, et al., 2021).
NASA performed its first systematic look for portable diagnostic technologies that would meet its spaceflight needs in 2010. The results of this first blood analysis market survey (Nelson and Chait, 2010), led to the decision to demonstrate the reusable Handheld Electrolytes and Lab Technology for Humans (rHEALTH) ONE analyzer (rHEALTH, Bedford, MA, United States of America) on ISS. The rHEALTH ONE analyzer uses flow cytometry methodology and the commercial standard sheath-based hydrodynamic focusing. Several modifications were necessary to transform the terrestrial fluid management system into one that would function in microgravity. The most significant modification was the addition of medical balloons inside the bottles to contain the sheath, cleaning, and waste fluids, while still permitting functionality in microgravity. The analyzer, containing two lasers and five detectors, was tested using four control samples of varying types for spectral overlap, particle size, linearity, and optical precision. The rHEALTH ONE was operated during a technology demonstration on ISS in May 2022 (Figure 12) to show that test results obtained in flight matched ground results. The favorable comparison provided evidence that hydrodynamic focusing and sheath-based flow cytometry can be accomplished in microgravity with the rHEALTH ONE analyzer, and further redesign and modifications within the planned next-generation devices will strive to augment and improve performance (Rea, et al., 2024).
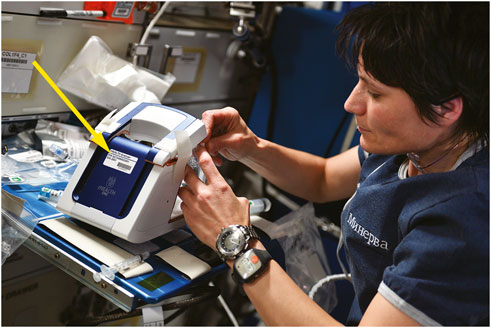
Figure 12. European Space Agency Astronaut Samantha Cristoforetti operates the rHEALTH ONE analyzer on the ISS in May 2022. The arrow points to the rHEALTH One analyzer (Photo Credit: NASA).
As distance from Earth increases for future exploration missions, there will be a need to collect, process, and analyze clinical samples in flight which differs from the current operational paradigm on ISS, where there is a reliance on returning blood and biological samples to the ground for terrestrial analysis. Additionally, there is expected to be limited to no refrigerated storage ability. NASA’s XMIPT performed a new market survey in 2023 to identify existing and in-development laboratory analysis devices using updated evaluation criteria, reflecting NASA’s evolving requirements for use on future long duration spaceflight missions. The desired capabilities of a point-of-care laboratory analyzer include the ability to perform analyses of the targeted analytes provided in Table 1 for medical diagnosis. Additional desirable capabilities include compact low mass and volume devices, low sample volume requirements (e.g., via a fingerstick) or non-invasive collection, minimal operational maintenance, minimal reliance on cloud services, the ability to store and integrate results into a centralized medical data system (e.g., via electronic health records), FDA or equivalent approval, and hardware ruggedization for survival within austere environments.
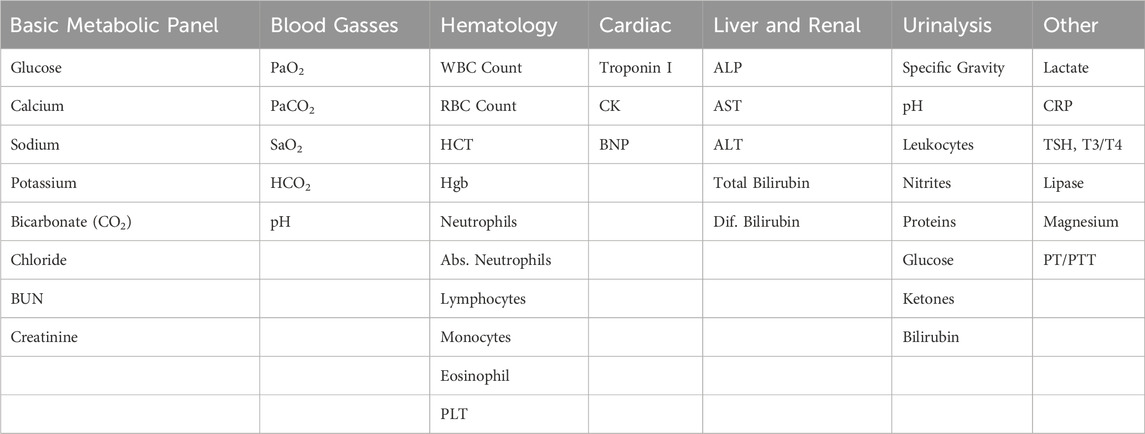
Table 1. Lists of highly desired targeted analytes for use in medical diagnoses. These lists continue to evolve as more is learned about exploration medical system requirements.
The market survey identified two dozen potential devices of interest. The highest-ranking devices were highly integrated analyzers capable of combining multiple analysis techniques like hematology, clinical chemistry, and immunoassay into one device. Some devices were FDA-approved, but several are still emerging technologies. All the solutions identified are expected to need various levels of further development to successfully operate in a spaceflight or microgravity environment. Challenging tradeoffs will need to be evaluated between developing a singular highly complex, highly integrated device that meets all of the requirements for exploration or identifying a combination of smaller more discrete devices that when combined meet the total needs for exploration. It also remains to be seen whether NASA’s requirements will be able to be met with COTS devices, or whether customized development for spaceflight use will be necessary. While the need for an in-situ laboratory analysis capability is driven largely by medical operational need to reduce overall in-flight medical risk, collaborative efforts are also underway to identify research specific analytes and, where possible, identify technologies that meet the need of both operations and research.
3.3 Operational medical decision support software and informatics
In-flight medical decision support software that guides the crew through diagnosis and treatment processes, as well as medical informatics such as electronic health records, inventory tracking capability, and data analysis that is integrated within the vehicle’s data architecture, is needed for enabling data-driven medical decision making during increasingly Earth-independent operations.
3.3.1 Resource tracking
Currently, NASA relies mainly on crew self-reporting to manage and maintain the medical inventory aboard the ISS. When medical equipment or medications are used in flight, crew members notify the ground operations team who update the terrestrial inventory database. As time goes by, the ground database may become less accurate due to failures to report all inventory utilization. The ability to maintain an accurate inventory becomes more critical for future long duration missions, since the crew will need to become more autonomous when quickly finding and utilizing medical items or when evaluating alternative treatments where limited or no resupply opportunities exist.
The Medical Consumables Tracking (MCT) project provided a demonstration of real-time tracking of medical supplies aboard the ISS. The MCT system design utilized Radio Frequency Identification Device (RFID) technology and was comprised of a reader, interrogator, scanner, antennas, a transponder, and a single-board computer all operating on battery power. Prior to demonstration, RFID tags were attached to medical consumables stored in the Convenience Medication Pack, which consists mostly of oral and topical pharmaceuticals. The pack contained roughly 280 tagged items and was pre-supplied to the ISS for use in the MCT flight demonstration with the intent of demonstrating that the system could correctly identify when a medical item was removed from the kit and securely transmit data to the ground.
The MCT system was demonstrated aboard the ISS between December 2016 and July 2018 (Figure 13). The system was installed in the Crew Healthcare System (CHeCS) Resupply Stowage Rack (RSR) and designed to perform an automated inventory cycle every 30 days. A switch was also located on the exterior of the CHeCS resource supply rack locker door, which would allow crew to manually initiate an inventory cycle. During the time MCT was installed, it collected data from the Medical Convenience Pack and completed over 130 scans in various testing configurations. When a scan cycle was completed, an inventory report of RFID tagged items detected within the locker were transmitted wirelessly to the Joint Station Local Area Network and downloaded to mission support. Overall, the demonstration was successful, with a 95% identification accuracy of the removed items. The inaccuracy resulted from one anomalous tag read, which caused one missing scan within the results. The engineering team theorized that the anomaly was due to radiation damage of some of the electronic parts. The engineering team captured lessons learned around inventory tag placement, optimizations to antenna design and med pack placement, and documented areas for design enhancements (Zoldak, 2018).
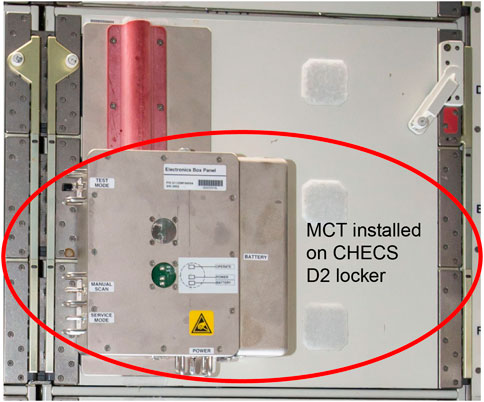
Figure 13. Medical Consumables Tracking (MCT) installed in the Crew Healthcare System (CHeCS) locker on ISS. The MCT system is circled within the photo (Photo Credit: NASA).
NASA’s XMIPT is currently in the process of designing the Automated Medical Inventory System (AMIS), which takes advantage of advancements in inventory tracking technologies while leveraging the lessons learned from MCT. AMIS is also planned to expand beyond medication tracking and may include other durables including treatment aids and diagnostic equipment as well as medical consumables. The goal of the new system is to automate the recording of the medical inventory across multiple vehicles and habitats during exploration space flight and reduce the manual effort associated with ground and in-flight medical inventory tracking to foster more Earth-independent medical operations.
3.3.2 Procedural guidance
Successful performance of medical procedures during missions beyond LEO requires novel solutions to replace real-time support from the ground since communication latencies will be longer as the crew travels farther from Earth. The Autonomous Medical Officer Support (AMOS) Software Technology Demonstration series (AMOS Tech Demo) on the International Space Station (ISS) is the initial trial of a novel software tool that shifts the emphasis from preflight training and real-time guidance, the current ISS paradigm, to in-flight JIT instruction, a new paradigm for crew medical autonomy (Figure 14). The initial autonomous guidance concepts and software design for this tool were developed and validated by the multi-disciplinary team of the ground-based Clinical Outcome Metrics for Optimization of Robust Training (COMfORT) study and were subsequently refined to produce the AMOS in-flight operational version. The COMfORT/AMOS platform introduces a novel, streamlined skill management concept for exploration missions; AMOS software in its current version features comprehensive training and guidance modules for urinary bladder and kidney ultrasound examinations.
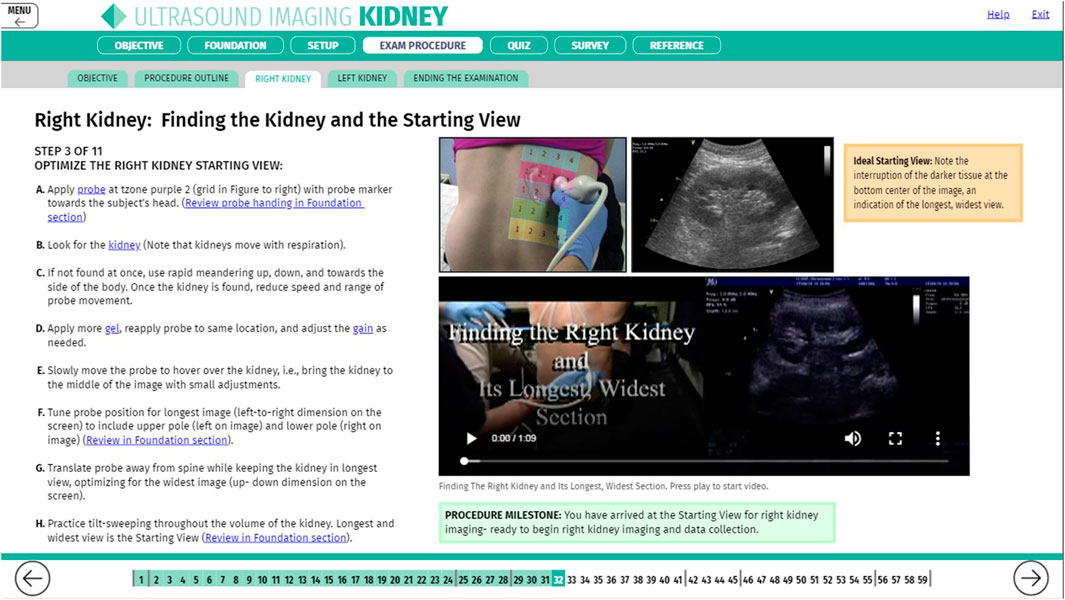
Figure 14. Screen capture of the Autonomous Medical Officer Support (AMOS) software depicting instructions for ultrasound imaging of the kidney.
Current ISS medical operations rely heavily on preflight training and real-time remote guidance, both of which become impractical or impossible in missions beyond LEO. Such missions will have limited medical evacuation options which also necessitates heavier dependence on the quality and overall success of in-flight medical procedures. Even if medical procedural skills and knowledge are mastered by exploration crew during the preflight period, these abilities may not be retained throughout the mission given the extended time lapse between training and use of these skills, and the myriad of potential medical situations preclude proper training for all scenarios. In contrast to the current practice of creating and using separate products for 1) preflight training, 2) in-flight JIT training, and 3) actual procedures, the AMOS platform is a single skill management tool for all mission phases, covering all training aspects as well as procedure execution. This novel all-encompassing approach effectively and efficiently consolidates skill management processes and maximizes both pre-trained and untrained performance.
The primary goal of the AMOS Tech Demo series is to confirm telemedical proof-of-concept through initial use of the platform for autonomous imaging activities in an operational setting. Success metrics encompassed 1) successful software deployment, 2) collection of click tracking data, and 3) recording of ultrasound images. Additional aims included collecting crew feedback on AMOS implementation and integrated training and procedure support concepts, as well as crew and team evaluation of AMOS use in an operational setting. Two ISS crewmembers participate in each in-flight Tech Demo; the Operator uses AMOS to perform ultrasound under software guidance, and the Subject serves as the “patient” for the ultrasound scans of the full bladder, empty bladder, right kidney, and left kidney. Ultrasound images are collected using the ISS Ultrasound 2, a Vivid-q™ (General Electric Healthcare, Chicago, IL, United States of America) device modified for spaceflight, with a 4C-RS broadband curved array transducer (General Electric Healthcare, Chicago, IL, United States of America). Imaging instances (cine-loops and still frames) were rated using a predefined, multi-component rubric which included elements such as target organ inclusion, sweep speed, average image quality, measurability, and effective content (clinical utility). Based on these parameters and the overall impression of the image or image series, the imaging task was assigned a success score (0–3; 0 = no clinical utility, 3 = optimal image quality, with a rating of 2 or higher considered clinically and operationally acceptable). The image or series was deemed successful if all targets scored a 2 or better. Crewmembers receive no preflight AMOS training and have no prior exposure to the AMOS software. AMOS tracks operator use and incorporates a user experience survey within each module.
As of the submission of this manuscript, two instances of the Tech Demo have been performed on the ISS. First use of the AMOS software demonstrated successful installation and deployment of the platform in the ISS server environment, and both Demos have resulted in highly positive user experience and successful ultrasound image collection of the kidney and urinary bladder. Both demonstrations were conducted in full crew autonomy with no assistance or interference from the ground. Lessons learned during the Tech Demos have allowed the team to identify areas within the procedures that require additional clarification, cautionary statements, or troubleshooting steps, and have also highlighted the importance of simple and intuitive crew interfaces. Additional AMOS Tech Demos are planned, which should include Operator/Subject teams of varied backgrounds to fully validate the platform. Success of these Tech Demos signifies the beginning of a shift from the existing model of preflight training with modest retention of conceptual and procedural knowledge (non-permanent skills), to a new paradigm of preflight familiarization with an in-flight JIT platform, which can be used as necessary to bolster effective skills for in-flight needs. While these Demos are specifically focused on medical applications, AMOS is a platform technology that can be applied to any discipline. The AMOS platform provides training and in-mission support using a single training and procedure instrument for all phases of skill management including acquisition, retention, and application. This approach also streamlines the training process, provides a more consistent experience for the user, and allows flexibility for JIT training of tasks throughout any mission with no prior task instruction.
3.3.3 Electronic health records
The NASA Office of the Chief Health and Medical Officer has implemented an Electronic Health Record (EHR) system to ensure a healthy workforce and working environment at NASA. NASA’s EHR system is used agency-wide but is at the forefront of astronaut health and fitness for duty tracking. NASA standards levy the requirement that all space missions provide a medical system infrastructure within which astronaut medical records are maintained (NASA-STD-3001 2023). Currently, NASA’s EHR system is intended only for ground use and is not accessible to in-mission ISS crew for medical decision support and communication. An in-flight EHR-like capability of providing the crew with health data, medical store-and-forward communications, and necessary medical administrative tools is critical for enabling NASA’s standard of healthcare during increasingly autonomous operations.
The XMIPT project called Medical Exploration Development and Implementation Scoping (MEDIScope) developed and reviewed objectives and concept of operations for an Exploration Electronic Health Record (xEHR) with project stakeholders, developed preliminary high-level requirements, and coordinated the handoff of the project to an engineering design and implementation team at NASA JSC. This team will develop system requirements for the xEHR with inputs from subject matter experts. Following final requirements development, a team will be chosen to develop the xEHR and to integrate with a NASA in house integrated data architecture project so that xEHR data can be used within advanced analytics to inform decision making within medical decision support tools. Ultimately, the xEHR will then be validated through demonstrations on future space exploration platforms such as within a Lunar orbital habitat.
3.4 Modifications, customizations, and challenges to overcome for medical device use in the space environment
The spaceflight environment differs greatly from terrestrial norms. Many terrestrial designs work with the assistance of gravity or actively against it, which may lead to undercompensation or overcompensation of designs when gravity is removed. Fluids can be particularly challenging to work with in microgravity due to difficulties such as unwanted bubble formation and improper mixing of various solutions. Even simple actions such as setting down materials or cleaning up a spill become much more complex when these items are no longer constrained by gravity, but instead can become dominated by surface tension, get lost behind panels, or come in contact with other crewmembers. In isolated and tightly enclosed microgravity spaces, components must remain fastened or tethered, liquids, powders, and gases safely contained, and interference with other onboard systems (including the human body) minimized. Common conveniences in any house, laboratory, or hospital such as ample space, open containers, fresh air, natural convection, refrigeration, and unlimited access to utilities, consumables, and waste disposal, are limited or nonexistent in space habitats and vehicles. Care must be taken not to pollute the habitat environment from foreign substances, such as celestial dust acquired outside the vehicle, or even from the byproducts of cleaning supplies used to fight contaminants. The vehicle’s ECLSS is a closed system and the water, atmospheric, and waste systems must be capable of accommodating anything that is introduced into the environment during the mission. Anything off-gassing from the devices or any toxic or hazardous by-products need to be planned for and appropriately managed by all of the ECLSS systems as the mission progresses. The non-physical, wireless connectivity space also needs to be considered. It can also be crowded, with signals and communications from competing vital systems needing to be managed while minimizing any noise that is present on the electromagnetic spectrum.
All devices used in-mission must maintain their calibration under the conditions described above. The devices also need to maintain good working order throughout the long duration missions, as there is typically minimal or no stowage available for maintenance items or replacements, although this could change as advances in additive manufacturing allows 3-D printing of spare parts and consumables in space (Sacco and Moon, 2019). High velocity particles from cosmic radiation can penetrate image sensors and reduce the quality of the images they produce. Radiation also has the potential to damage complex circuitry, corrupt software, and impair electronic functionality. Therefore, radiation hardened electronic components that are resistant to damage or malfunction due to radiation exposure must be used within devices that contain these types of components. Increased radiation exposure can also accelerate the degradation of mission resources, making alternate or additional packing to protect from radiation a consideration. Radiation can further reduce resource shelf-life, which is already a challenge since shelf-life requirements of mission resources are often greater than the typical terrestrial shelf-life, which are commonly designed to be less than 2 years.
The medical devices must also accommodate biological changes. The organs and fluids of the human body are more free-floating when in microgravity and will not always be in their textbook positions or shapes, which may confound image interpretation in some cases. As the human body adapts to the various gravitational environments, standard terrestrial references may not be completely accurate. For example, if an algorithm is trained using terrestrial data where fluid pools in a gravity-dependent manner, is the algorithm still accurate for a micro-gravity environment? Spaceflight technology will need to be flexible enough to allow a new “normal” to be set. Cells and substructures can also change shape in response to gravity changes. These impediments may cause the imaging optics and software of the analysis devices to improperly recognize the cells or to count them incorrectly. This could lead to incorrect conclusions about the health status of a crewmember when the result actually stems from the inability of the device to accommodate physical and cellular changes due to microgravity.
Prior to facing the challenges of the space environment, devices must first withstand the vibrations and gravity forces experienced during their launch into space. Protection against acoustically driven vibrations within space habitats and vibrations experienced during transport to and from a celestial body is also necessary. Devices are screened through various tests, analyses, and hazard assessments, using testing standards such as those described in (Jang and Park, 2020). As emissions, stored energy, and materials are identified and weighed against allowable limits, modifications are made to ensure safe transport and function. Methods like conformal coating and stacking cantilevered components are common practice. Kapton® tape (DuPont de Nemours, Inc., Wilmington, DE, United States of America) and other types of metal shielding provide electrical insulation, flammability precautions, and electromagnetic interference protection. Unconditioned areas of space vehicles can experience drastic changes in temperature and pressure, which may cause components and containers to expand and burst if not given appropriate consideration. Thus, many fluid assemblies are specifically designed with rigid containers in addition to underfilling to allow for excess gas volume. The risks that remain are addressed with mitigation strategies that are incorporated into the operational procedures created during the planning process.
4 Discussion
This paper provides information about the solutions to medical conditions LEO astronauts currently experience and the conditions with a likelihood of occurrence during exploration missions to the Moon and Mars. The ISS medical system has been robust enough to manage the spaceflight medical events that have occurred; however, challenges exist as the medical systems for future exploration missions are designed. Challenges exist due to the mass, volume, and power constraints associated with the small size of the exploration space vehicles and with the long distances the missions will travel from Earth. The subset of NASA Human System Capability Gaps highlighted within this paper define some of the advancements in technology that are necessary for building an exploration medical system suitable for mitigating the spaceflight medical risk. These gaps include Medical Imaging, Diagnostics, and Treatment Technologies, In-situ Sample Storage, Processing, and Analysis, and Operational Medical Decision Support Software and Informatics. Examples of technologies and on-going NASA development, modification, and demonstration efforts aimed at closing each of these gap areas was provided within this review.
The terrestrial market for medical devices is significantly larger, by many orders of magnitude, than the customer pool for spaceflight-ready medical devices. Therefore, there are significant opportunities to leverage technology from academia or products from the commercial sector. Existing technology and equipment can be evaluated for how well it suits the spaceflight needs and whether it will function properly, safely, and accurately in a space environment. Sometimes devices will function correctly in space as is, but often there is a need to modify the terrestrial design to make it spaceflight ready. Stakeholders of spaceflight medical devices may also work to influence the design of Earth-based capabilities to enable a dual use in space. If these steps still do not result in spaceflight ready capabilities, then NASA and its international partners can look to perform in-house development of unique spaceflight technologies. Similarly, given the challenges and expense associated with performing technology demonstrations on the ISS, demonstrations in less expensive spaceflight analogs or on reduced gravity, sub-orbital, or commercial partner orbital spaceflights need to be considered as alternate demonstration platforms. While it is necessary to use the spaceflight environment, such as ISS, to reach the highest spaceflight ready TRL levels, appropriately leveraging analog platforms allows some design questions to be resolved in a less burdensome manner.
NASA currently relies heavily on market surveys and simplified trade studies to determine medical device research priorities. In the future, the IMPACT tool, mentioned above as the evidence-based tool designed to assess medical risk and aid medical system design for exploration-class missions, could be used to prioritize which capabilities and resources buy down the most medical risk associated with the exploration missions. This will enable a quantitative way for deciding which technologies to further evaluate, demonstrate, miniaturize, or develop. As an example, only three of the Human System Capability Gaps were addressed in this paper. There are other key areas of interest which also need to be investigated and invested in, such as the Crew Health and Performance Integrated Data Architecture Gap, the Integrated Medical Simulation Technologies Gap, and the Semi-autonomous Behavioral Health and Performance Technologies Gap (Thompson, et al., 2023). In addition to the state-of-the-art technology available today, new technologies will emerge and technologies now in their infancy will mature to be spaceflight ready as the future exploration missions come closer to occurring. Technologies and methods such as machine learning, artificial intelligence, virtual and augmented reality, and holographic technology are examples of what will likely be incorporated into exploration medical technology, particularly for use in procedural guidance, JIT training, and clinical decision support (Ebnali, et al., 2022). Additionally, methods for performing medical procedures not currently considered on ISS because of the short evacuation time from LEO, such as the ability to perform surgeries in space (Hayden, et al., 2013), will also need to be considered.
Technology development, modification, and demonstration of individual capabilities is necessary but not sufficient for a functional exploration medical system. These capabilities need to be integrated together into a medical system that is then integrated into a comprehensive CHP system. Then this CHP system needs to be interfaced with other vehicle systems to ensure interoperability, efficiency, and effectiveness, resulting in a system of systems within the vehicle. Once the device components of the medical system are defined and become spaceflight ready, then it will be desirable to demonstrate the devices within an integrated medical system that includes data storage and analysis capabilities, procedural guidance and training features and clinical decision support. The ultimate goal of exploration mission preparation will then be demonstration of a functioning integrated CHP system, complete with, for example, environmental monitoring sensors, physiological and psychological countermeasure equipment, performance monitoring sensors, in addition to an optimal medical system, and all tied together by an integrated data architecture.
NASA establishes collaborations with academia, small businesses, larger industries, other federal agencies including the military, and international partners when interests in medical device development align. This helps to ensure realized technology developments can provide benefits not only in space but also on Earth. In some cases, the challenges of accurate functionality in remote, austere space environments overlap with the military’s needs for battlefield medical capabilities. In addition, medical device ease of use and telemedicine capabilities are synergistic with Earth-based home medical monitoring and telemedicine applications. Finally, portable, low power consuming technologies overlap with the medical capability needs within developing nations.
In conclusion, this paper has provided details about the NASA approach to buying down risk for the various medical conditions that need to be managed in space, and the challenges that need to be overcome before realizing effective medical systems for Lunar and Martian missions. Several of NASA’s on-going medical technology developments and evaluations have been described. These include modifications needed for proper function in the space environment and the results of capability evaluations performed in space or within Earth-based space analog platforms. These are efforts on the long roadmap to realizing missions to the Moon and Mars where medical risk outcomes are successfully managed. By addressing these technology gaps, with technology that is currently available or with state-of-the-art advancement, we can minimize overall medical risk to the crew and increase the likelihood of safe and successful space exploration missions.
Author contributions
BL: Conceptualization, Data curation, Formal Analysis, Investigation, Methodology, Validation, Visualization, Writing–original draft, Writing–review and editing. CS: Conceptualization, Funding acquisition, Project administration, Resources, Writing–original draft, Writing–review and editing. RM: Conceptualization, Data curation, Formal Analysis, Investigation, Methodology, Validation, Visualization, Writing–original draft. RV: Conceptualization, Data curation, Formal Analysis, Investigation, Methodology, Visualization, Writing–original draft. KC: Conceptualization, Funding acquisition, Project administration, Resources, Writing–original draft. JY: Conceptualization, Data curation, Formal Analysis, Investigation, Methodology, Validation, Visualization, Writing–original draft. DE: Conceptualization, Data curation, Formal Analysis, Investigation, Methodology, Software, Validation, Visualization, Writing–original draft, Writing–review and editing. AS: Conceptualization, Funding acquisition, Project administration, Resources, Writing–original draft. VB: Conceptualization, Data curation, Formal Analysis, Investigation, Methodology, Software, Validation, Visualization, Writing–original draft. MW: Conceptualization, Data curation, Formal Analysis, Investigation, Methodology, Software, Validation, Visualization, Writing–original draft. JL: Conceptualization, Funding acquisition, Project administration, Writing–review and editing. RS: Conceptualization, Funding acquisition, Project administration, Writing–review and editing. MT: Conceptualization, Funding acquisition, Project administration, Writing–review and editing. BE: Conceptualization, Funding acquisition, Project administration, Writing–review and editing. KL: Conceptualization, Funding acquisition, Project administration, Writing–review and editing.
Funding
The author(s) declare that financial support was received for the research, authorship, and/or publication of this article. Funding for the projects and technologies described in this manuscript and for the labor necessary to write the manuscript was provided by the Exploration Medical Capability Element of the NASA Human Research Program and by the Exploration Medical Integrated Product Team of the NASA Mars Campaign Office.
Acknowledgments
These successful technology developments and demonstrations required significant work from teams at NASA, NASA contractors, and commercial companies. Without their past effort, this manuscript would not have been possible.
Conflict of interest
Authors RM, JY, DE, AS, VB were employed by the KBR. Authors RV and KC were employed by ZIN Technologies, Inc.
The remaining authors declare that the research was conducted in the absence of any commercial or financial relationships that could be construed as a potential conflict of interest.
Publisher’s note
All claims expressed in this article are solely those of the authors and do not necessarily represent those of their affiliated organizations, or those of the publisher, the editors and the reviewers. Any product that may be evaluated in this article, or claim that may be made by its manufacturer, is not guaranteed or endorsed by the publisher.
References
Abercromby, A., Douglas, G., Kalogera, K., Somers, J., Suresh, R., Thompson, M., et al. (2022). “NASA crew health and performance capability development for exploration: 2021 to 2022 overview,” in 51st international conference on environmental systems.
Antonsen, E. L., Connell, E., Wilma, A., Reynolds, R. J., Buckland, D. M., and Van Baalen, M. (2023). Updates to the NASA human system risk management process for space exploration. npj Microgravity 9 (1), 72. doi:10.1038/s41526-023-00305-z
Antonsen, E. L., Myers, J. G., Boley, L., Arellano, J., Kerstman, E., Kadwa, B., et al. (2022). Estimating medical risk in human spaceflight. npj Microgravity 8 (1), 8. doi:10.1038/s41526-022-00193-9
Auñón-Chancellor, S. M., Pattarini, J. M., Moll, S., and Sargsyan, A. (2020). Venous thrombosis during spaceflight. N. Engl. J. Med. 382 (1), 89–90. doi:10.1056/nejmc1905875
Blue, R., Nusbaum, D., and Antonsen, E. (2019). Development of an accepted medical condition list for exploration medical capability scoping, NASA/TM-2019-220299. Houston, TX: NASA.
Burg, A., Boggs, K. G., Goodliff, K., McVay, E., Benjamin, G., and Elburn, D. (2021). “Architecture robustness in NASA's Moon to Mars capability development,” in IEEE aerospace conference proceedings 2021-march.
Choate, A., Harris, D., Nickens, T., Kessler, P., and Simon, M. (2023). “NASA's Moon to Mars (M2M) transit habitat refinement point of departure design,” in IEEE aerospace conference proceedings 2023-march.
Creech, S., Guidi, J., and Elburn, D. (2022). “Artemis: an overview of NASA's activities to return humans to the Moon,” in 2022 IEEE aerospace conference (AERO) (IEEE).
Cromwell, R. L., Huff, J. L., Simonsen, L. C., and Patel, Z. S. (2021). Earth-based research analogs to investigate space-based health risks. New Space 9 (4), 204–216. doi:10.1089/space.2020.0048
Crucian, B., Babiak-Vazquez, A., Johnston, S., Pierson, D. L., Mark Ott, C., and Sams, C. (2016). Incidence of clinical symptoms during long-duration orbital spaceflight. Int. J. General Med. 9, 383–391. doi:10.2147/ijgm.s114188
Crucian, B., Russell, V., Calaway, K., Miller, R., Rubins, K., Hopkins, M., et al. (2021). Spaceflight validation of technology for point-of-care monitoring of peripheral blood WBC and differential in astronauts during space missions. Life Sci. Space Res. 31, 29–33. doi:10.1016/j.lssr.2021.07.003
Doarn, C. R. (2011). Medical policy development for human spaceflight at NASA: an evolution. Aviat. Space Environ. Med. 82 (11), 1073–1077. doi:10.3357/asem.3103.2011
Doarn, C. R., Polk, J. D., Grigoriev, A., Marc Comtois, J., Shimada, K., Weerts, G., et al. (2021). A framework for multinational medical support for the international space station: a model for exploration. Aerosp. Med. Hum. Perform. 92 (2), 129–134. doi:10.3357/amhp.5771.2021
Ebnali, M., Goldsmith, A. J., Burian, B., Atamna, B., Duggan, N. M., Fischetti, C., et al. (2022). AR-coach: using augmented reality (AR) for real-time clinical guidance during medical emergencies on deep space exploration missions. Healthc. Med. Devices 51, 67–75. doi:10.54941/ahfe1002100
Garbino, A., Hew-Yang, M. Y., Estep, P., Siders, B., Dillon, L., Suri, K., et al. (2024). Development, validation and approval of a planetary extravehicular activity prebreathe protocol: NASA exploration atmosphere tests 1 & 2. Chicago, IL: Aerospace Medical Association Annual Meeting.
Gilkey, K. M., McRae, M. P., Griffin, E. A., Kalluri, A. S., and Myers, J. G. (2012). Bayesian analysis for risk assessment of selected medical events in support of the integrated medical model effort. Cleveland, OH: NASA Glenn Research Center. NASA/TP-2012-217120.
Goodliff, K. E., Merancy, N. F., Bhakta, S. S., Rucker, M. A., Rei-Po Chai, P., Ashurst, T. E., et al. (2023). Moon-to-Mars architecture definition document (ESDMD-001), NASA/TP-20230002706. Washington, DC: NASA.
Hayden, J. A., George, M. P., Burgess, J. E., and Antaki, J. F. (2013). A hermetically sealed, fluid-filled surgical enclosure for microgravity. Aviat. Space, Environ. Med. 84 (12), 1298–1303. doi:10.3357/asem.3751.2013
Jang, J., and Park, J. W. (2020). Simplified vibration PSD synthesis method for MIL-STD-810. Appl. Sci. Switz. 10 (2), 458. doi:10.3390/app10020458
Johnston, R. S., Dietlein, L. F., and Berry, C. A. (1975). Biomedical results of Apollo, NASA SP-368. Washington, DC: National Aeronautics and Space Administration.
Keenan, A., Young, M., Saile, L., Boley, L., Walton, M., Kerstman, E., et al. (2015). “The Integrated Medical Model: a probabilistic simulation model predicting in-flight medical risks,” in 45th international conference on environmental systems (Bellevue, WA: ICES), 2015–2071.
Kerstman, E. L., Scheuring, R. A., Barnes, M. G., DeKorse, T. B., and Saile, L. G. (2012). Space adaptation back pain: a retrospective study. Aviat. Space Environ. Med. 83 (1), 2–7. doi:10.3357/asem.2876.2012
Kreykes, A. J., Suresh, R., Levin, D., and Hilmers, D. C. (2023). Selecting medical conditions relevant to exploration spaceflight to create the IMPACT 1.0 medical condition list. Aerosp. Med. Hum. Perform. 94 (7), 550–557. doi:10.3357/amhp.6199.2023
Levin, D. R., Steller, J., Anderson, A., Lemery, J., Benjamin, E., Hilmers, D. C., et al. (2023). Enabling human space exploration missions through progressively Earth independent medical operations (EIMO). IEEE Open J. Eng. Med. Biol. 4, 162–167. doi:10.1109/ojemb.2023.3255513
Marmolejo, J., and Ewert, M. (2016). “Human exploration system test-bed for integration and advancement (HESTIA) support of future NASA deep-space missions,” in AIAA Houston Section annual technical symposium. Houston, TX.
McQuillen, J. B., McKay, T. L., Griffin, D. V. W., Brown, D. F., and John, T. Z. (2011). Final report for intravenous fluid generation (IVGEN) spaceflight experiment, NASA/TM-2011-217033. Cleveland, OH: NASA.
Mehta, P., and Bhayani, D. (2017). Impact of space environment on stability of medicines: challenges and prospects. J. Pharm. Biomed. Analysis 136, 111–119. doi:10.1016/j.jpba.2016.12.040
NASA JSC-CN-24908 (2011). CHeCS (crew health care systems): international space station (ISS) medical hardware catalog version 10.0. Washington, DC: National Aeronautics and Space Administration.
NASA OCHMO-TB-033 (2023). Spaceflight experience and medical care, rev D. Washington, DC: National Aeronautics and Space Administration.
NASA SP-2016-6105 (2016). NASA system engineering handbook, rev 2. Washington, DC: National Aeronautics and Space Administration.
NASA SP-20205003605 (2020). Technology readiness assessment: best practices guide. Washington, DC: National Aeronautics and Space Administration.
NASA-STD-3001 (2023). NASA space flight human-system standard volume 1, revision C: crew health. Washington, DC: National Aeronautics and Space Administration. Vol 1, Rev C.
Nelson, E. S., and Chait, A. (2010). Portable diagnostics technology assessment for space missions, Part 2: market survey, NASA/TM-2010-215845/Part 2. Cleveland, OH: NASA.
Nelson, E. S., Lewandowski, B., Licata, A., and Myers, J. G. (2009). Development and validation of a predictive bone fracture risk model for astronauts. Ann. Biomed. Eng. 37 (11), 2337–2359. doi:10.1007/s10439-009-9779-x
Nowadly, C. D., Trapp, B. D., Robinson, S. K., and Richards, J. R. (2019). Resuscitation and evacuation from low Earth orbit: a systematic review. Prehospital Disaster Med. 34 (5), 521–531. doi:10.1017/s1049023x19004734
Pohlen, M., Carroll, D., Kim Prisk, G., and Sawyer, A. J. (2022). Overview of lunar dust toxicity risk. npj Microgravity 8 (1), 55. doi:10.1038/s41526-022-00244-1
Ramachandran, V., Dalal, S., Scheuring, R. A., and Jones, J. A. (2018). Musculoskeletal injuries in astronauts: review of pre-flight, in-flight, post-flight, and extravehicular activity injuries. Curr. Pathobiol. Rep. 6 (3), 149–158. doi:10.1007/s40139-018-0172-z
Ray, K., Tyler, H., Perusek, G., and Funk, N. (2022). Intravenous fluid generation Mini (IVGEN Mini) summary and related research, NASA/TM-20220012816. Cleveland, OH.
Rea, D. J., Miller, R. S., Crucian, B. E., Valentine, R. W., Cristoforetti, S., Bearg, S. B., et al. (2024). Single drop cytometry onboard the international space station. Nat. Commun. 15, 2634. (in Press). doi:10.1038/s41467-024-46483-6
Richardson, R., and Piper, S. (2022). Crew medical officer training and role of ground support for international space station (ISS) operations. Aerosp. Med. Hum. Perform. 93 (3), 218.
Sacco, E., and Moon, S.K. (2019). Additive manufacturing for space: status and promises. Int. J. Adv. Manuf. Technol. 105 (10), 4123–4146. doi:10.1007/s00170-019-03786-z
Scheuring, R. A., Mathers, C. H., Jones, J. A., and Wear, M. L. (2009). Musculoskeletal injuries and minor trauma in space: incidence and injury mechanisms in U.S. Astronauts. Aviat. Space, Environ. Med. 80 (2), 117–124. Available at: http://openurl.ingenta.com/content/xref?genre=article&issn=0095-6562&volume=80&issue=2&spage=117. doi:10.3357/asem.2270.2009
Seyedmadani, K., Tucker, K. A., Reyna, B., Akay, Y. M., Akay, M., and Fogarty, J. A. (2023). Processes for designing innovative biomedical hardware to use in space and on Earth. IEEE Open J. Eng. Med. Biol. 4, 184–189. doi:10.1109/ojemb.2023.3270393
Smith, S. M., Davis-Street, J. E., Fontenot, T. B., and Lane, H. W. (1997). Assessment of a portable clinical blood analyzer during space flight. Clin. Chem. 43 (6), 1056–1065. doi:10.1093/clinchem/43.6.1056
Straub, J. E., Plumlee, D. K., Schultz, J. R., and Torin McCoy, J. (2011). “Chemical analysis results for potable water from ISS expeditions 21 through 25,” in 41st international conference on environmental systems 2011 (Portland, OR: ICES), 2011. doi:10.2514/6.2011-5152
Thompson, M., Lehnhardt, K., Easter, B., Lemery, J., and Suresh, R. (2023). Identifying and closing medical capability gaps for human spaceflight missions beyond low Earth orbit. Aerosp. Med. Hum. Perform. 94 (4), 237.
Walton, M. E., and Kerstman, E. L. (2020). Quantification of medical risk on the international space station using the integrated medical model. Aerosp. Med. Hum. Perform. 91 (4), 332–342. doi:10.3357/amhp.5432.2020
Watkins, P., Hughes, J., Gamage, T. V., Knoerzer, K., Ferlazzo, M. L., and Banati, R. B. (2022). Long term food stability for extended space missions: a review. Life Sci. Space Res. 32, 79–95. doi:10.1016/j.lssr.2021.12.003
Zoldak, J. (2018). Medical consumables tracking-GRC. Available at: https://taskbook.nasaprs.com/tbp/index.cfm?action=public_query_taskbook_content&TASKID=11212.
Keywords: space exploration, medical imaging, vital sign measurement, intravenous fluid administration, medical oxygen, blood analysis, procedural guidance, international space station
Citation: Lewandowski BE, Schkurko CM, Miller RS, Valentine RW, Calaway KM, Yang JD, Ebert DJ, Sargsyan A, Byrne V, Walton M, Lemery J, Suresh R, Thompson MS, Easter BD and Lehnhardt KR (2024) Technology modification, development, and demonstrations for future spaceflight medical systems at NASA. Front. Space Technol. 5:1384457. doi: 10.3389/frspt.2024.1384457
Received: 09 February 2024; Accepted: 17 June 2024;
Published: 15 July 2024.
Edited by:
Ilaria Cinelli, Independent Researcher, Torino, ItalyReviewed by:
Jack J. W. A. van Loon, VU Amsterdam, NetherlandsErik Seedhouse, Embry–Riddle Aeronautical University, United States
Christiane Heinicke, University of Bremen, Germany
Copyright © 2024 Lewandowski, Schkurko, Miller, Valentine, Calaway, Yang, Ebert, Sargsyan, Byrne, Walton, Lemery, Suresh, Thompson, Easter and Lehnhardt. This is an open-access article distributed under the terms of the Creative Commons Attribution License (CC BY). The use, distribution or reproduction in other forums is permitted, provided the original author(s) and the copyright owner(s) are credited and that the original publication in this journal is cited, in accordance with accepted academic practice. No use, distribution or reproduction is permitted which does not comply with these terms.
*Correspondence: B. E. Lewandowski, beth.e.lewandowski@nasa.gov