- 1Dipartimento di Ingegneria Meccanica, Chimica e dei Materiali, University of Cagliari, Cagliari, Italy
- 2CRS4, Center for Advanced Studies, Research and Development in Sardinia, Cagliari, Italy
- 3Sardinia AeroSpace District (DASS), Cagliari, Italy
- 4CNR-INO Istituto Nazionale di Ottica, Firenze, Italy
- 5Istituto Nazionale di Astrofisica (INAF), Osservatorio Astrofisico di Arcetri, Firenze, Italy
- 6Department of Life and Environmental Sciences, University of Cagliari, Cittadella Universitaria di Monserrato, , Monserrato, Italy
- 7Department of Biomedical Science, University of Sassari, Sassari, Italy
- 8Department of Clinical and Movement Neurosciences, Institute of Neurology, University of College London, London, United Kingdom
- 9Department of Medical Sciences and Public Health, University of Cagliari, Monserrato’s Campus, Monserrato, Italy
The long-term solution to problems like overcrowding, fossil fuel depletion, climate change, and decreasing natural resource availability could be overcome through space colonization and human presence in space, as well as the exploitation of extraterrestrial natural resources. In keeping with this, the objective of this work is to analyze current advancements in technology development for deep space exploration and colonization made by our research team as well as by other organizations with which we are collaborating. First, a method for producing tangible goods suited for industrial or civil installations on the Moon, Mars, or asteroids, using in situ available regolith as the main resource, is discussed. In this regard, a new process based on the occurrence of self-propagating high-temperature synthesis (SHS) reactions was developed for the fabrication of composite ceramics to be used as construction materials. A theoretical analysis of the process using proper dimensionless numbers is also described to offer potential explanations of the key experimental evidences presented in the relevant literature. For instance, it is found that free convection likely plays a crucial role to make SHS front velocity higher under terrestrial conditions when the reaction ignition is carried out from the bottom side, instead of the top side, of reacting mixture. Next, a method that uses the atmosphere and regolith of Mars as raw feedstock to produce in situ useful material such as oxygen, water, food, fuels and fertilizers, is considered. In the next section, the potential for cultivating Spirulina platensis to provide nourishment for the Martian crew is examined. The possible use of sintered lunar regolith simulants such as JSC-1A is also considered for potential thermal energy storage and solar energy harvesting applications, within the context of resource exploitation. Sintered regolith simulant exhibited, compared to the native material in powder form, superior solar absorptance, which makes it suitable for sunlight absorbers in architectures with a cavity-like solar receiver. Finally, a new study is reported which combines biochemical and biophysical approaches in order to compare, under simulated microgravity and under terrestrial conditions, the functioning and structure of red blood cells, over various intervals of time.
1 Introduction
In-Situ Resource Utilization (ISRU) and In Situ Fabrication and Repair (ISFR) technologies are essential components for space exploration and colonization. ISRU technologies can provide life support consumables, propellants, construction materials, and energy, to a crew stationed on a moon, planet, or asteroid (Sanders and Larson, 2011; Mason and Rucker, 2019; Schlüter and Cowley, 2020; Baldry et al., 2022). ISFR technologies, in contrast, aim to meet requirements related to the fabrication and repair of materials and equipments, in situ, at the location where they operate (MlYAZAKI and Osamu, 2002; Miyazaki and Odawara, 2003; Moore et al., 2004; Bassler et al., 2006; Faierson et al., 2010). To this end, an Italian task force comprised of the University of Cagliari, the Italian Astrophysics Institute (INAF), the National Research Council (CNR), the University of Sassari, and the Center of Research, Development, and Advanced Studies in Sardinia (CRS4), is currently engaged in research activities aimed at the development of both ISRU and ISFR technologies.
The goal of this work in this context is to put together the published results obtained by such task force in the field of deep space exploration in a time span from 2012 to 2023. Briefly, the results discussed in what follows regard: the possibility to use high temperature self-propagating reactions to produce structural elements on Mars and Moon; an ISRU process involving several process units to produce food, fuel, oxygen, water, and other consumables on Mars to sustain long-term crewed missions; the optical characterization of lunar regolith for its possible utilization as solar absorber; the use of microalgae to produce edible biomass to feed the crew during the mission; the effect of microgravity on erythrocytes. While the latter topic do not strictly regard the use of ISRU and ISFR technologies, it deals with the possible effects on the human body of microgravity conditions taking place during a crewed mission on Moon or Mars, during which the above technologies are used. For this reason, we considered to be useful to include and discuss these results in the final part of the paper.
2 Manufacturing physical assets in extraterrestrial environments
Until 2011, before Cao. et al. patent was filed (Cao et al., 2011) and the related papers published (Corrias et al., 2012a; Corrias et al., 2012b), only few works were addressed in the literature to the development of processes for the fabrication of protection structures making use of available in situ Lunar resources (Allen et al., 1994; Toutanji et al., 2005; Martirosyan and Luss, 2006; Tucker et al., 2006; Faierson et al., 2010). To this aim, Allen et al. (1994) proposed a processing route where a regolith simulant was sintered by radiant and microwave heating. Lunar regolith was also used for producing a thermo-plastic material (Toutanji et al., 2005) and fiberglass reinforced Lunar concrete (Tucker et al., 2006). The other developed processes were based on combustion synthesis-type reactions for the obtainment of ceramic composites using Lunar regolith simulants in the starting mixture (Martirosyan and Luss, 2006; Faierson et al., 2010). Along these lines, Cao et al. (Cao et al., 2011) developed an ISFR process for manufacturing physical assets necessary for industrial and/or civil facilities on Mars, the Moon, and/or asteroid, in addition to the kit of equipment and materials required to achieve this goal. The resulting ISFR process includes the operating stages shown schematically in Figure 1.
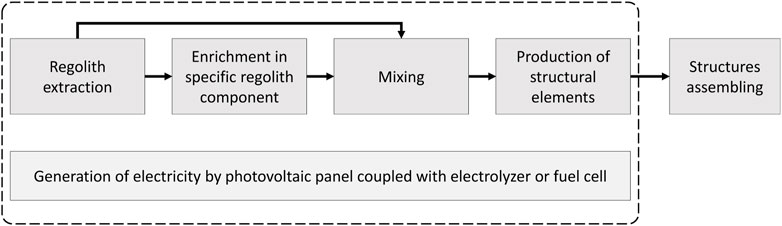
FIGURE 1. Schematic flow-sheet of the process for manufacturing physical assets for civil and/or industrial facilities on Moon and Mars (adapted from Concas et al.,2012, licensed CC-BY-4.0).
The first stage of the process is the regolith extraction from the lunar or martian soil. This regolith is then enriched with a particular component, such as iron oxide, which is widely present in Mars minerals, that can be obtained in situ by magnetic separation. The third step involves mixing the enriched regolith, when necessary, with aluminum powder carried from Earth.
The following step, which represents the heart of the entire process, involves self-propagating high temperature combustion reactions occurring within the mixture formed by the previous step. These reactions are able to propagate spontaneously, upon local ignition, at high temperatures (generally above 1,800–2,000 K) in the form a combustion wave (Merzhanov and Borovinskaya, 1972; Munir and Anselmi-Tamburini, 1989; Varma et al., 1998a). They are particularly known for being exploited to develop the technique known in the literature as Self-propagating High-temperature Synthesis (SHS), which allows to produce a wide range of advanced materials such as ceramics, composites, intermetallics, solid solutions, functionally graded materials, etc. (Merzhanov and Borovinskaya, 1972; Munir and Anselmi-Tamburini, 1989; Merzhanov, 1995; Hlavacek and Puszynski, 1996; Varma et al., 1998b; Cao et al., 2011; Corrias et al., 2012b; Concas et al., 2012).
Also known as Combustion Synthesis, the SHS method, has gained popularity due to its short reaction time, low energy requirements, simple equipment, and capacity to produce complex or metastable phases.
That reacting step is carried out in a specific reactor that serves as the process core unit and is expected to be able to operate under lunar and Martian conditions (low temperatures, microgravity, and a rarefied atmosphere of CO2), thus allowing the manufacture of structural elements of selected size and shape using appropriate molds. Such aspects are of great practical importance because the process involves a very simple reaction using a low external power supply while yielding solid final products with suitable mechanical properties for construction applications (Corrias et al., 2012a). The experiments involved the use of simulants of Lunar (JSC) and Martian (JSC and Mojave) regolith and a suitable reducing agent in the form of powders. Lunar JSC consisted mainly of ilmenite, plagioclase, olivine and Ca-rich pyroxene, while the main constituents of Martian JSC and Mojave Martian regoliths are ilmenite and olivine, plagioclase feldspar, pyroxene, iron oxides (magnetite, hematite). Further ilmenite was added to the lunar regolith simulant, while additional hematite (Fe2O3) was introduced in the mixture containing martian regolith simulants. Then both lunar and martian enriched simulants were mixed with Al powders which acted as reducing agent.
The resulting solid mixtures were compacted in the form of cylindrical pellets (length and diameter of about 25 mm and 11 mm, respectively) by means of a hydraulic press.
The pellets were then inserted in a reaction chamber wherein vacuum (30 mbar) was previously created. Whitin the reactor, a power supply provided the energy input necessary to ignite the redox reaction between Al and ilmenite, for the case of Lunar regolith, or between Al and hematite, for the case of Martian regolith, at one sample end. Very high temperatures (generally above 1,526°C) were achieved in the sample due to the high exothermic character of the redox reactions described above (Corrias et al., 2012a). The high exothermicity of reactions permits also the reactions to self-propagate along the pellet until the combustion front achieves the opposite end of the sample. At this point only reactions products are obtained in the form of cylindrical pellets. Parallelepiped (14 mm × 17 mm × 33 mm) shaped specimens have been also prepared (Corrias et al., 2012b). In the latter case, the obtained products represent just the structural components shown in Figure 2. The resultant structural elements, as shown in Figure 3, can be used to construct civil and/or industrial facilities on the Moon or Mars.
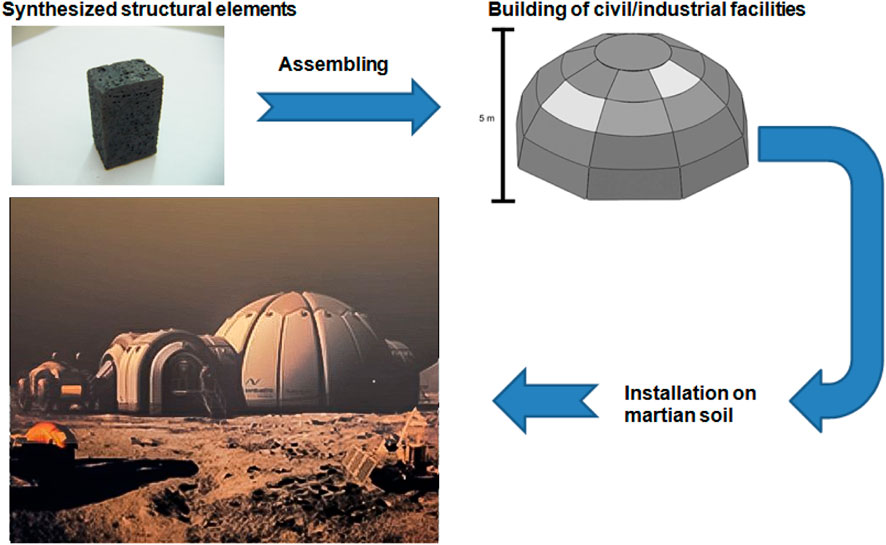
FIGURE 3. Implementation of the invented process on Moon and Mars (adapted from Concas et al., 2012, licensed CC-BY-4.0).
Compressive strength values of the obtained SHS samples, in the range of 25.8–27.2 MPa (Corrias et al., 2012a), are significantly higher with respect to those (10–18 MPa) reported in the literature for products resulting from the geothermite-based process proposed by Faierson et al. (2010). Final validation of the regolith-derived samples obtained by Cao et al. (Cao et al., 2011) requires a their complete characterization, not only from the mechanical point of view but also from the optical one, etc., which will be addressed in future dedicated works.
The main stage of the patented process was also tested in October 2010 in the framework of the 53rd parabolic flight campaign held in Bordeaux, France with experiments performed under microgravity conditions aboard a custom Airbus-300 during thirty parabolas of three completed missions (Corrias et al., 2012b).
To highlight the possible effects of gravity on SHS reactions and the related products, the synthesis of TiB2-xTiAl and TiB2-xTiAl3 composites was performed, other than under terrestrial conditions, also at low-gravity during the 32nd ESA Parabolic Flights Campaign (Bordeaux, March 2002). The main findings from such parabolic flights experiments have been summarized in Section 3, along with the relevant references, when introducing theoretical considerations.
3 Theoretical considerations on combustion synthesis reactions
Several parameters are known to affect self-propagating high-temperature reactions which are exploited in this work to obtain physical assets from lunar and martian regolith (Munir and Anselmi-Tamburini, 1989; Varma et al., 1998a). In particular, reaction stoichiometry, reactant particle size, green density (i.e., the total mass of compacted powders before reaction divided by the corresponding volume), cooling and heating rates, and gravitational field strength, all have a significant impact on the SHS process dynamics as well as on the morphology and properties of the final product (Munir and Anselmi-Tamburini, 1989; Varma et al., 1998a). For example, combustion front velocity is typically found to decrease with an increase of particle size, whereas its dependence on green mixture density is characterized by a maximum in correspondence of an intermediate density level (Munir and Anselmi-Tamburini, 1989; Varma et al., 1998a). This behavior is caused by the influence of the latter parameters on the system reactivity, together with the compact thermal conductivity.
In addition, stoichiometry influences the exothermicity of the synthesis reaction and, consequently, the dynamics of the combustion process (Munir and Anselmi-Tamburini, 1989; Varma et al., 1998a).
The introduction of inert species, which are not directly involved in the reaction process, causes heat subtraction and, as a result, a reduction in combustion temperature. Other operational parameters can be considered in the same way. In this regard, gravity has been demonstrated to play a distinct and important role in reactions involved in self-propagating high-temperature processes (Shteinberg et al., 1991; Odawara et al., 1993; Yi et al., 1996; Mukasyan et al., 1997; Mukasyan and Pelekh, 1997; Odawara, 1997; Merzhanov et al., 1998; Yi et al., 1998; Tanabe et al., 1999; Merzhanov et al., 2000; Axelbaum, 2001; Lau et al., 2001; Medda et al., 2001; Merzhanov et al., 2001; Mukasyan et al., 2001; Merzhanov, 2002; Castillo et al., 2003; Mukasyan et al., 2005).
Indeed, combustion synthesis and associated structure formation mechanisms include several stages, such as melting of reactants and products, spreading of the melt, droplet coalescence, convection and diffusion, buoyancy of solid particles, and the solidification of liquid product, the majority of which are impacted by gravity (Yi et al., 1996; Merzhanov, 2002). Generated gaseous and liquid species are particularly subjected to gravity-driven flow, convection or vapor transport (Beysens, 2022). These phenomena are likely to have a significant effect on both the stability of SHS reactions and products phase morphology (Mukasyan et al., 1997; Tanabe et al., 1999).
It follows that investigating the impact of gravity on the aforementioned phenomena is critical in order to determine the detailed mechanism of structure formation and reaction evolution. Low-gravity experiments can, in particular, reveal the general mechanism of structure formation and combustion without the confounding effect of gravity, in addition to facilitating a direct comparison with data from comparable ground-based experiments.
Some general conclusions have been drawn based on the most relevant experimental results reported in the literature on combustion synthesis reactions conducted under low-gravity conditions (Mukasyan et al., 1997; Mukasyan and Pelekh, 1997; Lau et al., 2001; Merzhanov, 2002). For instance, the SHS reaction wave under reduced gravity typically travels slower than the corresponding observation on the ground. Furthermore, bottom ignition of the sample produces under terrestrial conditions higher combustion velocities than top ignition (Yi et al., 1996; Mukasyan et al., 1997; Mukasyan and Pelekh, 1997; Tanabe et al., 1999; Mukasyan et al., 2001; Merzhanov, 2002).
Additionally, neither ignition configuration nor the gravitational level have a significant effect on combustion temperature. On the other hand, final products synthesized under low-gravity have finer and more uniform microstructures than those synthesized under normal gravity (Yi et al., 1996; Mukasyan et al., 1997; Lau et al., 2001; Mukasyan et al., 2001; Merzhanov, 2002).
A thorough theoretical general analysis of potential effects of gravity on combustion synthesis has been performed (Locci et al., 2006) with the goal of elucidating the above experimental results. The effect of gravity on heat and mass transport within the reacting sample across different spatial scales was specifically investigated—as shown schematically in Figure 4. The arrows illustrate the potential paths by which gravity may affect transport phenomena involved in combustion synthesis processes, which ultimately determine the experimental results observed.
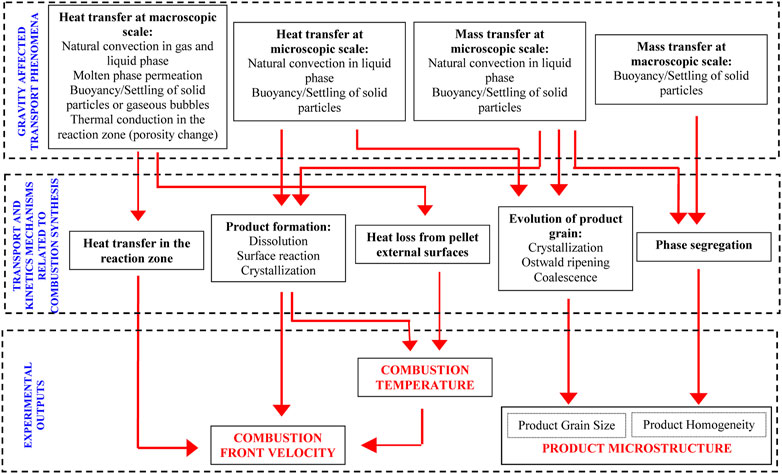
FIGURE 4. Schematic representation of the theoretical analysis performed on combustion reactions (adapted from Locci et al., 2006, with permission from American Institute of Chemical Engineers).
The first step investigated the gravity contribution to heat transfer inside the pellet region where the most relevant physico-chemical phenomena typical of SHS are observed: the reaction zone. The next step took into account the effect of gravity upon the rate of product formation: this is dependent on heat and mass transport phenomena occurring at the microscopic level, i.e., product grains and/or reactant particles.
An explanation of the experimental behavior of combustion temperature is derived combining the above analysis with that relating to the effect of gravity-level on heat losses from the pellet external surface, where the characteristic length can be identified with the pellet size (macroscopic scale).
The experimental results for combustion front velocity were subsequently analyzed by taking into account gravity effect on heat transfer inside the reaction zone, on the product formation rate and on combustion temperature. Gravity impact at the microscopic level on heat and mass transfer was also considered to account for differences in grain size obtained in products obtained under distinct gravity-level environments (Yi et al., 1996; Mukasyan et al., 1997; Lau et al., 2001; Mukasyan et al., 2001; Merzhanov, 2002). Finally, we clarified the role of the gravitational field in the formation of product microstructures in terms of phase distribution by describing mass transport phenomena pertaining at both micro and macroscopic scales, as well as their effect on phase segregation.
A comprehensive analysis based on appropriate dimensionless numbers (Locci et al., 2006) indicates that, during SHS the gas phase natural convection has negligible effect on heat loss to the surroundings off the exterior surface of the reacting pellet. Since the rate by which chemical reactions release heat was found to be insensitive to g-level, the finding above suggests that gravity is unlikely to have significant influence on combustion temperature, as is consistent with experimental evidence.
Sample porosity variations caused by pellet expansion, even if slight, are expected to affect heat transfer within the reaction zone (i.e., apparent thermal conductivity) (Shteinberg et al., 1991; Tanabe et al., 1999) Thus, when occurring under reduced gravity, the SHS reaction can be expected to exhibit a relatively slower combustion front and an earlier quenching extinction.
Furthermore, under terrestrial conditions, the combustion front exhibits a relatively higher velocity when ignition is performed from the bottom side of the pellet instead of the top side, which is commonly observed. This phenomenon can be reasonably attributed to the occurrence of free convection within the molten phase formed in the reaction zone. Indeed, such phenomenon determines the preheating of the reactants present ahead of the combustion wave, thus facilitating its propagation.
The next step of this activity will consist in the detailed theoretical analysis of the SHS process proposed in the patent by Cao et al. (Cao et al., 2011) and related papers (Corrias et al., 2012a; Corrias et al., 2012b) for the obtainment of physical assets from Lunar regolith under low-gravity conditions.
4 An ISRU process to sustain human missions on Mars
While ISFR is specifically dedicated to fabrication and repair of infrastructure and equipment, the objective of the general ISRU approach is to utilize extraterrestrial resources to produce consumables and create new services and so significantly decrease the payload, cost, and risk-factor for near and mid-term space exploration. The use of ISRU can thus represent a great boost to crewed missions of Mars and Moon because it greatly increase the techno-economic feasibility of the mission. When focusing on Mars resources, the ones available for human use are carbon dioxide, argon, nitrogen, and atmospheric traces of water vapor, in addition to water in the regolith (Herri and Chassefière, 2012). In principle, suitable ISRU processing technologies can convert these raw resources into useful products or materials, furnishing numerous benefits for permanent crewed missions. Moreover, the Environmental Control and Life Support Systems (ECLSSs) enable waste recycling and the subsequent production of useful materials such as water, oxygen, and food. Although the ultimate goal of modern ECLSS systems is to achieve a closed loop capable of meeting the crew’s primary needs (in terms of oxygen, water, and food), experiments and mathematical simulations show that, to date, only a small percentage of the required amount of these materials can be produced by recycling wastes (Poughon et al., 2009). ECLSS systems, which are routinely used on the International Space Station, (ISS) consist of a collection of units to remove inorganic and organic catabolites (like humidity, exhaust atmosphere, feces and other kinds of liquid or solid waste) and so to allow astronauts to sustain physiological parameters in the cabin or crew habitat. At the time when the second patent by Cao et al. (2012) was filed no processes capable to produce consumables to sustain crewed missions on Mars by integrating ECLSS with ISRU could be found in the patent and scientific literature. The patent (Spacex. No Title, 2023) here refers to a particular ISRU process that works synergistically with an ECLSS. The purpose of this combination is to ensure the crew’s self-sufficiency in meeting both energy and material requirements for producing essential resources like oxygen, water, food, as well as other consumables such as fertilizers and fuels. Starting from the Martian atmosphere and soil, this technology (Cao et al., 2012) aims to produce water for drinking and washing, breathable oxygen, as well as energy, along with ammonia and hydrogen for propellants, ammonium nitrate and nitric acid for fertilizers, and edible biomass (c.f. Figure 6; Table 2).
Figure 5 depicts the basic idea of the filed patent regarding the synergistic participation of ISRU and ECLSS. In this regard, the ISRU plant is composed of two sections that interact: the biological and the physico-chemical (c.f. Figure 6).
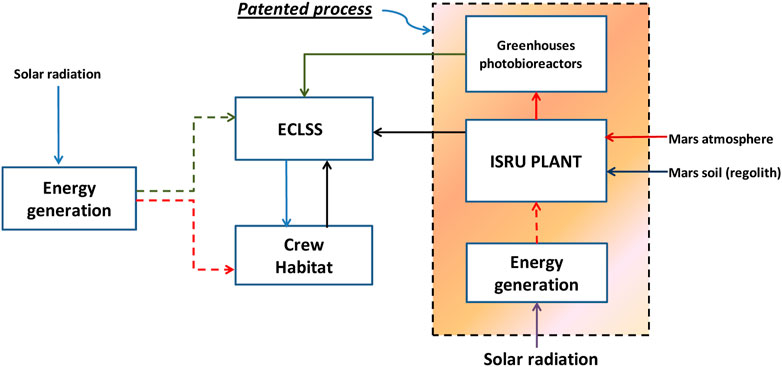
FIGURE 5. Schematization of baseline concept for the synergic combination of ISRU and ECLSS systems (adapted from Concas et al., 2012, licensed CC-BY-4.0).
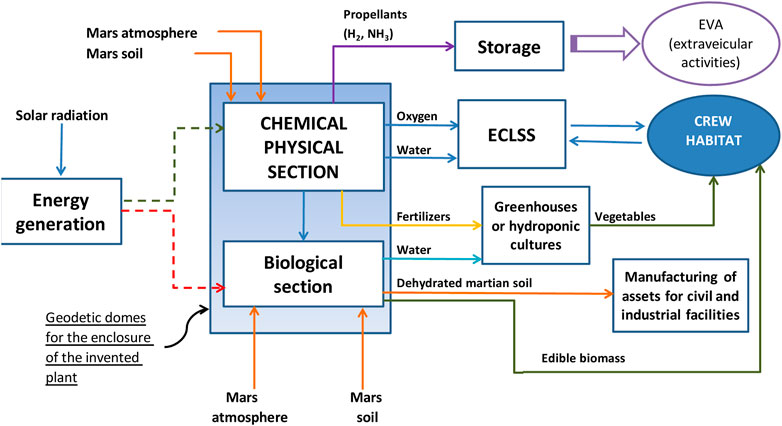
FIGURE 6. Schematic flow-sheet of the invented ISRU process (adapted from Concas et al., 2012, licensed CC-BY-4.0).
In the physico-chemical section, the juxtaposition and combination of different plant units designed specifically to function under Martian conditions permits a production of oxygen, water, and propellants necessary for a crew of six, in addition to suitable quantities of fertilizer for use by the biological section. The optimal operating conditions, i.e., the ones permitting to obtain the desired mass flows of consumables for the crew, have been determined using relevant mathematical models.
The physico-chemical section main plant units are supplied by water adsorption reactors (Williams et al., 1995), geodetic domes, solid state compressors, water and CO2 electrolyzers, temperature swing adsorbers (Rapp et al., 1997), magnetrons (Wiens et al., 2001), electrosynthesis reactors, absorption mini-towers and catalytic reactors (Ostwald, 1907), as well as photovoltaic panels for energy.
The biological section uses photobioreactors and greenhouses to produce edible biomass and photosynthetic oxygen from natural resources (e.g., CO2 from the regolith and atmosphere) or products synthesized from the physico-chemical section. Table 1 shows examples of the quantities of energy and consumables produced by the ISRU plant in relation to a given design case which consumes the amount of natural resources reported in the same Table.
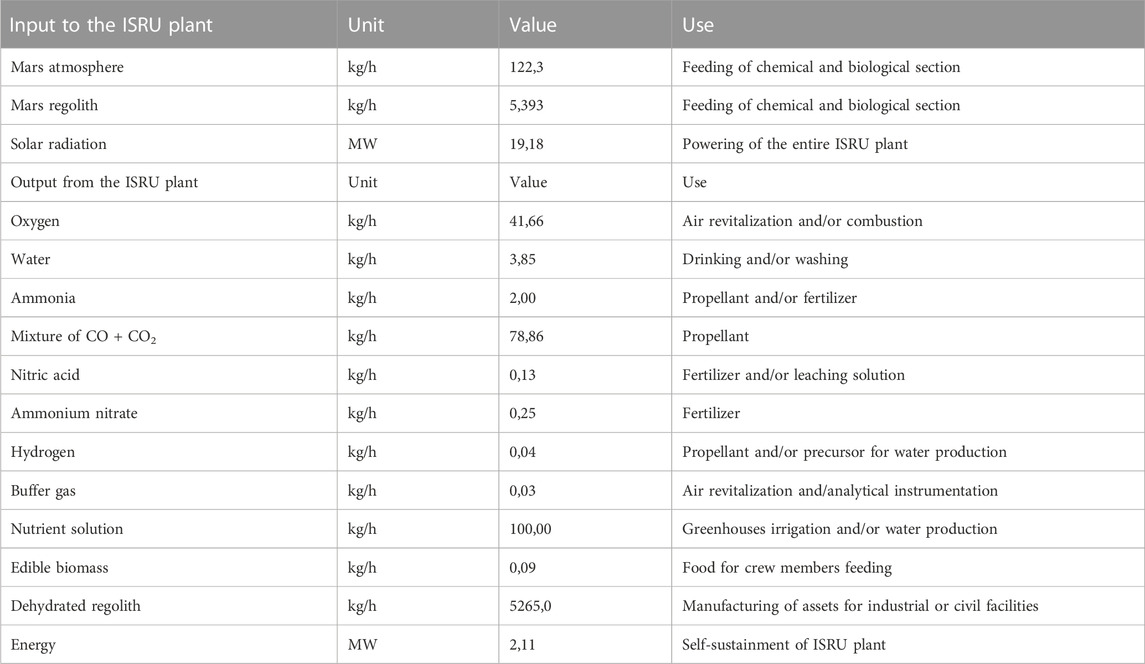
TABLE 1. Summary of employed resources and produced consumables with the proposed ISRU process for a specific design case [adapted from (Cao et al., 2012)].
To assess the economic and technical feasibility of such a process, the payload of the entire ISRU plant must be calculated. Table 2 shows a summary of the payload of such a plant.
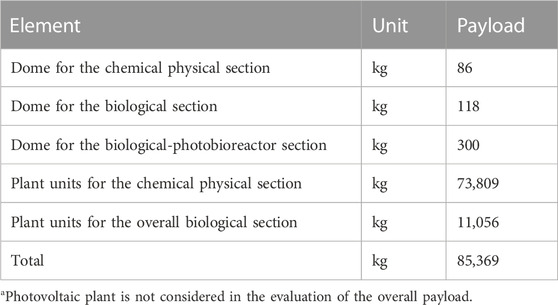
TABLE 2. Summary of the payloads for the proposed ISRU system for a specific design casea [adapted from (Cao et al., 2012)].
In this regard, it should be noted that, SpaceX claims to be owner of technology, including a Starship spacecraft and Super Heavy rocket–collectively referred to as Starship–that is capable to carry both crew and cargo to Earth orbit, the Moon, Mars and beyond for a total payload of 150 metric tonnes fully reusable and 250 metric tonnes expendable (Spacex. No Title, 2023). So the complete ISRU plant could be on Mars with two missions.
The next step of this activity will consist in the experimental implementation of the process proposed by the patent to verify whether it can be actually and profitably carried out on Mars.
5 Edible microalgae on Mars by exploiting in situ-available resources
Only a few papers or patents (Miyajima, 2017; Abney et al., 2018; Miyajima, 2019; Nangle et al., 2020) address the technological development needed to integrate ECLSS and ISRU systems to support crewed missions to Mars of the type described above. In particular, they involve simulations that typically depend on assumptions about the performance of the ISRU-ECLSS system that do not accord well with experimental data obtained by apparatus designed to operate under Martian-like conditions. In this scenario, the multi-disciplinary field of bioengineering techniques involving microalgae, macroalgae, fungi, and bacteria is now firmly established in the implementation of regenerative ECLSS (Belz et al., 2014; Revellame et al., 2021; Verseux et al., 2021), and some studies have confirmed the possibility to use microorganisms to transform available resources on Mars, such as the atmosphere and regolith, into practical supplies. For instance, Santomartino et al. (2022) described the latest developments in the principles of space biomining, suggesting that selected microorganisms could be used not only to extract structural metals, mineral nutrients, water, and oxygen but also to recycle waste and support regenerative life support systems (Rapp, 2018). Noteworthy is that food production could reasonably be guaranteed using the In-situ materials (Eichler et al., 2021; Revellame et al., 2021; Verseux et al., 2021; Kasiviswanathan et al., 2022; Caporale et al., 2023). However, most In-situ Resource Utilization (ISRU) technologies proposed to date rely on physico-chemical methods that produce oxygen and propellants from the Martian atmosphere and regolith, but do not generate foodstuffs (Rapp, 2018; De Man, 2019). In-situ food production remains the main bottleneck for crewed missions, since what ECLSSs can contribute is fairly limited compared to the needs of a crew of six astronauts, which is estimated to be 3,000 cal sol−1 (Cao et al., 2021; Fais et al., 2022a) (and references therein). Thus, ISRU technologies are required for food production on Mars. Although some studies explore the cultivation of crops, fungi, and algae on Mars, few papers address the conversion of Martian resources into edible biomass under conditions similar to those found on Earth. There is even less literature addressing the use of resources available in the Martian environment to support the growth of cyanobacteria and microalgae (Verseux et al., 2016). Moreover, there are few experimental studies related to the production of edible biomass from microalgae in space that consider the effect of microgravity, which is 0.3 g on Mars (Fais et al., 2022a). However, it has been experimentally demonstrated, aboard the International Space Station (ISS), that it is possible to perform the extraction of rare earth elements from basaltic rocks through bioleaching under microgravity and Mars gravity conditions. The three microorganisms used, Spirulina desiccabilis, B. subtilis, and C. metallidurans, proliferated within a miniaturized space biomineral reactor, thus demonstrating not only the possibility of growing microorganisms under conditions different from Earth’s gravity but also the efficacy of microbe-mineral interactions in promoting self-sufficient human presence beyond Earth (Cockell et al., 2020). Therefore, in order to investigate the possibility of cultivating microalgae and cyanobacteria under microgravity conditions, using some of the resources present on Mars, and following the paradigms described above. A novel process to grow edible microalgae on Mars by exploiting in situ available resources has been proposed. Newly developed experimental apparatus has allowed the growth of Spirulina platensis (S. platensis) a cyanobacterium called under conditions of microgravity (Cao et al., 2021; Fais et al., 2022a), inside heated and pressurized domes. This offers opportunity to assess the use of cyanobacteria and microalgae as potential food sources within the structure of crewed missions on Mars by relying on ISRU technologies. This method also uses a simulant of the urine produced by the crew members, that can be considered a potential constituent of the alga’s growth medium. The corresponding ISRU-ECLSS process is pictured in Figure 6, and is subject of a recent patent proposal by Cao et al. (2012).
The S. platensis strain was selected over others due to its protein content and higher nutritional power, which make it a food suitable for astronauts. Indeed, various studies have indicated S. platensis to be a superfood, and it has been called the “food of the future” due to its elevated nutritional value (Jung et al., 2019). A variety of foods are now manufactured from it (Fais et al., 2022b).
Because of its high protein content and rapid growth in mineral constituted environments, space industries and agencies have developed an interest in incorporating such strains into research programs by utilizing the ISRU-ECLSS process (Montague et al., 2012). On a dry weight basis, S. platensis consists of up to 70% complete protein with high digestibility and elevated biological value, 20% carbohydrates and 10% lipids. S. platensis has antioxidant activity in line with its carotenoid, and Vitamin E content, as well as anti-inflammatory, antitumor, and immunostimulant activity (Fais et al., 2022b).
The growth of S. platensis has been investigated inside a specially developed device that simulates microgravity and a pure CO2 atmosphere. The culture medium contained a mixture of appropriate quantities of regolith leachate and urine simulants. The goal was to evaluate potential food production on Mars using in situ available resources (Cao et al., 2021; Fais et al., 2022a). The results obtained demonstrated that the chosen strain grew better under simulated Martian conditions (with the in situ produced growth medium) than under terrestrial conditions adopting an optimal growth medium (c.f. Figure 7). Indeed, under Earth conditions, biomass productivity was around 0.048 g L−1 day−1, while on Mars, productivity was around 11 g L−1 day−1. With about 15 m3 of culture that productivity value could meet the protein requirements of a six-member crew. While several factors might have influenced this result, the most important included the CO2-rich atmosphere which prevents carbon starvation and microgravity that might have reduced aggregation and settling, thus allowing a better nutrient diffusion toward single cells. On the other hand, this latter effect should be confirmed by dedicated experimental activity, since a work retrieved in the literature reports the if there are different components in the solution with different molecular weights, microgravity can lead to “segregation” and “stratification” if proper mixing is not provided (Bechini et al., 2021).
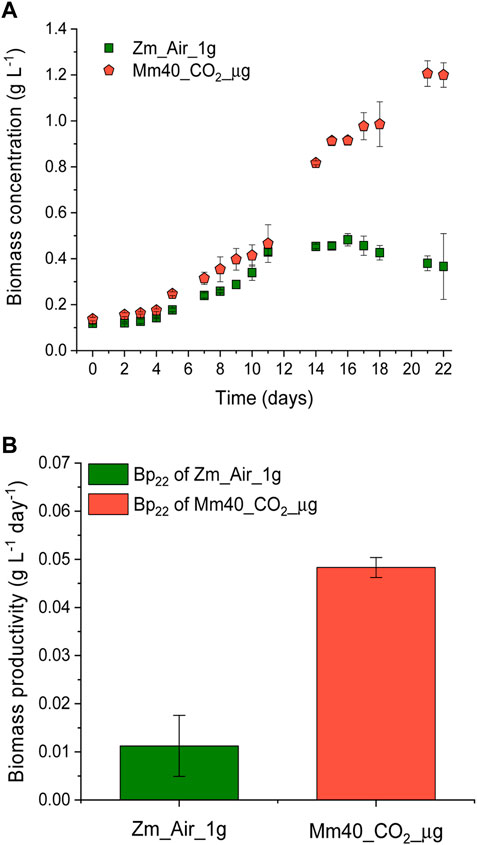
FIGURE 7. Synergic effects of all the operating conditions that would take place on Mars according to the process on the time evolution of Spirulina platensis concentration strain that grows better under simulated Martian conditions than under terrestrial conditions adopting an optimal growth medium called Zm in air and on Earth gravity 1g (A) and on the biomass productivity after 22 days of cultivation (B) – (adapted from Fais et al., 2022, with permission from Acta Astronautica). Notations: Mm40_CO2_μg growth in a medium consisting for 40%v of Martian Medium under CO2 atmosphere and microgravity; Zm_Air_1 g refers to the growth in pure Z-medium under air and Earth gravity.
Scope exists for further research to better understand those aspects, and to evaluate the effects of low temperatures and cosmic radiation prevalent on Mars, which were not examined in the study. Note, in this regard the cultivation of algae might theoretically be achieved inside pressurized, heated inflatable domes located within an open pond, the creation of which would necessitate the excavation and lining of the soil. Such a design would mean a lower payload being needed for the pond’s realization.
6 Optical characterization of lunar regolith simulant
So far, we have focused on novel ISRU technologies to support human missions on Mars. Other targets of space exploration include the Moon and asteroids, whose surfaces are devoid of atmosphere. The surface of the Moon, due to its high solar irradiance, provides favorable conditions for harvesting solar energy, given an appropriate site (Kaczmarzyk et al., 2018).
However, the long duration of lunar nights makes energy storage a significant issue. Thermal energy storage (TES) may be the best option for collecting energy for future extraterrestrial colonies or robotic stations. The most natural TES candidate material appears to be regolith, once it has been processed into bulk bodies to improve its thermal properties (Fleith et al., 2020; Palos et al., 2020).
In the latter regard, the technologies and components potentially required for solar energy storage on the Moon and related stages (heat transport, conversion to electricity, etc.) has been recently analyzed in the literature (Palos et al., 2020).
Along these lines, and from an ISRU standpoint, we have developed an assessment procedure for the optical characterization of a simulant of lunar regolith in order to estimate the potential of pristine and sintered real regolith for thermal energy storage and solar energy harvesting.
The composition and thermal stability of a sieved simulant JSC-1A regolith was initially investigated (Licheri et al., 2022).
Then powders were first processed for 3 min at 700°C and 900°C (heating rate: 100°C/min), in a 20 Pa vacuum, under 15 MPa mechanical pressure, using an efficient hot-pressing technique known as Spark Plasma Sintering (SPS) (Licheri et al., 2022). SPS was also used by Zhang et al. (2020) for the consolidation of FJS-lunar soil simulants. In our work, the preparation of sintered samples from JSC-1A Lunar regolith was carried out using a 515 model SPS apparatus (Fuji Electronic Industrial Co., Ltd., Kanagawa, Japan). The latter equipment is able to apply electric current intensities and mechanical load levels up to 1,500 A and 50 kN, respectively.
The disks obtained, which had relative densities of about 86 (700°C) and 98% (900°C), were then characterized in terms of microstructure and composition. No additional phases were detected after SPS as compared to the original regolith. This was despite some variations in their relative quantities, especially for the glassy part, on the increase of sintering temperature from 700°C to 900°C. For samples sintered at 700°C and 900°C, there has been extensive evaluation and discussion of optical properties including integrated solar absorptance, spectral emittance/absorptance, and integrated thermal emittance estimated from −173°C to 1,026°C (Licheri et al., 2022).
According to thermophysical property literature results, the optical properties of regolith are altered by powder sintering in a process-dependent manner (c.f. Figure 8). Sintering temperature, in particular, has been shown to raise spectral emittance/absorptance when compared to pristine powders.
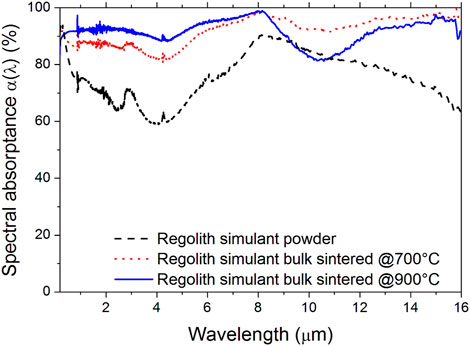
FIGURE 8. Absorptance spectra of sintered specimens obtained from lunar regolith simulant and the reference powder sample (adapted from Licheri et al., 2022, with permission from Acta Astronautica).
On the other hand, thermal emittance and integrated solar absorptance at all temperatures under consideration were increased. As discussed in detail in Licheri et al. (2022), the discrepancies in the optical properties of regolith simulant before and after being processed by SPS are due to the different morphological, structural and compositional characteristics of such samples. Indeed, as previously mentioned, the original pristine powders are transformed by SPS into 86 (700°C) and 98% (900°C) dense samples. In addition, while negligible changes from the compositional viewpoint are observed when the powders are processed by SPS at 700°C, a reduction in the content of the glassy phase is obtained at 900°C. All these changes apparently affect the optical behaviour of these samples.
Analogously to sunlight receivers on Earth, the ideal material to be employed on the Moon should possess high solar absorptance and low thermal emittance, i.e., superior spectral selectivity. Based on the obtained results both sintered pellets showed superior solar absorptance and thermal emittance compared to original regolith in powder form. The latter one displayed its maximum thermal emittance (0.80) at 26,85°C, while such parameter decreased to 0.73 at −173,15°C and 0.69 at 826,85°C. Absorptance values of samples sintered at 700°C and 900°C were 0.88 and 0.92, respectively, with emittance values in the range 0.87–0.96 (700°C) and 0.91–0.93 (900°C).
Accordingly, the higher solar absorbance makes sintered regolith blocks more suitable to be employed for sunlight absorbers in architectures with a cavity-like solar receiver. In particular, the sintered sample produced at 900°C is found to be the most promising candidate for operating temperatures below 726,85°C, due to the correspondingly highest solar absorptance and lowest thermal emittance. On the other hand, the opposite situation is encountered at operating temperatures above 726,85°C, with the SPS specimen produced at 700°C which is preferable, due to its relatively lower thermal emittance.
The results obtained might open up promising avenues for ISRU applications of lunar regolith.
The next steps of this work will involve different aspects. One of the most relevant will be related to the possibility to produce by SPS larger components from lunar regolith, also characterized by geometrical shapes different from the simple cylindrical ones produced and characterized in Licheri et al. (2022). In this regard, it should be noted that the obtainment of larger samples is strictly related to the maximum electric current that the employed SPS apparatus is able to provide. Indeed, while the current intensity applied by the equipment used in Licheri et al. (2022) could not exceed 1500 A, powerful SPS machines available on the market are capable to guarantee significantly higher current levels (above 10,000 A), thus allowing for the production of larger sized-components. In addition, more complex shapes can be obtained using suitably designed powders containers (die/punches), also taking advantage of appropriate sacrificial materials (Manière et al., 2016). The scale-up of the process and the fabrication of complex shape components based on lunar regolith will be both highly facilitated by the use of mathematical models of the SPS process as that recently developed in the literature by Locci et al. (2020).
7 Red blood cells behavior under microgravity conditions
Exposure to microgravity during space flight is known to involve a variety of health risks to the human body, increasing vulnerability to secondary conditions like radiation effects and physiological changes such as muscle atrophy, lysis, neocytolysis, metabolic variations, and hydrostatic pressure changes (Schimmerling, 1995; Schcolnik-Cabrera et al., 2014; Buonanno et al., 2015). Microgravity conditions and radiation can stimulate a variety of stressors in astronauts during spaceflight with negative consequences for their health (Garrett-Bakelman et al., 2019; Afshinnekoo et al., 2020). Oxidative stress (Stein and Leskiw, 2000), due to a disequilibrium between oxidant and antioxidant species (Pizzino et al., 2017; Tsamesidis et al., 2020), is the primary cause of cellular damage in astronauts.
Previous studies (Pantaleo et al., 2016; Remigante et al., 2021) were designed to present up-to-date information on band three protein, with a focus on its functional role in oxidative stress conditions and oxidative stress-related disease. The resultant reactive oxygen species (ROS) primarily include hydrogen peroxide (H2O2), superoxide anion (O2−), as well as free radicals. A rise in oxidants leads to the imbalance towards oxidizing species known to be responsible for a variety of pathologies in humans and are a cause of ageing.
In particular, astronauts face a number of health issues upon their return to Earth, including a 20% decrease in bone density, muscle atrophy, cognitive, immune system and endocrine disorders–as well as cardiovascular dysfunction (Hughes-Fulford et al., 1998). Significant variations in phospholipids were observed in samples of blood from Russian cosmonauts (Ivanova et al., 2006). A rise in phosphatidylcholine level is associated with a rise in membrane rigidity.
Changes in the physicochemical properties (permeability and microviscosity) of the erythrocyte plasma membrane can affect oxygen transfer efficiency, the structure of haematoporphyrin and the state of the haemoglobin. Furthermore, thin layer chromatography along with densitometric analysis of stained dots has been applied to determine composition of lipid and phospholipid in erythrocyte membranes. Although this technique provides data about the entire lipid class, it does not cater for the identification of specific molecules within that class–so it remains difficult to clarify the biochemical mechanisms involved (Ivanova et al., 2006).
However, existing studies have mostly focused on data collected post-spaceflight, with very little data describing changes occurring during the actual flight. Regarding red blood cells (RBCs), spaceflight is known to cause oxidative stress (Rizzo et al., 2012), though the exact role of microgravity is not yet known, and there is evidence of variation in blood homeostasis such as pseudopolycythemia or space anaemia (Alfrey et al., 1996).
Astronauts exhibit a diminution in plasma volume and thus a rise in erythrocyte relative volume during the early days of low gravity conditions (Kunz et al., 2017; Trudel et al., 2022). The adaptive response of the body to such conditions is a decrease in erythropoietin synthesis and an increase in erythrocyte clearance (Charles et al., 1994). Consequently, haemoconcentration occurs due to a rise in haematocrit, which is aided by a rapid fall in erythrocyte selective counting and haemolysis (Risso et al., 2014).
Following from the considerations above and the high cost of space experiments, there is a rising demand for the development of technologies to secure near zero-gravity environmental conditions, nearer to Earth (Unsworth and Lelkes, 1998).
Onboard the ISS: a g-force between 10−3 and 10−6 is used to simulate microgravity conditions, resulting in a weightless environment (Dinarelli et al., 2018). International space agencies have developed and validated such conditions using 3D-clinostats and random positioning machines (RPM) (Dinarelli et al., 2018; Kiss et al., 2019; Clary et al., 2022)–(c.f. Figure 9). These instruments generate a rapid rotation along three axes, to obtain zero gravity (Herranz et al., 2013; Wuest et al., 2015).
The few studies that have been conducted to investigate the behavior of RBCs under simulated micro-gravity, show that the cells rapidly respond to the environment, first as a metabolic adaptive response and then as fixed morphological and structural alterations, resulting in alterations or modulations of functionality (Serova et al., 1993; van Loon, 2007; Herranz et al., 2013; Wuest et al., 2015; Dinarelli et al., 2018).
The literature provides no existing data concerning the changes to RBCs under microgravity conditions, so a novel approach has recently been presented (Manis et al., 2022). It aims to better understand changes related to cell membrane components, through the applications of various biophysical and biochemical techniques, such as confocal microscopy and scanning electron microscopy (SEM), as well as the potential exploitation offered by chromatographic and mass spectrometry. It proposes to fully evaluate the function and structure of RBCs under normal and under 3D-clinostat-delivered microgravity.
Investigations using confocal microscopy SEM, TAC, ROS, GSH, MDA, and GSH analysis, and lipid profile evaluation reveal that samples under simulated microgravity showed remarkable changes in RBC membrane stiffness and cytoskeletal architecture (c.f. Figure 10)–while no such changes are observed, over time, at normal gravity.
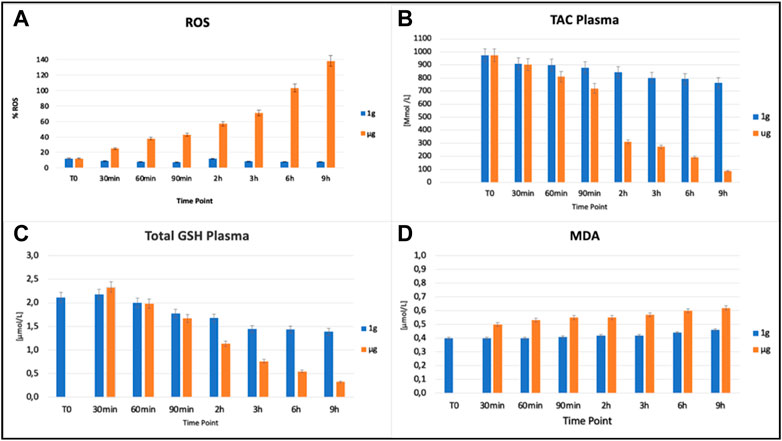
FIGURE 10. Evaluation of extracellular ROS level (A), TAC (B), GSH (C), and MDA (D) on plasma samples exposed to simulated microgravity (μg) and earth gravity conditions (1 g) at different time points (adapted from Manis et al., 2022, licensed CC-BY-4.0).
The above studies has added to our understanding of the biological mechanisms involved in red cell morphology and membrane property changes, and makes a significant contribution to our understanding of human cell behavior in microgravity.
Work is being done to analyze additional cell types, with a focus on those that could be important for future human missions, and to involve researchers and institutions at the international and national level in order to reinforce cooperation in this critical field of inquiry within the context of deep space exploration.
The outcomes and future directions of this research are concerned with the basic investigation of the intrinsic mechanisms that underpin the conduct of various types of human cell in conditions of microgravity, and ought thus to be able to yield useful contributions to the understanding and potential prevention of those classical health risks associated with astronauts operating in scenarios of deep space.
8 Concluding remarks
Interplanetary travel and exploration are becoming more concrete, through the plans formulated by international space agencies on deep space exploration missions. It is also quite well recognized that, in the absence of space programs, we would not have, for example, GPS, solar cells, accurate weather forecasting, or the ultraviolet filters used in cameras and sunglasses. If something happened on the Earth, such as a heavy asteroid impact, continuous climate changes, economic insecurity or viral pandemics stressing the world resources, our species of eight billion humans could be wiped out. Thus, the colonization of other bodies in the solar system (or constructing suitable habitats in orbit) by harnessing novel ISRU and ISFR technologies present a way to create a “backup” of humanity to survive whatever happens to the Earth. The scientific and technological activities and results reviewed in this work may be seen as a useful contribution to make that possible and sustain people independently of Earth. Such results can be summarized as follows: a novel technique based on SHS reactions has been proposed to produce structural elements from Mars and Moon regolith; a process which exploits regolith and atmosphere for the production of different consumables to sustain crewed missions on Mars has been designed; optical properties of a pristine and sintered lunar regolith simulant were evaluated in view of the possible utilization of such material as solar absorber; the possibility to produce microalgae on Mars has been demonstrated by performing experiments under microgravity using astronauts’ urine, regolith leachate and atmospheric CO2 simulants; the effects of microgravity on the growth and viability of erythrocytes is experimentally investigated.
Author contributions
Conceptualization, GC; investigation, GF, CM, AM, TC, and GU; data curation, RL, AL, ACi, PC, MP, GF, NL, and GB; writing—review and editing, GC; supervision, ACo, RO, ES, AD, and AP. All authors contributed to the article and approved the submitted version.
Funding
This work has been developed within the framework of the project e. INS-Ecosystem of Innovation for Next Generation Sardinia (cod. ECS 00000038) funded by the Italian Ministry for Research and Education (MUR) under the National Recovery and Resilience Plan (NRRP)—MISSION 4 COMPONENT 2, “From research to business” INVESTMENT 1.5, “Creation and strengthening of Ecosystems of innovation” and construction of “Territorial R&D Leaders”. The “SMS—Space Manufacturing in-situ—Realizzazione in contesti spaziali di manufatti” project (cod. ARS01_01361), sponsored by the Italian Ministry of Education, University and Research, Italy (Cod. CUP: B25F21001330005), is also acknowledged.
Conflict of interest
The authors declare that the research was conducted in the absence of any commercial or financial relationships that could be construed as a potential conflict of interest.
Publisher’s note
All claims expressed in this article are solely those of the authors and do not necessarily represent those of their affiliated organizations, or those of the publisher, the editors and the reviewers. Any product that may be evaluated in this article, or claim that may be made by its manufacturer, is not guaranteed or endorsed by the publisher.
References
Abney, M., Sanders, J., and Perry, J. (2018). A discussion of integrated life support and in situ resource utilization architectures for Mars surface missions.
Afshinnekoo, E., Scott, R. T., MacKay, M. J., Pariset, E., Cekanaviciute, E., Barker, R., et al. (2020). Fundamental biological features of spaceflight: Advancing the field to enable deep-space exploration. Cell. 183 (5), 1162–1184. doi:10.1016/j.cell.2020.10.050
Alfrey, C. P., Udden, M. M., Leach-Huntoon, C., Driscoll, T., and Pickett, M. H. (1996). Control of red blood cell mass in spaceflight. J. Appl. Physiol. 81 (1), 98–104. doi:10.1152/jappl.1996.81.1.98
Allen, C. C., Graf, J. C., and McKay, D. S. (1994). “Sintering bricks on the moon,” in Engineering, construction, and operations in space IV, 1220–1229.
Axelbaum, R. L. M. J. (2001). Microgravity combustion, fire in fall. Cleveland, OH: NASA Glenn Research Center.
Baldry, M., Gurieff, N., and Keogh, D. (2022). Imagining sustainable human ecosystems with power-to-x in-situ resource utilisation technology. Acta Astronaut. 192, 190–198. doi:10.1016/j.actaastro.2021.12.031
Bassler, J. A., Bodiford, M. P., Hammond, M. S., King, R., Mclemore, C. A., Hall, N. R., et al. (2006). “In situ fabrication and repair (ISFR) technologies; new challenges for exploration,” in 44th AIAA Aerospace Sciences Meeting and Exhibit, Reno, Nevada, January 09, 2006–January 12, 2006. doi:10.2514/6.2006-350
Bechini, M., Bechini, N., Brandonisio, A., Lavagna, M., and Scapigliati, G. (2021). “Oxygen harvesting from eukaryotic green algae cultivation on moon’s surface,” in 72nd International Astronautical Congress, Dubai, United Arab Emirates, October 25–29, 2021.
Belz, S., Buchert, M., Bretschneider, J., Nathanson, E., and Fasoulas, S. (2014). Physicochemical and biological technologies for future exploration missions. Acta Astronaut. 101, 170–179. doi:10.1016/j.actaastro.2014.04.023
Beysens, D. (2022). Editorial: Transport phenomena in microgravity. Front. Sp. Technol. 3. doi:10.3389/frspt.2022.1092802
Buonanno, M., De Toledo, S. M., Howell, R. W., and Azzam, E. I. (2015). Low-dose energetic protons induce adaptive and bystander effects that protect human cells against DNA damage caused by a subsequent exposure to energetic iron ions. J. Radiat. Res. 56 (3), 502–508. doi:10.1093/jrr/rrv005
Cao, G., Alessandro, C., Giacomo, F., Gilberto, G., Alessia, M., and Antonella, P. (2021). Process and kit to investigate microgravity effect on animal/vegetable cells under extraterrestrial cultivation conditions and cultivation process thereof to sustain manned space missions. International Patent System. PCT/EP2021/078376.
Cao, G., Concas, A., Corrias, G., Licheri, R., Orru’, R., Pisu, M., et al. (2011). Fabrication process of physical assets for civil and/or industrial structures on the surface of Moon, Mars and/or asteroids. https://hdl.handle.net/11584/91162
Cao, G., Concas, A., Corrias, G., Licheri, R., Orrù, R., and Pisu, M. (2012). A process for the production of useful materials to sustain manned space missions on Mars through in-situ resources utilization. WO2013014606A1. PCT/IB2012/053754.
Caporale, A. G., Palladino, M., De Pascale, S., Duri, L. G., Rouphael, Y., and Adamo, P. (2023). How to make the Lunar and Martian soils suitable for food production - assessing the changes after manure addition and implications for plant growth. J. Environ. Manage 325 (1), 116455. doi:10.1016/j.jenvman.2022.116455
Castillo, M., Moore, J. J., Schowengerdt, F. D., Ayers, R. A., Zhang, X., Umakoshi, M., et al. (2003). Effects of gravity on combustion synthesis of functionally graded biomaterials. Adv. Sp. Res. 32 (2), 265–270. doi:10.1016/s0273-1177(03)90261-4
Charles, J., Bungo, M., and Fortner, G. (1994). “Cardiopulmonary function,” in Space physiology and medicine. 3rd ed (Philadelphia, PA, USA: Lea & Febiger).
Clary, J. L., France, C. S., Lind, K., Shi, R., Alexander, J. S., Richards, J. T., et al. (2022). Development of an inexpensive 3D clinostat and comparison with other microgravity simulators using Mycobacterium marinum. Front. Sp. Technol. 3. doi:10.3389/frspt.2022.1032610
Cockell, C. S., Santomartino, R., Finster, K., Waajen, A. C., Eades, L. J., Moeller, R., et al. (2020). Space station biomining experiment demonstrates rare Earth element extraction in microgravity and Mars gravity. Nat. Commun. 11 (1), 5523. doi:10.1038/s41467-020-19276-w
Concas, A., Corrias, G., Orrù, R., Licheri, R., Pisu, M., and Cao, G. (2012). Remarks on ISRU and ISFR technologies for manned missions on moon and Mars. Eurasian Chem. J. 14 (3), 243–248. doi:10.18321/ectj120
Corrias, G., Licheri, R., Orrù, R., and Cao, G. (2012b). Optimization of the self-propagating high-temperature process for the fabrication in situ of Lunar construction materials. Chem. Eng. J. 193–194, 410–421. doi:10.1016/j.cej.2012.04.032
Corrias, G., Licheri, R., Orrù, R., and Cao, G. (2012a). Self-propagating high-temperature reactions for the fabrication of Lunar and Martian physical assets. Acta Astronaut. 70, 69–76. doi:10.1016/j.actaastro.2011.07.022
Dinarelli, S., Longo, G., Dietler, G., Francioso, A., Mosca, L., Pannitteri, G., et al. (2018). Erythrocyte’s aging in microgravity highlights how environmental stimuli shape metabolism and morphology. Sci. Rep. 8 (1), 5277. doi:10.1038/s41598-018-22870-0
Eichler, A., Hadland, N., Pickett, D., Masaitis, D., Handy, D., Perez, A., et al. (2021). Challenging the agricultural viability of martian regolith simulants. Icarus 354, 114022. doi:10.1016/j.icarus.2020.114022
Faierson, E. J., Logan, K. V., Stewart, B. K., and Hunt, M. P. (2010). Demonstration of concept for fabrication of lunar physical assets utilizing lunar regolith simulant and a geothermite reaction. Acta Astronaut. 67 (1), 38–45. doi:10.1016/j.actaastro.2009.12.006
Fais, G., Manca, A., Bolognesi, F., Borselli, M., Concas, A., Busutti, M., et al. (2022b). Wide range applications of Spirulina: From earth to space missions. Mar. Drugs 20 (5), 299. doi:10.3390/md20050299
Fais, G., Manca, A., Concas, A., Pantaleo, A., and Cao, G. (2022a). A novel process to grow edible microalgae on Mars by exploiting in situ-available resources: Experimental investigation. Acta Astronaut. 201, 454–463. doi:10.1016/j.actaastro.2022.09.058
Fleith, P., Cowley, A., Canals Pou, A., Valle Lozano, A., Frank, R., López Córdoba, P., et al. (2020). In-situ approach for thermal energy storage and thermoelectricity generation on the Moon: Modelling and simulation. Planet Space Sci. 181, 104789. doi:10.1016/j.pss.2019.104789
Garrett-Bakelman, F. E., Darshi, M., Green, S. J., Gur, R. C., Lin, L., Macias, B. R., et al. (2019). The nasa twins study: A multidimensional analysis of a year-long human spaceflight. Science 364(6436), eaau8650–52. doi:10.1126/science.aau8650
Herranz, R., Anken, R., Boonstra, J., Braun, M., Christianen, P. C. M., de Geest, M., et al. (2013). Ground-based facilities for simulation of microgravity: Organism-specific recommendations for their use, and recommended terminology. Astrobiology 13 (1), 1–17. doi:10.1089/ast.2012.0876
Herri, J-M., and Chassefière, E. (2012). Carbon dioxide, argon, nitrogen and methane clathrate hydrates: Thermodynamic modelling, investigation of their stability in Martian atmospheric conditions and variability of methane trapping. Planet Space Sci. 73 (1), 376–386. doi:10.1016/j.pss.2012.07.028
Hlavacek, V., and Puszynski, J. A. (1996). Chemical engineering aspects of advanced ceramic materials. Ind. Eng. Chem. Res. 35 (2), 349–377. doi:10.1021/ie9501034
Hughes-Fulford, M., Tjandrawinata, R., Fitzgerald, J., Gasuad, K., and Gilbertson, V. (1998). Effects of microgravity on osteoblast growth. Gravitational Sp. Biol. Bull. Publ. Am. Soc. Gravitational Sp. Biol. 11 (2), 51–60.
Ivanova, S. M., Morukov, B. V., Labetskaia, O. I., Iarlykova, I. V., Levina, A. A., and Kozinets, G. I. (2006). Morphobiochemical assay of the red blood system in members of the prime crews of the International Space Station. Aviakosmicheskaia i Ekol. meditsina = Aerosp. Environ. Med. 40 (3), 9–15.
Jung, F., Krüger-Genge, A., Waldeck, P., and Küpper, J. H. (2019). Spirulina platensis, a super food? J. Cell. Biotechnol. 5, 43–54. doi:10.3233/jcb-189012
Kaczmarzyk, M., Gawronski, M., and Piatkowski, G. (2018). “Global database of direct solar radiation at the Moon’s surface for lunar engineering purposes,” in E3S web of conferences, 1–12.
Kasiviswanathan, P., Swanner, E. D., Halverson, L. J., and Vijayapalani, P. (2022). Farming on Mars: Treatment of basaltic regolith soil and briny water simulants sustains plant growth. PLoS One 17 (8), e0272209. doi:10.1371/journal.pone.0272209
Kiss, J. Z., Wolverton, C., Wyatt, S. E., Hasenstein, K. H., and van Loon, J. J. W. A. (2019). Comparison of microgravity analogs to spaceflight in studies of plant growth and development. Front. Plant Sci. 10, 3–4. doi:10.3389/fpls.2019.01577
Kunz, H., Quiriarte, H., Simpson, R. J., Ploutz-Snyder, R., McMonigal, K., Sams, C., et al. (2017). Alterations in hematologic indices during long-duration spaceflight. BMC Hematol. 17, 12. doi:10.1186/s12878-017-0083-y
Lau, C., Mukasyan, A., Pelekh, A., and Varma, A. (2001). Mechanistic studies in combustion synthesis of NiAl-TiB2 composites: Effects of gravity. J. Mater Res. 16 (6), 1614–1625. doi:10.1557/jmr.2001.0224
Licheri, R., Orrù, R., Sani, E., Dell’Oro, A., and Cao, G. (2022). Spark plasma sintering and optical characterization of lunar regolith simulant. Acta Astronaut. 201, 164–171. doi:10.1016/j.actaastro.2022.09.016
Locci, A. M., Licheri, R., Orrù, R., Cincotti, A., Cao, G., De Wilde, J., et al. (2006). Low-gravity combustion synthesis: Theoretical analysis of experimental evidences. AIChE J. 52 (11), 3744–3761. doi:10.1002/aic.11006
Locci, A. M., Orrù, R., and Cao, G. (2020). Modeling of Electric Current Assisted Sintering: An extended fluid-like approach for the description of powders rheological behavior. Chem. Eng. Res. Des. 154, 283–302. doi:10.1016/j.cherd.2019.12.001
Manière, C., Durand, L., Weibel, A., Chevallier, G., and Estournès, C. (2016). A sacrificial material approach for spark plasma sintering of complex shapes. Screen. Mater 124, 126–128. doi:10.1016/j.scriptamat.2016.07.006
Manis, C., Manca, A., Murgia, A., Uras, G., Caboni, P., Congiu, T., et al. (2022). Understanding the behaviour of human cell types under simulated microgravity conditions: The case of erythrocytes. Int. J. Mol. Sci. 23 (12), 6876. doi:10.3390/ijms23126876
Martirosyan, K. S., and Luss, D. (2006). “Combustion synthesis of ceramic composites from Lunar soil simulant,” in 37th annual lunar and planetary science conference, 1896.
Mason, L., and Rucker, M. (2019). “Common power and energy storage solutions to support lunar and Mars surface exploration missions,” in Proceedings of the international astronautical congress (Washington, DC: International Astronautical Congress (IAC)).
Medda, E., Orrù, R., Cao, G., Fry, J., and Guignè, J. Y. Z. M. (2001). Effects of microgravity on high-temperature self-propagating reactions. Proc. 1st Int. Symp. Microgravity Res. Phys. Sci. Biotechnol. Esa Sp-454, 299.
Merzhanov, A. (2002). SHS processes in microgravity activities: First experiments in space. Adv. Sp. Res. 29, 487–495. doi:10.1016/s0273-1177(01)00646-9
Merzhanov, A. G., and Borovinskaya, I. P. (1972). Self-spreading high-temperature synthesis of refractory inorganic compounds. Dokl. Akad. Nauk. SSSR, Seriya Khimiya. 204 (2), 366–369.
Merzhanov, A. G. (1995). History and recent developments in SHS. Ceram. Int. 21 (5), 371–379. doi:10.1016/0272-8842(95)96211-7
Merzhanov, A. G., Rogachev, A. S., Rumanov, E. N., Sanin, V. N., Sytchev, A. E., Shcherbakov, V. A., et al. (2001). Influence of microgravity on self-propagating high-temperature synthesis of refractory inorganic compounds. Cosm. Res. 39 (2), 210–223. doi:10.1023/a:1017511631494
Merzhanov, A. G., Rogachev, A. S., Sanin, V. N., Scherbakov, V. A., and Sytschev, A. E. Y. V. (1998). “Self-propagating high-temperature synthesis (SHS) under microgravity,” in Joint I pan-pacific basin workshop and IV Japan-China workshop on microgravity. Editor H. Azuma (Japan Soc Microgravity Appl), 119.
Merzhanov, A. G., Sanin, V. N., and Yukhvid, V. I. (2000). On a peculiarity of structure formation in combustion of high-caloric metallothermic compounds under microgravity conditions. Dokl. Phys. 45 (3), 93–96. doi:10.1134/1.171714
Miyazaki, E., and Odawara, O. (2003). SHS technology for in-situ resource utiluzation in space. 12(4), 8505.
MlYAZAKI, E., and Osamu, O. (2002). Effects of microgravity and pressure on combustion synthesis applied to in-situ resource utilization. J. Sp. Technol. Sci. 18 (1), 1_17–21_25. doi:10.11230/jsts.18.1_17
Montague, M., McArthur, G. H., Cockell, C. S., Held, J., Marshall, W., Sherman, L. A., et al. (2012). The role of synthetic biology for in situ resource utilization (ISRU). Astrobiology 12, 1135–1142. doi:10.1089/ast.2012.0829
Moore, J., Zhang, X., Yi, H. C., Guigné, J. Y., and Mannerbino, A. (2004). The application of self-propagating high temperature (combustion) synthesis (SHS) for in-space fabrication and repair.
Mukasyan, A., Lau, C., and Varma, A. (2005). Influence of gravity on combustion synthesis of advanced materials. AIAA J. 43 (2), 225–245. doi:10.2514/1.8972
Mukasyan, A., Pelekh, A., Varma, A. R. A., Rogachev, A., and Jenkins, A. (1997). Effects of gravity on combustion synthesis in heterogeneous gasless systems. AIAA J. 35 (12), 1821–1828. doi:10.2514/3.13757
Mukasyan, A. S., Lau, C., and Varma, A. (2001). Gasless combustion of aluminum particles clad by nickel. Combust. Sci. Technol. 170 (1), 67–85. doi:10.1080/00102200108907850
Mukasyan, A. S., and Pelekh, A. V. A. (1997). Combustion synthesis in gasless systems under microgravity conditions. J. Mater Synth. Process 5 (5), 391–400.
Munir, Z. A., and Anselmi-Tamburini, U. (1989). Self-propagating exothermic reactions: The synthesis of high-temperature materials by combustion. Mater Sci. Rep. 3 (7), 277–365. doi:10.1016/0920-2307(89)90001-7
Nangle, S. N., Wolfson, M. Y., Hartsough, L., Ma, N. J., Mason, C. E., Merighi, M., et al. (2020). The case for biotech on Mars. Nat. Biotechnol. 38, 401–407. doi:10.1038/s41587-020-0485-4
Odawara, O., Mori, K., and Tanji, A. Y. S. (1993). Thermite reaction in a short microgravity environment. J. Mater Synth. Proc. 1, 203.
Odawara, O. (1997). Microgravitational combustion synthesis. Ceram. Int. 23 (3), 273–278. doi:10.1016/s0272-8842(96)00060-0
Palos, M. F., Serra, P., Fereres, S., Stephenson, K., and González-Cinca, R. (2020). Lunar ISRU energy storage and electricity generation. Acta Astronaut. 170, 412–420. doi:10.1016/j.actaastro.2020.02.005
Pantaleo, A., Ferru, E., Pau, M. C., Khadjavi, A., Mandili, G., Mattè, A., et al. (2016). Band 3 erythrocyte membrane protein acts as redox stress sensor leading to its phosphorylation by p72 Syk. Oxid. Med. Cell. Longev. 2016, 1–11. doi:10.1155/2016/6051093
Pizzino, G., Irrera, N., Cucinotta, M., Pallio, G., Mannino, F., Arcoraci, V., et al. (2017). Oxidative stress: Harms and benefits for human health. Oxid. Med. Cell. Longev. 2017, 1–13. doi:10.1155/2017/8416763
Poughon, L., Farges, B., Dussap, C. G., Godia, F., and Lasseur, C. (2009). Simulation of the MELiSSA closed loop system as a tool to define its integration strategy. Adv. Sp. Res. 44 (12), 1392–1403. doi:10.1016/j.asr.2009.07.021
Rapp, D., Karlmann, P. B., Clark, D. L., and Carr, C. M. (1997). Adsorption compressor for acquisition and compression aof atmospheric C02 on Mars.
Rapp, D. (2018). Use of extraterrestrial resources for human space missions to moon or mars. in Use of extraterrestrial resources for human space missions to moon or Mars.
Remigante, A., Morabito, R., and Marino, A. (2021). Band 3 protein function and oxidative stress in erythrocytes. J. Cell. Physiol. 236 (9), 6225–6234. doi:10.1002/jcp.30322
Revellame, E. D., Aguda, R., Chistoserdov, A., Fortela, D. L., Hernandez, R. A., and Zappi, M. E. (2021). Microalgae cultivation for space exploration: Assessing the potential for a new generation of waste to human life-support system for long duration space travel and planetary human habitation. Algal Res. 55, 102258. doi:10.1016/j.algal.2021.102258
Risso, A., Ciana, A., Achilli, C., Antonutto, G., and Minetti, G. (2014). Neocytolysis: None, one or many? A reappraisal and future perspectives. Front. Physiology 5, 54–10. doi:10.3389/fphys.2014.00054
Rizzo, A. M., Corsetto, P. A., Montorfano, G., Milani, S., Zava, S., Tavella, S., et al. (2012). Effects of long-term space flight on erythrocytes and oxidative stress of rodents. PLoS One 7 (3), e32361. doi:10.1371/journal.pone.0032361
Sanders, G. B., and Larson, W. E. (2011). Integration of in-situ Resource Utilization into lunar/Mars exploration through field analogs. Adv. Sp. Res. 47 (1), 20–29. doi:10.1016/j.asr.2010.08.020
Santomartino, R., Zea, L., and Cockell, C. S. (2022). The smallest space miners: Principles of space biomining. Extremophiles 26 (1), 7. doi:10.1007/s00792-021-01253-w
Schcolnik-Cabrera, A., Labastida-Mercado, N., Nancy, D., and Mercado, L. (2014). How is hematology involved in the era of aerospace medicine? Syst. hematological changes astronaut. Vol. 15.
Schimmerling, W. (1995). Space and radiation protection: Scientific requirements for space research. Radiat. Environ. Biophys. 34 (3), 133–137. doi:10.1007/bf01211538
Schlüter, L., and Cowley, A. (2020). Review of techniques for in-situ oxygen extraction on the moon. Planet. Space Sci. 181, 104753. doi:10.1016/j.pss.2019.104753
Serova, L. V., Leon, G. A., Chel’naia, N. A., and Sidorenko, L. A. (1993). The effect of weightlessness on erythrocyte resistance in vivo and in vitro. Aviakosmicheskaia i Ekol. meditsina = Aerosp. Environ. Med. 27 (2), 54–57.
Shteinberg, A. S., Scherbakov, V. A., Martynov, V. V., and Mukhoyan, M. Z. M. A. (1991). Self-propagating high-temperature synthesis of high-porosity materials under zero-g conditions. Dokl. Dokl. Akad. Nauk. SSSR 318 (337).
Spacex. No Title (2023). Spacex. Available at: www.spacex/vehicles/starship/index.html.
Stein, T. P., and Leskiw, M. J. (2000). Oxidant damage during and after spaceflight. Am. J. Physiol. - Endocrinol. Metab. 278 (3 41-3), 375–382. doi:10.1152/ajpendo.2000.278.3.e375
Tanabe, Y., Sakamoto, T., Okada, N., Akatsu, T., Yasuda, E., Takasu, S., et al. (1999). Effect of gravity on titanium carbide foams by self-propagation high-temperature synthesis. J. Mater Res. 14 (4), 1516–1523. doi:10.1557/jmr.1999.0203
Toutanji, H., Glenn-Loper, B., and Schrayshuen, B. (2005). “Strength and durability performance of waterless lunar concrete,” in 43rd AIAA aerospace sciences meeting and exhibit - meeting papers.
Trudel, G., Shahin, N., Ramsay, T., Laneuville, O., and Louati, H. (2022). Hemolysis contributes to anemia during long-duration space flight. Nat. Med. 28 (1), 59–62. doi:10.1038/s41591-021-01637-7
Tsamesidis, I., Egwu, C. O., Pério, P., Augereau, J-M., Benoit-Vical, F., and Reybier, K. (2020). An LC-MS assay to measure superoxide radicals and hydrogen peroxide in the blood system. Metabolites 10 (5), 175. doi:10.3390/metabo10050175
Tucker, D., Ethridge, E., and Toutanji, H. (2006). “Production of glass fibers for reinforcing lunar concrete,” in Collection of technical papers - 44th AIAA aerospace sciences meeting.
Unsworth, B. R., and Lelkes, P. I. (1998). Growing tissues in microgravity. Nat. Med. 4 (8), 901–907. doi:10.1038/nm0898-901
van Loon, J. J. W. A. (2007). Some history and use of the random positioning machine, RPM, in gravity related research. Adv. Sp. Res. 39 (7), 1161–1165. doi:10.1016/j.asr.2007.02.016
Varma, A., Rogachev, A. S., Mukasyan, A. S., and Hwang, S. (1998b). in Combustion synthesis of advanced materials: Principles and applications. Editor J. B. Wei (Academic Press), 79–226.
Varma, A., Rogachev, A. S., Mukasyan, A. S., and Hwang, S. (1998a). Combustion synthesis of advanced materials: Principles and applications. Adv. Chem. Eng.
Verseux, C., Baqué, M., Lehto, K., De Vera, J. P. P., Rothschild, L. J., and Billi, D. (2016). Sustainable life support on Mars - the potential roles of cyanobacteria. Int. J. Astrobiol. 15 (1), 65–92. doi:10.1017/s147355041500021x
Verseux, C., Heinicke, C., Ramalho, T. P., Determann, J., Duckhorn, M., Smagin, M., et al. (2021). A low-pressure, N2/CO2 atmosphere is suitable for cyanobacterium-based life-support systems on mars. Front. Microbiol. 12, 611798. doi:10.3389/fmicb.2021.611798
Wiens, J. H., Bommarito, F., Blumenstein, E. P., Ellsworth, M. E., Cisar, T. E., McKinney, B. C., et al. Water extraction from martian soil. In 2001.
Williams, J. D., Coons, S. C., and Bruckner, A. F. (1995). Design of a water vapor adsorption reactor for martian in situ resource utilization. J. Br. Interplanet. Soc. 48, 347–354.
Wuest, S. L., Richard, S., Kopp, S., Grimm, D., and Egli, M. (2015). Simulated microgravity: Critical review on the use of random positioning machines for mammalian cell culture. BioMed Res. Int. 2015, 1–8. doi:10.1155/2015/971474
Yi, H-C., Varma, A., Rogachev, A. S., and McGinn, P. J. (1996). Gravity-induced microstructural nonuniformities during combustion synthesis of Intermetallic−Ceramic composite materials. Ind. Eng. Chem. Res. 35 (9), 2982–2985. doi:10.1021/ie950750v
Yi, H. C., Woodger, T. C., Moore, J. J., and Guigné, J. Y. (1998). The effect of gravity on the combustion synthesis of metalceramic composites. Metall. Mater Trans. B Process Metall. Mater Process Sci. 29 (4), 889–897. doi:10.1007/s11663-998-0148-1
Keywords: ISRU, ISFR, effect of microgravity, deep space exploration, process development
Citation: Cao G, Concas A, Orrù R, Licheri R, Sani E, Dell’Oro A, Fais G, Manis C, Manca A, Uras G, Caboni P, Locci AM, Cincotti A, Lai N, Congiu T, Faa G, Pisu M, Brelstaff G and Pantaleo A (2023) Recent advances on ISRU technologies and study of microgravity impact on blood cells for deep space exploration. Front. Space Technol. 4:1146461. doi: 10.3389/frspt.2023.1146461
Received: 17 February 2023; Accepted: 14 June 2023;
Published: 19 July 2023.
Edited by:
Antonio Mattia Grande, Polytechnic University of Milan, ItalyReviewed by:
Ivan Troisi, Polytechnic University of Milan, ItalyAdvenit Makaya, European Space Research and Technology Centre (ESTEC), Netherlands
Copyright © 2023 Cao, Concas, Orrù, Licheri, Sani, Dell’Oro, Fais, Manis, Manca, Uras, Caboni, Locci, Cincotti, Lai, Congiu, Faa, Pisu, Brelstaff and Pantaleo. This is an open-access article distributed under the terms of the Creative Commons Attribution License (CC BY). The use, distribution or reproduction in other forums is permitted, provided the original author(s) and the copyright owner(s) are credited and that the original publication in this journal is cited, in accordance with accepted academic practice. No use, distribution or reproduction is permitted which does not comply with these terms.
*Correspondence: Giacomo Cao, Z2lhY29tby5jYW9AdW5pY2EuaXQ=