- 1Space Robotics (SpaceR) Research Group, Interdisciplinary Centre for Security, Reliability and Trust (SnT), University of Luxembourg, Luxembourg, Luxembourg
- 2Spacety EU-Luxembourg, Esch-Sur-Alzette, Luxembourg
Space debris is positioned as a fatal problem for current and future space missions. Many effective space debris removal methods have been proposed in the past decade, and several techniques have been either tested on the ground or in parabolic flight experiments. Nevertheless, no uncooperative debris has been removed from any orbit until this moment. Therefore, to expand this research field and progressing the development of space debris removal technologies, this paper reviews and compares the existing technologies with past, present, and future methods and missions. Moreover, since one of the critical problems when designing space debris removal solutions is how to transfer the energy between the chaser/de-orbiting kit and target during the first interaction, this paper proposes a novel classification approach, named ET-Class (Energy Transfer Class). This classification approach provides an energy-based perspective to the space debris phenomenon by classifying how existing methods dissipate or store energy during the first contact.
1 Introduction
As a result of the existence of humankind in space starting from the last century, the Earth orbits have a crucial space debris pollution problem caused by millions of space debris varying at different geometries and masses (Kessler and Cour-Palais, 1978; Klinkrad and Sdunnus, 1997; Tomizaki et al., 2021). Events such as the collision between Iridium 33 and Cosmos 2,251 satellites and the Chinese anti-satellite missile test on non-functional meteorological satellite Fengyun FY-1C (Butterworth-Heinemann et al., 2010a; Hakima et al., 2018) increased the space debris problem dramatically. This situation constitutes a vital case since operational satellites are in danger of crashing and threatened to be destroyed by space debris (Klinkrad et al., 1995; NASA Safety Standard-Office of Safety and Mission Assurance, 1995; Blagun et al., 1999; Takano et al., 1999; Walker et al., 2001; Wnuk, 2001; Marks, 2008). Moreover, newly planned satellite missions may be interrupted by satellites already placed in different orbital trajectories (Bess, 1975). The Kessler Syndrome states that the number of space debris is exponentially increasing, and eventually, there will not be a chance for a spacecraft to be placed in any orbit in an appropriate manner (Yoshida and Araki, 1994; Vasile, 2015; Drmola and Hubik, 2018; Kawamoto et al., 2020). To tackle this problem, many space industry companies and organizations work to deal with the existing space debris orbiting Earth (Rex, 1998). Space debris removal missions are needed to solve the space debris problem (Alby, 1997). However, the key question is what type of mechanism is needed to tackle this issue? There are various types of space debris having different geometries, masses, velocities, and materials. There is no single space debris removal mechanism that can deal with all types of space debris (New Scientist, 2012; Vasile, 2016). Therefore, classifying space debris removal systems is crucial to get a meaningful insight into the space debris problem before planning any space debris removal mission.
Many space debris removal technologies and missions are still in concept development stages and have not reached the high Technology Readiness Level (TRL) (Mandeville and Alby, 1997; Jehn, 2001; van der Pas et al., 2014). The highest TRL ever reached for space debris removal is TRL-6, which is Level 6 + System Adequacy Validated in Simulated Environment. However, this TRL has been reached only for a few methods. The main reasons for this situation are that space debris removal missions are overly expensive (Okamoto and Yamamoto, 2020) and some space debris removal techniques require Guidance Navigation and Control (GNC) infrastructure, consisting of both hardware and software components, and are extremely advanced (Benvenuto et al., 2015; Rivolta et al., 2019; De Jongh et al., 2020; Song et al., 2020; Marchionne et al., 2021; Silvestrini and Lavagna, 2021). Furthermore, it is also hard to convince sponsors and get funding due to the reasons mentioned above.
To underscore the abovementioned crucial facts and look for novel solutions, the ESA completed a 4-day virtual conference for the space debris problem, namely, the eighth European Conference on Space Debris, between the 20th and 23rd of April 2021. In the conference, several topics were emphasized, such as dramatically increasing AI market, the development of cost-effective and highly advanced computational methods (Bazzocchi et al., 2021; Safety Security, 2022a), and new material types. Space debris is a growing concern for astronauts and satellites as companies launch more missions into space, including mega-constellations (Anttonen et al., 2021) such as the SpaceX’s Starlink project, which now numbers more than 1.400 satellites already in orbit (Order Starlink, 2022).
The space debris problem creates prominent danger for the new-born space tourism market too. Companies such as Blue Origin owned by Jeff Bezos (Blue Origin, 2022), Virgin Galactic owned by Richard Branson (Galactic, 2022), and SpaceX owned by Elon Musk (Spacex, 2022a) plan to use reusable rockets to carry space tourists. Apple co-founder Steve Wozniak is starting a private space company called Privateer (Independent, 2022). The NSR Space Tourism and Travel Markets Report estimates that the space tourism market will generate 7.9 B USD by 2030 (NSR, 2022).
Current classification methods existing in the literature approach the space debris removal problem from the structural point of view, such as the type of mechanism or whether the method is passive or active (Cartmell and McKenzie, 2008; Flores-Abad et al., 2014; Shan et al., 2016; Ellery, 2019; Mark and Kamath, 2019). However, one of the main problems faced when designing space debris removal strategies is how to transfer the energy between the debris (target) and the chaser during the first interaction (Flury, 1994). For example, throughout ADR (Active Space Debris Removal) missions, one should consider that it is possible to push away debris floating in space if a mismatch/misalignment problem occurs during the first interaction. Answering the questions such as how the momentum energy flows between the chaser/de-orbiting kit and target satellites, how much energy needs to be dissipated, or how much energy needs to be stored during the first interaction is critical for the mission’s success.
Therefore, existing debris removal methodologies and missions are summarized throughout this paper. Additionally, a novel space debris removal classification approach, named ET-Class (Energy Transfer Class), is proposed to understand the space debris removal phenomenon in terms of the way the first interaction between the chaser (or the de-orbiting kit) and target occurs, which is of great importance to plan new missions. The practical value of ET-Class is that it allows understanding the trends of space debris removal research and the lacking areas/parts in terms of energy transfer occurring at the first interaction between the chaser satellite/de-orbiting kit and target satellite. There will be more solutions in the future than bringing the debris into the atmosphere and burning, or pushing it to a higher orbit, such as collecting all the debris in a specific orbit and using all of them for recycling purposes.
Companies, institutions, and organizations that intend to conduct future space debris removal missions must be aware of energy distribution and energy transfer mechanisms between the chaser satellite/de-orbiting kit and target satellite during the first interaction, mainly because part of the mission objective is not only capturing/de-orbiting the debris but also to do it in such a way that no more debris is created (because of the rigidity of the space debris, a fracture is highly possible during the first interaction) and minimum energy is consumed for the desired trajectory (Toda and Yasaka, 1993).
This article groups existing debris removal methods under four classes:
• ET1 potential energy dissipation: this class encompasses approaches that focus on the idea of decreasing the potential energy of the debris at the first interaction. The reference point of the potential energy calculation is Earth. While the potential energy of the debris is being dissipated, the debris is getting closer to Earth, which means that it will dive into a denser atmosphere. As a secondary output, the debris’s kinetic energy is also dissipated since it encounters and hits more atmospheric particles. However, this kinetic energy dissipation obtained as a secondary output is caused by the potential energy dissipation of the debris. As mentioned before, the classes defined in ET-Class describe what happens only at the first interaction between the chaser satellite/de-orbiting kit and target satellite in terms of energy transfer. The de-orbiting kit on the target satellite, such as the tether, solar radiation force-based drag sail, or inflated system, decreases the potential energy of the target satellite while pushing into the atmosphere, and due to the friction, the target satellite coupled with the de-orbiting kit is destroyed. One exception, not working as a de-orbiting kit but as a chaser satellite, is the ion-beam shepherd method. An ion-beam projector is integrated on the chaser satellite and applies highly concentrated ions on the target satellite towards the Earth direction so that the target satellite’s potential energy is dissipated.
• ET2 impact energy dissipation: this class focuses on methods that decrease the impact energy of the debris when the first interaction occurs. Much debris has enormous velocities up to 28.100 km/h. Even the chaser satellites with advanced GNC infrastructure are not capable of perfectly aligning with the velocity of the debris. This velocity difference and friction forces produce an impact during the first mechanical interaction, which should be absorbed by the components used in this technology. After the chaser and the target satellites become a single mass, they can either get into the atmosphere and burn or move to outer space. In addition to harpoon and space net mechanisms, there are different types of robotic capturing mechanisms under this category, such as rigid and flexible robotic.
• ET3 neutral energy balance: this class includes methods that do not require dissipating potential/impact energy during the first interaction between the chaser and the target satellites. The only existing method in this class is the magnetic capturing method. The first interaction between the chaser and target satellites is perfectly isolated in terms of energy transfer until they become a single integrated mass. In the market, cooperative magnetic docking mechanisms are being used to achieve this purpose. There will be no energy transfer in theory during the first interaction, only minimized energy transfer in the application. Since the debris is made of ferromagnetic material and the chaser has electromagnet infrastructure, virtual damping and spring effects that can be manipulated by the controller of the electromagnet play a compliance mechanism role. By adjusting the sequence of the positive/negative balancing forces of the electromagnet at high frequency, the impact energy of debris onto the chaser satellite during the first interaction will be minimized, which is of great advantage and the main reason why future missions in the market will use this type of technology.
• ET4 destructive energy absorption: in this class, the target satellite, as debris, is being destroyed by the chaser satellite using destructive tools, such as peripheral laser devices.
The details of the proposed novel classification are given in the following sections. The article is organized as follows: in Section 2, ADR challenges are described. Section 3 reviews state-of-the-art space debris removal methods and classifies them according to the ET-Class. Section 4 reviews and classifies past and future missions. Finally, Section 5 presents the conclusions and the direction of future work.
2 Active Space Debris Removal Challenges
2.1 The Problem
It is estimated by the European Space Agency (ESA) that 130 million objects smaller than 1 cm, 900.000 objects between 1 and 10 cm, and 34.000 objects greater than 10 cm are orbiting Earth up to the enormous speeds of 28.100 km/h (Pontijas Fuentes et al., 2019; Astroscale, 2022). Even though their masses are relatively small, their impact factor is exceptionally high due to the momentum parameter (Bernhard et al., 2001; Smirnov et al., 2010). By now, only 39% of the satellites sent to space are operational (ESA’s Annual Space Environment Report, 2020). To solve this bitter situation, the UN General Assembly COPUOS (Committee on the Peaceful Uses of Outer Space) and the International Telecommunications Union (ITU) suggest following some guidelines for future space missions. They suggest putting efforts to shorten the lifetime of debris or debris removal operation should not create more debris (Krisko et al., 2001; Butterworth-Heinemann et al., 2010b; Martinez and Kendall, 2019; United Nations Office for Outher Space Affairs, 2019). There are advanced mathematical methods to use the remnant propellant of the Jet Propulsion System (JPS) (Golubek et al., 2019), or an even more energy-efficient Electric Rocket Propulsion System (ERPS) (Golubek et al., 2020), for optimal de-orbiting operation. However, the satellites already placed in orbits were not designed to satisfy the abovementioned properties. Consequently, many orbits are crowded with several non-functional satellites and their scattered components (Frey et al., 2017; Liu et al., 2021), where many of them are uncooperative.
This panorama reveals the urgent need for ADR missions to clean the current space debris orbiting Earth. The severity of the space debris pollution problem can be seen from the ESA’s dramatic space debris image given in Figure 1.
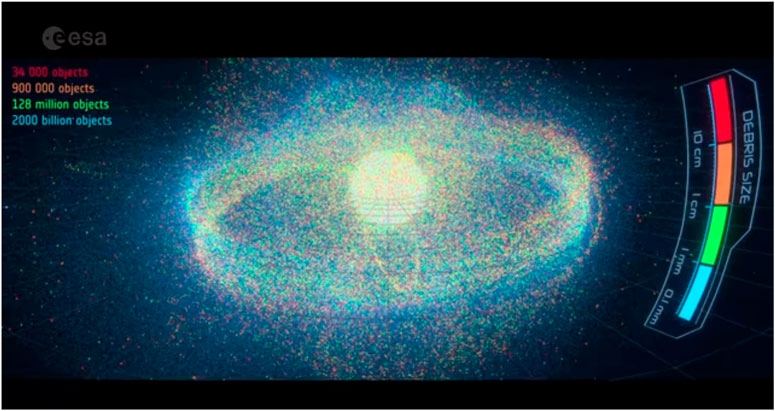
FIGURE 1. ESA’s space debris image showing the varying size of space debris orbiting Earth (ESA’s Annual Space Environment Report, 2020).
One of the recent examples of the seriousness of the space debris problem is the problem experienced by the SpaceX’s Crew-2 mission on 23 April 2021. The mission was a routine transfer of astronauts to the International Space Station (ISS). However, on their way to the ISS, the astronauts of the SpaceX’s Crew-2 mission for the NASA had a shocking experience when a piece of space debris passed unexpectedly very close to the Dragon capsule (Spacex, 2022b).
A similar example occurred in September 2020 to the Expedition 63 crew, which had to temporarily relocate to the Russian part in the ISS when a piece of space debris threatened. A piece from Japan’s H-2A F40 rocket stage came within 1.39 km of the ISS (Wikipedia, 2022). On 12 May 2021, a 35 cm diameter hole was found on Canadarm2. The ISS, which is about the size of a football field, periodically has to adjust its orbit to avoid pieces of space debris. The latest event that affected the ISS was observed on 15 November 2021. The Russian military shot one of its Soviet-era defunct satellites for a missile test, generating more than 1,500 pieces of space debris. This situation forced ISS astronauts to take shelter for 2 hours in capsules to return them to Earth in the collision event. These examples depict the urgent need for debris-free orbits.
2.2 Challenges
Space has a very different environment from Earth’s surface and atmosphere, which is much more severe in terms of radiation, temperature changes, lack of friction, etc. (Koryanov et al., 2021). The zero-g condition of space makes hardware behave differently. Although any hardware must go through verification and validation tests before it is launched to space, this is still not sufficient to assure that it will work in space (Kato, 2001). For many government and commercial space missions, extensive testing, verification, and validation processes of the hardware and software components are required (Flury, 1992; Weisbin et al., 2003; New Scientist, 2008; Dobos and Prazak, 2019; Larsén et al., 2019; Yu et al., 2021a).
Capturing and de-orbiting a large piece of a defunct space object is not a small challenge from technical, legal, and financial points of view. Considering that many targets need to be removed every year, the arising question is how to make these debris removal operations affordable and which organizations or governments would pay for the service (Vitt, 1989; Perek, 1991). The ESA’s artistic image dramatically shows the severity of the space debris problem, as shown in Figure 1. This image again raises the question of whether multiple targets could be removed in a single mission or not. Because of this, ADR technologies should not have to rely on specific characteristics of the debris or interfaces on the debris to accomplish the mission but instead should have the capability to appeal to various types of space debris. Docking with an uncooperative target has been already achieved involving human astronauts (such as in the case of the rescue of Intelsat VI) (ESA’s Annual Space Environment Report, 2020) and autonomously with a cooperative target by the Astroscale’s Elsa-d mission (Astroscale, 2022). However, many other challenges remain.
To remove debris from space, the properties of the debris have crucial importance. There are two types of debris, cooperative and uncooperative. Cooperative debris provides data about its location and mostly has a supporting mechanism for docking or achieving the mechanical interaction between the chaser and target satellites. However, uncooperative debris has neither a supportive mechanism nor provides data to the chaser satellite. This issue constitutes a vital problem for debris removal operations. Moreover, most space debris has various topologies and considerable high angular momentum. One can imagine how hard it is to capture those target satellites when they do not provide data or do not have a supportive mechanism (Isbell and Tedeschi, 1993; Bashurov et al., 1997; Bonnal et al., 2013; Yu et al., 2021b). Luc Piguet, the founder and CEO of ClearSpace, comments “At orbital velocities, even a screw can hit with explosive force, which cannot be shielded against by mission designers. Instead, the threat needs to be managed through the active removal of debris items” (Fernandez, 2017).
Currently, two types of approaches are used to mitigate the space debris problem, active and passive. Active approaches are based on servicing satellites to de-orbit space debris objects. On the other hand, passive approaches are mounted onto the object when its mission starts and activated when it is complete for de-orbiting. Passive approaches will help clean up space in the long run; however, they solely cannot meet the immediate needs for mitigating collision risks (Grundmann et al., 2021).
To sum up, the technologies that come to our lives in this era bring enormous opportunities to tackle the challenges mentioned above. The solution to these challenges will positively contribute to the space industry’s core and side markets shortly. Mainly for companies and institutions working in the ADR topic, tackling those challenges is not an option but an emergent obligation. Thus, concept studies covering these technologies need to be prepared and pushed to high TRL levels, such as TRL-7, which is Level 7 + System Adequacy Validated in Space.
3 Review of State-of-the-Art Methods
Up to now, many methods for space debris removal have been proposed in the literature. According to their characteristics, the methods are divided into several categories, such as harpoon capturing, net capturing, rigid capturing, and flexible capturing. Some methods do not use the mechanic capturing principle to remove the space debris but use other principles, such as inflated, foaming, and tethering. Advantages and drawbacks exist in any of those options, and there is not a single space debris removal method that can deal with all kinds of space debris. The following sections present the most remarkable space debris removal methods that have been developed so far, categorizing them according to the ET-Class proposed in this paper. Figure 2 presents the distribution of the existing approaches under different ET-Classes. The figure provides the highest TRL reached for each method, based on the NASA’s well-known TRL definition (Straub, 2015).
3.1 Methods ET1: Potential Energy Dissipation
Energy Transfer Class 1 includes methods that focus on decreasing the potential energy of the debris. In the state of the art, five methods fit within this category: 1) inflated method, which decreases the potential energy of the debris by manipulating the debris’s ballistic coefficient, 2) tethering method, which uses the ionosphere’s particles to create drag force, 3) foaming method, in which the chaser satellite sprays foam onto the target debris via a nozzle, 4) solar radiation force, which uses the solar radiation to create drag force, and 5) ion-beam shepherd method, which applies an ion beam on the debris and creates drag force. Figure 3 shows examples of these concepts.
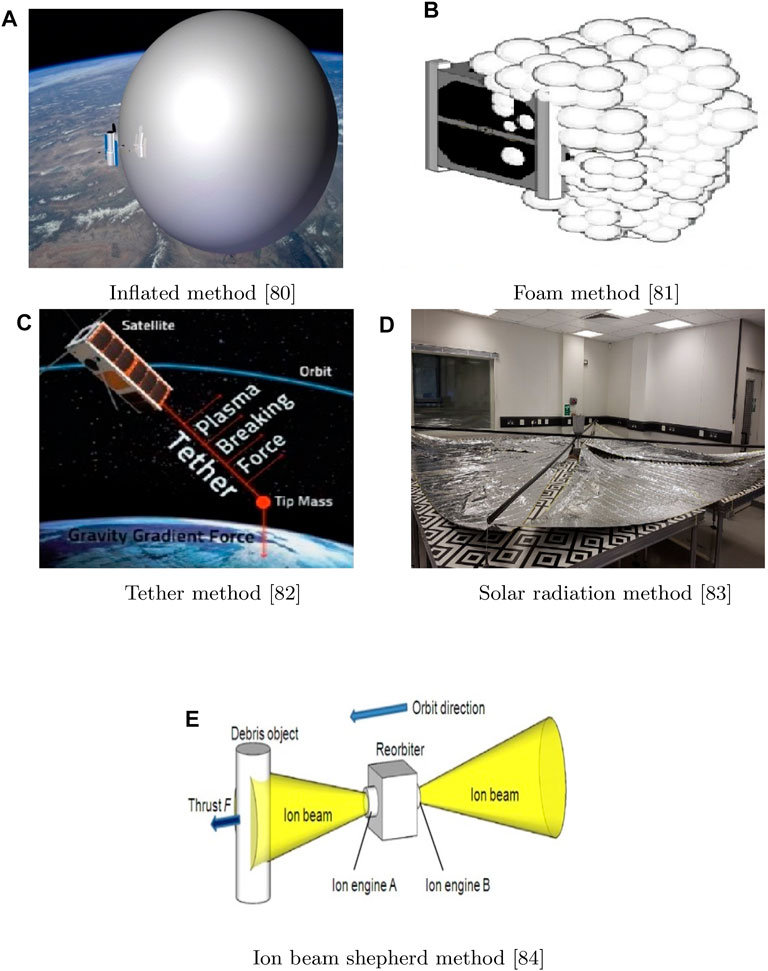
FIGURE 3. Examples of existing ET1 methods: potential energy dissipation. Energy Transfer Class 1 methods focus on decreasing the potential energy of the debris (A) inflated method, (B) foam method, (C) tether method, (D) solar radiation method, and (E) ion-beam shepherd method (Nock et al., 2010; Pergola et al., 2011; Kitamura et al., 2014; Janhunen et al., 2017; Aglietti et al., 2020).
3.1.1 Inflated Method
The idea of the inflated method is to change the ballistic coefficient of the structure so that the gravitational force on the system can easily be manipulated. In the end, the system’s potential energy will be decreased while the pulling force towards Earth will be increased. When the debris enters the atmosphere, the friction will handle the dissipation of the kinetic energy. The most well-known study that researched this topic is the Gossamer Orbit Lowering Device (GOLD) (Nock et al., 2010). The summary of the concept is that the inflated balloon mechanism is integrated into the target satellite before it is launched. When a de-orbiting operation is needed, the envelope is filled with gas. The envelope material is ultra-thin and lightweight. Therefore, even a tiny amount of gas is enough to inflate the balloon. The drawback of this concept is the high risk of the possible damage to the balloon by other space debris having dangerously high momentum (Robinson, 2003), or this balloon-like structure can damage other operational satellites in space since it has no advanced positioning control system. The research conducted for this method was kept in concept development and simulation phases. No inflated method-based mission has been organized yet, and the highest TRL ever reacted for this method is TRL-4.
3.1.2 Tethering Method
Space tethers are essentially used for propulsion purposes in space. These tethers are made of electrical conductor materials that can create a voltage difference between the edges of the tether. There are two common tethering methods in the literature, electrostatic tethering and electrodynamic tethering, and the detailed descriptions of these methods are given in the following subsections. When the tether physically interacts with the charged particles of the ionosphere in LEO, drag force is generated on the tether. Therefore, this principle is used to de-orbit any space object (Hou et al., 2020). The space tethers can be integrated on a spacecraft even before it is launched as an onboard system or attached by a different spacecraft in space. When the tether is spooled out, the debris’s potential energy decreases while the pulling force towards Earth is increased. This pulling force turns the potential energy of the debris into kinetic energy. Space tether systems are varying in terms of many different aspects, such as open-loop or closed-loop control (Sun and Zhong, 2020; Zhu et al., 2021) and rigid or elastic (Zhao et al., 2020; Razzaghi et al., 2021; Shan and Shi, 2021; Hu et al., 2022; Zhang et al., 2022).
Space tethers are very famous and have a wide range of application areas. When firstly proposed, the actual purpose of the electrodynamic tether method was to change the orbit of satellites. The NASA’s Gemini-11 mission demonstrated passive attitude stabilization of the two spacecraft connected by a tether and generated artificial gravity by spinning the integrated spacecraft. In ASI (Italian Space Agency) TSS-1 and ASI TSS-1R missions, it was aimed to generate electrical energy based on the Faraday effect. The ESA’s YES2 mission was focused on testing the usage of tethers as a de-orbiting propulsion system (Messidoro et al., 2017). The tethering applications conducted so far use a de-orbiting kit integrated on the target satellite before it is launched. The tethering process is triggered when the life of the satellite ends. Therefore, the de-orbiting kit and the target satellite start to have interaction. The disadvantage of this approach is that it is not valid for already existing uncooperative targets.
• Electrostatic tether method: the idea behind this tethering method is the creation of electrical potential between the edges of the tether so that its interaction with the charged particles of the ionosphere (Griffith et al., 2009) generates a drag force that pulls the space debris towards Earth. An integrated battery produces the electrical potential, and unlike the electrodynamic tether method, this battery is not charged by the particles of the ionosphere. One of the most advanced works conducted so far is the Finnish Meteorological Institute’s Electrostatic Tether Plasma Brake (Janhunen et al., 2017) in which verification and validation were performed with Aalto-1 satellite (3-U CubeSat). The advantage of the electrostatic tether over the electrodynamic tether is that the open-loop control of the electrostatic tether brings a severe amount of simplicity. The electrodynamic tether is frequently charged by the ionosphere’s particles so that this charge needs to be integrated into the closed-loop structure of the control algorithm. On the contrary, there is no charging requirement for electrostatic tethers. The electrostatic tether is controlled via DC voltage of an integrated battery (Tarabini Castellani et al., 2020). Electrostatic tether systems can be used not only for removing space debris but also for many different purposes, such as planetary exploration (Quadrelli et al., 2017) and space elevators (Bolonkin, 2005). The highest TRL ever reached for this technology is TRL-6.
• Electrodynamic tether method: the advantage of the electrodynamic tether method is that the battery of the electrodynamic tether can be charged several times using the ions located in the ionosphere (Schaub and Sternovsky, 2014; Sanchez-Arriaga and Chen, 2017). So, the battery’s energy can repetitively be used via sophisticated control methodologies, such as sliding mode control or adaptive control approaches (Chen et al., 2020a), to generate extra drag force along the tether. The charging property of the ions cannot be used for electrostatic tethers, and the particles are used to create only the drag force. Compared with electrostatic tethers, electrodynamic tethers can bring the non-functional satellite to Earth faster since the ionosphere charges its battery. The first disadvantage of this method is that it cannot work outside of LEO due to the non-existence of charged particles, i.e., insufficient magnetic intensity (Kawamoto et al., 2006). Numerical simulation is one of the most important aspects when a tether system is designed (Jankovic et al., 2020). However, simulation results cannot solely prove the reliability of the proposed methods. Validation and verification steps in the space environment are needed to show the effectiveness of any approach. In the work of van der Heide and Kruijff, 2001, the tethering method was compared with other alternative methods and advantages and disadvantages were emphasized. The second disadvantage is that the magnitude of the Lorentz force is not always enough to take the debris into the atmosphere in a short period. The third disadvantage is the complexity of deploying a long tether; there is always a chance that the tether may fold. The longer the tether is, the bigger the Lorentz force generated is. However, this long tether can collide easily with any object flying close by, like the ISS orbiting in LEO. The highest TRL ever reached for this technology is TRL-6. To sum up, different approaches in the literature for the electrodynamic tether method vary by material type, tether length, tether spooling mechanism etc., yet the fundamental principle of electrodynamic tether stays unchanged for different studies.
3.1.3 Foaming Method
The foaming method is based on spraying foam onto the target debris and manipulating its ballistic coefficient. The most important advantage of this method is that there is no need for a docking mechanism or de-tumbling the target so that this method is applicable for non-cooperative targets having high angular velocities. While the chaser satellite has proximity rendezvous with space debris, the foaming process is started as follows: the foam is sprayed towards the target satellite (Pergola et al., 2011), the foam ball that is created at the end increases the surface area of the target, the target slows down since its surface area interacts with the atmospheric particles more intensively, the rising area-to-mass ratio of the foam-covered debris generates an atmospheric drag force, the target’s potential energy is initially dissipated because of the enhancement of the friction and pressure of the atmospheric particles that hit towards the increased surface, and after the target is fully covered by the foam and becomes a foam ball, it starts getting into the atmosphere and burns. The contradictory point is that the foam should be stiff enough so that the foam ball will not be destroyed by small pieces of other space debris existing in the same orbit, and new space debris will not be created (Andrenucci et al., 2011). If the foam ball is overly soft, it will not last long. In conclusion, the chemical composition of the foam needs to have a balance between the stiffness and softness spectrum. This issue constitutes the disadvantage of using this method: determining these coefficients for varying space debris types and topology. The foam technology has also different usage areas in space, such as space debris shielding (Rostilov and Ziborov, 2021). No foam-based mission has been organized yet, and the concept has low TRL (Opiela, 2009). The highest TRL ever reached is TRL-4.
3.1.4 Solar Radiation Force Method
The idea behind the solar radiation force method is based on using the solar radiation pressure to create drag force and decrease the altitude, potential energy in other words, of the satellite, as long as the orientation of the surface of the solar sail wings is well aligned, as orthogonal, with the solar array.
Some of the advantages of this method are that solar sails are scalable, which means that it can be applied to a varying range of weight and geometry. Moreover, because of the topology of the principle, the method is easy to model (Borja and Tun, 2006; Johnson et al., 2012; Serfontein et al., 2021), easy to deploy (Lücking et al., 2011; Stiles et al., 2013), and passive (Fernandez et al., 2014; Seefeldt, 2017; Seefeldt et al., 2017). Passiveness of the method brings certain advantages in terms of energy consumption [extra˙73] [extra˙86].
Solar radiation pressure applied onto the wings of the solar sail, which have orthogonality with the solar array, generates a drag force that pushes the satellite towards Earth. Active control of the solar wings is crucially needed to ensure orthogonality throughout the process. While the potential energy of the debris is being dissipated using the solar sail wings, the distance of the debris to Earth is decreasing, which means that the debris will get into a more dense atmosphere. As a secondary output, the debris’s kinetic energy is dissipated too since it encounters atmospheric particles more frequently. The more the potential energy of the debris dissipated, the more the kinetic energy dissipated; this causality creates an infinite loop between the potential and kinetic energy dissipations. Another type of drag force is the atmospheric drag. The atmospheric drag is strictly dependent on the atmosphere’s density, which changes considerably throughout the orbit. Moreover, the atmospheric drag force is also dependent on the geomagnetic effect of the solar activity, which changes along the year.
A solar sail can generate both. However, a solar sail is generally used in higher orbits where there is no or neglectable atmospheric drag, and the atmospheric drag occurs closer to Earth, such as in LEO. The drawback of this method is that it highly depends on the Sun’s orientation. The method is not applicable for the altitude below 750 km because the atmosphere absorbs radiation. One of the most known examples of the solar radiation force method is the RemoveDEBRIS-Drag Sail mission. The method is promising, and advanced research has been conducted since it satisfies many debris removal aspects, such as minimal energy consumption via passive solar sails, compactness, and scalability for different satellite models planned to be launched in future. For space debris removal applications, the highest TRL ever reached with this method is TRL-6, achieved via the RemoveDEBRIS mission. The details of this mission are given in Section 4.
3.1.5 Ion-Beam Shepherd Method
This method is based on applying a plasma ion beam onto the space debris and lowering its altitude (lower potential energy, in other words) over time. The electromechanical model of this method relies on the dynamics of ion actuation (Bombardelli and Pelaez, 2011). Outputs of this method with different ion actuation models are given in the work of Merino et al., 2011, and Merino et al., 2013. Fundamentally, the ion beam acts as a physical link between the chaser and target satellites, and when properly controlled, it works as a stable, spring-like mechanism to transfer the necessary forces on the debris for de-orbiting purposes. During the ion-beam actuation, the relative position stability between the chaser satellite and the debris can be ensured using the
This concept can be applied to non-cooperative and cooperative targets on both LEO and GEO orbits (Bombardelli et al., 2013; Kitamura et al., 2014). Cooperative targets can provide their location to be de-orbited by the chaser satellite, yet this method was developed to find a solution primarily for uncooperative targets. The velocity of ions is exceptionally high, around 38 km/s for xenon ions at 1-kV beam, and a higher power energy source can increase this value. The method is highly space debris topology dependent, and energy consumption requirement is hard to overcome. No ion-beam shepherd mission has been organized yet, and the concept has low TRL. The highest TRL ever reached for this method is TRL-4.
3.2 Methods ET2: Impact Energy Dissipation
Energy Transfer Class 2 includes methods that should decrease the impact energy of the debris when the first contact occurs between the chaser satellite and debris. To reduce this impact energy, GNC infrastructure or compliance mechanisms of the chaser satellite can be used. Four methods fit within this category. 1. The harpoon capturing method creates impact energy on the chaser with a harpoon shot. When the harpoon pierces the debris, mechanical contact that secures the chaser and target satellites creates impact energy on the chaser satellite. 2. The net capturing method: when the net catches the debris, the mechanical connection is secured between the chaser and target satellites so that some amount of the momentum of the debris is accumulated by the chaser satellite, or even be manipulated by the chaser satellite’s propulsion system. 3. The rigid capturing method should have a compliance mechanism that decreases the impact energy of the debris during the capturing phase. The rigid capturing method can be supported with the tentacle design architecture. 4. The flexible capturing method, which softly complies with the debris during the capturing phase and decreases debris’s impact energy with flexible links.
3.2.1 Harpoon Capturing Method
In this method, the chaser satellite deploys a harpoon mechanism to penetrate space debris (Dudziak et al., 2015) (Figure 4A). After the successful shot, the chaser satellite, harpoon, and target become integrated, the satellite and target are connected via an elastic tether, and the chaser satellite will pull the debris to re-enter the atmosphere and burn together or fly to a graveyard orbit. One disadvantage of this method is that it is tough to shoot an object tumbling at a high angular velocity in space. To achieve this, the chaser satellite needs to be supported by an advanced GNC infrastructure to get aligned and close-proximity rendezvous with the debris. The harpoon method is among the promising debris capturing methods, so there is ongoing research on this topic, both in simulation and experiment levels. An uncertain part of the simulation studies conducted for this method is the difficulty of proving the results since the simulation results may not be fully compatible with the experimental results. Furthermore, verification and validation processes of the product in space are needed (Forshaw et al., 2017). Some simulation studies conducted in this field include harpoon modelling (Forshaw et al., 2016), piercing geometry modelling (Pražák, 2021), pulling mechanism modelling, and GNC infrastructure support for harpoon systems (Surrey, 2022). The disadvantage of this method is that an unsuccessful shot may create more space debris depending on the debris material and targeted point, and there is only one chance to shoot (von Kries, 2002). The concept missions using harpoon technology are given in Review Technologies and Missions.
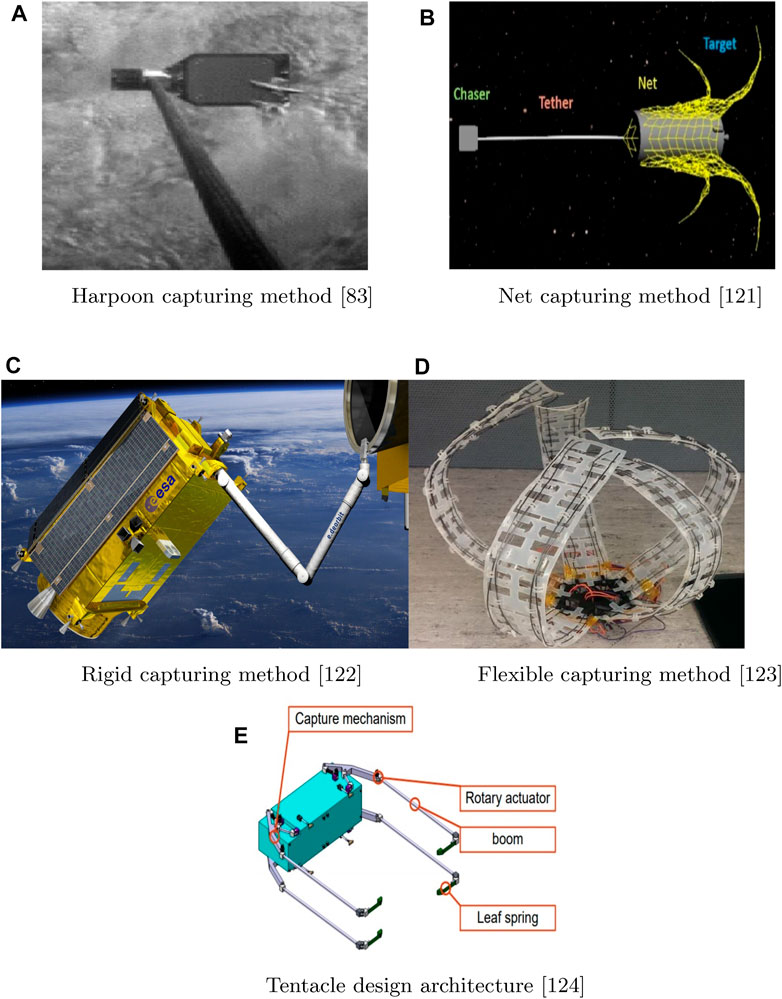
FIGURE 4. Examples of existing ET2 methods: impact energy dissipation. (A) Harpoon capturing method. (B) Net capturing method. (C) Rigid capturing method. (D) Flexible capturing method. (E) Tentacle design architecture (Meyer et al., 2014; Feng et al., 2019; Aglietti et al., 2020; Botta et al., 2020; Safety Security, 2022b).
3.2.2 Net Capturing Method
The net capturing method is based on shooting a flexible net onto the target debris. The net is shot from a chaser satellite by releasing corner masses outward in the target debris’s orientation. Therefore the net wraps the debris. This method has several advantages; for instance, the net capturing method enables shooting from a relatively long distance between chaser satellite and target debris such that close rendezvous and docking operations with the debris are not obligatory, and there is not necessary to de-tumble the target satellite before capturing (Botta et al., 2020). In other words, this method does not require a chaser satellite that has to achieve proximity rendezvous with precision. Moreover, the method is independent of the debris’s geometry, which means that the net can catch space debris in different scales (Bischof et al., 2003; Medina et al., 2017). The most common materials used for net design are Kevlar, Dyneema, Vectran, and Zylon (Zinner et al., 2011; Lavagna et al., 2012; Benvenuto and Carta, 2013). The disadvantage of this method is determining the elasticity of the net’s material and geometry of the net’s topology since these parameters will dramatically affect the first interaction dynamics (Bessonneau and Marichal, 1998; Tibert and Gardsback, 2005; Gao et al., 2012). Another disadvantage of this method is that the net can be shot only once.
In conclusion, net-based capturing methods in the literature are very similar since the main principle is to use a net to capture space debris. The only difference in the proposed studies is the nets’ geometric and material properties. Several concept missions have been developed for net capturing methods, and the research for the applicability of this method is kept active by different institutions. More detailed information about this method and related research are given in Review of Technologies and Missions. The highest TRL ever reached for this method is TRL-6.
3.2.3 Rigid Capturing Method
The rigid capturing method can be evaluated under the impact energy dissipation category since as soon as the mechanical interaction is ensured between the chaser and target satellites, impact energy occurs on the chaser satellite. This operation is needed to achieve robust capturing because both objects are rigid, and any misalignment of the contact between the chaser and debris can push the debris far away from the chaser’s rigid capturing mechanism, especially in case where the object is tumbling at high angular velocity (Wu et al., 2018). Therefore, the rigid capturing method is more applicable for cooperative targets that have a docking port/mechanism (Somov et al., 2019). After completing the capturing phase, the chaser satellite can carry the target satellite anywhere in space, to the atmosphere or an outer orbit. Several studies vary from the simulation to experiment level, conducted to research the applicability of rigid robotic capturing systems for space debris mitigation problems. Any rigid capturing mechanism needs to be lightweight, easy to control, and compatible with the varying topology of space debris (Nishida et al., 2018). In the literature, there are several rigid robotic system designs from single arm to multiple arms (Yan et al., 2020), in which the control strategy of multiple arms to catch the debris is collaborative. Multiple arms are controlled by more sophisticated control system approaches, such as sliding mode control and adaptive control (Wang et al., 2021a), compared with control system approaches for single-arm robotic capture. For single-arm rigid capturing methods, the mostly well-known PID control approach is enough to achieve position and velocity control of the end-effector (Chen et al., 2020b). In some cases of multiple rigid robotic arms usage, one of the arms is used to catch the debris, whereas the other arm is used for manoeuvring purposes and creating rotational and translational movements in space (Yang et al., 2019). On another point, there is ongoing research for autonomy and teleoperation of rigid capturing mechanisms in space (Meng et al., 2018). However, most of these works cannot go beyond the theoretical level since it is tough to realize sophisticated rigid capturing scenarios in frictionless space conditions.
A tentacle-like structure was proposed by the ROGER project of the ESA (Liu et al., 2014). One of the scopes of the ROGER project was to design a rigid capturing system with a tentacle design to get the first interaction with uncooperative space debris in the geostationary orbit. ROGER aimed to create a basic research infrastructure for this capture mechanism. The geostationary orbit is critical, since many commercial and military telecommunication satellites exist in GEO. The goal was to carry the debris to the outer orbit after a successful catch. However, high TRL never reached the project since it was concept research. TRL-4 is achieved at the end of the project.
One problem of rigid capturing methods is to get a robust mechanical interaction with a fully rigid structure in space since rigidness creates a lack of appropriate impact energy dissipation in a friction-free environment. In other words, it is tough to catch a rigid object with a rigid structure in an environment that has no friction (Sallaberger et al., 1997; Rembala and Ower, 2009).
3.2.4 Flexible Capturing Method
The flexible capturing method can be evaluated under the impact energy dissipation category since the capturing mechanisms of this method decrease the impact energy of the debris at the first contact. Several flexible capturing mechanisms fit with the scope of this method, such as shape memory alloy capturing mechanisms or pneumatic capturing mechanisms (Sinatra et al., 2019). Verification and validation processes of pneumatic capturing mechanisms have given satisfying results for on-ground conditions, yet the performance is still questionable for the zero-gravity condition of space. Moreover, the air consumption in space is critical and needs to be limited due to the necessary air consumption of the propulsion system. Therefore, the industry is inclined to use shape memory alloy-based capturing mechanisms as a flexible method in space. The actuation of this flexible capturing system is realized by the electrical voltage difference applied to the shape memory alloy material. The capturing mechanism made of shape memory alloy material fully complies with the debris during the capturing phase and reduces its impact energy. During this coherence, the chaser satellite absorbs the impact energy of the debris. After the full capture is ensured, the debris can be moved into the atmosphere or outer space. The recent advancement of shape memory alloys is becoming a hot topic in the material science discipline. The actuation of the mechanical structures made of this kind of material does not require a considerable high-power consumption. In other words, energy consumption is a critical matter for every space application, so these materials are excellent candidates for space debris capturing mechanisms since the structures that are made of shape memory alloys can easily be re-shaped by temperature or electrical potential gradient (Viscuso et al., 2021). The most advanced study conducted for this topic is MEDUSA. MEDUSA has arms that can grasp any object with flexible structures actuated by an electrical signal. When a simple electrical signal triggers the circuits consisting of nitinol wires of MEDUSA, the arms of MEDUSA start to change their topology and grasp the space debris (Feng et al., 2019). Several experiments were conducted in ground conditions for MEDUSA, and the results were satisfying in terms of robustness of the mechanical interaction. Another advantage of using shape memory alloy material for space applications is that since it can be controlled by either open loop or closed loop very effectively, the computational complexity of the control system is manageable (Lu et al., 2019; Wang et al., 2021b). No flexible capturing method-based mission has been organized yet, and the concept has low TRL, which is TRL-4. However, the method is promising since there is much ongoing research for the flexible capturing method, as given in the abovementioned references.
3.2.5 Other Technologies
In addition to the previously mentioned concepts, some technologies are being used as part of the concepts classified as ET2, such as adhesive material technology or tentacle design architecture. For example, gecko-inspired adhesive pads or voltage-induced electrostatic adhesion props can be assembled on the end-effector of both rigid and flexible capturing mechanisms (DeLuca et al., 2013). The tentacle-like design may consist of multiple joint-link couples with rigid robotic arms or flexible robotic arms. Figure 5 shows examples of robotic grippers with adhesive technology.
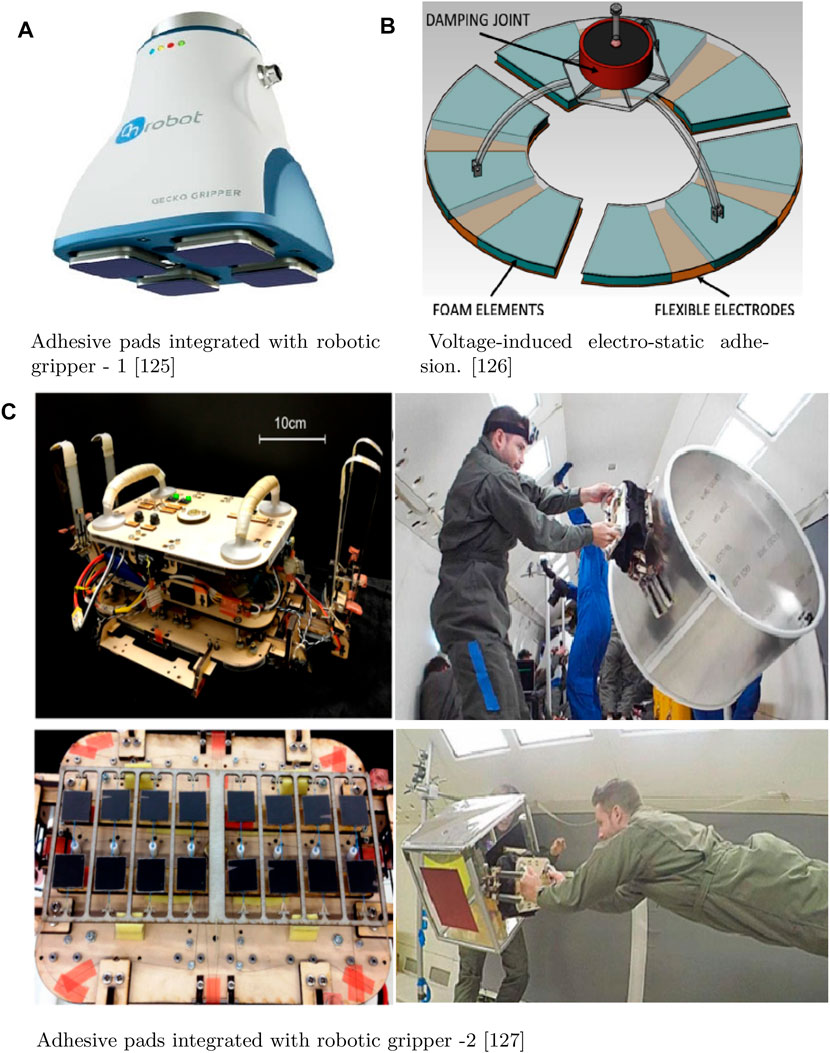
FIGURE 5. Examples of adhesive technology. (A) Adhesive pads integrated with robotic gripper-1. (B) Voltage-induced electrostatic adhesion. (C) Adhesive pads integrated with robotic gripper-2 (DeLuca et al., 2013; Jiang et al., 2017; Onrobot, 2022).
One of the most troublesome tasks in space is to achieve a robust mechanical interaction between the chaser and target satellites. Grasping tasks using robotic arms/grippers are not easy, and the infrastructure needs to be supported by dedicated GNC hardware and software components. In this sense, adhesive materials have been proposed to hit and stick on the surface of the debris and slow down its velocity (Crane, 2017; Zhang et al., 2021). The first adhesive technology for ADR was proposed by Astroscale with the idea of using the technology to reduce the complexity of the mechanical interaction or to de-tumble the debris before the capturing processes are initiated (Astroscale, 2022).
The JPL of the NASA proposed another use of the adhesive technology. They developed a gecko-like adhesive material for uncooperative space debris (Modabberifar and Spenko, 2018; Onrobot, 2022). The tests of this product were conducted in parabolic flights and gave satisfactory results. However, despite the promising results, no mission that uses adhesive material has been organized yet. The technology has low TRL (TRL-4). Nevertheless, the horizon for this technology is very promising since the research of this type of material is ongoing for many different space applications. Moreover, any adhesive material type can be combined and integrated with different space-related material and structure types to synthesize hybrid concepts with higher TRL. For instance, an adhesive material can support shape memory alloys or tentacle-like structures.
3.3 Methods ET3: Neutral Energy Balance
Energy Transfer Class 3 includes methods where the first interaction between the chaser and target satellites is perfectly isolated in terms of energy transfer until they become a single integrated mass. During the first interaction, there is no need of dissipating impact or potential energy.
The only example that has been developed under this category is the magnetic capturing method. In magnetic capture, the first interaction between the capturing system and the debris occurs without changing the potential energy and without an impact energy transfer. Instead, the kinetic energy of both the chaser and the target satellites is balanced to minimize the impact energy; i.e., the electromagnetic coils play a perfect compliance mechanism role, even though it is not a physical compliance mechanism.
3.3.1 Magnetic Capturing Method
When the magnetic coupling is generated between a ferromagnetic object and an electromagnetic coil, virtual spring and damping elements occur between two objects. By controlling the electromagnet using specific control algorithms, the coefficients of the damping and spring elements can be manipulated iteratively, which means that the transferred impact energy can be controlled iteratively (Liu et al., 2018; Yalçın et al., 2018; Yalçın and Erkan, 2021) since the damping is the dissipative energy element. In contrast, the spring is the energy storage element in control theory. The chaser can both push and pull the target, which means that by adjusting the sequence of the push/pull forces at high frequency, the impact of debris onto the chaser satellite will be minimized. One can say that if the chaser pushes the target, the target pushes the chaser as well due to Newton’s well-known third law (when two bodies interact, they apply forces to one another that are equal in magnitude and opposite in direction). However, this opposite reaction force can be compensated by the propulsion system of the chaser satellite. As the name of the category refers to neutral energy balance, the kinetic energy of both chaser and target satellites is balanced to minimize the impact energy. In other words, the electromagnetic coils play a perfect compliance mechanism role, even though it is not a physical compliance mechanism.
The magnetic force is only used for soft capture/soft docking of the debris when the close-proximity operation is achieved. The advantage of this method is the low possibility of crash and fracture since the virtual damping and spring elements generated by the magnetic field create a virtual cushion between two objects. However, the disadvantage of this method is that either debris needs to be cooperative with a ferromagnetic docking port or uncooperative with a ferromagnetic body.
In recent years, magnetic systems have been extensively used in many applications, from clean-room design to robotic capturing. The magnetic system of ELSA-d mission, given in Figure 6, uses the magnetic capturing method since the chaser satellite uses a unique magnetic coil to soft capture/soft docking the debris. ELSA-d consists of two satellites stacked together, a chaser designed to safely remove debris from the orbit and a target satellite that serves as a piece of mock-up debris, launched by GK Launch Services into a 550 km orbit on a Soyuz rocket from the Baikonur Cosmodrome in Kazakhstan on Monday, 22 March 2021, at 6:07 a.m. (UTC) (Astroscale, 2022).
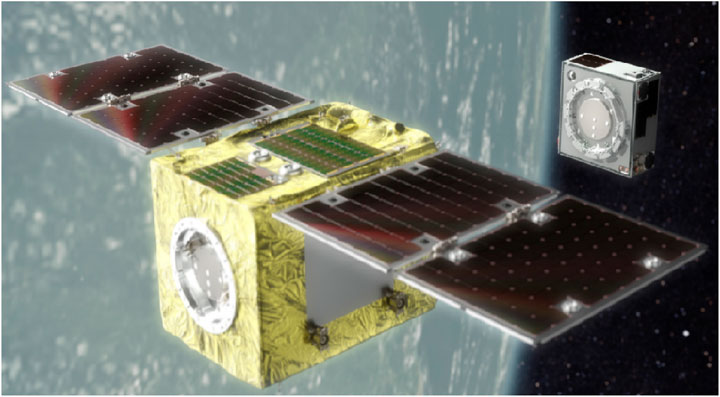
FIGURE 6. Example of existing ET3 methods: neutral energy balance: magnetic capturing method (Astroscale, 2022).
The chaser is equipped with proximity rendezvous technologies and a magnetic docking mechanism, while the target, mimicking a defunct satellite, has a magnetic plate that allows docking. The chaser will repeatedly release and dock with the target in a series of technical demonstrations, proving the capability to find and dock with debris. Demonstrations include target search, target inspection, target rendezvous, and both non-tumbling and tumbling docking. The highest TRL ever reached is TRL-6.
3.4 Methods ET4: Destructive Energy Absorption
Energy Transfer Class 4 includes methods that aim at destroying the debris.
3.4.1 Laser Method
This method aims at destroying small debris targets in case where the laser beam has enough energy (Esmiller et al., 2014). Examples of this method are given in Figure 7. For chaser satellites, one of the main problems in space is how to carry a battery that can produce enough energy to create a laser beam since the weight is a severe constraint for any space application. If the laser beam does not contain enough energy, or the battery is not capable of doing so, then to thoroughly burn the debris, the chaser satellite will change the debris’s kinetic or potential energy depending on the orientation of the laser beam. Therefore, the residual mass will keep on existing in the orbit. However, the industry is inclined to thoroughly burn the debris in space using a laser system for future applications. The State Key Laboratory of Laser Propulsion from China has been developing a space-based laser system, as assembled on a chaser satellite, that is capable of targeting debris whose diameter is up to 20 cm and consisting of mostly aluminium-, carbon-, and iron-based materials (Shen et al., 2014). They claim that, for an aluminium debris particle with a 10 cm diameter, the required laser pulse energy to destroy the debris is 1 kJ.
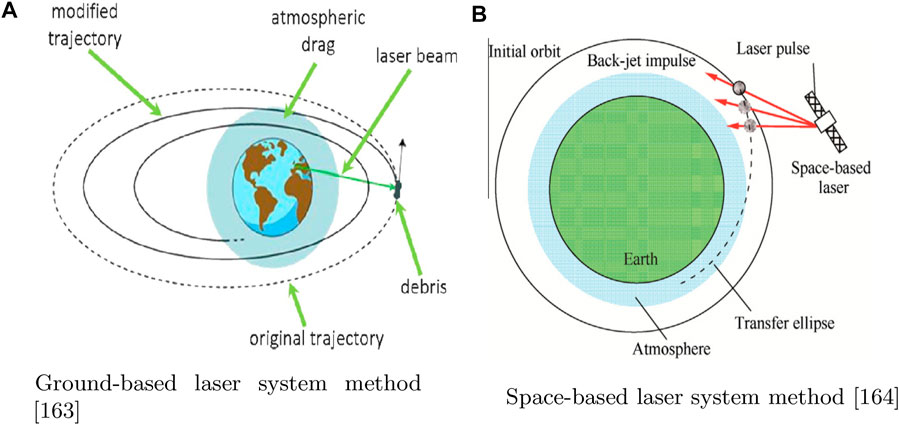
FIGURE 7. Examples of existing ET4 methods: destructive energy absorption. These methods aim at destroying the debris (A) ground-based laser system method and (B) space-based laser system method (Esmiller et al., 2014; Shen et al., 2014).
On the other hand, the current de-orbiting applications of laser focus on changing the orbiting trajectory of the debris by sending a laser beam from an earth-based station. An example of this concept is the Project ORION (Orbital Debris Removal Using Ground-Based Sensors and Lasers) (Phipps et al., 1996; Phipps et al., 1997). In ORION, a ground-based system to shoot the debris is proposed. According to the team, a laser destruction system combined with a space debris detection system can work for a varying distance of space debris, between 1 cm and 500 km.
Ground-based laser methods contain many negative aspects, such as the possible interference of other objects between the laser beam and debris. Additionally, the laser’s energy is not enough to melt the debris if the target is too big. In this case, the debris may be divided into several pieces or change its trajectory in an uncontrolled way. The Australian space company Electro Optic Systems is developing a pair of ground-based lasers to tackle small debris orbiting Earth (Securing Space, 2022). Generally speaking, there are several difficulties to overcome for this method, such as debris topology and material dependency, high energy consumption, or a possible collision/interfere risk with some other object in space. All these are crucial parameters for the chaser satellites orbiting in space. Currently, the technology has low TRL, which is TRL-4, and no laser system method-based mission has been organized yet (Phipps et al., 2019; Peltoniemi et al., 2021).
4 Review of Technologies and Missions
No uncooperative debris has been removed from any orbit yet. However, there are a few missions that push to achieve this goal. The most successful ones of these missions are summarized throughout this section and categorized according to the proposed ET-Class (Figure 8). Most of the missions organized so far focus mainly on the potential and impact energy dissipation classes (Christol, 1994; Rex, 1997; Trur, 2021).
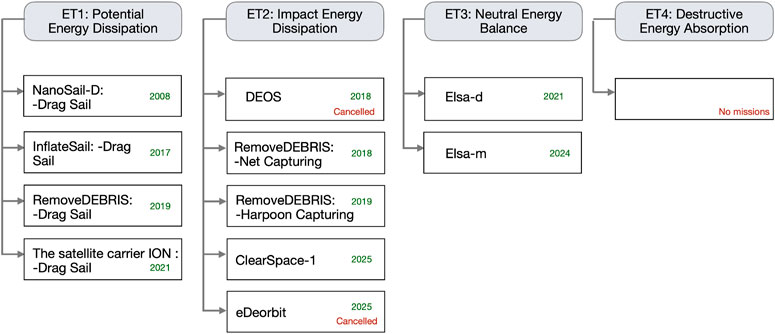
FIGURE 8. Space debris removal past and future missions classified according to the proposed ET-class.
4.1 Missions ET1: Potential Energy Dissipation
Within this section, we present the missions that correspond to ET1. Examples of the concepts are given in Figure 9.
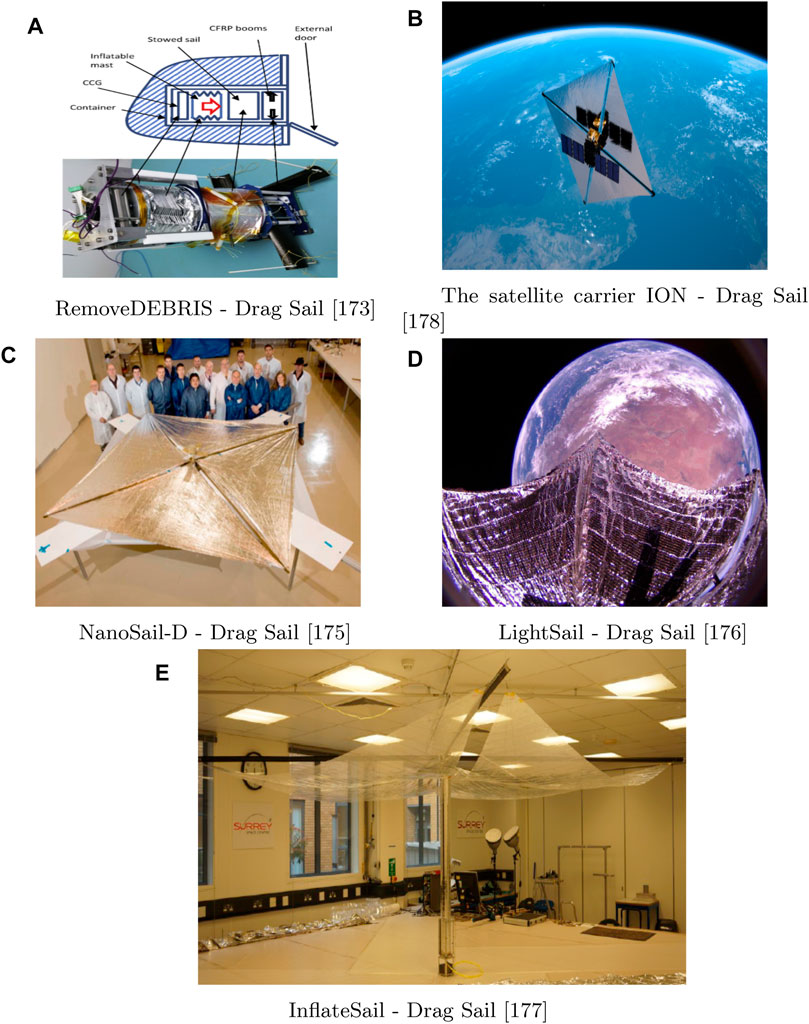
FIGURE 9. Examples of ET1 missions: potential energy dissipation. (A) RemoveDEBRIS-Drag Sail. (B) The satellite carrier ION-Drag Sail. (C) NanoSail-D-Drag Sail. (D) LightSail-Drag Sail. (E) InflateSail-Drag Sail (Johnson et al., 2011; Underwood et al., 2019; Forshaw et al., 2020; Spencer et al., 2021; European Space Agency, 2022).
4.1.1 RemoveDEBRIS-Drag Sail
RemoveDEBRIS was a satellite research and development project to demonstrate different ADR technologies, such as the net, the harpoon, and the drag sail concepts (Aglietti et al., 2020; Forshaw et al., 2020). The Surrey Space Centre led the mission, and the satellite was manufactured by Surrey Satellite Technology Ltd. (SSTL). The platform was equipped with a net, a harpoon, a laser ranging instrument, a drag sail, and two CubeSats. Unique mechanisms were adapted to the platform to validate different ADR technologies. For example, to test the harpoon ADR concept, a target was assembled to the chaser satellite with a link; thus, it did not have a tumbling state, and no close-proximity operation was needed.
After end-to-end testing, the spacecraft was placed in an ISS cargo transfer bag and assembled with the CRS-14 SpaceX Dragon Spacecraft. The Dragon resupply mission with RemoveDEBRIS onboard was launched on April 2, 2018, arriving at the ISS on April 4, 2018. The deployment of the satellite took place on 20 June 2018. The satellite deployed a large sail, and the drag sail brought RemoveDEBRIS from the low orbital altitude of the space station into Earth’s atmosphere to burn.
4.1.2 The Satellite Carrier ION-Drag Sail
In June 2021, an ADEO-N solar sail was mounted on the 220 kg “ION Platform” from D-Orbit (Dorbit, 2022), which was launched on a Falcon9 by SpaceX. The ION Platform is a space vehicle that can transport many satellites in an orbit and release them into distinct orbital points, reducing the time from launch. After 6 months of launch, the ADEO-N will open its 3.5 square metre drag sail, and the spacecraft will start to de-orbit. An in-orbit verification and validation of a bigger ADEO-L drag sail is planned for a future EU mission.
4.1.3 NanoSail-D-Drag Sail
The NanoSail-D mission was launched onboard a Falcon-1 rocket in August 2008. The overall system contained a sail subsystem integrated into a CubeSat-2U for a main CubeSat 1U body. Due to budget limitations, no onboard camera or sensory infrastructure was installed to image the deployed sail or measure the attitude dynamics. Unfortunately, Falcon-1 rocket malfunctioned during the launch, and the NanoSail-D could not be deployed (Johnson et al., 2011). NanoSail-D was not dedicated to showing debris removal properties. Instead, it was planned to test the navigation capabilities of the sail.
4.1.4 LightSail-Drag Sail
The LightSail programme organized by the Planetary Society includes two missions, LightSail-1 and LightSail-2. LightSail-1 was launched on 20 May 2015, and the mission objectives were to test preliminary and straightforward CubeSat functionalities about the solar sail deployment process. The avionics part was assembled into a 1U volume, whereas the solar sail part was integrated into a 2U volume. After the success of the LightSail-1 mission, the LightSail-2 mission was planned to be organized to test more advanced scenarios, such as active control of the solar sail. The active control was achieved using a single-axis momentum wheel and magnetic torque tools. Compared with the NanoSail-D mission, LightSail missions have sophisticated sensory and actuation infrastructures, such as onboard cameras, momentum, and magnetic actuation parts. The Planetary Society announced that LightSail-2 mission success on 31 July 2019 (Spencer et al., 2021). LightSail-2 was not dedicated to showing debris removal properties. Instead, it was planned to test the navigation capabilities of the sail.
4.1.5 InflateSail-Drag Sail
The InflateSail mission was a preliminary part of the RemoveDEBRIS-Drag Sail mission. The InflateSail mission was organized by the Surrey Space Centre and the Von Karman Institute. The total weight of the 3U CubeSat was 3.2 kg and equipped with a 1 m-long inflatable mast and a 10 m2 drag sail. InflateSail successfully demonstrated the de-orbiting process of a CubeSat in LEO for the first time using European drag sail technologies (Underwood et al., 2019). It was launched at 505 km altitude on 23 June 2017 and de-orbited 72 days after the deployment of the drag sail.
4.2 Missions ET2: Impact Energy Dissipation
The missions classified in the impact energy dissipation category are given in this section. Examples of the concepts explored in the missions are given in Figure 10.
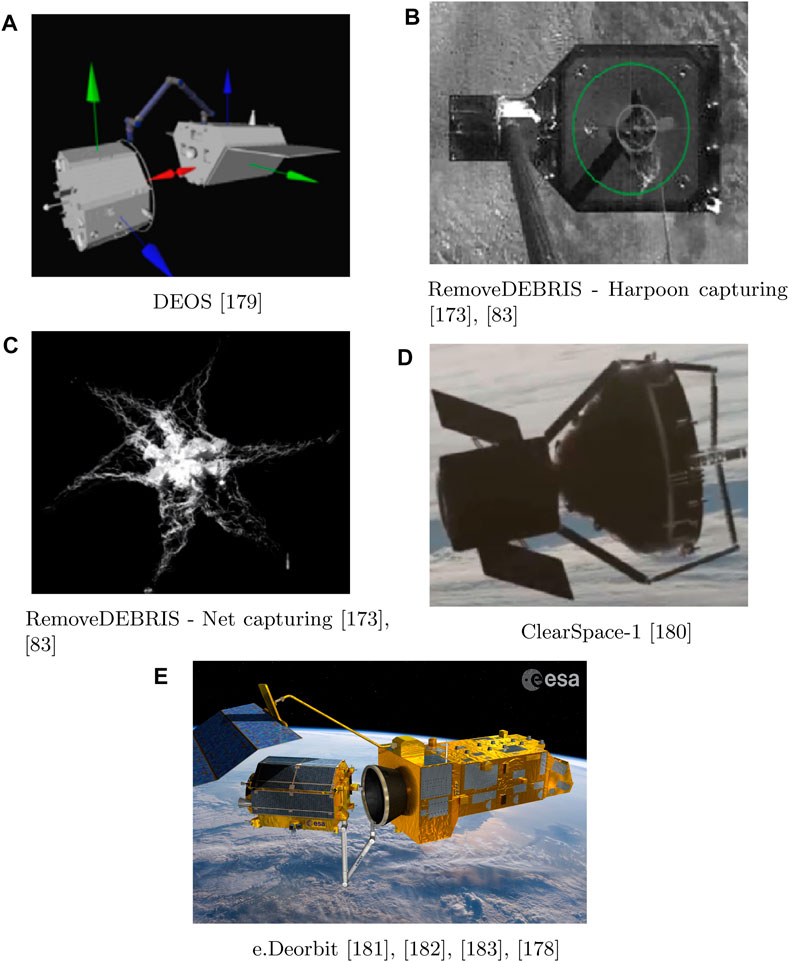
FIGURE 10. Examples of ET2 missions: impact energy dissipation. (A) DEOS. (B) RemoveDEBRIS-Harpoon capturing. (C) RemoveDEBRIS-Net capturing. (D) ClearSpace-1. (E) e.Deorbit (Rank et al., 2011; Cieslak et al., 2019; Henry et al., 2019; Aglietti et al., 2020; Estable et al., 2020; Forshaw et al., 2020; Clear Space, 2022; European Space Agency, 2022).
4.2.1 DEOS
The DEOS (Deutsche Orbitale Servicing) mission can be evaluated under the impact energy dissipation category since the idea was to use a rigid robotic arm to achieve interaction with the target satellite and manipulate it in the orbit (Posch et al., 2012). This operation requires intensive impact energy dissipation at the first contact. DEOS was organized by DLR (German Space Agency). It was a mission to practice conducting tasks, such as maintenance or debris removal. DEOS consisted of two satellites, a client and a servicer. The client acts as the satellite requiring maintenance or removal. The servicer carries out the duty on the client and uses a robotic manipulator controlled remotely (Rank et al., 2011). The two satellites were to be launched together and brought into the orbit at 550 km height. DLR started the DEOS mission in 2012, and three phases were completed: phase 0- concept phase, exploring different systems and mission concepts, phase A- feasibility phase, where the technical aspects and uncertainty risks were researched, and phase B- preliminary design definition phase, where technical requirements of the system were defined. DEOS was expected to be launched in 2018, but the project was cancelled after phase B because of technical difficulties.
4.2.2 RemoveDEBRIS-Net and Harpoon Capturing
RemoveDEBRIS was deployed in June 2018 and focused on demonstrating different ADR technologies.
The Net Demonstration
A CubeSat named DebrisSat 1 deployed a balloon meant to simulate a piece of space debris (Forshaw et al., 2020). From a short distance, approximately 7 m, the RemoveDEBRIS satellite attempted to capture the debris with a net and then move this package to enter Earth’s atmosphere and burn up. Airbus produced the net. On 16 September 2018, it demonstrated the net ability to capture a deployed simulated object. The outputs of this demonstration proved that the net capturing method could effectively catch space debris from a short distance.
The Harpoon Demonstration
On 8 February 2019, SSTL demonstrated the RemoveDEBRIS harpoon mission, fired at 20 m per second speed, penetrating the target extended from the satellite on a 1.5 m rigid boom. The experiment gave satisfactory results in terms of successful space debris piercing (Aglietti et al., 2020). However, it is important to mention that the target was connected to the satellite with a rigid link in the experiment, which means that it was cooperative in terms of position stability. On the other hand, the target consisted of metallic alloys, which opens the question of whether the harpoon method can pierce different materials, such as composite materials. Because of this, more demonstrations are required to analyze the system’s viability to capture targets made of different materials or having different geometries or angular velocities.
4.2.3 e.Deorbit
In 2012, the ESA launched the Clean Space Initiative to tackle the environmental impact of the space debris problem (Cieslak et al., 2019; Henry et al., 2019). Since the beginning, a central focus of Clean Space has been decreasing space debris through “design for destroying.” As time goes by, the ESA Clean Space Initiative has focused on both in-orbit servicing and ADR topics and started to work on the e. Deorbit mission. In the e. Deorbit mission, a 1,600 kg spacecraft was to be launched into an orbit at an altitude of 800 + 1,000 km (LEO). Once in the orbit, the spacecraft would rendezvous with the defunct satellite Envisat which is in an unknown condition, uncooperative, and probably tumbling at high angular velocity.
Within the preparation phase of the e. Deorbit mission, several concepts were analyzed and studied, 1) a robotic arm-based solution that catches the target and 2) a flexible link-based solution (capturing the target using a net or a harpoon). From this point of view, the e. Deorbit mission can be evaluated under the impact energy dissipation category since all methods include impact energy dissipation requirement at the first interaction. The launch was planned for 2025 onboard a Vega launch vehicle. However, the funding of the mission stopped in 2018. Instead, ESA member states now focus on the ClearSpace-1 mission, which is now under the development phase.
4.2.4 ClearSpace-1
In 2019, the ClearSpace start-up was selected by the ESA to lead the first ADR mission by 2025 (Clear Space, 2022). In 2025, ClearSpace will launch the first ADR mission called ClearSpace-1. It will rendezvous, capture, and de-orbit to burn up in the atmosphere the upper part of a Vespa (Vega Secondary Payload Adapter), which was left in a “gradual disposal” orbit (altitude 801 km by 664 km). There will be no de-tumbling or separate docking mechanism. The rigid robotic capturing mechanism will include tentacles for the first mechanical interaction with the space debris. The tentacles will encircle the debris and align with it before dragging it into the lower levels of the atmosphere.
ClearSpace-1’s Vespa target is 112 kg (classified as a small satellite). Its compact shape and homogeneous geometry make it an appropriate first step before progressing to larger, more advanced captures by the subsequent missions. The future goal of the ClearSpace-1 mission is to achieve multiple capturing operations in a single mission. The ClearSpace-1 mission will be launched into a 500 km LEO for initial feature tests before moving to the target orbit for rendezvous and capture. In the end, the chaser combined with the Vespa target will de-orbit and burn in the atmosphere.
4.3 Missions ET3: Neutral Energy Balance
This section presents Elsa-d and Elsa-m missions classified in the neutral energy balance category. Examples of the concepts explored in the missions are shown in Figure 11.
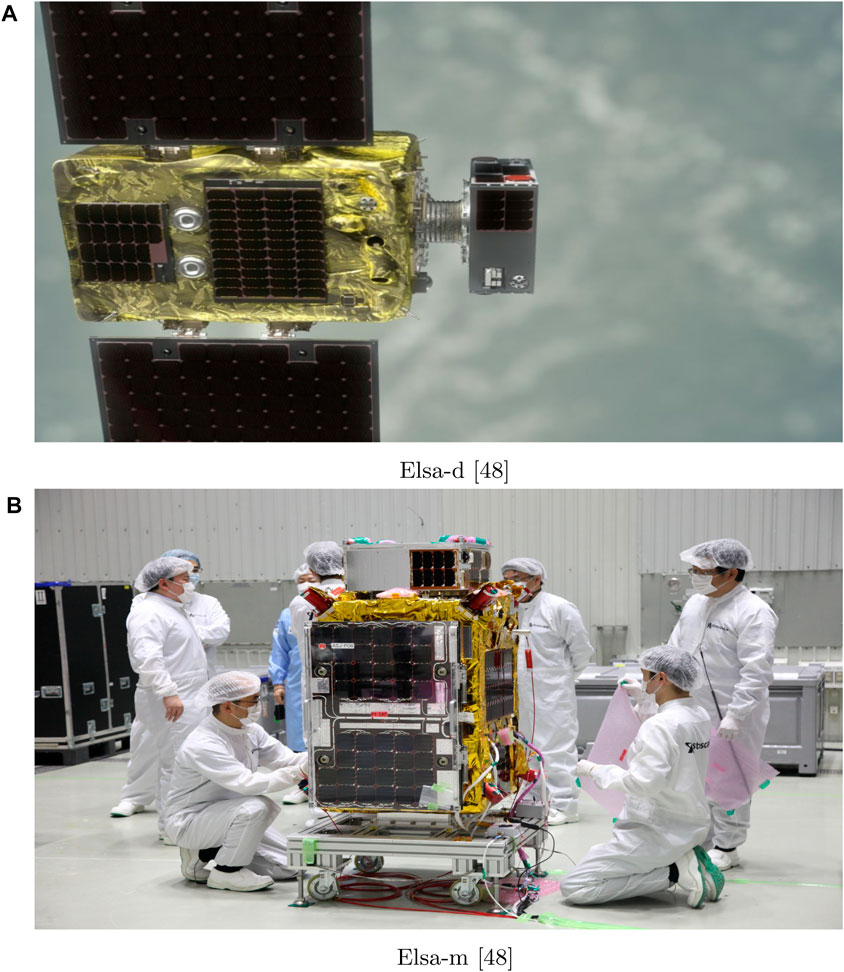
FIGURE 11. Examples of ET3 missions: neutral energy balance. (A) Elsa-d. (B) Elsa-m (Astroscale, 2022).
4.3.1 Elsa-d
The mission will validate an innovative soft-capture/soft-docking mechanism, as well as the CONOPS (Concept of Operations) for capturing and removing non-tumbling and tumbling targets from the orbit. The target satellite has a DP (docking plate) that can be used to interact with the magnetic coil of the chaser satellite. Moreover, the chaser satellite has an autonomy mode, which means that the chaser satellite is able to operate either by itself or receiving commands from the ground. If needed, different algorithms can be uploaded from the ground station to the chaser satellite during the operation phase. The chaser minisatellite’s weight is 180 kg, and the target microsatellite’s weight is 20 kg.
The target satellite incorporates S-band communications, GPS positioning, a 3-axis control system, and a laser retro-reflector. The constellation platform is designed for 5–100 kg range operational missions. It also carries an HD camera and lighting to record the capture sequences during the eclipse in the absence of light. The ELSA-d mission was launched by GK Launch Services into a 550 km orbit on a Soyuz rocket from Kazakhstan on 22 March 2021, at 6:07 a.m. (UTC), and still is in operation phase. The data flow, such as odometry, energy consumption, and trajectory data flows, is being realized between the satellite and ground station. Nevertheless, the data recorded by the embedded devices will be investigated during the post-operation phase after the mission ends. The outputs of the mission have not been shared with the public yet.
4.3.2 Elsa-m
In May 2021, Astroscale signed a £2.5 M agreement for research and development of space debris removal technology innovations with OneWeb (Astroscale, 2022). The communication infrastructure of the Elsa-m mission will be supported by OneWeb and will be based on 5G technology. Within ELSA-m, the technology to tackle multiple retired satellites in a single mission will be verified and validated, and it is claimed that the technology will be ready to use by 2024. This multi-client strategy will reduce launch and operation costs. Each constellation satellite will carry a passive magnetic docking port (DP) consisting of permanent physical magnets. The DP will be used to create a weak magnetic attraction force for the re-assembly of the chaser and target satellites, while the chaser satellite’s magnetic coil system generates a magnetic field. Sophisticated control algorithms can drive the magnetic coil of the chaser satellite to create the appropriate magnetic field patterns. These control algorithms can be uploaded to the chaser satellite from the ground station even when the operation is on. In this way, the chaser satellite will catch multiple satellites in the constellation.
4.4 Missions ET4: Destructive Energy Absorption
In this category, no mission has been organized yet. The concepts presented in Section 3.4 are still in low TRL.
5 Conclusion
The existing taxonomies approach the space debris removal problem from the point of view of the mechanism’s structure, or whether the method is passive or active. However, many existing concepts depend on an effective first interaction between the chaser and target satellites. Finding a way to dissipate and/or store the debris’s potential energy and impact energy is vital to remove debris successfully. The study assists in understanding the SOTA in terms of existing debris removal technologies, their current state (TRL and missions), and on generating awareness on the importance of the first interaction between the chaser satellite and the debris, pointing out how different concepts handle this first interaction.
This paper presented a novel energy-based classification approach for space debris removal concepts and missions, named ET-class. It classifies the existing methods into four categories, according to how the energy between the chaser and target satellites is dissipated at first contact: ET1 potential energy dissipation, ET2 impact energy dissipation, ET3 neutral energy balance, and ET4 destructive energy absorption. The industry is inclined to research and develop different approaches using several technologies, as pointed out throughout the study. The missions classified in neutral energy balance and impact energy dissipation categories are the most recent and promising ones, as explained in the paper. However, varying technologies related to the space industry are under research and development phases, requiring intensive, multidisciplinary perspectives and massive research funds. Moreover, the cost/efficiency curve complicates the situation in finding concrete research direction and appropriate funds to investigate several technology fields. Companies, institutions, and organizations have difficulties having the budget to flourish their research on new technologies. Thus, the future landscape of space debris removal is still not very clear.
The authors believe that the proposed energy-based ET-Class will give insights to companies, institutions, and organizations for future missions and help to understand what are the critical points for successful space debris removal operations. ET-Class will allow understanding the space debris removal research trends and the missing points in terms of the energy transfer at the first interaction between the chaser/de-orbiting kit and target satellites.
Author Contributions
Conceptualization: BY, CM, MHD, GR, JZ, and MO-M; methodology: BY, CM, MHD, GR, JZ, and MO-M; formal analysis: BY and CM; investigation: BY, CM, and MHD; writing—original draft preparation: BY; writing—review and editing: BY and CM; funding acquisition: GR, JZ, and MO-M; and all authors have read and agreed to the published version of the manuscript.
Funding
This study has been conducted by SnT-SpaceR and Spacety Luxembourg, supported by Luxembourg National Research Fund (FNR) for “High fidELity tEsting eNvironment for Active Space Debris Removal - HELEN”, Project Ref: BRIDGES2021/MS/15836393.
Conflict of Interest
The authors declare that the research was conducted in the absence of any commercial or financial relationships that could be construed as a potential conflict of interest.
Publisher’s Note
All claims expressed in this article are solely those of the authors and do not necessarily represent those of their affiliated organizations, or those of the publisher, the editors, and the reviewers. Any product that may be evaluated in this article, or claim that may be made by its manufacturer, is not guaranteed or endorsed by the publisher.
References
Aglietti, G. S., Taylor, B., Fellowes, S., Salmon, T., Retat, I., Hall, A., et al. (2020). The Active Space Debris Removal mission RemoveDebris. Part 2: In Orbit Operations. Acta Astronautica 168, 310–322. doi:10.1016/j.actaastro.2019.09.001
Alby, F. (1997). CNES Operational Practices for Space Debris Risk Limitation and protectionEnlarging the Scope of Space Applications. Acta Astronautica 40, 283–290. doi:10.1016/S0094-5765(97)85729-3
Alpatov, A., Khoroshylov, S., and Bombardelli, C. (2018). Relative Control of an Ion Beam shepherd Satellite Using the Impulse Compensation Thruster. Acta Astronautica 151, 543–554. doi:10.1016/j.actaastro.2018.06.056
Andrenucci, M., Pergola, P., and Ruggiero, A. (2011). “Active Removal of Space Debris-Expanding Foam Application for Active Debris Removal,” in ESA Final Report ACT-RPT- MAD-ARI- 10–6411,201. Availbale at: https://www.esa.int/gsp/ACT/doc/ARI/ARI7.20Study7.20Report/ACT-RPT-MAD-ARI-10-6411-Pisa-Active_Removal_of_Space_Debris-Foam.pdf.
Anttonen, A., Kiviranta, M., and Höyhtyä, M. (2021). Space Debris Detection over Intersatellite Communication Signals. Acta Astronautica 187, 156–166. doi:10.1016/j.actaastro.2021.06.023
Astroscale (2022). Astroscale. Availbale at: https://www.astroscale.com/(Accessed January 7, 2022).
Bashurov, V. V., Bebenin, G. V., Belov, G. V., Bukharev, Y. N., Zhukov, V. I., Ioilev, A. G., et al. (1997). Experimental Modelling and Numerical Simulation of High- and Hypervelocity Space Debris Impact to Spacecraft Shield protection. Int. J. Impact Eng. 20, 69–78. doi:10.1016/S0734-743X(97)87480-5
Bazzocchi, M. C. F., Sánchez-Lozano, J. M., and Hakima, H. (2021). Fuzzy Multi-Criteria Decision-Making Approach to Prioritization of Space Debris for Removal. Adv. Space Res. 67, 1155–1173. doi:10.1016/j.asr.2020.11.006
Benvenuto, R., and Carta, R. (2013). “Active Debris Removal System Based on Tethered Nets: Experimental Results,” in Proceedings of the 9th Pegasus AIAA Student Conference, Milan, Italy. Availbale at: https://re.public.polimi.it/handle/11311/873358.
Benvenuto, R., Salvi, S., and Lavagna, M. (2015). Dynamics Analysis and GNC Design of Flexible Systems for Space Debris Active Removal. Acta Astronautica 110, 247–265. doi:10.1016/j.actaastro.2015.01.014
Bernhard, R. P., Christiansen, E. L., and Kerr, J. H. (2001). Space Shuttle Meteoroid and Orbital Debris Impact Damage. Int. J. Impact Eng. 26, 33–38. doi:10.1016/S0734-743X(01)00073-2
Bess, T. D. (1975). Mass Distribution of Orbiting Man-Made Space Debris in NASA Technical Note, Technical Note D-8108, 1975. Availbale at: https://ntrs.nasa.gov/citations/19760007896.
Bessonneau, J. S., and Marichal, D. (1998). Study of the Dynamics of Submerged Supple Nets (Applications to Trawls). Ocean Eng. 25, 563–583. doi:10.1016/S0029-8018(97)00035-8
Bischof, B., Kerstein, L., Starke, J., Guenther, H., and Foth, W. P. (2003). “Roger Robotic Geostationary Orbit Restorer,” in 54th International Astronautical Congress of the International Astronautical Federation, the International Academy of Astronautics, and the International Institute of Space Law, Bremen, Germany. doi:10.2514/6.iac-03-iaa.5.2.08
Blagun, V. P., Kulik, S. V., and Lukyashchenko, V. I. (1999). Russian Space agency Activities on the Problem of Technogenic Space Debris. Adv. Space Res. 23, 271–274. doi:10.1016/S0273-1177(99)00013-7
Blue Origin (2022). New Shepard 19th Mission. Availbale at: https://www.blueorigin.com/(Accessed January 7, 2022).
Bolonkin, A. A. (2005). “Space Elevator, Transport System for Space Elevator, and Tether System,” in Non-Rocket Space Launch and Flight (Oxford: Elsevier Science), 1–37. 978-0-08-044731-5. doi:10.1016/B978-008044731-5/50032-9
Bombardelli, C., and Pelaez, J. (2011). Ion Beam Shepherd for Contactless Space Debris Removal. J. Guidance, Control Dyn. 34, 916–920. doi:10.2514/1.51832
Bombardelli, C., Urrutxua, H., Merino, M., Peláez, J., and Ahedo, E. (2013). The Ion Beam shepherd: A New Concept for Asteroid Deflection. Acta Astronautica 90, 98–102. doi:10.1016/j.actaastro.2012.10.019
Bonnal, C., Ruault, J.-M., and Desjean, M.-C. (2013). Active Debris Removal: Recent Progress and Current Trends. Acta Astronautica 85, 51–60. doi:10.1016/j.actaastro.2012.11.009
Borja, J. A., and Tun, D. (2006). Deorbit Process Using Solar Radiation Force. J. Spacecraft Rockets 43, 685–687. doi:10.2514/1.9508
Botta, E. M., Miles, C., and Sharf, I. (2020). Simulation and Tension Control of a Tether-Actuated Closing Mechanism for Net-Based Capture of Space Debris. Acta Astronautica 174, 347–358. doi:10.1016/j.actaastro.2020.04.052
Butterworth-Heinemann (2010). “Draft International Convention on the Removal of Hazardous Space Debris,” in Space Safety Regulations and Standards. Editors N. P. Joseph, and S. J. Ram (Oxford: Butterworth-Heinemann), 449–458. doi:10.1016/B978-1-85617-752-8.10040-6
Butterworth-Heinemann (2010). “UN COPUOS Space Debris Mitigation Guidelines,” in Space Safety Regulations and Standards. Editors N. P. Joseph, and S. J. Ram (Oxford: Butterworth-Heinemann), 475–479. 978-1-85617-752-8. doi:10.1016/B978-1-85617-752-8.10043-1
Cartmell, M. P., and McKenzie, D. J. (2008). A Review of Space Tether Research. Prog. Aerospace Sci. 44, 1–21. doi:10.1016/j.paerosci.2007.08.002
Chen, G., Wang, Y., Wang, Y., Liang, J., Zhang, L., and Pan, G. (2020). Detumbling Strategy Based on Friction Control of Dual-Arm Space Robot for Capturing Tumbling Target. Chin. J. Aeronautics 33, 1093–1106. doi:10.1016/j.cja.2019.04.019
Chen, S., Li, A., Wang, C., and Liu, C. (2020). Adaptive Sliding Mode Control for Deployment of Electro-Dynamic Tether via Limited Tension and Current. Acta Astronautica 177, 842–852. doi:10.1016/j.actaastro.2019.12.025
Christol, C. Q. (1994). Scientific and Legal Aspects of Space Debris. Acta Astronautica 34, 367–383. doi:10.1016/0094-5765(94)90273-9
Cieslak, J., Henry, D., Colmenarejo, P., Branco, J., Santos, N., Serra, P., et al. (2019). “Assessment of a Supervisory Fault-Hiding Scheme in a Classical Guidance, Navigation and Control Setup: the e. Deorbit mission,” in 2019 4th Conference on Control and Fault Tolerant Systems (SysTol), 7–12. doi:10.1109/systol.2019.8864753
Clear Space (2022). Shaping Sustainability beyond Earth. Availbale at: https://clearspace.today/(Accessed January 7, 2022).
Crane, L. (2017). Gecko-like Gripper to Snag Space Trash. New Scientist 235, 12. doi:10.1016/S0262-4079(17)31299-X
De Jongh, W. C., Jordaan, H. W., and Van Daalen, C. E. (2020). Experiment for Pose Estimation of Uncooperative Space Debris Using Stereo Vision. Acta Astronautica 168, 164–173. doi:10.1016/j.actaastro.2019.12.006
DeLuca, L. T., Bernelli, F., Maggi, F., Tadini, P., Pardini, C., Anselmo, L., et al. (2013). Active Space Debris Removal by a Hybrid Propulsion Module. Acta Astronautica 91, 20–33. doi:10.1016/j.actaastro.2013.04.025
Dobos, B., and Prazak, J. (2019). To Clear or to Eliminate? Active Debris Removal Systems as Antisatellite Weapons. Space Policy 47, 217–223. doi:10.1016/j.spacepol.2019.01.007
Dorbit (2022). Leader in Space Logistics and Orbital Transportation Services. Availbale at: https://www.dorbit.space/(Accessed January 7, 2022).
Drmola, J., and Hubik, T. (2018). Kessler Syndrome: System Dynamics Model. Space Policy 44-45, 29–39. doi:10.1016/j.spacepol.2018.03.003
Dudziak, R., Tuttle, S., and Barraclough, S. (2015). Harpoon Technology Development for the Active Removal of Space Debris. Adv. Space Res. 56, 509–527. doi:10.1016/j.asr.2015.04.012
Ellery, A. (2019). Tutorial Review on Space Manipulators for Space Debris Mitigation. Robotics 8, 34. doi:10.3390/robotics8020034
Esa's Annual Space Environment Report (2020). Reference GEN-DB-LOG- 00288-OPS-SD. Availbale at: https://www.esa.com/.
Esmiller, B., Jacquelard, C., Eckel, H.-A., and Wnuk, E. (2014). Space Debris Removal by Ground-Based Lasers: Main Conclusions of the European Project CLEANSPACE. Appl. Opt. 53, I45–I54. doi:10.1364/AO.53.000I45
Estable, S., Pruvost, C., Ferreira, E., Telaar, J., Fruhnert, M., Imhof, C., et al. (2020). Capturing and deorbiting Envisat with an Airbus Spacetug. Results from the ESA e.Deorbit consolidation phase study. J. Space Saf. Eng. 7, 52–66. doi:10.1016/j.jsse.2020.01.003
European Space Agency (2022). ESA Blog Navigator. Availbale at: https://blogs.esa.int/(Accessed January 7, 2022).
Feng, L., Ginsberg, S., Martinez, P., Herdrich, G., Laufer, R., Ehresmann, M., et al. (2019). “MEDUSA-mechanism for Entrapment of Debris Using Shape Memory Alloy,” in 1st ESA NEO and Debris Detection Conference.
Fernandez, J. M. (2017). “Advanced Deployable Shell-Based Composite Booms for Small Satellite Structural Applications Including Solar Sails,” in International Symposium on Solar Sailing-Kyoto. Availbale at: https://ntrs.nasa.gov/citations/20170001569.
Fernandez, J. M., Visagie, L., Schenk, M., Stohlman, O. R., Aglietti, G. S., Lappas, V. J., et al. (2014). Design and Development of a Gossamer Sail System for Deorbiting in Low Earth Orbit. Acta Astronautica 103, 204–225. doi:10.1016/j.actaastro.2014.06.018
Flores-Abad, A., Ma, O., Pham, K., and Ulrich, S. (2014). A Review of Space Robotics Technologies for On-Orbit Servicing. Prog. Aerospace Sci. 68, 1–26. doi:10.1016/j.paerosci.2014.03.002
Flury, W. (1992). Activities on Space Debris in Europe. Acta Astronautica 26, 469–476. doi:10.1016/0094-5765(92)90117-2
Flury, W. (1994). Summary of the First European Conference on Space Debris. Acta Astronautica 34, 25–32. doi:10.1016/0094-5765(94)90239-9
Forshaw, J. L., Aglietti, G. S., Fellowes, S., Salmon, T., Retat, I., Hall, A., et al. (2020). The Active Space Debris Removal mission RemoveDebris. Part 1: From Concept to Launch. Acta Astronautica 168, 293–309. doi:10.1016/j.actaastro.2019.09.002
Forshaw, J. L., Aglietti, G. S., Navarathinam, N., Kadhem, H., Salmon, T., Pisseloup, A., et al. (2016). RemoveDEBRIS: An In-Orbit Active Debris Removal Demonstration mission. Acta Astronautica 127, 448–463. doi:10.1016/j.actaastro.2016.06.018
Forshaw, J. L., Aglietti, G. S., Salmon, T., Retat, I., Roe, M., Burgess, C., et al. (2017). Final Payload Test Results for the RemoveDebris Active Debris Removal mission. Acta Astronautica 138, 326–342. doi:10.1016/j.actaastro.2017.06.003
Frey, S., Lemmens, S., Bastida Virgili, B., Flohrer, T., and Gass, V. (2017). Impact of End-Of-Life Manoeuvres on the Collision Risk in Protected Regions. Acta Astronautica 138, 417–422. doi:10.1016/j.actaastro.2017.06.012
Galactic (2022). Virgin Galactic. Availbale at: https://www.virgingalactic.com/(Accessed January 7, 2022).
Gao, S., Yin, Y., Sun, X., and Sun, Y. (2012). “Dynamic Simulation of Fishing Net Based on Cubic B-Spline Surface,” in AsiaSim 2012 (Berlin, Heidelberg: Springer Berlin Heidelberg), 141–148. doi:10.1007/978-3-642-34387-2_17
Golubek, A., Dron’, M., Dubovik, L., Dreus, A., Kulyk, O., and Khorolskiy, P. (2020). Development of the Combined Method to De-orbit Space Objects Using an Electric Rocket Propulsion System. Eejet 4 (106), 78–87. doi:10.15587/1729-4061.2020.210378
Golubek, M. A., Dubovik, L., Dreus, A., and Heti, K. (2019). Analysis of Ballistic Aspects in the Combined Method for Removing Space Objects from the Near Earth Orbits. Eejet 2 (98), 49–54. doi:10.15587/1729-4061.2019.161778
Griffith, G., and Goka, T. (2009). “The Space Environment,” in Safety Design for Space Systems. Editors G. E Musgrave, M. L. Axel (Skip), and S. Tommaso (Burlington: Butterworth-Heinemann), 7–104. 978-0-7506-8580-1. doi:10.1016/B978-0-7506-8580-1.00002-6
Grundmann, P. J. T., Hillebrandt, M., and Zander, M. (2021). Performance Analysis and mission Applications of a New Solar Sail Concept Based on Crossed Booms with Tip-Deployed Membranes. Adv. Space Res. 67, 2736–2745. doi:10.1016/j.asr.2020.10.001
Hakima, H., Bazzocchi, M. C. F., and Emami, M. R. (2018). A Deorbiter CubeSat for Active Orbital Debris Removal. Adv. Space Res. 61, 2377–2392. doi:10.1016/j.asr.2018.02.021
Henry, D., Cieslak, J., Torres, J. Z., Colmenarejo, P., Branco, J., Santos, N., et al. (2019). “Model-based fault diagnosis and tolerant control: the ESA's e.Deorbit mission,” in 2019 18th European Control Conference (ECC), 4356–4361. doi:10.23919/ECC.2019.8796282
Hou, C., Yang, Y., Yang, Y., Yang, K., Zhang, X., and Lu, J. (2020). Electromagnetic-launch-based Method for Cost-Efficient Space Debris Removal. Open Astron. 29, 94–106. doi:10.1515/astro-2020-0016
Hu, W., Du, F., Zhai, Z., Zhang, F., and Deng, Z. (2022). Symplectic Analysis on Dynamic Behaviors of Tethered Tug-Debris System. Acta Astronautica 192, 182–189. doi:10.1016/j.actaastro.2021.12.028
Independent (2022). Independent. Availbale at: https://www.independent.co.uk/(Accessed January 7, 2022).
Isbell, W. M., and Tedeschi, W. J. (1993). Hypervelocity Research and the Growing Problem of Space Debris. Int. J. Impact Eng. 14, 359–372. doi:10.1016/0734-743X(93)90034-5
Janhunen, P., Toivanen, P., and Envall, E. (2017). “Electrostatic Tether Plasma Brake,” in ESA CleanSat Building Block 15 (BB15) Final Report. Availbale at: https://www.electric-sailing.fi/papers/BB15-LSIversion-with-execsum.pdf.
Jankovic, M., Yüksel, M., Babr, M. M., Letizia, F., and Braun, V. (2020). Space Debris Ontology for ADR Capture Methods Selection. Acta Astronautica 173, 56–68. doi:10.1016/j.actaastro.2020.03.047
Jehn, R. (2001). Comparison of the 1999 Beam-Park experiment Results with Space Debris Models. Adv. Space Res. 28, 1367–1375. doi:10.1016/S0273-1177(01)00419-7
Jiang, H., Hawkes, E. W., Fuller, C., Estrada, M. A., Suresh, S. A., Abcouwer, N., et al. (2017). A Robotic Device Using Gecko-Inspired Adhesives Can Grasp and Manipulate Large Objects in Microgravity. Sci. Robot. 2, 4545. doi:10.1126/scirobotics.aan4545
Johnson, L., Whorton, M., Heaton, A., Pinson, R., Laue, G., and Adams, C. (2011). NanoSail-D: A Solar Sail Demonstration mission. Acta Astronautica 68, 571–575. doi:10.1016/j.actaastro.2010.02.008
Johnson, L., Young, R., Barnes, N., Friedman, L., Lappas, V., and McInnes, C. (2012). Solar Sails: Technology and Demonstration Status. Int. J. Aeronaut. Space Sci. 13, 421–427. doi:10.5139/ijass.2012.13.4.421
Kato, A. (2001). Comparison of National Space Debris Mitigation Standards. Adv. Space Res. 28, 1447–1456. doi:10.1016/S0273-1177(01)00449-5
Kawamoto, S., Makida, T., Sasaki, F., Okawa, Y., and Nishida, S.-i. (2006). Precise Numerical Simulations of Electrodynamic Tethers for an Active Debris Removal System. Acta Astronautica 59, 139–148. doi:10.1016/j.actaastro.2006.02.035
Kawamoto, S., Nagaoka, N., Sato, T., and Hanada, T. (2020). Impact on Collision Probability by post mission Disposal and Active Debris Removal. J. Space Saf. Eng. 7, 178–191. doi:10.1016/j.jsse.2020.07.012
Kessler, D. J., and Cour-Palais, B. G. (1978). Collision Frequency of Artificial Satellites: The Creation of a Debris Belt. J. Geophys. Res. 83, 2637–2646. doi:10.1029/JA083iA06p02637
Kitamura, S., Hayakawa, Y., and Kawamoto, S. (2014). A Reorbiter for Large GEO Debris Objects Using Ion Beam Irradiation. Acta Astronautica 94, 725–735. doi:10.1016/j.actaastro.2013.07.037
Klinkrad, H., Sdunnus, H., and Bendisch, J. (1995). Development Status of the ESA Space Debris Reference Model. Adv. Space ResearchSpace Debris 16, 93–102. doi:10.1016/0273-1177(95)98757-F
Klinkrad, H., and Sdunnus, H. (1997). Concepts and Applications of the MASTER Space Debris Environment Model. Adv. Space Res. 19, 277–280. doi:10.1016/S0273-1177(97)00012-4
Koryanov, V., Toporkov, A., and Pozdnyakov, A. (2021). The Concept of a Long-Term Service Station to Increase the Life Duration of Some Satellites or to Remove Space Debris. J. Space Saf. Eng. 8, 23–28. doi:10.1016/j.jsse.2021.02.002
Krisko, P. H., Johnson, N. L., and Opiela, J. N. (2001). EVOLVE 4.0 Orbital Debris Mitigation Studies. Adv. Space Res. 28, 1385–1390. doi:10.1016/s0273-1177(01)00425-2
Larsén, A. K., Chen, Y., Bruschetta, M., Carli, R., Cenedese, A., Varagnolo, D., et al. (2019). A Computationally Efficient Model Predictive Control Scheme for Space Debris Rendezvous. IFAC-PapersOnLine 52, 103–110. doi:10.1016/j.ifacol.2019.11.077
Lavagna, M., Armellin, R., Bombelli, A., and Benvenuto, R. (2012). “Debris Removal Mechanism Based on Tethered Nets,” in International Symposium on Artificial Intelligence, Robotics and Automation in Space. Availbale at: https://eprints.soton.ac.uk/360808/.
Liu, E., Yan, Y., and Yang, Y. (2021). Analysis and Determination of Capture Area for Space Debris Removal Based on Reachable Domain. Adv. Space Res. 68.3, 1613–1626. doi:10.1016/j.asr.2021.03.017
Liu, J., Cui, N., Shen, F., and Rong, S. (2014). Dynamics of Robotic Geostationary Orbit Restorer System during Deorbiting. IEEE Aerosp. Electron. Syst. Mag. 29, 36–42. doi:10.1109/MAES.2014.130197
Liu, X., Lu, Y., Zhou, Y., and Yin, Y. (2018). Prospects of Using a Permanent Magnetic End Effector to Despin and Detumble an Uncooperative Target. Adv. Space Res. 61, 2147–2158. doi:10.1016/j.asr.2018.01.033
Lu, Y., Xie, Z., Wang, J., Yue, H., Wu, M., and Liu, Y. (2019). A Novel Design of a Parallel Gripper Actuated by a Large-Stroke Shape Memory alloy actuatorInternational Journal of Mechanical Sciences. Int. J. Mech. Sci. 159, 74–80. doi:10.1016/j.ijmecsci.2019.05.041
Lücking, C., Colombo, C., and McInnes, C. R. (2011). “A Passive De-orbiting Strategy for High Altitude Cubesat Missions Using a Deployable Reflective Balloon,” in 8th IAA Symposium on Small Satellites for Earth Observation, Berlin, Germany. Availbale at: https://strathprints.strath,ac.uk/41233/1/McInnes_CR_Pure_A_passive_de_orbiting_strategy_for_high_altitude_CubeSat_missions_using_a_deployable_reflective_balloon_Mar_2011.pdf.
Mandeville, J. C., and Alby, F. (1997). Modelling of Space Debris and Meteoroids. Adv. Space Res. 19, 291–300. doi:10.1016/s0273-1177(97)00016-1
Marchionne, C., Sabatini, M., and Gasbarri, P. (2021). GNC Architecture Solutions for Robust Operations of a Free-Floating Space Manipulator via Image Based Visual Servoing. Acta Astronautica 180, 218–231. doi:10.1016/j.actaastro.2020.11.049
Mark, C. P., and Kamath, S. (2019). Review of Active Space Debris Removal Methods. Space Policy 47, 194–206. doi:10.1016/j.spacepol.2018.12.005
Marks, P. (2008). Network-style Attack Could Reduce the Threat from Space Debris. New Scientist 200, 26. doi:10.1016/S0262-4079(08)62565-8
Martinez, P., and Kendall, D. (2019). UN COPUOS Working Group Reaches Agreement on 21 Guidelines to Promote Space Sustainability. Space Res. Today 204, 10–14. doi:10.1016/j.srt.2019.03.012
Medina, A., Cercós, L., Stefanescu, R. M., Benvenuto, R., Pesce, V., Marcon, M., et al. (2017). Validation Results of Satellite Mock-Up Capturing experiment Using Nets. Acta Astronautica 134, 314–332. doi:10.1016/j.actaastro.2017.02.019
Meng, D., Lu, W., Xu, W., She, Y., Wang, X., Liang, B., et al. (2018). Vibration Suppression Control of Free-Floating Space Robots with Flexible Appendages for Autonomous Target Capturing. Acta Astronautica 151, 904–918. doi:10.1016/j.actaastro.2018.07.044
Merino, E. M., Bombardelli, C., Urrutxua, H., Pelaez, J., and Summerer, L. (2011). “Space Debris Removal with an Ion Beam Shepherd Satellite: Target-Plasma Interaction,” in 47thAIAA/ASME/SAE/ASEEJoint Propulsion Conference Exhibit, San Diego, CA, USA. doi:10.2514/6.2011-6142
Merino, M., Ahedo, E., Bombardelli, C., Urrutxua, H., Pelaez, J., et al. (2013). Ionbeam shepherd Satellite for Space Debris Removal. Prog. Propulsion Phys. 4, 789–802. Availbale at: https://ep2.uc3m.es/assets/docs/pubs/other_contributions_and_patents/meri13b%20–%20Ion%20beam%20shepherd%20for%20sapce%20debris%20removal.pdf.(
Messidoro, A., Marconi, L., Conte, M., Chiesa, A., Gambacciani, G., Maffioli, L., et al. (2017). “Tethers as pulling capture technology for e.deorbit and net/harpoon-based adr missions,” in 7th European Conference on Space Debris.
Meyer, J., Scheper, M., Janovsky, R., Mato, J. V., Taubmann, G., Eguen, J. C., et al. (2014). “Clamping Mechanism-A Tentacles Based Capture Mechanism for Active Debris Removal,” in 65th International Astronautical Congress.
Modabberifar, M., and Spenko, M. (2018). A Shape Memory alloy-actuated Gecko-Inspired Robotic Gripper. Sensors Actuators A: Phys. 276, 76–82. doi:10.1016/j.sna.2018.04.018
Nasa Safety Standard-Office of Safety and Mission Assurance (1995). Guidelines and Assessment Procedures for Limiting Orbital Debris, NASA NSS 1740 (1995) 14. Availbale at: https://ntrs.nasa.gov/citations/19960020946.
New Scientist (2012). Junk Radio Signals Track All Space Debris in One Go. New Scientist 216.2893, 6. doi:10.1016/S0262-4079(12)63036-X
New Scientist (2008). Space Debris to Be Legacy of Space War. New Scientist 198.2653, 23. doi:10.1016/S0262-4079(08)61034-9
Nishida, S. I., Uenaka, D., Matsumoto, R., and Nakatani, S. (2018). Lightweight Robot Arm for Capturing Large Space Debris. J. Electr. Eng. doi:10.17265/2328–2223/2018.05.00410.17265/2328-2223/2018.06.004
Nock, K., Gates, K., Aaron, K., and McRonald, A. (2010). “Gossamer Orbit Lowering Device (GOLD) for Safe and Efficient De-orbit,” in AIAA/AAS Astrodynamics Specialist Conference. doi:10.2514/6.2010-7824
Nsr (2022). NSR. Availbale at: https://www.nsr.com/(Accessed January 7, 2022).
Okamoto, H., and Yamamoto, T. (2020). A Novel Concept of Cost-Effective Active Debris Removal Spacecraft System. J. Space Saf. Eng. 7, 345–350. doi:10.1016/j.jsse.2020.0710.1016/j.jsse.2020.07.014
Onrobot (2022). Onrobot. Availbale at: https://onrobot.com/en/node/35 (Accessed January 7, 2022).
Opiela, J. N. (2009). A Study of the Material Density Distribution of Space Debris. Adv. Space Res. 43, 1058–1064. doi:10.1016/j.asr.2008.12.013
Order Starlink (2022). Order Starlink. Availbale at: https://www.starlink.com/(Accessed January 7, 2022).
Peltoniemi, J. I., Wilkman, O., Gritsevich, M., Poutanen, M., Raja-Halli, A., Näränen, J., et al. (2021). Steering Reflective Space Debris Using Polarised Lasers. Adv. Space Res. 67, 1721–1732. doi:10.1016/j.asr.2021.01.002
Perek, L. (1991). Space Debris and the World Community. Space Policy 7, 9–12. doi:10.1016/0265-9646(91)90041-F
Pergola, P., Ruggiero, A., Andrenucci, M., and Summerer, L. (2011). “Low-thrust Missions for Expanding Foam Space Debris Removal,” in Proceedings of the 32nd International Electric Propulsion Conference, Wiesbaden, Germany. Availbale at: http://electricrocket.org/IEPC/IEPC-2011-126.pdf.
Phipps, C. R., Albrecht, G., Friedman, H., Gavel, D., George, E. V., Murray, J., et al. (1996). ORION: Clearing Near-Earth Space Debris Using a 20-kW, 530-nm, Earth-Based, Repetitively Pulsed Laser. Laser Part. Beams 14, 1–44. doi:10.1017/S0263034600009733
Phipps, C. R., Baker, K. L., Libby, S. B., Liedahl, D. A., Olivier, S. S., Pleasance, L. D., et al. (2019). “A Laser-Optical System to Remove Low Earth Orbit Space Debris,” in 1st ESA NEO and Debris Detection Conference. Availbale at: https://conference.sdo.esoc.esa.int/proceedings/sdc6/paper/29/SDC6-paper29.pdf.
Phipps, C. R., and Reilly, J. P. (1997). “ORION: Clearing Near-Earth Space Debris in Two Years Using a 30-kW Repetitively-Pulsed Laser,” in XI International Symposium on Gas Flow and Chemical Lasers and High-Power Laser Conference. International Society for Optics and Photonics. SPIE. Editors R. H. Denis, and J. B. Howard, Vol. 3092, 728–731. doi:10.1117/12.270174
Pontijas Fuentes, I., Bonetti, D., Letterio, F., Vicario de Miguel, G., Blanco Arnao, G., Palomo, P., et al. (2019). Upgrade of ESA's Debris Risk Assessment and Mitigation Analysis (DRAMA) Tool: Spacecraft Entry Survival Analysis Module. Acta Astronautica 158, 148–160. doi:10.1016/j.actaastro.2017.12.001
Posch, A., Schwientek, A. O., Sommer, J., and Fichter, W. (2012). Comparison of Filter Techniques for Relative State Estimation of In-Orbit Servicing Missions. IFAC Proc. Volumes 45, 35–40. doi:10.3182/20120213-3-in-4034.00009
Pražák, J. (2021). Dual-use Conundrum: Towards the Weaponization of Outer Space? Acta Astronautica 187, 397–405. doi:10.1016/j.actaastro.2020.12.051
Quadrelli, M. B., Ono, M., and Jain, A. (2017). Modeling of Active Tether System Concepts for Planetary Exploration. Acta Astronautica 138, 512–529. doi:10.1016/j.actaastro.2016.11.010
Rank, P., Mühlbauer, Q., Naumann, W., and Landzettel, K. (2011). “DEOS Automation and Robotics Payload,” in 11th Symposium on Advanced Space Technologies in Robotics and Automation, ESA/ESTEC, Noordwijk. Availbale at: http://robotics.estec.esa.int/ASTRA/Astra2011/Presentations/session7204b/01_rank.pdf.
Razzaghi, P., Al Khatib, E., Bakhtiari, S., and Hurmuzlu, Y. (2021). Real Time Control of Tethered Satellite Systems to De-orbit Space Debris. Aerospace Sci. Technol. 109, 106379. doi:10.1016/j.ast.2020.106379
Rembala, R., and Ower, C. (2009). Robotic Assembly and Maintenance of Future Space Stations Based on the ISS mission Operations Experience. Acta Astronautica 65, 912–920. doi:10.1016/j.actaastro.2009.03.064
Rex, D. (1997). Space Debris Mitigation and Space Systems Design. Acta Astronautica 41, 311–316. doi:10.1016/S0094-5765(98)00090-3
Rex, D. (1998). Will Space Run Out of Space? the Orbital Debris Problem and its Mitigation. Space Policy 14, 95–105. doi:10.1016/S0265-9646(98)00004-6
Rivolta, A., Lunghi, P., and Lavagna, M. (2019). GNC & Robotics for on Orbit Servicing with Simulated Vision in the Loop. Acta Astronautica 162, 327–335. doi:10.1016/j.actaastro.2019.06.005
Robinson, E. Y. (2003). Spacecraft for Removal of Space Orbital Debris. US Patent 6,655,637. Availbale at: https://patents.google.com/patent/US6655637B1/en (Accessed December 2, 2003).
Rostilov, T. A., and Ziborov, V. S. (2021). Experimental Study of Shock Wave Structure in Syntactic Foams under High-Velocity Impact. Acta Astronautica 178, 900–907. doi:10.1016/j.actaastro.2020.10.022
Safety Security (2022). 8th European Conference on Space Debris - Links for media. Availbale at: https://www.esa.int/Safety_Security/Space_Debris/8th_European_Conference_on_Space_Debris_-_links_for_media (Accessed January 7, 2022).
Safety Security (2022). Safety Security. Availbale at: https://www.esa.int/Safety_Security (Accessed January 7, 2022).
Sallaberger, C.Space Plan Task Force, Canadian Space Agency (1997). Canadian Space Robotic Activities. Acta Astronautica 41, 239–246. doi:10.1016/S0094-5765(98)00082-4
Sanchez-Arriaga, G., and Chen, X. (2017). “Modeling and Perspectives of Low- Work-Function Electrodynamic Tethers to Deorbit Space Debris,” in 7th European Conference on Space Debris, Darmstadt, Germany. Availbale at: https://conference.sdo.esoc.esa.int/proceedings/sdc7/paper/447.
Schaub, H., and Sternovsky, Z. (2014). Active Space Debris Charging for Contactless Electrostatic Disposal Maneuvers. Adv. Space Res. 53, 110–118. doi:10.1016/j.asr.2013.10.003
Securing Space (2022). Securing Space. Availbale at: https://www.eos-aus.com/space/(Accessed January 7, 2022).
Seefeldt, P. (2017). A Stowing and Deployment Strategy for Large Membrane Space Systems on the Example of Gossamer-1. Adv. Space Res. 60, 1345–1362. doi:10.1016/j.asr.2017.06.006
Seefeldt, P., Spietz, P., Sproewitz, T., Grundmann, J. T., Hillebrandt, M., Hobbie, C., et al. (2017). Gossamer-1: Mission Concept and Technology for a Controlled Deployment of Gossamer Spacecraft. Adv. Space Res. 59, 434–456. doi:10.1016/j.asr.2016.09.022
Serfontein, Z., Kingston, J., Hobbs, S., Holbrough, I. E., and Beck, J. C. (2021). Drag Augmentation Systems for Space Debris Mitigation. Acta Astronautica 188, 278–288. doi:10.1016/j.actaastro.2021.05.038
Shan, M., Guo, J., and Gill, E. (2016). Review and Comparison of Active Space Debris Capturing and Removal Methods. Prog. Aerospace Sci. 80, 18–32. doi:10.1016/j.paerosci.2015.11.001
Shan, M., and Shi, L. (2021). Post-capture Control of a Tumbling Space Debris via Tether Tension. Acta Astronautica 180, 317–327. doi:10.1016/j.actaastro.2020.12.049
Shen, S., Jin, X., and Hao, C. (2014). Cleaning Space Debris with a Space-Based Laser System. Chin. J. Aeronautics 27, 805–811. doi:10.1016/j.cja.2014.05.002
Silvestrini, S., and Lavagna, M. (2021). Neural-aided GNC Reconfiguration Algorithm for Distributed Space System: Development and PIL Test. Adv. Space Res. 67, 1490–1505. doi:10.1016/j.asr.2020.12.014
Sinatra, N. R., Teeple, C. B., Vogt, D. M., Parker, K. K., Gruber, D. F., and Wood, R. J. (2019). Ultragentle Manipulation of Delicate Structures Using a Soft Robotic Gripper. Sci. Robot. 4. doi:10.1126/scirobotics.aax5425
Smirnov, N. N., Kiselev, A. B., Kondratyev, K. A., and Zolkin, S. N. (2010). Impact of Debris Particles on Space Structures Modeling. Acta Astronautica 67, 333–343. doi:10.1016/j.actaastro.2010.03.003
Somov, Y., Butyrin, S., and Somov, S. (2019). Guidance and Control of a Space Robot-Manipulator at Approach and Capturing a Passive Satellite. IFAC-PapersOnLine 52, 538–543. doi:10.1016/j.ifacol.2019.11.299
Song, J., Pirat, C., Gass, V., Xu, G., Zhang, Z., and Zhang, J. (2020). X-ray Pulsar-Based GNC System for Formation Flying in High Earth Orbits. Acta Astronautica 170, 701–711. doi:10.1016/j.actaastro.2020.02.015
Spacex (2022). Spacex. Availbale at: https://www.spacex.com/(Accessed January 7, 2022).
Spacex (2022). Spacex. Availbale at: https://www.spacex.com/updates/crew-2-mission/index.html (Accessed January 7, 2022).
Spencer, D. A., Betts, B., Bellardo, J. M., Diaz, A., Plante, B., and Mansell, J. R. (2021). The LightSail 2 Solar Sailing Technology Demonstration. Adv. Space Res. 67, 2878–2889. doi:10.1016/j.asr.2020.06.029
Stiles, L. A., Schaub, H., Maute, K. K., and Moorer, D. F. (2013). Electrostatically Inflated Gossamer Space Structure Voltage Requirements Due to Orbital Perturbations. Acta Astronautica 84, 109–121. doi:10.1016/j.actaastro.2012.11.007
Straub, J. (2015). In Search of Technology Readiness Level (TRL) 10. Aerospace Sci. Technol. 46, 312–320. doi:10.1016/j.ast.2015.07.007
Sun, X., and Zhong, R. (2020). Tether Attachment point Stabilization of Noncooperative Debris Captured by a Tethered Space System. Acta Astronautica 177, 784–797. doi:10.1016/j.actaastro.2019.12.012
Surrey (2022). Harpoon Successfully Captures Space Debris. Availbale at: https://www.surrey.ac.uk/news/harpoon-successfully-captures-space-debris (Accessed January 7, 2022).
Takano, T., Tajima, T., Satoh, T., and Arimoto, Y. (1999). Space Debris Measurements in Japan. Adv. Space Res. 23, 55–65. doi:10.1016/S0273-1177(98)00230-0
Tarabini Castellani, L., Ortega, A., Giménez, A., Urgoiti, E., Sánchez-Arriaga, G., Borderes-Motta, G., et al. (2020). Low Work-Function Tether Deorbit Kit. J. Space Saf. Eng. 7, 332–339. doi:10.1016/j.jsse.2020.07.001
Tibert, G., and Gardsback, M. (2005). “Space Webs Final Report ESA Advanced Concepts Team Report,” in Report ACT-RPT-MAD-ARI-05-4109a. Availbale at: https://www.esa.int/gsp/ACT/doc/ARI/ARI%20Study%20Report/ACT-RPT-MAD-ARI-05-4109a-SpaceWebs-KTH.pdf.
Toda, S., and Yasaka, T. (1993). Space Debris Studies in Japan. Adv. Space Res. 13, 289–298. doi:10.1016/0273-1177(93)90601-7
Tomizaki, H., Kobayashi, R., Suzuki, M., Karasawa, N., Hasegawa, S., and Makihara, K. (2021). Assessment of Space Debris Collisions against Spacecraft with Deorbit Devices. Adv. Space Res. 67, 1526–1534. doi:10.1016/j.asr.2020.12.018
Trur, A. (2021). Governance Aspects of Space Sustainability: The Role of Epistemic Actors as Enablers of Progress. Acta Astronautica 180, 451–459. doi:10.1016/j.actaastro.2020.10.012
Underwood, C., Viquerat, A., Schenk, M., Taylor, B., Massimiani, C., Duke, R., et al. (2019). InflateSail De-orbit Flight Demonstration Results and Follow-On Drag-Sail Applications. Acta Astronautica 162, 344–358. doi:10.1016/j.actaastro.2019.05.054
United Nations Office for Outher Space Affairs (2019). Space Debris Mitigation Standards Adopted by States and International Organizations. Availbale at: https://www.unoosa.org/.
van der Heide, E. J., and Kruijff, M. (2001). Tethers and Debris Mitigation. Acta Astronautica 48, 503–516. doi:10.1016/S0094-5765(01)00074-1
van der Pas, N., Lousada, J., Terhes, C., Bernabeu, M., and Bauer, W. (2014). Target Selection and Comparison of mission Design for Space Debris Removal by DLR׳s Advanced Study Group. Acta Astronautica 102, 241–248. doi:10.1016/j.actaastro.2014.06.020
Vasile, M. (2015). Preface: Advances in Asteroid and Space Debris Science and Technology - Part 1Advances in Asteroid and Space Debris Science and Technology-Part 1. Adv. Space Res. 56, 365–366. doi:10.1016/j.asr.2015.05.031
Vasile, M. (2016). Preface: Advances in Asteroid and Space Debris Science and Technology - Part 2. Adv. Space Res. 57, 1605–1606. doi:10.1016/j.asr.2016.02.021
Viscuso, S., Gualandris, S., de Ceglia, G., and Visentin, V. (2021). “Shape Memory Alloys for Space Applications,” in Shape Memory Alloy Engineering (Second Edition). Editor A Concilio. Second Edition (Boston: Butterworth-Heinemann), 609–623. doi:10.1016/B978-0-12-819264-1.00018-2
von Kries, W. (2002). The Demise of the ABM Treaty and the Militarization of Outer Space. Space Policy 18, 175–178. doi:10.1016/S0265-9646(02)00016-4
Walker, R., Martin, C. E., Stokes, P. H., Wilkinson, J. E., and Klinkrad, H. (2001). Analysis of the Effectiveness of Space Debris Mitigation Measures Using the delta Model. Adv. Space Res. 28, 1437–1445. doi:10.1016/S0273-1177(01)00445-8
Wang, W., Tang, Y., and Li, C. (2021). Controlling Bending Deformation of a Shape Memory alloy-based Soft Planar Gripper to Grip Deformable Objects. Int. J. Mech. Sci. 193, 106181. doi:10.1016/j.ijmecsci.2020.106181
Wang, X., Shi, L., and Katupitiya, J. (2021). A Strategy to Decelerate and Capture a Spinning Object by a Dual-Arm Space robotAerospace Science and Technology. Aerospace Sci. Technol. 113, 106682. doi:10.1016/j.ast.2021.106682
Weisbin, C. R., Schenker, P. S., Easter, R., and Rodriguez, G. (2003). “Space AI and Robotics-Robotic Colonies,” in Encyclopedia of Physical Science and Technology (Third Edition). Editor A. M. Robert. Third Edition (New York: Academic Press), 397–401. 978-0-12-227410-7. doi:10.1016/B0-12-227410-5/00895-4
Wikipedia (2022). Expedition 63. Availbale at: https://en.wikipedia.org/wiki/Expedition_63 (Accessed January 7, 2022).
Wnuk, E. (2001). Orbital Evolution of Space Debris. Adv. Space Res. 28, 1397–1402. doi:10.1016/S0273-1177(01)00443-4
Wu, S., Mou, F., Liu, Q., and Cheng, J. (2018). Contact Dynamics and Control of a Space Robot Capturing a Tumbling Object. Acta Astronautica 151, 532–542. doi:10.1016/j.actaastro.2018.06.052
Yalçın, B. C., and Erkan, K. (2021). 3-DoF Zero Power Micro Vibration Isolation via Linear Matrix Inequalities Based on H∞ and H2 Control Approaches. Mech. Syst. Signal Process. 153, 107506. doi:10.1016/j.ymssp.2020.107506
Yalçın, B. C., Sever, M., and Erkan, K. (2018). Observer-based H2 Controller Design for a Vibration Isolation Stage Having Hybrid Electromagnets. J. Low Frequency Noise, Vibration Active Control. 37 (4), 1134–1150. doi:10.1177/1461348418782170
Yan, L., Xu, W., Hu, Z., and Liang, B. (2020). Multi-objective Configuration Optimization for Coordinated Capture of Dual-Arm Space Robot. Acta Astronautica 167, 189–200. doi:10.1016/j.actaastro.2019.11.002
Yang, S., Wen, H., and Jin, D. (2019). Trajectory Planning of Dual-Arm Space Robots for Target Capturing and Base Manoeuvring. Acta Astronautica 164, 142–151. doi:10.1016/j.actaastro.2019.08.004
Yoshida, H., and Araki, M. (1994). Social Impact of Space Debris. Acta Astronautica 34, 345–355. doi:10.1016/0094-5765(94)90271-2
Yu, Y., Yang, F., Yue, H., Lu, Y., Li, S., and Zhao, H. (2021). Prospects of De-tumbling Large Space Debris Using a Two-Satellite Electromagnetic Formation. Adv. Space Res. 67, 1816–1829. doi:10.1016/j.asr.2020.12.039
Yu, Y., Yang, F., Yue, H., Lu, Y., Li, S., and Zhao, H. (2021). Prospects of De-tumbling Large Space Debris Using a Two-Satellite Electromagnetic Formation. Adv. Space Res. 67, 1816–1829. doi:10.1016/j.asr.2020.12.039
Zhang, G., Zhang, Q., Feng, Z., Chen, Q., and Yang, T. (2021). Dynamic Modeling and Simulation of a Novel Mechanism for Adhesive Capture of Space Debris. Adv. Space Res. 68, 3859–3874. doi:10.1016/j.asr.2021.06.041
Zhang, K., Lu, K., Gu, X., Fu, C., and Zhao, S. (2022). Dynamic Behavior Analysis and Stability Control of Tethered Satellite Formation Deployment. Sensors 22, 62. doi:10.3390/s22010062
Zhao, Y., Zhang, F., and Huang, P. (2020). Capture Dynamics and Control of Tethered Space Net Robot for Space Debris Capturing in Unideal Capture Case. J. Franklin Inst. 357, 12019–12036. doi:10.1016/j.jfranklin.2020.04.037
Zhu, W., Si, J., Pang, Z., and Du, Z. (2021). Rapid Deployment and Continuous Shape Maintenance of Tethered-Space Net Robot Based on Single-Pulse Action. Adv. Space Res. 67, 1477–1489. doi:10.1016/j.asr.2020.12.007
Keywords: space debris removal, space missions, Kessler syndrome, space debris removal method classification, space debris mitigation
Citation: Yalçın BC, Martinez C, Hubert Delisle M, Rodriguez G, Zheng J and Olivares-Mendez M (2022) ET-Class: An Energy Transfer-Based Classification of Space Debris Removal Methods and Missions. Front. Space Technol. 3:792944. doi: 10.3389/frspt.2022.792944
Received: 11 October 2021; Accepted: 12 January 2022;
Published: 02 March 2022.
Edited by:
Alberto Buzzoni, Astrophysics and Space Science Observatory of Bologna (INAF), ItalyReviewed by:
Andrii Dreus, Oles Honchar Dnipropetrovsk National University, UkrainePouria Razzaghi, George Washington University, United States
Copyright © 2022 Yalçın, Martinez, Hubert Delisle, Rodriguez, Zheng and Olivares-Mendez. This is an open-access article distributed under the terms of the Creative Commons Attribution License (CC BY). The use, distribution or reproduction in other forums is permitted, provided the original author(s) and the copyright owner(s) are credited and that the original publication in this journal is cited, in accordance with accepted academic practice. No use, distribution or reproduction is permitted which does not comply with these terms.
*Correspondence: Barış Can Yalçın, YmFyaXNjYW4ueWFsY2luQHVuaS5sdQ==