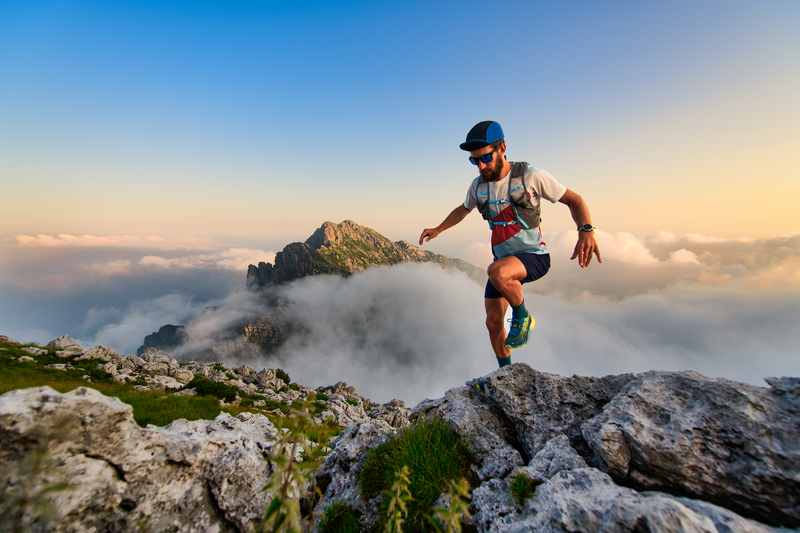
95% of researchers rate our articles as excellent or good
Learn more about the work of our research integrity team to safeguard the quality of each article we publish.
Find out more
REVIEW article
Front. Space Technol. , 07 March 2023
Sec. Space Exploration
Volume 3 - 2022 | https://doi.org/10.3389/frspt.2022.1000788
This article is part of the Research Topic Challenges and Solutions for Operating in Extreme Space Environments View all articles
Nature benefits from a progressive evolution over millions of years, always adapting and finding individual solutions for common problems. Hence, a pool of diverse and efficient solutions exists that may be transferable to technical systems. Biomimetics or bio-inspiration has been used as a design approach for decades, revolutionizing products and processes throughout various industries. Thus, multiple examples can also be found in the space sector, since many characteristics found in biological organisms are also essential for space systems like response-stimuli adaptability, robustness and lightweight construction, autonomy and intelligence, energy efficiency, and self-repair or healing capabilities. This review focuses on biomimetics within the field of aerospace engineering and summarizes existing bio-inspired concepts such as drilling tools (wood wasp ovipositor drilling), telescopes (lobster eye optics), or gasping features (gecko feet adhesion capabilities) that have already been conceptualized, partially tested, and applied within the space sector. A multitude of biological models are introduced and how they may be applicable within the space environment. In particular, this review highlights potential bio-inspired concepts for dealing with the harsh environment of space as well as challenges encountered during rocket launches, space system operations and space exploration activities. Moreover, it covers well-known and new biomimetic concepts for space debris removal and on-orbit operations such as space-based energy production, servicing and repair, and manufacture and assembly. Afterwards, a summary of the challenges associated with biomimetic design is presented to transparently show the constraints and obstacles of transferring biological concepts to technical systems, which need to be overcome to achieve a successful application of a biomimetic design approach. Overall, the review highlights the benefits of a biomimetic design approach and stresses the advantage of biomimetics for technological development as it oftentimes offers an efficient and functional solution that does not sacrifice a system’s reliability or robustness. Nevertheless, it also underlines the difficulties of the biomimetic design approach and offers some suggestions in how to approach this method.
Biomimetics, bio-inspiration or biomimetic design describes the process of getting inspired by nature and using biological concepts for the development of technical solutions. Features or mechanisms of interest are extensively studied to understand their functioning, before they are abstracted and transferred to technical applications, products and processes (Wanieck et al., 2017). Biomimetic design has been used for some time now and several biomimetic concepts have been adapted and implemented as different products and technologies, now well-established on the market. Prominent examples are the lotus paint mimicking the lotus leaves’ hydrophobic tendencies to repel dirt and water (Solga et al., 2007; Spaeth and Barthlott, 2008), and Velcro inspired by the hooks of thistles to achieve reusable fastening (Velco, 1955; Menon et al., 2006). Many more concepts can be found in the prototype and testing stage, with the goal to establish products and technologies throughout industries. Within the maritime sector for example, biomimetics has been heavily researched in relation to reducing the frictional resistance of ship hulls on the example of shark skin (Figures 1A, B) (Ibrahim et al., 2021, 2018; Fu et al., 2017; Wen et al., 2014; Oeffner and Lauder, 2012) and the salvinia plant (Oeffner et al., 2021; Walheim et al., 2021), thereby significantly reducing the fuel consumption and emissions associated with waterborne transport. In the transportation sector, bio-inspired concepts have been applied for the development of cars (puffer fish) (Kozlov et al., 2015), trains (kingfisher bill) (Figures 1C, D) (Foo et al., 2017; Hu et al., 2018), and even airplanes (winglets of birds) (Figures 1E, F) (Guerrero et al., 2012). Furthermore, improved aerodynamic characteristics through whale-mimicking tubercles on the edge of wind turbine blades, ventilation fans, and windmills have been achieved within the energy sector (Figures 1G, H) (Fish et al., 2011; Ng et al., 2017; Zhang et al., 2020), and more efficient construction ways presented by using fold structures based on tree leaves or insect wings and growth patterns adapted from organism’s exoskeletons within architecture and construction (Pohl and Nachtigall, 2015).
FIGURE 1. (A) Microscopic photograph of the riblet surface structure of shark skin. Reproduced from (Wen et al., 2014), with permission from COMPANY OF BIOLOGISTS LTD, (B) photograph of a bio-inspired riblet foil. Reproduced from (Wen et al., 2014), with permission from COMPANY OF BIOLOGISTS LTD, (C) sketch of a kingfisher bird. Reproduced under CC-BY-4.0, Hu et al. (2018), (D) photo of a train inspired by the kingfisher’s beak shape. Reproduced under CC-BY-4.0, Hu et al. (2018), (E) photograph of a bold eagle showing of its winglets on its wing tip. Reproduced under CC-BY-4.0, Guerrero et al. (2012), (F) biomimetic transfer of winglets onto commercial airplanes Reproduced from Pixabay. (G) photograph of a humpback whales fin with turbercles in its edge. Reproduced from Pixabay, (H) concept transfer of turbercles onto a wind turbine blade. Reproduced from Fish, F. E. (2009), with permission from Spie (Karleena Burdick, Assistant editor) and Dr. Frank Fish.
More recently, advances have especially been achieved in the fields of robotics with e.g., elephant trunk-inspired robotic arms (Zhao et al., 2018; Mazzolai et al., 2019) and compound eye-sensors (Bora et al., 2018; Agrawal and Dean, 2019) among many others. Lastly, biomimetics cannot only be used as direct design approach but has also been proven to improve processes such as 3D-printing (Zhu et al., 2021), design (Kamps et al., 2017), and manufacture and assembly (Schranz et al., 2020). The examples mentioned above describe only a small portion of available bio-inspired technical solutions, but already demonstrate that biomimetics is a viable approach for the design of a diverse range of solutions in extreme environments and under competitive requirements.
Just as in other industries, biomimetics is not a foreign approach when it comes to aerospace engineering. Since there is currently no proof of biological life within the harsh environment that is space anywhere but on Earth, no direct natural examples can be used as a model. Therefore, the space sector presents a prime use case for biomimetic design as it describes the process of understanding the underlying natural mechanisms and transferring them into technical applications no matter of the original biological function rather than simply copying them. Several systems such as drilling tools [wood wasp (Pitcher et al., 2020)], telescopes [lobster eye (Tamagawa et al., 2020)], gasping features [gecko feet (Jiang et al., 2017)] and many more have already been conceptualized and partially applied in space technology development and present solutions, where conventional technologies are not able to mimic and compete with the highly optimised biological model.
The space environment, however, presents an especially challenging setting due to the existing conditions of low to zero gravity, high temperature fluctuations, elevated levels of UV, electromagnetic and particulate radiation, reactive atomic oxygen, as well as natural micrometeoroids and space debris (Finckenor and Groh, 2015; Aïssa et al., 2019). Despite the initial assumption that biological concepts may be unsuitable for the space environment based on their evolution under significantly different environmental conditions, biomimetics oftentimes offers an efficient and functional solution that does not sacrifice a system’s reliability or robustness (Menon et al., 2006). Due to the variety of features available in nature, many different approaches for similar actions can be found and selected based on specific system requirements. In addition, many features formed in biological organisms are also essential for space systems such as response-stimuli, adaptability, robustness and lightweight construction, autonomy and intelligence, energy efficiency, and self-repair or healing capabilities (Ayre, 2004; Egan et al., 2015). Such biological characteristics can be transferred and adapted to improve or even revolutionize traditional engineering methods. Hence, several studies and research activities have already considered nature as model for aerospace technology development.
This article summarizes the most recent advances in biomimetic research and developments within the space industry and presents future-oriented concepts as well as ideas for various innovative technologies that could lead future development and revolutionize existing space systems. In addition, cutting-edge mechanisms that have not yet been investigated in detail but present great promise to improve conventional systems will be highlighted. Lastly, challenges and limitations of the biomimetic design approach are introduced to deliver a comprehensive overview over the potential and problems of biomimetics in general.
All of the mentioned biological concepts are summarized in Section 8 Supplementary Table S1 below, stating their feature or function of interest together with a short description of how it works as well as an example application within the aerospace industry, other industries or sectors of application, and the respective literature.
The interest in a biomimetic design approach applied to aerospace engineering is rapidly growing as indicated by the graph in Figure 2, showing an increase of articles, books and other scientific documents over the past 10 years.
FIGURE 2. Graph indicating the exponential increase in the number of publications published related to the topic of biomimetics for aerospace applications. Key word search was conducted using the ScienceDirect database with the following key words: (biomimetics OR bionics OR bio-inspired OR bioinspired) AND (aerospace OR space), from 2002 to 2022 (State 10.10.2022). Note that the number of publications in 2022 can be anticipated to increase slightly until the end of the year.
This literature review was conducted based on reviews, research articles, book chapters, book reviews, and mini reviews from the databases ScienceDirect, SpringerLink, Google Scholar, and ResearchGate. The following keywords were used as sources of search records: biomimetics, bio-inspired, bionic, aerospace, and space. This review aims at presenting the most recent advances within aerospace research and highlighting innovative and prominent biomimetic concepts currently under consideration or with significant potential for the aerospace industry. Therefore, the results were filtered to include innovative research and studies from 2018 up to August 2022. Using the snowballing approach, more background information about valuable and interesting concepts was used to describe biological models in more detail and display the full picture in terms of existing research of single concepts. Only literature relevant to the research question was included, while articles with controversial content, methods or conclusions were excluded. Thus, this literature review provides context for the application of biomimetics within aerospace engineering and delivers background information for new research. It therefore acts as a general guide to what is already known and applied in regard to biomimetics and aerospace engineering, as well as what concepts and models hold potential for future investigation.
As mentioned above, the space environment presents multiple extreme characteristics fatal to living beings on Earth. Nevertheless, mechanisms can be found that show promising performances in the protection of organisms to cope with ionization radiation, extreme temperatures, electromagnetic interference, micro asteroids and space debris.
In humans and other living beings as well as some fungi and bacteria, the biopolymer Melanin is found in cells and is mainly responsible to protect the cell from UV or ionizing radiation. Therefore, synthetic Melanin was produced and investigated for its wavelength absorbing and radiation protection potential with applications in aerospace engineering and other industries (Turick et al., 2011; Li W. et al., 2020; Vasileiou and Summerer, 2020). Another biological mechanism to deal with high levels of radiation can be found in bdelloid rotifers. They display a very effective DNA repair mechanism to restore ionization radiation damage (Gladyshev and Meselson, 2008; Wiles and Schurko, 2020), which may be transferrable or adaptable in the future to produce radiation-tolerant coatings and materials. Especially for manned missions, the protection against UV radiation is vital for the health and survival of humans spending extended periods of time in space (Williams, 2022).
Due to the highly fluctuating temperatures within the space environment and, therefore, the enormous range of extremes, heat flow as well as temperature management and control are crucial steps to maintain the integrity of space systems. By incorporating a circulatory systems inspired by biological organisms into solar panels, experiments have demonstrated the potential for a symbiotic use of a single structure for a component’s structural efficiency as well as thermal management (Williams et al., 2007). Furthermore, bio-inspired porous carbon ablators were assessed regarding their thermal protection of spacecrafts during the re-entry process into planetary atmospheres, showing promising results to produce enhanced thermal protection even at high temperatures (Poloni et al., 2022).
On the other side of the temperature spectrum, many biological organisms can be found with distinctly evolved mechanisms to protect against freezing temperatures and the subsequent formation of ice crystals in their cells and tissue. Examples for this are ice-binding proteins or antifreeze proteins and glycolipids, which are currently applied within the fields of agriculture, cryobiology and food technology, but also hold great promise for material technology and therefore, aerospace engineering by preventing metals from frost formation and liquid materials from freezing (Bredow and Walker, 2017; Li and Guo, 2018; Zhou et al., 2019; Białkowska et al., 2020; Xiang et al., 2020).
Recently, researchers have taken first strides into developing lightweight and flexible materials for the protection of structures and equipment against electromagnetic radiation. Experiments show that electromagnetic interference can be successfully shielded by substituting conventional metal shields with ones inspired by cellular architecture with tiny pores mimicking cell walls as aerogels shown in Figure 3 (Liu et al., 2018; Zeng et al., 2020).
FIGURE 3. (A) Diagram how the electromagnetic interference shielding works with aerogels and a graphical representation of different sample performances throughout literature. Reproduced from Zhou et al (2021), with permission from Elsevier, (B) Photograph of the microstructure in the longitudinal (g)) and transverse (h)) planes of a MXene/CNF hybrid aerogels with 17wt% ultrathin cellulose nanofibrils content. Reproduced under CC-BY-4.0, Zeng et al. (2020).
While micrometeoroids have been a threat to space systems since the beginning of space flight, the likelihood of a collision with one is rather small compared to the ever-increasing quantity of anthropogenic space debris circulating Earth's orbits. Hence, collisions with such debris become an ever more likely problem. Two options are available for dealing with space debris in orbit: avoidance maneuvers or shielding against collisions. Shielding is very expensive, only possible to a certain degree (dependent on size and velocity of debris) and adds to the mass of the system, inadvertently reducing payload capabilities. Avoidance maneuvers are often an alternative, sometimes, however, a must to escape collisions with large debris. While maneuvers are not only fuel-costly, they also require knowledge and tracking of existing debris (OECD, 2020). Until 2020, the International Space Station (ISS), continuously home to a crew of astronauts since 1999, was forced to conduct 27 collision avoidance maneuvers. Another four instances occurred, where debris was not detected in time, demanding an emergency evacuation by the crew (Johnson, 2012; ODPO, 2020). Other unoccupied space systems such as CubeSats, which are usually not equipped with their own propulsion system, are not capable of performing debris evading maneuvers. Besides these obvious challenges, more costs associated with space debris are related to the repair or replacement of spacecrafts, space situational awareness activities, data-blackouts when evading maneuvers are conducted, and insurance costs for operational space systems (OECD, 2020). Hence, finding cost-efficient and effective ways for debris detection and tracking can have enormous economic benefits and make space travel even safer.
In nature, several concepts can be found that deal with similar problems as well. Dragonflies, for example, are able to pursue their prey within a turbulent environment and distracting stimuli, yet still manage to capture a selected target with a 97% success rate. They do so by using so-called small target motion detector neurons, which are very sensitive to target contrast. Hence, they present an efficient and highly adaptable visual processing system to prevent collisions with their surrounding, which has already been modified and transferred into tracking algorithms (Bagheri et al., 2017; Colonnier et al., 2019). Investigations by Bagheri et al. (2017) showed that their dragonfly-inspired algorithm presented a higher average success (47.6%) in tracking small targets within a natural scene compared to conventional algorithms (maximum average success: 41.9%). Likewise, locusts demonstrate an attractive mechanism to avoid collisions using their lobula giant movement detector neurons. Those enable locusts to recognize approaching obstacles even in low-contrast conditions or textured backgrounds in motion. Once a collision alert is triggered, the locust can adapt its behavior mid-flight to alter its trajectory and avoid the collision. Since this collision avoidance mechanisms seems very promising, it has already been considered for the implementation of smart vehicle technology and robotic navigation with obstacle avoidance (Yue et al., 2006; Keil et al., 2018; Wang et al., 2021b). Therefore, it shows potential for application within the context of aerospace engineering and space exploration.
Space systems designated for the exploration of extra-terrestrial bodies face an immense challenge right at the beginning of their mission. The landing of unmanned spacecrafts on the surface of another planet is oftentimes violent and associated with enormous impact forces. Therefore, several actions and measures have been taken to protect sensitive equipment and payloads against those forces. Likewise, nature too deals with great impact forces and has spent millions of years of evolutionary development cycles to evolve perfectly matched strategies and extraordinarily sophisticated systems to deal with the existing challenges such as great impacts. Thus, space technologies that have only been developed over the course of a few decades could highly benefit by learning from nature.
The seeds of trees fall from great heights to reach the bottom and have therefore developed multiple different protection mechanisms for the valuable payload of DNA contained within. The seeds of the plant Tragopogon dubius, for example, are attached to stalked parachutes, which have evolved to increase the aerodynamic drag of the seed and therefore slow its descent, which not only allows the seed to survive but also increase the distance travelled during its descent from the tree in order to propagate the species as far as possible (Pandolfi et al., 2012). While parachutes are the conventional solution for slowing the descent of space systems, velocity reduction may be optimized using parachutes of plants that have evolved over centuries.
In contrast to slowing the seed and thereby reducing the negative effects of hitting the ground, many species of nuts have evolved differently. Instead, they produce a rigid layer of protective shell around their seed, designated to protect it during impact (Islam et al., 2021). Likewise, insects form very stiff cuticles to protect against predators. These cuticles consist of hard composite structures formed with chitin fibers arranged in distinct patterns, which enable insects to cope with extensive amounts of strain and load. The composite structure is able to adapt to external forces and change its thickness, stiffness and orientation of fibers accordingly (Jullien et al., 2020; Stamm et al., 2021). This bio-material on its own has shown to produce a tensile strength of 130 MPa and an elastic modulus of 2,900 MPa, which can further be increased through combination with other materials such as polymers or resins (Gadgey and Bahekar, 2017; Hou et al., 2021). Marine species like mollusk shells, too, make use of a multilayered structure called nacre to build their own homes and as protection, which demonstrates a composite architecture of impressive mechanical characteristics (Yaraghi and Kisailus, 2018). Different species of shells produce different types of nacre some of which present a Young’s modulus of 60–70 GPa and a tensile strength of 70–100 MPa. (Barthelat et al., 2016; Jiao et al., 2019; Askarinejad et al., 2021). Nacre is also known for its great fracture propagation and deflection properties based on their high stiffness and fracture toughness. Gao et al. (2017) compared the mechanical properties of the natural nacre produced by the species Cristaria plicata and artificial composite materials, demonstrating higher flexural strength (267 MPa) and similar fracture toughness (1.9 MPa/m2) for the artificial material compared to natural nacre (172 MPa, 2.4 MPa/m2, respectively). Other mechanical parameters of the artificial biomaterial like ultimate stiffness and elastic modulus reached values of 18.6 GPa (Gao et al., 2017) and 11 GPa (Raj et al., 2020), respectively.
A third option of dealing with high impact forces is demonstrated by the peel of the pomelo fruit. Instead of evolving hard materials capable of preventing fractures of the plant’s fruit or slowing its descent, the peel of the pomelo fruit demonstrates a thick layer with open cell foam structure of varying pore size as depicted in Figure 4A), which protects the fruit inside from damage when falling from trees of up to 10 m in height. Experiments have uncovered a dissipation of up to 90% of the impact energy while testing on free falling pomelo fruits. They therefore present excellent impact damping and energy dissipating capabilities (Ortiz et al., 2018). More recently, the beneficial features of the pomelo’s peel have been recognized by the scientific community and several articles have been published studying and modelling the foam-like structure (Thielen et al., 2013; Bührig-Polaczek et al., 2016; Ortiz et al., 2018; Li T.-T. et al., 2019). Hence, artificial versions of the foam may be applicable to dampen the effects of vibrations and reduce oscillations of space systems as well.
FIGURE 4. Pomelo peel as dampener, (A) Photographs of the honey pomelo’s peel. Reproduced from Pixabay, and a view under a digital microscope taken at different regions of the peel at 150-times magnification (ⓒ E. Banken), (B) Photograph of an Aluminium foam sample with branched Al2O3-fibre bundles and a magnified view of a fibre reinforced Aluminium-alloy foam sample showing the connection between the fibre bundle and the foam matrix, Reproduced from Bührig-Polaczek et al. (2016), with permission IOP Publishing.
While some animals protect vital organs through fluid immersion, which absorbs the shock rather than transferring it to the valuable payload, the woodpeckers and Australian cassowary are two types of birds that have evolved different features to protecting their brains from high impact forces. The woodpecker uses its elongated and pointed beak to penetrate the bark of trees and find insects to feed on. It achieves the penetration through high percussive speeds of up to 22 times per second, accomplishing frequencies between 20–25 Hz and an acceleration between 600 g—1,000 g (Bian and Jing, 2014). Hence, to protect its brain, the skull has formed so that the brain is tightly held in its position, while its tongue reaches from its beak all the way around the brain along the back of the skull. Hence, even under extensive stress, the brain is protected and does not get damaged in the process (Hollin, 2021). Likewise, the Australian cassowary uses its head to ram it into tree trunks in order to knock down fruits. It has a hollow fin-shaped protrusion of keratin on its head connected to its skull that is filled with a soft, rubber-like matrix. This protrusion can protect the bird from impact velocities up to 50 km/h and absorbs the shock (Widholm and Jawaharlal, 2016).
Bio-inspired concepts like these are heavily researched for applications on earth, such as the prevention of brain injuries, as well as for the protection for sensitive equipment in engineering, and thus, present valuable insights for the protection of space systems, too.
In contrast, an example for damage forestalling and injury prevention is the natural principles of load flux dependent design. Trees and bones demonstrate the ability to direct and redistribute cell growth and therefore structural reinforcement in areas experiencing high amounts of stress (Menon et al., 2007). Conch shells, on the other hand, present curved lamellae that can increase fracture resistance by about 30% compared to straight ones (Li and Li, 2019). Self-reinforcing materials like these could significantly contribute to extending the lifespan of space systems and react to unforeseen internal and external system stressors.
Apart from high impact forces, space systems are also exposed to brutal vibrations upon launch of the carrier rocket and need to be securely fastened to prevent damages. Furthermore, moving parts in spacecrafts are usually avoided to prevent the creation of unwanted oscillations and rotational forces. Oftentimes, components are added designated to counteract persisting vibrations and associated negative impacts (Menon et al., 2007; Garcia-Perez et al., 2019; Guo et al., 2019). Yet, these measures limit functionalities and influence the system design. Even under consideration of major precautions, micro-vibrations can be created during a spacecraft’s operation due to faulty calculations or external impacts. Thus, mechanisms to reduce oscillations and dampen the effect of vibrations find a broad range of application within the space sector (Kamesh et al., 2010; Yu et al., 2018).
Nature has brought forth multiple approaches to protect organisms from large oscillations and vibrations. One method of dealing with vibrations is their isolation as demonstrated by the human middle ear using an auxiliary mass mechanism among others (Kim and Kang, 2019; Wang et al., 2019; Yan et al., 2021). Fish, too, present a natural vibration dissipation through their hierarchical arrangement of scales in a compliant dermal tissue that allows for flexibility. They offer reversible non-linear stiffening and exhibit an interesting locking behaviour that is observed when the tissue is bent or twisted. First experiments show a viscous damping performance and direction-dependent frictional characteristics of the scales that can be adjusted and manipulated through alterations of the scale’s geometry (Ali et al., 2019). In the plant world, oscillation dissipation can be observed as well. Plant roots as well as tree trunks have been shown to provide structural dampening and transfer of vibration energy throughout their branches (Barth, 2008; Kovacic et al., 2018).
If the force and experienced stress on single components or parts gets too large, biological organisms and technical systems alike tend to break, fracture, bend and deform. Nevertheless, nature displays various mechanisms to deal with such damages and is able to repair components to restore their mechanical and practical function. Healing capabilities can be found in almost every living being as it is crucial for survival to maintain structural integrity, fluid balance, and function. Self-healing in organisms can take shape in various different approaches, using stored elastic energy, hierarchical structures, self-assembly systems, liquid exchange, cross-linking of proteins and many more (Speck and Speck, 2019). During the wound-closing and healing process, activities such as coagulation, cell proliferation and matrix deposition are deployed to close holes and fissures, restoring the organism back to almost its original state (Aïssa et al., 2019). Bauer and Speck (2012) investigated the healing capabilites of tree bark species and found a 55% restoration after only 30 min of initial external injury. Thus, tissue can be sealed over a short period of time, restoring the parts functionality without repairing its full mechanical properties. Methods to structurally repair any fissures and (partially) restore mechanical properties exist too, however, take much longer (Speck and Speck, 2019).
Self-healing and -repairing systems are crucial for space systems as aerospace companies and manufacturers go through enormous efforts to protect components and parts from structural or electrical failure. Furthermore, maintenance operations are either very costly or not existent at all. Hence, systems like self-repairing components can extend the lifetime of spacecrafts and prevent total system failures due to propagating fissures and cracks. Some mechanisms inspired by animal, plant and human tissue have been abstracted and implemented as self-repairing materials, especially for polymer composite materials. Four main methods for repair have been established, and come in either a capsule, particle form or an organized net (filled with fluid or made from wires) that are spread throughout the material and are triggered by internal or external stimuli (Cohades et al., 2018; Aïssa et al., 2019). Liquid-filled fibers or capsules incorporated into materials can excrete a dye for visual detection of damages or a resin-hardener combination that fill any occurring cracks and restore their mechanical properties (Nellesen et al., 2011; Speck and Speck, 2019).
In order to learn more about a planet or other extra-terrestrial body, the gathering of as much information as possible is crucial. While sensors and scans using optical and thermal equipment as payload on satellite flyby’s deliver a great deal of data on those bodies, it is vital for a deeper understanding to gather physical evidence and take samples of various materials to be analyzed for mineral content but also for traces of life (Zhang et al., 2022b). Eventually, this information will be used to plan and execute in situ resource utilization to e.g., build a future space base. Other applications include asteroid mining for rare elements and finding life on other planets.
One of the most prominent applications for biomimetics within the space industry are drilling processes for planetary exploration and sample acquisition. Thus, multiple concepts exist that have conceptualized bio-inspired methods and procedures to improve and enhance these activities. One well developed biomimetic concept is based on the reciprocating drilling motion of the wood wasp. Wood wasps use their serrated ovipositors as depicted in Figures 5A,B to drill into the bark of trees to deposit their eggs. They can achieve a drilling speed of approximately 1–1.5 mm/min, while its ovipositor is assumed to have a Young’s modulus of about 10 GPa (King and Vincent, 1995). This drilling method exhibits several favorable characteristics like its drilling efficiency and low overhead mass requirements. In addition, it circumvents the major challenge of rotary motion associated with conventional planetary drill designs. Several prototypes exist that have been tested intensively in a wide range of substrates ranging from fine regolith simulants to icy substrates as the one shown in Figure 5C. Sakes et al. (2020) developed a wood wasp inspired micro-drill prototype for minimal invasive surgery capable of achieving a stroke velocity of 4–8.77 mm/s and a transportation rate of up to 5.82 mg/s. Still, a wood-wasp inspired drills for aerospace applications have yet to be deployed and tested within the space environment (Gao et al., 2006; Gouache et al., 2010; Pitcher et al., 2020; Alkalla et al., 2021).
FIGURE 5. Wood wasp reciprocating drill, (A) Photograph of a wood wasp, (B) magnified view of its ovipositor consisting of two serrated valves, Reproduced from King and Vincent (1995), with permission from the authors, (C) schematic picture of a micro drill for aerospace applications. Reproduced under CC-BY-4.0, Pitcher et al. (2020).
A similar concepts is demonstrated by the locust digging into the soil to deposit their eggs by pulling their abdomen into the hole while simultaneously clearing the debris out of the digging path (Gao et al., 2007). Other biomimetic concepts exist based on the earthworm or mole and operate with a similar approach (Kubota et al., 2007; Lee et al., 2019).
Other organisms besides earth-dwelling animals have proven effective ways to drill into substrates, too. Plants heavily rely on their roots to form a secure and permanent attachment to the soil and the distribution of water and nutrients to enable the development of a seedling able to outcompete its competitors and go on to grow into a mature plant. Hence, roots of different plants have developed various ways to achieve effective anchoring. One great example is displayed by the seeds of the plant Erodium cicutarium, which bury themselves into substrates. This mechanism is thought to have developed improving the seed dispersion process of the plant. Its fruits develop a seed with an elongated and spirally twisted tail that, once in contact with the ground, is humidity driven and causes the seed to autonomously bury itself into the material (Pandolfi et al., 2012; Mancuso et al., 2014). Developing such a passive drilling mechanism dependent on abiotic conditions in the surrounding environment could provide a very useful, resource-saving and energy efficient mechanism to allow probes to bury themselves into the surface material of planets. Furthermore, the formation of complex networks of smaller and bigger roots may offer great possibilities for underground pathfinding and mapping of structures under the surface, which can help to determine geological compositions, traces of water and other valuable resources (Menon et al., 2006; Seidl et al., 2008).
In order to transport different probes or scientific equipment across planetary surfaces from one interesting spot to another, autonomous or remote-controlled robotic systems are required. Extra-terrestrial terrains are often found to be difficult with many obstacles and rough surface textures, which frequently display challenges and limitations to conventional wheeled robotic systems (Armour et al., 2007). Many bio-inspired concepts exist and present different characteristics dependent on the local demands and requirements. Limbed robots, for example, are useful for rough terrain with large obstacles and are often designed to mimic multi-legged organisms such as spiders due to their high stability, range of motion and surface adhesion (Gasparetto et al., 2008; Dürr et al., 2019; Lopez-Arreguin and Montenegro, 2020). Other concepts are based on the jumping mechanisms of locusts, frogs, kangaroos and shrimps dismissing the highly complex control systems and the maximum height of obstacles (Armour et al., 2007).
After the success of the first-ever human-made flying object on Mars, the Ingenuity Mars Helicopter has paved the way for a new level of extra-planetary exploration (Balaram et al., 2018). Hence, flying drones and other flight systems have become of more interest for the exploration and investigation of foreign bodies throughout the Universe. On Mars, one of the most significant challenges is the thin atmosphere, which makes the generation of sufficient lift and thrust necessary to get a system airborne difficult. Nature offers a range of locomotion types for different media, and therefore provides a large pool of propulsion systems to get inspired by. Babu Mannam et al. (2020), for example, investigated several biological examples of flapping wings for their potential use in light atmosphere environments and summarized a design methodology and relevant hydrodynamic aspects to evaluate bio-inspired concepts for their application to different environmental conditions found on planets.
Eventually, the long-term goal of many space enthusiasts is to create manned habitats on other planetary bodies, elevating humanity from a single planet-based species to one that wonders the Universe. Hence, biomimetic concepts involved in the creation of habitats and continuously sustaining life under adverse environmental conditions will eventually become of much more interest. And with it, all of the biomimetic concepts already widely applied throughout many industries nowadays on Earth. This includes radiation protection, nutrition, waste management, as well as medical care (Jemison and Olabisi, 2021). ESA’s Advanced Concepts Team even investigated the possibility of mimicking the hibernation behavior of mammalians (e.g., Spermophilus tridecemlinneatus) to achieve human hypometabolic state for long duration journeys (Menon et al., 2007).
One example with great potential are the water-living lotus plants, which have developed superhydrophobic surfaces that cause water droplets to simply roll off, naturally keeping the leaf clean and continously guaranteeing access to oxygen in the surrounding air. Another water-living plant species is Salvinia molesta, which is able to trap air on the leaf’s surface and therefore can retain an air layer on top of their leaves in times where the leaves are temporarily fully submerged in water (Barthlott et al., 2016; Gobalakrishnan et al., 2020). Those mechanisms have been abstracted and applied throughout multiple industries but also hold merit for aerospace engineering. In fact, they have been proposed to prevent icing in small airplanes (Piscitelli et al., 2020) and water retention in a human life support system in spacecrafts (Rasheed and Weislogel, 2019). More importantly, such surfaces have been conceptualized for reducing dust and ice on solar panels on Earth (Wu et al., 2022), which is also of relevance for orbiting systems. Likewise, animals, too, have self-cleaning abilities similar to plants. The toe pads of geckos and tree frogs (Crawford et al., 2012; Hawkes et al., 2015) or the rigid forewings of dung beetles (Sun et al., 2012) present interesting methods keeping water from settling onto the surface of functional body parts. Mechanisms like these have been proposed for their implementation for anti-reflection, anti-fogging, micro-manipulators and self-healing in space systems (Xu et al., 2016), and are especially useful for things like lenses and visors, sensitive instruments such as batteries and power systems (Williams, 2022).
Space debris has become a major topic of concern, as defunct satellites, rocket upper stages and fragments are starting to threaten the operation of functional satellites, thereby endangering satellite communication, weather observations, and climate monitoring on earth (Sannigrahi, 2017; ESA, 2019; OECD, 2020). Hence, recent efforts have concentrated on space debris removal and mitigation measures (Ansdell, 2010), and options for more sustainable mission design as well as the establishment of mitigation guidelines have been proposed. These guidelines include provisions for incorporating removal systems such as drag sails into spacecraft design prior to launch for their eventual end-of-life (United Nations, 2010; Stokes et al., 2019). Especially drag sails have already been discussed within the scope of biomimetic design in terms of efficient folding and storage mechanisms, and several alternatives have been proposed, mimicking seeds and their dispersal mechanisms (Pandolfi et al., 2012; Pandolfi and Izzo, 2013) or tree and plant leaves (de Focatiis and Guest, 2002; Jasim and Taheri, 2018).
While space agencies and companies have focused on space debris capture and developing removal missions, to date no such mission has ever been performed. The first-ever operation simulating debris removal was conducted during the controlled activities of the RemoveDEBRIS mission in 2019, where a harpoon and a net were deployed to capture a previously released and well-known target body (Aglietti et al., 2020; Forshaw et al., 2020). In a joined venture of ESA and Cleanspace, the first debris removal mission Cleanspace-1 is scheduled to perform the first debris removal service of a VEGA secondary payload adapter as early as 2025 (Biesbroek et al., 2021). While these efforts displays a huge leap in space debris removal and tests showed promising results, harpoons are still associated with high risks of additional debris production due to the forces required to penetrate the target’s surface material while preventing a large impulse generation and thus pushing the target from its current course. Furthermore, complex rope dynamics between chaser and target have not been investigated in this mission and present a technical challenge (Zhang et al., 2021). Both, nets and harpoons offer great opportunities for their adaptation by using biological models as inspiration. Nets have frequently been under investigation in biomimetic research ranging from textiles (Blamires et al., 2020; Gu et al., 2020) to optical fibers (Tow et al., 2018). Harpoons, too, have been studied extensively and concepts have been proposed for surgical tools, modelling tips and shafts after the bee’s stingers (Sahlabadi and Hutapea, 2018) and mosquito’s probiscis (Li A. D. R. et al., 2020), among others.
One of the most prominent and probably furthest developed biomimetic example for space applications is the gecko tape. The tape exhibits a specifically structured surface modelled after the example of a gecko’s feet. Each toe of the gecko presents a highly adaptive microstructure with hundreds of hairs called setae as depicted in Figure 6A), each exertig a van der Walls force onto components, allowing the gecko to carry its own body weight up vertical surfaces or hang upside down (Kim et al., 2008). This mechanism has been replicated by multiple companies in form of a reusable adhesive tape with different microstructures as shown in Figure 6B), and tested extensively for various applications and consumer needs (Brodoceanu et al., 2016; Alizadehyazdi et al., 2020; Busche et al., 2020; Cauligi et al., 2020; Sameoto et al., 2022). One of these tapes was even sent to the ISS in 2019 to test its adhesive capabilities under micro-gravity conditions (Parness, 2017). Hence, gecko-inspired tape presents a great alternative that can achieve lasting and reusable attachment to objects in space. While some parameters such as the lasting adhesive capability or the effects of space dust on its performance still need to be explored further, gecko tape presents a great example for a substitute product within the space environment.
FIGURE 6. From gecko feet to adhesive tape, (A) Gecko foot and magnified view of the microstructures responsible for the adhesion thanks to van der Waals forces. Reproduced from Sameoto et al. (2022), with permission from Elsevier, (B) Magnified view of microstructures of different adhesive tape inspired by gecko feet, Reproduced from Aksak et al. (2007) Copyright 2007 American Chemical Society. Republished with permission; Reproduced from Murphy et al. (2007), with permission from Taylor and Francis.
Current robotic arms used for extravehicular operations on the ISS (Roa et al., 2017) and debris capturing concepts (Estable et al., 2020) suffer from shearing areas, reserve movement capabilities or chaser movement among other things (Behrens et al., 2012; Estable et al., 2020). Several of these limitations can be improved by taking advantages of nature’s diverse range of available biological examples. Octopi, for example, make use of eight identical and flexible arms for locomotion, grasping and reaching, as well as capturing food. These arms consist of mostly muscle tissue, which is able to selectively contract and therefore control their movements very efficiently (Cianchetti et al., 2015). Robotic systems inspired by octopi arms range from multi-actuator systems (Zhao et al., 2020) to soft robotics (Mazzolai et al., 2019) and have already been proposed for space debris removal (Le Letty et al., 2014; Shan et al., 2016; Jia et al., 2017). Their great mobility, maneuverability and adaptability makes them very suitable to wrap around complex target shapes. Likewise, other interesting biological models for similar tasks are presented by elephant’s trunks or seahorse tails. The trunks of elephants are highly flexible organs with multiple degrees of freedom and therefore presents higher obstacle avoidance capabilities and an increased workspace flexibility (Yang and Zhang, 2014; Zhao et al., 2018). A similar segmented approach is demonstrated by seahorses, which use their tail mainly for grasping activities involving different diameter objects such as plant stems. Seahorses present a continuously decreasing square cross-section in their tail made from four individual plates connected through special joints. This arrangements provide the seahorse with great bending and torsion abilities for grasping, especially of a diverse range of shapes and sizes. In addition, thanks to its specialized construction, their tails shows great fracture resistances under crushing and impact forces (Porter et al., 2015). The concept has been transferred into a multitude of prototypes for robotic arms (Porter and Ravikumar, 2017; Li L. et al., 2019; Zhang et al., 2022a), one of which is displayed in Figure 7.
FIGURE 7. Seahorse tail inspired robotic arm. (A) nano-CT image of a seahorse tail skeleton, (B) CAD model of the square cross-sectioned prototype during twisting and bending movements, (C) photograph of a square cross-sectioned 3D printed prototype, Reproduced from Porter et al. (2015) , with permission from The American Association for the Advancement of Science.
Within the scope of the ESA-funded BIOINSPACED project (acronym for bio-inspired solutions for space debris removal) conducted from June 2020 to February 2022, several bio-inspired space debris removal scenarios were established, incorporating a diverse range of biomimetic concepts. The project aimed to support ESA’s initiative to mitigate space debris and reduce its negative impact on current and future missions. After analyzing the technical requirements for space debris removal missions, nature’s pool of organisms was investigated to find suitable concepts and mechanisms with distinct benefits for space applications. Collected ideas were assessed to identify the most promising ones, that were then integrated into holistic mission scenarios. As the last step, one of these scenarios was selected for a low-tech implementation in the form of a simple demonstrator depicted in Figure 8, underlining the benefits and variety of applications for bio-inspired technical solutions for space debris removal (Banken et al., 2021, 2022).
FIGURE 8. Photographs of the simple demonstrator developed within the BIOINSPACED project and the project logo.
Besides the final demonstrator, the project also generated a comprehensive overview over biomimetic concepts and scenarios with further potential for aerospace engineering. One mission scenario conceptualized the use of the swarming behavior of ants to enable autonomous communication among several small and lightweight spacecrafts as shown in Figure 9A). These were theorized to organize themselves in specific patterns without requiring manual input commands, and therefore converge and arrange around a target object autonomously (Gro et al., 2006; Garnier et al., 2007; Divband Soorati et al., 2019). Processes like this can deliver a greater amount of details on target behavior, especially tumbling and rotational motion, which are important factors for contact maneuvers (Stacy and D’Amico, 2018). In addition to target inspection, they can be used for target capture in the form of attachment to the debris and removal, using their own propulsion in unison to deorbit the object.
FIGURE 9. Sketches of the scenarios developed within the scope of the BIOINSPACED project. (A) Chaser vehicle carries several sub-units capable of observing, attaching, and deorbiting a target object in unison based on swarm algorithms (e.g., ant behaviour after Katiyar et al. (2015), (B) Chaser vehicle with tactile sensing appendages passively wrapping around slow-moving target upon contact modelled after the trunk of elephants (ⓒ 12138562, pixabay.com).
Another interesting feature identified during the project was tactile sensing within the space environment displayed in Figure 9B). Currently, most existing spacecrafts rely on optical sensors to determine a target’s speed, rotation, and overall orbital mechanics. Yet, systems like these are known to suffer from e.g., dynamic illumination conditions or solar glare (Yilmaz et al., 2017). Nature provides a board range of organisms, which make use of tactile sensing as primary sensory input like mammals with vibrissae and insect antenna’s. Some have already been explored in the field of robotics (Pearson et al., 2011; Lee et al., 2022). Paired with haptic sensors mimicking human skin and touch receptors capable of recognizing stimuli such as force, vibration and temperatures, as well as detecting hardness, force, slip, shapes, and textures (Yi et al., 2018), they offer great opportunities for on-orbit operations involving close contact with (un-)cooperative targets (Haschke, 2015; Pacchierotti et al., 2015; Xin et al., 2016; Yi et al., 2018), where high levels of flexibility and sensitivity are of great importance.
Further biomimetic concept extracted during the BIOINSPACED project with great benefits for aerospace systems are potential vibration sensors on the example of elephant feet (Lane et al., 2020), and touch recognition modelled after thigmotropism of plants (Vidoni et al., 2013, 2015).
Activities such as the BIOINSPACED project support the identification of new and innovative approaches for technical problems, while delivering an overview over existing concepts. Within the scope of the project, many promising concepts and scenarios were identified where mimicking a biological model could improve conventional aerospace systems or overcome current challenges. While none of the presented concepts comes without their challenges, they offer great alternatives and innovative ideas to approach conventional engineering limitations and disrupt existing constraints.
Solar-based power generation is one of the main sustainable energy carriers used on Earth. With ever-increasing global energy demands and rising pressure to switch to more sustainable fuel alternatives enforced by legislations and regulations such as the European Green Deal (specifically to reduce emissions by 55% until 2030 and to net zero by 2050 (European Commission, 2019), research has focused on improving solar-based efficiencies, storage capacities and overall performances.
Space-based solar power generation, which has recently become more of a priority to major space agencies around the globe (ESA, 2022), has clear advantages over Earth-bound solar power, because it does not suffer from a limited period for power generation. It is possible to produce solar energy independent of day- and night-times as well as atmospheric losses. Especially weather conditions, which usually highly impact production and storage capacities with risk of damaging involved devices, are eliminated with space-based systems. Hence, the main portion of collected solar energy can be converted into microwave power, which can then be beamed to any location on Earth as illustrated in Figure 10 free to use (Gosavi et al., 2021). Especially since the development of space-based solar cells is funded on the existing use of solar power as main sources of energy for many spacecrafts and satellites, technological development is rapid and in high demand.
FIGURE 10. (A) Photograph of the Pachliopta aristolochiae butterfly, (B) Photograph of the nanostructure referred to as ‘disordered nanoholes’ that enhance light absorption, (C) Bio-inspired nanoholes etched into an amorphous silicon based photovoltaic absorber, Reproduced from Landgraf (2017), with permission from Julia Rohnstock (Assistant editor KIT).
Multiple biological examples can be found that use solar radiation to their advantage and either require it for internal temperature regulation [invertebrates, amphibians, reptiles (Norris and Kunz, 2012)] or photosynthesis (Hammarström and Wasielewski, 2011) for example. These effects can be adapted and transferred to technologies and processes involved in solar energy generation. Butterfly wings demonstrate periodic nanostructures producing an antireflective effect used to heat their flight muscles, which has been conceptualized and applied as lightweight solar concentrators. Similar nanostructures can also be found in the eyes of moths (Chen et al., 2011, 2014; Shanks et al., 2015; Vasileiou et al., 2021).
Solar cell development has also been influenced by the model of leaves, which present very efficient approaches for capturing and utilizing solar radiation. The combination of different types of tissue cells as well as intercellular air space was mimicked, producing a highly flexible organic solar cell with power conversion efficiencies of ca. 16% (Qu et al., 2020; Meng et al., 2021). Furthermore, to increase the wavelength spectrum absorbable by conventional solar cells, the charge transfer properties of biomolecules have been used as inspiration, adapted and transferred to achieve absorption of x-ray radiation (Cook et al., 2009b). Other applications for bio-inspired concepts include improved thermal characteristics of solar power systems mimicking hierarchical porous leaf structures (Shi et al., 2021), structural assembly and deployment of solar arrays on orbit, and radiation tracking (Sharma and Purohit, 2014; Jasim and Taheri, 2018).
Current research and developments for orbital spacecraft operations can be separated into on-orbit servicing, focused on extending the life of an existing spacecraft in orbit, and on-orbit manufacture and assembly, describing the construction of a new structure from modular components (Piskorz and Jones, 2018). Both operations have already been demonstrated successfully: on-orbit servicing by remotely inspecting and repairing the Hubble Space Telescope during its five servicing missions, allowing the telescope to deliver valuable science today, roughly 30 years after its launch (Boyd et al., 2017); and on-orbit assembly by the construction of the ISS starting in 1998, demonstrating the first multi-system structure designed for assembly in space. Over the past two decades, this structure has grown extensively, and with it its level of autonomy and control capabilities (Piskorz and Jones, 2018). Nevertheless, many of the previously specified bio-inspired concepts applicable to space debris removal can also be beneficial for servicing and repair activities. Contactless containment of a small satellite based on the example of a mouth or Venus flytrap (Shahinpoor, 2011; Banken et al., 2022), for example, would allow safe repairs and maintenance without the risk of spare parts, tools and spacecraft appendages to escape as additionally generated space debris. The Venus flytrap has already been used as an inspiration for advances in the fields of robotics (Shahinpoor, 2011; Falk et al., 2022; Tauber et al., 2022).
In addition, to make future space travel and exploration feasible from an economic and environmental standpoint, the lifetime of space systems need to be extended. While eco-design and self-repair can go a long way in preserving the function of operative systems (Ceschin and Gaziulusoy, 2016; Aïssa et al., 2019), they eventually fall victim to damages or their natural end-of-life. Hence, servicing, exchange and repair are proposed to re-use still functional parts and only replacing impaired or outdated components. The main biomimetic concepts found for on-orbit maintenance and repair are docking and grasping mechanisms for a servicing spacecraft to attach to an orbiting system. These include, for example, the previously introduced gecko adhesion and insect-like crawling robots (Xie et al., 2021). Furthermore, bio-inspired grasping and robotic manipulation have also already been discussed frequently for their use and application for on-orbit servicing and repair (Dai et al., 2020; Ellery, 2020; Ogundipe and Ellery, 2020).
On the ISS, several measures have been put in place for manual maintenance and smaller repairs, such as handrails along the entire outer module body for astronauts to safely maneuver to areas of interest. Hence, several intelligent, human-like robots have been developed within the past few decades, designated to perform tasks alongside astronauts and execute servicing on the space station (Jiang et al., 2022). One of them is the MonkeyBot with hands at the end of four appendages to navigate on the outside of the space station and carry out visual inspections (Wang et al., 2013). Other concepts for servicing the space station include chameleon-like (Ni et al., 2013), vine-like (Wooten and Walker, 2015) and tendril-like robots (Mehling et al., 2006).
Moreover, sustainable space exploration will and cannot be limited to earth-bound manufacture, assembly, and subsequent launch from Earth. This is especially valid for large structures, requiring an enormous payload capacity or multiple launches of separated subcomponents, leading to astronomical expenses (Sacco and Moon, 2019), and huge quantities of emissions contributing to climate change (Ross and Vedda, 2018). Hence, it has become apparent, that sustained future space exploration can only be achieved using in situ resources from other planets or asteroids (Ghidini, 2018), and manufacturing and assembling entire structures on-orbit (Rognant et al., 2019). On-orbit manufacture and assembly are not new ideas within aerospace engineering. They have been discussed for decades, and concepts and demonstrations have focused on the assembly of pre-made structures, enabling the construction of much larger erections than could be launched in a single spacecraft (Easdown, 2020). These present features like interfaces designed for modularity and connection to additional systems in space. In fact, the first mission including a structure for self-assembly, namely the new James Webb Telescope was launched in December 2021, and its successful erection will act as flagship for large-scale structures for autonomous self-assembly in space (Roa et al., 2019).
Another, even larger structure to be deployed within the space environment is the idea of the International Planetary Sun Shield (IPSS). Global temperatures are rising, and climate change is moving quick, resulting in several goals and actions by international policymakers. Despite ongoing efforts of politicians and legislators, climate goals are predicted to remain unfulfilled until 2050, which would have disastrous temperature increases of 2°C as a consequence (IPCC, 2019). The IPSS concept presents a futuristic idea aiming to reduce global warming by expanding a huge ultra-light Sun shield in space to indirectly shade Earth from radiation. In order to present an effective outcome, this shield requires an enormous reflective surface area in the range of million square kilometers to be positioned rather close to the Sun at Sun-Earth Lagrange 1. At this size, launching parts and components separately from Earth becomes unrealistic and expensive, creating the need for on-orbit manufacture and assembly for the required solar sail (Centers et al., 2020; Jehle et al., 2020; Fuglesang and Herreros Miciano, 2021; Roy, 2022). Here, biomimetics could be a valuable attribute when looking for pioneering and ground-breaking solutions for extravagant applications.
Additive manufacturing is the most frequently discussed technique for the production of systems and components in space, and describes a process where a component is first sliced into 2D layers, and then traced with lines of material in a pre-defined manner to produce an entirely new 3D component (Dordlofva et al., 2016). The best known additive manufacturing technique by the public is 3D printing, where a variety of materials such as polymers, but also metals, biological substances and ceramics are layered to form a part (Gralow et al., 2020). This type of manufacturing offers a more diverse range of possible applications as it becomes easier to produce very complex geometries than with conventional subtractive manufacturing methods. Like the excitement and success of additive manufacturing on Earth, the space industry has been exploring these processes as a valid option for on-orbit manufacturing. Starting with printing individual spacecraft sub-systems to be included and launched in real life satellites (Sacco and Moon 2019), NASA launched the first 3D printer to the ISS and tested its printing capabilities in zero gravity, demonstrating the possibility for in-space production of parts (Prater et al., 2019). Since then, additive manufacturing has been investigated for its potential and feasibility for on-orbit manufacture ranging from single components to entire spacecrafts, as well as using “local” or in situ resources by sourcing the Lunar and Martian surface regolith (Grundström et al., 2021; Laot et al., 2021).
Additive manufacturing is particularly interesting for biomimetics because it is based on similar principles as can be found in nature. Intricate structures can be manufactured and formed while making efficient use of raw materials and available energy. As presented in Figure 11A), many biological concepts can be used for a diverse range of applications within the field of additive manufacturing (Yang et al., 2018), ranging from mechanics [formation of mollusk shells (Yang et al., 2018; Yaraghi and Kisailus, 2018)] and optics [compound eye of insects (Zhao et al., 2017; Yu et al., 2020)], to electrics [mechanoreceptors (Yi et al., 2018)] and shape-changing mechanisms [phototropism of sunflowers (Sharma and Purohit, 2014)] and medical applications [artificial organs and tissue (Zhu et al., 2021)]. Furthermore, biomimetics can be applied to achieve structural optimization, producing a part that uses less material but presents the same structural integrity as conventionally crafted parts. This allows the manufacture of more complex and new components without sacrificing any mechanical properties (Gralow et al., 2020; Yang et al., 2022).
FIGURE 11. (A) Biomimetic concepts and their possible influence within different aspects of 3D printing and additive manufacturing. Reproduced from Yang et al. (2018), with permission from John Wiley and Sons. (B) Photograph of a spider spinning its net, (C) Model of SpiderFab, the bio-inspired assembly robot developed to construct large structures in space. Reproduced under CC-BY-4.0, Hoyt et al. (2013).
Once individual components and parts are produced on-orbit, they need to be assembled. Assembling subsystems into highly stable constructs are common principles in biology on a micro- and macroscopic scale. Beavers, for example, demonstrate a great ability to build large structurally sound dams using wood to limit water flow in rivers. Their construction behavior is of great interest, since they are able to adapt to a range of hydrological features and species of wood, and still manage to build constructions comparable to human structures (Cheng and Hou, 2016). One advanced concept for on-orbit assembly was demonstrated by the SpiderFab, a self-fabricating satellite inspired by a spider spinning its web. Instead of a web, the system, as depicted in Figure 11B), is employed to build and assemble large apertures and multifunctional structures (Hoyt et al., 2013). On a microscopic scale, cells and organic components such as peptides have been under investigation for their self-assembly capabilities, especially in molecular biology and precision medicine (Levin et al., 2020; Yang and Jiang, 2020). Yet, it may be possible to learn from these assembly methods and apply them on a much larger scale for the application in space.
More processes involved in on-orbit manufacture and assembly can benefit from bio-inspiration, such as artificial intelligence [neural networks (Krichmar et al., 2019; Wang et al., 2021c)], robotics [gripping (Jiang et al., 2015; Jia et al., 2017)] (see Section 3), and resource acquisition [drilling (Pitcher et al., 2020)] (see Subsection 2.3). Therefore, biomimetics demonstrates an application potential in almost every aspect when it comes to on-orbit processes. They may vary in impact and benefit for the resulting system, and use different biological forms, functions, or processes as a model. Nevertheless, the existing literature and current increase of prosperity as well as the growing interest in the field of biomimetics imply that further research activities should be focused on including bio-inspired design into aerospace engineering.
At the pinnacle of on-orbit manufacture and assembly stands a very forward-thinking concept for long-duration space exploration missions of self-replicating robots. Space systems are equipped with all necessary parts and systems to be able to replicate parts and components that allow it to extend and exchange their own parts on demand. Self-replication in itself can already be considered a bio-inspired process based on the natural propagation and reproduction of species (Ellery, 2017). Nevertheless, other bio-inspired technologies and components necessary for the self-replication of space systems have already been tested and proven by e.g., the production of bio-inspired semiconductors for electronic integrated circuits manufacturing (Girish et al., 2022).
The literature presented in this review summarizes merely a snippet of the vast diversity of biological concepts and mechanisms available. The review highlights how they can be adapted and transferred to improve, optimize, and advance technical solutions within the space sector. It demonstrates the potential of biodiversity and offers insights into innovative design approaches by highlighting unconventional ways for the development and design of processes, products, and interactions. Especially in terms of innovative and environmentally-friendly design, bio-inspiration has proven beneficial over the past decades. Therefore, it presents a multitude of advantages that might be explored within the fields of aerospace engineering and possibly support the evolution of a range of new products and processes necessary to pursue future-oriented goals within space exploration.
Nevertheless, despite the efforts conducted in this field of research, several challenges exist that hinder a persistent use of biomimetics throughout industries, which are listed in Table 1. The study of biomimetics has only experienced increasing momentum in recent years with more and more publications available over the past decades as presented in Figure 2 (Wanieck et al., 2017; Wanieck, 2022), even though the first ideas and methods have been identified as early as the 1950s in arts and design history (Montana-Hoyos et al., 2022). This phenomenon is attributed to three main limitations:
1. Lack of understanding and investigatory techniques of the biological system and its functioning
2. Missing tools and methods to assess, investigate and determine biomimetic concepts
3. Expertise barriers causing a lack of communication between fields
TABLE 1. List of benefits and challenges of the biomimetic design approach covered within this review.
Many very interesting biological concepts remain poorly understood, since their function has mainly been linked to specific characteristics rather than putting them into context with the entire system (Bechtel, 2012). Therefore, part of the research is based on (educated) assumptions and ideas rather than proven knowledge about mechanisms and processes. Furthermore, nature oftentimes works on a microscopic level, with a multitude of tiny structures, arrangements and conditions that collaboratively achieve a function on a higher level. Hence, it is very complex to investigate the functionality of micro- and nanostructures as well as their interaction within the organism and what they are responsible for (Hwang et al., 2015). Even after system functionalities have been identified, much research has to be invested into the repeatability and scalability of concepts, which usually demonstrates to be the more difficult part for an adaptation and transfer (Sharma and Sarkar, 2019).
Moreover, biomimetic design and the process of investigating biological models is associated with great uncertainty as research does not always pan out to be applicable to technology. While this is true for most fields of research, the lack of systematic approaches, strategies and guidelines highlights this uncertainty further (Graeff et al., 2021). Even when biological models are sufficiently understood, their complexity often exceeds available technical capabilities and thus need to be scaled back during the implementation phase. The difficult part is to do that while keeping the biomimetic function in tact. Oftentimes, secondary effects or accompanying solutions help maintain or produce the desired function in the first place (Habib, 2011; Gralow et al., 2020). For example, while shark-skin inspired riblet hull coatings still suffer from some degree of fouling, sharks do not deal with the same issue. Their skin structure and body undulation are much more complex and interact with one another, which is assumed to prevent a permanent attachment of marine biomass (Ibrahim et al., 2021). Furthermore, during the implementation phase, technological constraints and manufacturability often become a limiting factor. While additive manufacturing techniques, for example, have come a long way and enable the production of very advanced shapes and products of various materials, they still present limitations that have to be considered when designing bio-inspired systems (Habib, 2011; Gralow et al., 2020). Once a biomimetic mechanism and technology performs well in a laboratory setting, it needs to be scaled up to match their real world application, which is just as difficult.
Within problem-driven design, it is important to determine the appropriate requirements and constraints that must apply to a solution. However, since biomimetics remains a relatively recent design approach, the quantity and quality of tools and methods to investigate biological organisms and evaluate the suitability of their function for technical applications is limited (Sharma and Sarkar, 2019). At the other end of the process, it is important to evaluate the value and functionality of the innovation and if it actually improves the desired aspects compared to the conventional product to achieve a competitive advantage (Yen et al., 2014).
Furthermore, the lack of communication and information dissipation between disciplines further impedes the use of the biomimetic design approach. In order to overcome expertise barriers, several entities and organizations are working on establishing and maintaining databases that are user-friendly and can easily be used to find solutions for experts of different sectors (e.g.,BiOPS, IDEA-INSPIRE, BioTRIZ) (Wanieck et al., 2017; Wanieck, 2022). The website AskNature.org even provides a publicly available database with more than 1700 biological strategies developed by living things that achieve thousands of different functions (Deldin and Schuknecht, 2014; Biomimicry Institute, 2021). Their intention is to provide readily available information for engineers, technicians, and scientists to adapt and transfer biological mechanisms onto technical products, processes, and systems. Yet, due to the vast diversity of models available in nature, these databases struggle to demonstrate the complete picture of the enormous possibilities. In addition, included models oftentimes refer to certain areas of application or types of organisms and appear skewed, thereby only providing an imbalanced array of models (Graeff et al., 2020). Moreover, tools and databases focusing on biological systems alone oftentimes neglect their extended use and function throughout the environment and their role among species, which can reduce comprehensibility of concepts drastically and cause loss of information. Hence, while many of these databases support biomimetic design, they can lead to misinterpretations and transfer failures, inevitably resulting in the abandonment of the entire approach (Graeff et al., 2021). Another problem for non-biology experts is how to find appropriate models to investigate regarding their suitability to technical challenges. On the one hand, existing literature involving biomimetic design has been found to lack the appropriate labelling, making it difficult to find. On the other hand, papers have falsely been branded ‘biomimetic research’, thereby not only confusing experts of different fields but also causing frustration (Lepora et al., 2013).
Since this issue was recognized by experts throughout various sectors and fields of research, they have come together to form networks such as BIOKON (German), The Biomimicry Institute (United States), Biomimicry Innovation Lab (United Kingdom), and the Global Biomimicry Network (international), to overcome these challenges and facilitate knowledge of biological phenomena into other disciplines like engineering (Sharma and Sarkar, 2019). However, one remaining constraint has been determined to be the difference in terminology throughout the disciplines, which has hampered the communication of concepts, benefits, and limitations among experts. In addition, concepts are often found to be too complex to compare them with anything known to the opposite party, thereby prohibiting the propagation of a detailed understanding of principles and systems required to transfer them into a working technical design (Yen et al., 2014). Therefore, to achieve the most effective biomimetic design approach, companies often prefer to have an interdisciplinary team of biologists, chemists, engineers, technicians and scientists working closely together to close the existing gaps and enable a more efficient and successful design process (Graeff et al., 2021). Still, transition gaps between the idea, its implementation and the creation of a profitable product persist (Sharma and Sarkar, 2019). Thus, biomimetics does not always deliver ideal solutions that can be perfectly and easily transferred onto technical systems. It can be viewed as more of an inspirational source that can deliver crucial input and understandings into the development process of products and processes (Wanieck (2022)).
This summary presents the most prominent currently existing biomimetic concepts, technologies and processes developed for space technologies and highlights innovative ideas to be investigated in the future. While the majority of these concepts and approaches are still under investigation and present a low technological readiness level, their benefits and potential to improve common aerospace technologies and strategies has been demonstrated. Due to the vast quantity of mechanisms and features found in nature, biomimetics can be applied to almost any area of technological advancements and can support traditional engineering in a large field of applications. This is especially related to the topics discussed in this review: protection against the harsh space environment, space debris capture and removal as well as on-orbit operations.
Certainly, more biological concepts with potential for adaptation and transfer into technical systems within the space sector exist. Nevertheless, the presented concepts provide a broad overview and insight into the diversity and multitude of possibilities available. Moreover, concepts were highlighted and proposed for some of the most interesting areas of application within the aerospace sector to date, namely planetary exploration, space debris removal, space-based solar power as well as on-orbit servicing, manufacture, and assembly.
Lastly, several challenges associated with biomimetic design have been identified that hinder the use of bio-inspiration during the development of innovative technologies for future applications. Yet, biomimetics has been proven to provide multiple helpful observations and can improve conventional space systems by proposing novel and creative ideas, even when dealing with the extremely challenging environmental conditions of space.
The authors confirm contribution to the paper as follows: study conception and design, data collection, analysis and interpretation of results, and manuscript preparation: EB. Internal review: JO. All authors contributed to the article and approved the submitted version.
The BIOINSPACED study has received funding from the European Space Agency under grant agreement no. 4000130585 “Biomimicry (Biomimetics) for space debris mitigation” in the frame of ESA’s Discovery and Preparation studies.
The authors declare that the research was conducted in the absence of any commercial or financial relationships that could be construed as a potential conflict of interest.
All claims expressed in this article are solely those of the authors and do not necessarily represent those of their affiliated organizations, or those of the publisher, the editors and the reviewers. Any product that may be evaluated in this article, or claim that may be made by its manufacturer, is not guaranteed or endorsed by the publisher.
The Supplementary Material for this article can be found online at: https://www.frontiersin.org/articles/10.3389/frspt.2022.1000788/full#supplementary-material
Aglietti, G. S., Taylor, B., Fellowes, S., Salmon, T., Retat, I., Hall, A., et al. (2020). The active space debris removal mission removedebris. part 2: In orbit operations. Acta Astronaut. 168, 310–322. doi:10.1016/j.actaastro.2019.09.001
Agrawal, S., and Dean, B. (2019). “Digitization of biomimetic vision sensor based on the common housefly (musca domestica),” in Bioinspiration, biomimetics, and bioreplication IX. Editors A. Lakhtakia, R. J. Martín-Palma, and M. Knez (Bellingham, Washington, USA: SPIE), 25. doi:10.1117/12.2514114
Aïssa, B., Haddad, E., and Jamroz, W. R. (Editors) (2019). Self-Healing Materials: From fundamental concepts to advanced space and electronics applications (London, UK: Institution of Engineering and Technology). doi:10.1049/PBCS070
Aksak, B., Murphy, M. P., and Sitti, M. (2007). Adhesion of biologically inspired vertical and angled polymer microfiber arrays. Langmuir 23, 3322–3332. doi:10.1021/la062697t
Ali, H., Ebrahimi, H., and Ghosh, R. (2019). Frictional damping from biomimetic scales. Sci. Rep. 9, 14628. doi:10.1038/s41598-019-50944-0
Alizadehyazdi, V., Bonthron, M., and Spenko, M. (2020). Optimizing electrostatic cleaning for dust removal on gecko-inspired adhesives. J. Electrost. 108, 103499. doi:10.1016/j.elstat.2020.103499
Alkalla, M., Pang, X., Pitcher, C., and Gao, Y. (2021). Drod: A hybrid biomimetic undulatory and reciprocatory drill: Quantitative analysis and numerical study. Acta Astronaut. 182, 131–143. doi:10.1016/j.actaastro.2021.02.007
Amador-Vargas, S., Dominguez, M., León, G., Maldonado, B., Murillo, J., and Vides, G. L. (2014). Leaf-folding response of a sensitive plant shows context-dependent behavioral plasticity. Plant Ecol. 215, 1445–1454. doi:10.1007/s11258-014-0401-4
Anderson, C. V., and Deban, S. M. (2010). Ballistic tongue projection in chameleons maintains high performance at low temperature. Proc. Natl. Acad. Sci. U. S. A. 107, 5495–5499. doi:10.1073/pnas.0910778107
Ansdell, M. (2010). Active space debris removal: Needs, implications, and recommendations for today’s geopolitical environment. J. Public Int. Aff. 2010, 7–22.
Armour, R., Paskins, K., Bowyer, A., Vincent, J., Megill, W., and Bomphrey, R. (2007). Jumping robots: A biomimetic solution to locomotion across rough terrain. Bioinspir. Biomim. 2, S65–S82. doi:10.1088/1748-3182/2/3/S01
Askarinejad, S., Shalchy, F., and Rahbar, N. (2021). Role of interphase layers in mechanical properties of nacreous structures. Compos. Part B Eng. 225, 109255. doi:10.1016/j.compositesb.2021.109255
Ayre, M. (2004). Biomimetics applied to space exploration. WIT Trans. Ecol. Environ. 73. doi:10.2495/DN040591
Babu Mannam, N. P., Mahbub Alam, M., and Krishnankutty, P. (2020). Review of biomimetic flexible flapping foil propulsion systems on different planetary bodies. Results Eng. 8, 100183. doi:10.1016/j.rineng.2020.100183
Bagheri, Z. M., Wiederman, S. D., Cazzolato, B. S., Grainger, S., and O’Carroll, D. C. (2017). Performance of an insect-inspired target tracker in natural conditions. Bioinspir. Biomim. 12, 025006. doi:10.1088/1748-3190/aa5b48
Balaram, B., Canham, T., Duncan, C., Grip, H. F., Johnson, W., Maki, J., et al. (2018). Mars helicopter technology demonstrator. doi:10.2514/6.2018-0023
Banken, E., Schneider, V. E., Ben-Larbi, M. K., Pambaguian, L., and Oeffner, J. (2022). Biomimetic space debris removal: Conceptual design of bio-inspired active debris removal scenarios. CEAS Space J. doi:10.1007/s12567-022-00438-z
Banken, E., Schneider, V. E., Pohl, L., Kniep, J., Ströbel, R., Ben-Larbi, M. K., et al. (2021). “Assessing bioinspired concepts for space debris removal and evaluating their feasibility for simple demonstrator,” in 8th European Conference on Space Debris.
Barthelat, F., Yin, Z., and Buehler, M. J. (2016). Structure and mechanics of interfaces in biological materials. Nat. Rev. Mat. 1, 16007. doi:10.1038/natrevmats.2016.7
Barthlott, W., Mail, M., and Neinhuis, C. (2016). Superhydrophobic hierarchically structured surfaces in biology: Evolution, structural principles and biomimetic applications. Phil. Trans. R. Soc. A 374, 20160191. doi:10.1098/rsta.2016.0191
Bauer, G., and Speck, T. (2012). Restoration of tensile strength in bark samples of ficus benjamina due to coagulation of latex during fast self-healing of fissures. Ann. Bot. 109, 807–811. doi:10.1093/aob/mcr307
Bechtel, W. (2012). “Understanding biological mechanisms: Using illustrations from circadian rhythm research,” in History, philosophy and theory of the life sciences. Editors C. T. Wolfe, P. Huneman, and T. A. Reydon doi:10.1007/978-94-007-6537-5_22
Behrens, R., Poggendorf, M., Schulenburg, E., and Elkmann, N. (2012). “An elephant’s trunk-inspired robotic arm – trajectory determination and control,” in Proceedings of 7th German Conference, 417–421.
Białkowska, A., Majewska, E., Olczak, A., and Twarda-Clapa, A. (2020). Ice binding proteins: Diverse biological roles and applications in different types of industry. Biomolecules 10, 274. doi:10.3390/biom10020274
Bian, J., and Jing, X. (2014). “Biomimetic design of woodpecker for shock and vibration protection,” in 2014 IEEE International Conference on Robotics and Biomimetics (ROBIO 2014), 2238–2243. doi:10.1109/ROBIO.2014.7090670
Biesbroek, R., Aziz, S., Wolahan, A., Cipolla, S., Richard-Noca, M., and Piguet, L. (2021). “The cleanspace-1 mission: Esa and cleanspace team up to remove debris,” in 8th European Conference on Space Debris.
[Dataset] Biomimicry Institute (2021). It’s time to ask nature. Available from: https://asknature.org/.
Blamires, S. J., Spicer, P. T., and Flanagan, P. J. (2020). Spider silk biomimetics programs to inform the development of new wearable technologies. Front. Mat. 7. doi:10.3389/fmats.2020.00029
Bora, M., Kottapalli, A. G. P., Miao, J., and Triantafyllou, M. S. (2018). Sensing the flow beneath the fins. Bioinspir. Biomim. 13, 025002. doi:10.1088/1748-3190/aaa1c2
Boyd, I. D., Buenconsejo, R. S., Piskorz, D., Lal, B., Crane, K. W., and de La Rosa Blanco, E. (2017). On-orbit manufacturing and assembly of spacecraft. Alexandria, Virginia, USA: Institute for Defense Analysis.
Bredow, M., and Walker, V. K. (2017). Ice-binding proteins in plants. Front. Plant Sci. 8, 2153. doi:10.3389/fpls.2017.02153
Brodoceanu, D., Bauer, C. T., Kroner, E., Arzt, E., and Kraus, T. (2016). Hierarchical bioinspired adhesive surfaces-a review. Bioinspir. Biomim. 11, 051001. doi:10.1088/1748-3190/11/5/051001
Bu, Y., Wang, X., Bu, X., Mao, Z., Chen, Z., Li, Z., et al. (2023). Self-assembling nacre-like high-strength and extremely tough polymer composites with new toughening mechanism. J. Mater. Sci. Technol. 136, 236–244. doi:10.1016/j.jmst.2022.05.063
Bührig-Polaczek, A., Fleck, C., Speck, T., Schüler, P., Fischer, S. F., Caliaro, M., et al. (2016). Biomimetic cellular metals-using hierarchical structuring for energy absorption. Bioinspir. Biomim. 11, 045002. doi:10.1088/1748-3190/11/4/045002
Busche, J. F., Starke, G., Knickmeier, S., and Dietzel, A. (2020). Controllable dry adhesion based on two-photon polymerization and replication molding for space debris removal. Micro Nano Eng. 7, 100052. doi:10.1016/j.mne.2020.100052
Cauligi, A., Chen, T., Suresh, S. A., Dille, M., Ruiz, R. G., Vargas, A. M., et al. (2020). Design and development of a gecko-adhesive gripper for the astrobee free-flying robot.
Centers, R., Schertz, J., Scott, E., Jehle, A., and Carter-Cortez, V. (2020). “Space resources to stop global warming- a planetary sunshade,” in 71st International Astronautical Congress, CyberSpace Edition.
Ceschin, F., and Gaziulusoy, I. (2016). Evolution of design for sustainability: From product design to design for system innovations and transitions. Des. Stud. 47, 118–163. doi:10.1016/j.destud.2016.09.002
Chahl, J., and Mizutani, A. (2013). “Flight control using biomimetic optical sensors,” in Engineered Biomimicry. Editors A. Lakhtakia, and R. J. Martín-Palma (Amsterdam, Netherlands: Elsevier), 221–245. doi:10.1016/B978-0-12-415995-2.00009-X
Chen, J. D., Zhou, L., Ou, Q. D., Li, Y. Q., Shen, S., Lee, S. T., et al. (2014). Enhanced light harvesting in organic solar cells featuring a biomimetic active layer and a self-cleaning antireflective coating. Adv. Energy Mat. 4, 1301777. doi:10.1002/aenm.201301777
Chen, J., Xie, J., Wu, Z., Elbashiry, E. M. A., and Lu, Y. (2015). Review of beetle forewing structures and their biomimetic applications in China: (I) on the structural colors and the vertical and horizontal cross-sectional structures. Mat. Sci. Eng. C Mat. Biol. Appl. 55, 605–619. doi:10.1016/j.msec.2015.05.064
Chen, J. Y., Chang, W. L., Huang, C. K., and Sun, K. W. (2011). Biomimetic nanostructured antireflection coating and its application on crystalline silicon solar cells. Opt. Express 19, 14411–14419. doi:10.1364/OE.19.014411
Cheng, C. L., and Hou, J. H. (2016). Biomimetic robotic construction processes: An approach for adapting mass irregular-shaped natural materials. Robotics Des. Assembling 1, 133–142.
Cianchetti, M., Calisti, M., Margheri, L., Kuba, M., and Laschi, C. (2015). Bioinspired locomotion and grasping in water: The soft eight-arm octopus robot. Bioinspir. Biomim. 10, 035003. doi:10.1088/1748-3190/10/3/035003
Cohades, A., Branfoot, C., Rae, S., Bond, I., and Michaud, V. (2018). Progress in self-healing fiber-reinforced polymer composites. Adv. Mat. Interfaces 5, 1800177. doi:10.1002/admi.201800177
Colonnier, F., Ramirez-Martinez, S., Viollet, S., and Ruffier, F. (2019). A bio-inspired sighted robot chases like a hoverfly. Bioinspir. Biomim. 14, 036002. doi:10.1088/1748-3190/aaffa4
Cook, P. L., Johnson, P. S., Liu, X., Chin, A. L., and Himpsel, F. J. (2009a). Radiation damage in biomimetic dye molecules for solar cells. J. Chem. Phys. 131, 214702. doi:10.1063/1.3267849
Cook, P. L., Liu, X., Yang, W., and Himpsel, F. J. (2009b). X-ray absorption spectroscopy of biomimetic dye molecules for solar cells. J. Chem. Phys. 131, 194701. doi:10.1063/1.3257621
Cordero, R. J. B., Dragotakes, Q., Friello, P. J., and Casadevall, A. (2022). Melanin protects cryptococcus neoformans from spaceflight effects. Environ. Microbiol. Rep. 14 (4), 679–685. doi:10.1111/1758-2229.13078
Couturier, E., Du Courrech Pont, S., and Douady, S. (2009). A global regulation inducing the shape of growing folded leaves. PloS one 4, e7968. doi:10.1371/journal.pone.0007968
Crawford, N., Endlein, T., and Barnes, W. J. P. (2012). Self-cleaning in tree frog toe pads; a mechanism for recovering from contamination without the need for grooming. J. Exp. Biol. 215, 3965–3972. doi:10.1242/jeb.073809
Dai, H., Cao, X., Jing, X., Wang, X., and Yue, X. (2020). Bio-inspired anti-impact manipulator for capturing non-cooperative spacecraft: Theory and experiment. Mech. Syst. Signal Process. 142, 106785. doi:10.1016/j.ymssp.2020.106785
Das, R., Yadav, R. N., Sihota, P., Uniyal, P., Kumar, N., and Bhushan, B. (2018). Biomechanical evaluation of wasp and honeybee stingers. Sci. Rep. 8, 14945. doi:10.1038/s41598-018-33386-y
de Focatiis, D. S. A., and Guest, S. D. (2002). Deployable membranes designed from folding tree leaves. Philosophical Trans. R. Soc. Lond. Ser. A Math. Phys. Eng. Sci. 360, 227–238. doi:10.1098/rsta.2001.0928
Deldin, J. M., and Schuknecht, M. (2014). “The asknature database: Enabling solutions in biomimetic design,” in Biologically inspired design. Editors A. Goel, D. A. McAdams, and R. B. Stone
Deng, Z., Chen, F., Yang, Q., Bian, H., Du, G., Yong, J., et al. (2016). Dragonfly-eye-inspired artificial compound eyes with sophisticated imaging. Adv. Funct. Mat. 26, 1995–2001. doi:10.1002/adfm.201504941
Ding, H., Li, B., Wang, Z., Niu, S., Han, Z., and Ren, L. (2022). Bioinspired omnidirectional antireflective film with mechanical durability for efficient solar energy collection. Matter 5, 2990–3008. doi:10.1016/j.matt.2022.06.015
Divband Soorati, M., Heinrich, M. K., Ghofrani, J., Zahadat, P., and Hamann, H. (2019). Photomorphogenesis for robot self-assembly: Adaptivity, collective decision-making, and self-repair. Bioinspir. Biomim. 14, 056006. doi:10.1088/1748-3190/ab2958
Dordlofva, C., Lindwall, A., and Törlind, P. (2016). Challenges and opportunities for additive manufacturing in space applications. Nord. Des.
Dürr, V., Arena, P. P., Cruse, H., Dallmann, C. J., Drimus, A., Hoinville, T., et al. (2019). Integrative biomimetics of autonomous hexapedal locomotion. Front. Neurorobot. 13, 88. doi:10.3389/fnbot.2019.00088
Easdown, W. (2020). A mission architecture and systems level design of navigation, robotics and grappling hardware for an on-orbit servicing spacecraft.
Egan, P., Sinko, R., LeDuc, P. R., and Keten, S. (2015). The role of mechanics in biological and bio-inspired systems. Nat. Commun. 6, 7418. doi:10.1038/ncomms8418
Ellery, A. (2017). “Bioinspiration lessons from a self-replicating machine concept in a constrained environment,” in Proceedings of the 2017 IEEE International Conference on Robotics and Biomimetics.
Ellery, A. (2020). Tutorial review of bio-inspired approaches to robotic manipulation for space debris salvage. Biomimetics (Basel, Switz. 5. doi:10.3390/biomimetics5020019
[Dataset] ESA (2022). Esa reignites space-based solar power research. Available from: https://www.esa.int/Enabling_Support/Preparing_for_the_Future/Discovery_and_Preparation/ESA_reignites_space-based_solar_power_research.
Estable, S., Pruvost, C., Ferreira, E., Telaar, J., Fruhnert, M., Imhof, C., et al. (2020). Capturing and deorbiting envisat with an airbus spacetug. results from the esa e.deorbit consolidation phase study. J. Space Saf. Eng. 7, 52–66. doi:10.1016/j.jsse.2020.01.003
Falk, J. E., Auth, P., and Speck, T. (2022). Artificial venus flytraps: A research review and outlook on their importance for novel bioinspired materials systems. Front. Robot. AI 7, 75. doi:10.3389/frobt.2020.00075
Fan, J., Du, Q., Dong, Z., Zhao, J., and Xu, T. (2022). Design of the jump mechanism for a biomimetic robotic frog. Biomimetics 7, 142. doi:10.3390/biomimetics7040142
Fish, F. E. (2009). Biomimetics: Determining engineering opportunities from nature. Biomimetics Bioinspiration 7401, 740109. doi:10.1117/12.824106
Fish, F. E., Weber, P. W., Murray, M. M., and Howle, L. E. (2011). The tubercles on humpback whales’ flippers: Application of bio-inspired technology. Integr. Comp. Biol. 51, 203–213. doi:10.1093/icb/icr016
Floreano, D., and Mattiussi, C. (Editors) (2008). Bio-inspired artificial intelligence: Theories, methods, and technologies.
Foo, C. T., Omar, B., and Taib, I. (2017). Shape optimization of high-speed rail by biomimetic. MATEC Web Conf. 135, 00019. doi:10.1051/matecconf/201713500019
Forshaw, J. L., Aglietti, G. S., Fellowes, S., Salmon, T., Retat, I., Hall, A., et al. (2020). The active space debris removal mission removedebris. part 1: From concept to launch. Acta Astronaut. 168, 293–309. doi:10.1016/j.actaastro.2019.09.002
Fu, Y. F., Yuan, C. Q., and Bai, X. Q. (2017). Marine drag reduction of shark skin inspired riblet surfaces. Biosurface Biotribology 3, 11–24. doi:10.1016/j.bsbt.2017.02.001
Fuglesang, C., and Herreros Miciano, M. G. (2021). Realistic sunshade system at l1 for global temperature control. Acta Astronaut. 186, 269–279. doi:10.1016/j.actaastro.2021.04.035
Gadgey, K. K., and Bahekar, A. (2017). Studies on extraction methods of chitin from crab shell and investigation of its mechanical properties. Int. J. Mech. Eng. Technol. 8 (2), 220–231.
Gao, H. L., Chen, S. M., Mao, L. B., Song, Z. Q., Yao, H. B., Cölfen, H., et al. (2017). Mass production of bulk artificial nacre with excellent mechanical properties. Nat. Commun. 8, 287. doi:10.1038/s41467-017-00392-z
Gao, Y., Ellery, A., Jaddou, M., and Vincent, J. (2006). “Deployable wood wasp drill for planetary subsurface sampling,” in 2006 IEEE Aerospace Conference (Big Sky, MT: IEEE), 1–8. doi:10.1109/AERO.2006.1655756
Gao, Y., Ellery, A., Sweeting, M. N., and Vincent, J. (2007). Bioinspired drill for planetary sampling: Literature survey, conceptual design, and feasibility study. J. Spacecr. Rockets 44, 703–709. doi:10.2514/1.23025
Garcia-Perez, O. A., Trujillo-Franco, L. G., and Silva-Navarro, G. (2019). “On the adaptive vibration suppression on a flexible spatial structure,” in Topics in modal analysis & testing. Editors M. L. Mains, and B. J. Dilworth, 333–339. doi:10.1007/978-3-030-12684-1_35
Garnier, S., Gautrais, J., and Theraulaz, G. (2007). The biological principles of swarm intelligence. Swarm Intell. 1, 3–31. doi:10.1007/s11721-007-0004-y
Gasparetto, A., Vidoni, R., and Seidl, T. (2008). Kinematic study of the spider system in a biomimetic perspective.
Ghidini, T. (2018). Materials for space exploration and settlement. Nat. Mat. 17, 846–850. doi:10.1038/s41563-018-0184-4
Girish, T. E., Anupama, G. M., and Lakshmi, G. (2022). “Automated electronic integrated circuit manufacturing on the moon and Mars: Possibilities of the development of bio-inspired semiconductor technologies for space applications,” in Biomimicry for aerospace. Editors V. Shyam, M. Eggermont, and A. F. Hepp (Amsterdam, Netherlands: Elsevier), 459–475. doi:10.1016/B978-0-12-821074-1.00009-8
Giroud, S., Habold, C., Nespolo, R. F., Mejías, C., Terrien, J., Logan, S. M., et al. (2020). The torpid state: Recent advances in metabolic adaptations and protective mechanisms. Front. Physiol. 11, 623665. doi:10.3389/fphys.2020.623665
Gladyshev, E., and Meselson, M. (2008). Extreme resistance of bdelloid rotifers to ionizing radiation. Proc. Natl. Acad. Sci. U. S. A. 105, 5139–5144. doi:10.1073/pnas.0800966105
Gobalakrishnan, M., Anubama, S., Aravindan, S., and Bharath, V. (2020). Superhydrophobic nature of some plant leaves. Int. J. Eng. Appl. Sci. Technol. 5, 222–227. doi:10.33564/IJEAST.2020.v05i01.033
Gopal Krishna, B., and Tiwari, S. (2021). Bioinspired solar cells: Contribution of biology to light harvesting systems. Sustain. Material Solutions Sol. Energy Technol. 593, 593–632. doi:10.1016/B978-0-12-821592-0.00006-6
Gosavi, S. S., Mane, H. G., Pendhari, A. S., Magdum, A. P., Deshpande, S., Baraskar, A., et al. (2021). A review on space based solar power. JoTES. 6, 16–24. doi:10.46610/JoTES.2021.v06i01.003
Goss, D., Penick, C. A., Grishin, A., and Bhate, D. (2022). “Bio-inspired design and additive manufacturing of cellular materials,” in Biomimicry for aerospace. Editors V. Shyam, M. Eggermont, and A. F. Hepp (Amsterdam, Netherlands: Elsevier), 141–185. doi:10.1016/B978-0-12-821074-1.00003-7
Gouache, T., Gao, Y., Gourinat, Y., and Coste, P. (2010). “Wood wasp inspired planetary and Earth drill,” in Biomimetics, learning from nature. Editor A. Mukherjee doi:10.5772/8775
Graeff, E., Letard, A., Raskin, K., Maranzana, N., and Aoussat, A. (2021). Biomimetics from practical feedback to an interdisciplinary process. Res. Eng. Des. 1–27, 349–375. doi:10.1007/s00163-021-00356-x
Graeff, E., Maranzana, N., and Aoussat, A. (2020). Biological practices and fields, missing pieces of the biomimetics’ methodological puzzle. Biomimetics (Basel, Switz. 5. doi:10.3390/biomimetics5040062
Gralow, M., Weigand, F., Herzog, D., Wischeropp, T., and Emmelmann, C. (2020). Biomimetic design and laser additive manufacturing—A perfect symbiosis? J. Laser Appl. 32, 021201. doi:10.2351/1.5131642
Gro, R., Bonani, M., Mondada, F., and Dorigo, M. (2006). Autonomous self-assembly in swarm-bots. IEEE Trans. Robot. 22, 1115–1130. doi:10.1109/TRO.2006.882919
Grundström, B., Schild, T., and Cowley, A. (2021). Additive manufacturing of lunar regolith simulant using direct ink writing. Spool 8 (2), 55–69. doi:10.7480/SPOOL.2021.2.5268
Gu, Y., Yu, L., Mou, J., Wu, D., Zhou, P., and Xu, M. (2020). Mechanical properties and application analysis of spider silk bionic material. e-Polymers 20, 443–457. doi:10.1515/epoly-2020-0049
Guerrero, J. E., Maestro, D., and Bottaro, A. (2012). Biomimetic spiroid winglets for lift and drag control. Comptes Rendus Mécanique 340, 67–80. doi:10.1016/j.crme.2011.11.007
Guo, J., Damaren, C. J., and Geng, Y. (2019). Space structure vibration suppression using control moment gyroscope null motion. J. Guid. Control, Dyn. 42, 2272–2278. doi:10.2514/1.G004344
Ha, N. S., and Lu, G. (2020). A review of recent research on bio-inspired structures and materials for energy absorption applications. Compos. Part B Eng. 181, 107496. doi:10.1016/j.compositesb.2019.107496
Ha, N. S., Lu, G., and Xiang, X. (2018). High energy absorption efficiency of thin-walled conical corrugation tubes mimicking coconut tree configuration. Int. J. Mech. Sci. 148, 409–421. doi:10.1016/j.ijmecsci.2018.08.041
Habib, M. K. (2011). Biomimetics: Innovations and robotics. Int. J. Mechatronics Manuf. Syst. 4, 113. doi:10.1504/ijmms.2011.039263
Hammarström, L., and Wasielewski, M. R. (2011). Biomimetic approaches to artificial photosynthesis. Energy Environ. Sci. 4, 2339. doi:10.1039/c1ee90023d
Haschke, R. (2015). “Grasping and manipulation of unknown objects based on visual and tactile feedback,” in Motion and operation planning. Editors G. Carbone, and F. Gomez-Barvo (New York City: Springer International Publishing Switzerland), Vol. 29, 91–109. doi:10.1007/978-3-319-14705-5_4
Hawkes, E. W., Eason, E. V., Christensen, D. L., and Cutkosky, M. R. (2015). Human climbing with efficiently scaled gecko-inspired dry adhesives. J. R. Soc. Interface 12, 20140675. doi:10.1098/rsif.2014.0675
Hecox-Lea, B. J., and Mark Welch, D. B. (2018). Evolutionary diversity and novelty of dna repair genes in asexual bdelloid rotifers. BMC Evol. Biol. 18, 177. doi:10.1186/s12862-018-1288-9
Hespeels, B., Penninckx, S., Cornet, V., Bruneau, L., Bopp, C., Baumlé, V., et al. (2020). Iron ladies - how desiccated asexual rotifer adineta vaga deal with x-rays and heavy ions? Front. Microbiol. 11, 1792. doi:10.3389/fmicb.2020.01792
Hollin, G. (2021). Consider the woodpecker: The contested more-than-human ethics of biomimetic technology and traumatic brain injury. Soc. Stud. Sci. 52, 149–173. doi:10.1177/03063127211052513
Hou, J., Aydemir, B. E., and Dumanli, A. G. (2021). Understanding the structural diversity of chitins as a versatile biomaterial. Philos. Trans. A Math. Phys. Eng. Sci. 379, 20200331. doi:10.1098/rsta.2020.0331
Hoyt, R., Cushing, J., and Slostad, J. (2013). Spiderfab: Process for on-orbit construction of kilometer-scale apertures. Bothell, WA: Tethers Unlimited, Inc.
Hu, H. X., Tang, B., and Zhang, Y. (2018). Application of the bionic concept in reducing the complexity noise and drag of the mega high-speed train based on computer simulation technologies. Complexity 2018, 1–14. doi:10.1155/2018/3689178
Hwang, J., Jeong, Y., Park, J. M., Lee, K. H., Hong, J. W., and Choi, J. (2015). Biomimetics: Forecasting the future of science, engineering, and medicine. Int. J. Nanomedicine 10, 5701–5713. doi:10.2147/IJN.S83642
Ibrahim, M. D., Amran, S. N. A., Yunos, Y. S., Rahman, M. R. A., Mohtar, M. Z., Wong, L. K., et al. (2018). The study of drag reduction on ships inspired by simplified shark skin imitation. Appl. bionics biomechanics 2018, 7854321. doi:10.1155/2018/7854321
Ibrahim, M. D., Philip, S., Lam, S. S., and Sunami, Y. (2021). Evaluation of an antifouling surface inspired by Malaysian sharks Negaprion brevirostris and Carcharhinus leucas riblets. Tribol. Online 16, 70–80. doi:10.2474/trol.16.70
Ihssen, J., Braun, A., Faccio, G., Gajda-Schrantz, K., and Thöny-Meyer, L. (2014). Light harvesting proteins for solar fuel generation in bioengineered photoelectrochemical cells. Curr. Protein Pept. Sci. 15, 374–384. doi:10.2174/1389203715666140327105530
Imani, N., and Vale, B. (2022). Developing a method to connect thermal physiology in animals and plants to the design of energy efficient buildings. Biomimetics (Basel, Switz. 7. doi:10.3390/biomimetics7020067
Imani, N., and Vale, B. (2020). The development of a biomimetic design tool for building energy efficiency. Biomimetics (Basel, Switz. 5. doi:10.3390/biomimetics5040050
Ingrole, A., Aguirre, T. G., Fuller, L., and Donahue, S. W. (2021). Bioinspired energy absorbing material designs using additive manufacturing. J. Mech. Behav. Biomed. Mater. 119, 104518. doi:10.1016/j.jmbbm.2021.104518
IPCC (2019). Global warming of 1.5°c.an ipcc special report on the impacts of global warming of 1.5°c above pre-industrial levels and related global greenhouse gas emission pathways, in the context of strengthening the global response to the threat of climate change, sustainable development, and efforts to eradicate poverty. Geneva, Switzerland: International Panel on Climate Change.
Islam, M. K., Hazell, P. J., Escobedo, J. P., and Wang, H. (2021). Biomimetic armour design strategies for additive manufacturing: A review. Mater. Des. 205, 109730. doi:10.1016/j.matdes.2021.109730
Jasim, B., and Taheri, P. (2018). “An origami-based portable solar panel system,” in 2018 IEEE 9th Annual Information Technology, Electronics and Mobile Communication Conference (IEMCON) (Vancouver, BC, Canada: IEEE), 199–203. doi:10.1109/IEMCON.2018.8614997
Jehle, A., Scott, E., and Centers, R. (2020). A planetary sunshade built from space resources. AIAA Ascend. doi:10.2514/6.2020-4077.vid
Jemison, M., and Olabisi, R. (2021). Biomaterials for human space exploration: A review of their untapped potential. Acta biomater. 128, 77–99. doi:10.1016/j.actbio.2021.04.033
Jia, G., Li, B., Huang, H., Wu, Y., and Cao, Q. (2017). “Analysis of an underactuated biomimetic octopus hand for grasping space non-cooperative objects,” in IEEE International Conference on Cyborg and Bionic Systems.
Jiang, H., Hawkes, E. W., Arutyunov, V., Tims, J., Fuller, C., King, J. P., et al. (2015). “Scaling controllable adhesives to grapple floating objects in space,” in IEEE International Conference on Robotics and Automation (ICRA), 2828–2835. doi:10.1109/ICRA.2015.7139584
Jiang, H., Hawkes, E. W., Fuller, C., Estrada, M. A., Suresh, S. A., Abcouwer, N., et al. (2017). A robotic device using gecko-inspired adhesives can grasp and manipulate large objects in microgravity. Sci. Robot. 2, eaan4545–11. doi:10.1126/scirobotics.aan4545
Jiang, Z., Cao, X., Huang, X., Li, H., and Ceccarelli, M. (2022). Progress and development trend of space intelligent robot technology. Space Sci. Technol. 2022, 1–11. doi:10.34133/2022/9832053
Jiao, D., Qu, R. T., Weng, Z. Y., Liu, Z. Q., and Zhang, Z. F. (2019). On the fracture mechanisms of nacre: Effects of structural orientation. J. biomechanics 96, 109336. doi:10.1016/j.jbiomech.2019.109336
Johnson, N. L. (2012). Current characteristics and trends of the tracked satellite population in the human space flight regime. AIAA. doi:10.2514/6.IAC-06-B6.1.03
Jullien, A., Neradovskiy, M., Scarangella, A., and Mitov, M. (2020). Biomimicry of iridescent, patterned insect cuticles: Comparison of biological and synthetic, cholesteric microcells using hyperspectral imaging. J. R. Soc. Interface 17, 20200239. doi:10.1098/rsif.2020.0239
Jung, J. Y., Naleway, S. E., Yaraghi, N. A., Herrera, S., Sherman, V. R., Bushong, E. A., et al. (2016). Structural analysis of the tongue and hyoid apparatus in a woodpecker. Acta biomater. 37, 1–13. doi:10.1016/j.actbio.2016.03.030
Kamesh, D., Pandiyan, R., and Ghosal, A. (2010). Modeling, design and analysis of low frequency platform for attenuating micro-vibration in spacecraft. J. Sound Vib. 329, 3431–3450. doi:10.1016/j.jsv.2010.03.008
Kamps, T., Gralow, M., Schlick, G., and Reinhart, G. (2017). Systematic biomimetic part design for additive manufacturing. Procedia CIRP 65, 259–266. doi:10.1016/j.procir.2017.04.054
Kathpalia, R., and Verma, A. K. (2021). Bio-inspired nanoparticles for artificial photosynthesis. Mater. Today Proc. 45, 3825–3832. doi:10.1016/j.matpr.2021.03.214
Katiyar, S., Nasiruddin, I., and Ansari, A. Q. (2015). Ant colonyoptimization: A tutorial review. Natl. Conf. Adv. Power Control.
Keil, M. S., Roca-Moreno, E., and Rodriguez-Vazquez, A. (2018). A neural model of the locust visual system for detection of object approaches with real-world scenes.
Kheyraddini Mousavi, A., Leseman, Z. C., Palacio, M. L. B., Bhushan, B., Schricker, S. R., Sundaresan, V. B., et al. (2012). “Biomimetic mosquito-like microneedles,” in Encyclopedia of nanotechnology. Editor B. Bhushan (Dordrecht: Springer Netherlands), 276–284. doi:10.1007/978-90-481-9751-4_368
Kim, G. W., and Kang, J. (2019). The v-shaped band-stop vibration isolator inspired by middle ear. Appl. Acoust. 150, 162–168. doi:10.1016/j.apacoust.2019.02.013
Kim, S. W., Koh, J. S., Cho, M., and Cho, K. J. (2010). “Towards a bio-mimetic flytrap robot based on a snap-through mechanism,” in International Conference on Biomedical Robotics and Biomechatronics, 534–539. doi:10.1109/BIOROB.2010.5627994
Kim, S., Spenko, M., Trujillo, S., Heyneman, B., Santos, D., and Cutkosky, M. R. (2008). Smooth vertical surface climbing with directional adhesion. IEEE Trans. Robot. 24, 65–74. doi:10.1109/TRO.2007.909786
King, M., and Vincent, J. F. (1995). The mechanism of drilling by wood wasp ovipositors. Biomimetics 1995, 187–201.
Kogan, L., Weadon, T. L., Evans, T., DeVallance, D. B., and Sabolsky, E. M. (2015). Testing of tactile sensors for space applications. Sensors Smart Struct. Technol. Civ. Mech. Aerosp. Syst. 2015, 94352A. doi:10.1117/12.2085576
Kovacic, I., Zukovic, M., and Radomirovic, D. (2018). Sympodial tree-like structures: From small to large-amplitude vibrations. Bioinspir. Biomim. 13, 026002. doi:10.1088/1748-3190/aa9d1c
Kozlov, A., Chowdhury, H., Mustary, I., Loganathan, B., and Alam, F. (2015). Bio-inspired design: Aerodynamics of boxfish. Procedia Eng. 105, 323–328. doi:10.1016/j.proeng.2015.05.007
Kreuzwieser, J., Scheerer, U., Kruse, J., Burzlaff, T., Honsel, A., Alfarraj, S., et al. (2014). The venus flytrap attracts insects by the release of volatile organic compounds. J. Exp. Bot. 65, 755–766. doi:10.1093/jxb/ert455
Krichmar, J. L., Severa, W., Khan, M. S., and Olds, J. L. (2019). Making bread: Biomimetic strategies for artificial intelligence now and in the future. Front. Neurosci. 13, 666. doi:10.3389/fnins.2019.00666
Kubota, T., Nagaoka, K., Satoru, T., and Nakamura, T. (2007). “Earth-worm typed drilling robot for subsurface planetary exploration,” in Proceedings of the 2007 IEEE.
Lancer, B. H., Evans, B. J. E., Fabian, J. M., O’Carroll, D. C., and Wiederman, S. D. (2019). A target-detecting visual neuron in the dragonfly locks on to selectively attended targets. J. Neurosci. 39, 8497–8509. doi:10.1523/JNEUROSCI.1431-19.2019
[Dataset] Landgraf, M. (2017). Butterfly wing inspires photovoltaics: Light absorption can be enhanced by up to 200 percent. Available from: https://www.kit.edu/downloads/pi/PI_2017_153_Butterfly%20Wing%20Inspires%20Photovoltaics.pdf.
Lane, S., Barrett-Snyder, K., Lazarus, N., Alberts, W. C. K., and Hanrahan, B. (2020). Vibration sensing the mammalian way: An artificial pacinian corpuscle. Bioinspir. Biomim. 15, 046001. doi:10.1088/1748-3190/ab7ab6
Laot, M., Rich, B., Cheibas, I., Fu, J., Zhu, J. N., and Popovich, V. (2021). Additive manufacturing and spark plasma sintering of lunar regolith for functionally graded materials. spool 8 (2), 7–29. doi:10.7480/SPOOL.2021.2.5258
Lazarus, B. S., Velasco-Hogan, A., Gómez-del Río, T., Meyers, M. A., and Jasiuk, I. (2020). A review of impact resistant biological and bioinspired materials and structures. J. Mater. Res. Technol. 9, 15705–15738. doi:10.1016/j.jmrt.2020.10.062
Le Letty, R., Meyer, J. C., and Scheper, M. (2014). Clamping mechanism - a tentacles based capture mechanism for active debris removal.
Lee, H. J., Joyce, R., Kim, G., Kim, W., Han, S., and Lee, J. (2022). Antenna-shaped biomimetic tactile sensor using elastomers and conductive liquid. ACS Appl. Electron. Mat. 4, 4863–4872. doi:10.1021/acsaelm.2c00801
Lee, J., Kim, J., and Myung, H. (2019). “Design of forelimbs and digging mechanism of biomimetic mole robot for directional drilling,” in Proceedings of the 6th International Conference on Robot Intelligence Technology and Applications, 341–351. doi:10.1007/978-981-13-8323-6_28
Lepora, N. F., Verschure, P., and Prescott, T. J. (2013). The state of the art in biomimetics. Bioinspir. Biomim. 8, 013001. doi:10.1088/1748-3182/8/1/013001
Levin, A., Hakala, T. A., Schnaider, L., Bernardes, G. J. L., Gazit, E., and Knowles, T. P. J. (2020). Biomimetic peptide self-assembly for functional materials. Nat. Rev. Chem. 4, 615–634. doi:10.1038/s41570-020-0215-y
Li, A. D. R., Putra, K. B., Chen, L., Montgomery, J. S., and Shih, A. (2020a). Mosquito proboscis-inspired needle insertion to reduce tissue deformation and organ displacement. Sci. Rep. 10, 12248. doi:10.1038/s41598-020-68596-w
Li, H., and Li, X. (2019). The art of curved reinforcing in biological armors — Seashells. J. Bionic Eng. 16, 711–718. doi:10.1007/s42235-019-0057-9
Li, J., Xu, Z., Zhu, D., Dong, K., Yan, T., Zeng, Z., et al. (2021). Bio-inspired intelligence with applications to robotics: A survey. Intell. Robotics. doi:10.20517/ir.2021.08
Li, L., Jin, T., Tian, Y., Yang, F., and Xi, F. (2019a). Design and analysis of a square-shaped continuum robot with better grasping ability. IEEE Access 7, 57151–57162. doi:10.1109/ACCESS.2019.2914124
Li, Q., and Guo, Z. (2018). Fundamentals of icing and common strategies for designing biomimetic anti-icing surfaces. J. Mat. Chem. A Mat. 6, 13549–13581. doi:10.1039/C8TA03259A
Li, T. T., Wang, H., Huang, S. Y., Lou, C. W., and Lin, J. H. (2019b). Bioinspired foam composites resembling pomelo peel: Structural design and compressive, bursting and cushioning properties. Compos. Part B Eng. 172, 290–298. doi:10.1016/j.compositesb.2019.04.046
Li, W., Patil, A., Zhou, X., Wang, Z., Xiao, M., Shawkey, M. D., et al. (2020b). Characterization of broadband complex refractive index of synthetic melanin coatings and their changes after ultraviolet irradiation. Appl. Phys. Lett. 117, 203701. doi:10.1063/5.0024229
Ling, J., Song, Z., Wang, J., Chen, K., Li, J., Xu, S., et al. (2017). Effect of honeybee stinger and its microstructured barbs on insertion and pull force. J. Mech. Behav. Biomed. Mater. 68, 173–179. doi:10.1016/j.jmbbm.2017.01.040
Liu, R., Miao, M., Li, Y., Zhang, J., Cao, S., and Feng, X. (2018). Ultrathin biomimetic polymeric Ti3C2Tx MXene composite films for electromagnetic interference shielding. ACS Appl. Mat. Interfaces 10, 44787–44795. doi:10.1021/acsami.8b18347
Liu, Y., Qiu, X., Ma, H., Fu, W., and Yu, T. X. (2017). A study of woodpecker’s pecking process and the impact response of its brain. Int. J. Impact Eng. 108, 263–271. doi:10.1016/j.ijimpeng.2017.05.016
Lopez-Arreguin, A. J. R., and Montenegro, S. (2020). Towards bio-inspired robots for underground and surface exploration in planetary environments: An overview and novel developments inspired in sand-swimmers. Heliyon 6, e04148. doi:10.1016/j.heliyon.2020.e04148
Lu, Z., Cao, E., Wang, K., Mei, T., Wu, X., and Zhang, Q. (2017). “A catapult robot with chameleon-inspired multi-body elastic nested system,” in 2017 ieee international conference on robotics and biomimetics, December 5-8, 2017 (Macau sar, China.
Mancuso, S., Mazzolai, B., Comparini, D., Popova, L., Azzarello, E., Masi, E., et al. (2014). Subsurface investigation and interaction by self-burying biooinspired probes. Pontedera, Italy: European Space Agency, the Advanced Concepts Team. Ariadna Final Report 12-6402.
Mazzolai, B., Mondini, A., Tramacere, F., Riccomi, G., Sadeghi, A., Giordano, G., et al. (2019). Octopus–inspired soft arm with suction cups for enhanced grasping tasks in confined environments. Adv. Intell. Syst. 1, 1900041. doi:10.1002/aisy.201900041
Mehling, J. S., Diftler, M. A., Chu, M., and Valvo, M. (2006). “A minimally invasive tendril robot for in-space inspection,” in The First IEEE/RAS-EMBS International Conference on Biomedical Robotics and Biomechatronics, 690–695. doi:10.1109/BIOROB.2006.1639170
Meng, X., Hu, X., Zhang, Y., Huang, Z., Xing, Z., Gong, C., et al. (2021). A biomimetic self–shield interface for flexible perovskite solar cells with negligible lead leakage. Adv. Funct. Mat. 31, 2106460. doi:10.1002/adfm.202106460
Mengüç, Y., Röhrig, M., Abusomwan, U., Hölscher, H., and Sitti, M. (2014). Staying sticky: Contact self-cleaning of gecko-inspired adhesives. J. R. Soc. Interface 11, 20131205. doi:10.1098/rsif.2013.1205
Menon, C., Ayre, M., and Ellery, A. (2006). Biomimetics - a new approach for space system design. ESA Bull. 2006, 21–26.
Menon, C., Seidl, T., and Broschart, M. (2007). Missions to the outer solar system and beyond. Space Policy 63, 251–252. doi:10.1016/S0265-9646(98)00026-5
Montana-Hoyos, C., Daneluzzo, M., Tchakerian, R., Patel, S. V., and Morais, R. L. (2022). “Biomimicry and biodesign for innovation in future space colonization,” in Biomimicry for aerospace. Editors V. Shyam, M. Eggermont, and A. F. Hepp (Amsterdam, Netherlands: Elsevier), 3–39. doi:10.1016/B978-0-12-821074-1.00012-8
Morris, J. P., Wang, Y., Backeljau, T., and Chapelle, G. (2016). Biomimetic and bio-inspired uses of mollusc shells. Mar. genomics 27, 85–90. doi:10.1016/j.margen.2016.04.001
Mortimer, B., Walker, J. A., Lolchuragi, D. S., Reinwald, M., and Daballen, D. (2021). Noise matters: Elephants show risk-avoidance behaviour in response to human-generated seismic cues. Proc. R. Soc. B 288, 20210774. doi:10.1098/rspb.2021.0774
Moulton, D. E., Lessinnes, T., O’Keeffe, S., Dorfmann, L., and Goriely, A. (2016). The elastic secrets of the chameleon tongue. Proc. R. Soc. A 472, 20160030. doi:10.1098/rspa.2016.0030
Murphy, M. P., Aksak, B., and Sitti, M. (2007). Adhesion and anisotropic friction enhancements of angled heterogeneous micro-fiber arrays with spherical and spatula tips. J. Adhesion Sci. Technol. 21, 1281–1296. doi:10.1163/156856107782328380
[Dataset] NASA (2012). Technology readiness level. Available from: https://www.nasa.gov/directorates/heo/scan/engineering/technology/technology_readiness_level.
Nellesen, A., von Tapavicza, M., Bertling, J., Schmidt, A. M., Bauer, G., and Speck, T. (2011). Self-healing in plants as a model for self-repairing elastomer materials. Polym. Renew. Resour. 2, 149–156. doi:10.1177/204124791100200402
Ng, B. F., New, T. H. D., and Palacios, R. (2017). “Bio-inspired leading-edge tubercles to improve fatigue life in horizontal axis wind turbine blades,” in 35th wind energy symposium. doi:10.2514/6.2017-1381
Ni, J., Lv, J., Meng, Z., Feng, K., and Cai, J. (2021). Load-reduction mechanism of microstructure broach inspired by dung beetle surface. J. Manuf. Process. 64, 758–765. doi:10.1016/j.jmapro.2021.02.013
Ni, W., Zhang, B., Yang, H., Li, H., Jiang, Z., and Huang, Q. (2013). “Foot/hand design for a chameleon-like service robot in space station,” in International Conference on Robotics and Biomimetics, 215–220. doi:10.1109/ROBIO.2013.6739461
Nolte, A., Hennes, D., Izzo, D., Blum, C., Hafner, V., Gheysens, T., et al. (2017). A note on the depth-from-defocus mechanism of jumping spiders. Biomimetics 2, 3. doi:10.3390/biomimetics2010003
Norris, A. L., and Kunz, T. H. (2012). “Effects of solar radiation on animal thermoregulation,” in Solar radiation. Editor E. B. Babatunde (London, UK: InTech). doi:10.5772/34771
O’Connell-Rodwell, C. E. (2007). Keeping an “ear” to the ground: Seismic communication in elephants. Physiology 287, 287–294. doi:10.1152/physiol.00008.2007
ODPO (2020). Orbital debris quarterly news. Sugar Land, Texas, USA: NASA Orbital Debris Program Office.
OECD (2020). Space sustainability: The economics of space debris in perspective. Paris, France: Organisation for Economic Co-operation and Development.
Oeffner, J., Baars, A., Bretschneider, H., Focke, V., Hagemeister, N., Kesel, A., Editors, , et al. (2019). Towards biomimetic air retaining ship hull surfaces – AIRCOAT and its experimental and computational validation methods.
Oeffner, J., and Lauder, G. V. (2012). The hydrodynamic function of shark skin and two biomimetic applications. J. Exp. Biol. 215, 785–795. doi:10.1242/jeb.063040
Oeffner, J., Weisheit, J., Oikonomou, F., Schimmel, T., and Jahn, C. (2021). “Oeffner (2021) biomimetic self-adhesives foils instead of paints a business case for a sustainable ship hull coating_world of shipping Portugal,” in World of Shipping Portugal. An International Research Conference on Maritime Affairs.
Ogundipe, C., and Ellery, A. (2020). “Biomimetic control of spaceborne manipulator for debris removal and on-orbit servicing,” in i-SAIRAS2020.
Ortiz, J., Zhang, G., and McAdams, D. A. (2018). A model for the design of a pomelo peel bioinspired foam. J. Mech. Des. N. Y. 140. doi:10.1115/1.4040911
Pacchierotti, C., Prattichizzo, D., and Kuchenbecker, K. J. (2015). Displaying sensed tactile cues with a fingertip haptic device. IEEE Trans. Haptics 8, 384–396. doi:10.1109/TOH.2015.2445770
Pandolfi, C., Casseau, V., Fu, T. P., Jacques, L., and Izzo, D. (2012). “Tragopogon dubius, considerations on a possible biomimetic transfer,” in Conference on Biomimetic and Biohybrid Systems (Berlin, Heidelberg: Springer), 386–387.
Pandolfi, C., and Izzo, D. (2013). Biomimetics on seed dispersal: Survey and insights for space exploration. J. Bioinspiration Biomimetics 8, 1–9. doi:10.1088/1748-3182/8/2/025003
Parness, A. (2017). “Testing gecko-like adhesives aboard the international space station,” in AIAA SPACE and astronautics forum and exposition (Reston, Virginia: American Institute of Aeronautics and Astronautics). doi:10.2514/6.2017-5181
Patil, H. S., and Vaijapurkar, S. (2007). Study of the geometry and folding pattern of leaves of mimosa pudica. J. Bionic Eng. 4, 19–23. doi:10.1016/S1672-6529(07)60008-0
Pearson, M. J., Mitchinson, B., Sullivan, J. C., Pipe, A. G., and Prescott, T. J. (2011). Biomimetic vibrissal sensing for robots. Phil. Trans. R. Soc. B 366, 3085–3096. doi:10.1098/rstb.2011.0164
Pernigoni, L., Lafont, U., and Grande, A. M. (2021). Self-healing materials for space applications: Overview of present development and major limitations. CEAS Space J. 13, 341–352. doi:10.1007/s12567-021-00365-5
Piscitelli, F., Chiariello, A., Dabkowski, D., Corraro, G., Marra, F., and Di Palma, L. (2020). Superhydrophobic coatings as anti-icing systems for small aircraft. Aerospace 7, 2. doi:10.3390/aerospace7010002
Piskorz, D., and Jones, K. L. (2018). On-orbit assembly of space assets: A path to affordable and adaptable space infrastructure. El Segundo, CA, USA: The aerospace corporation.
Pitcher, C., Alkalla, M., Pang, X., and Gao, Y. (2020). Development of the third generation of the dual-reciprocating drill. Biomimetics (Basel, Switz. 5. doi:10.3390/biomimetics5030038
Pohl, G., and Nachtigall, W. (2015). Biomimetics for architecture & design. Cham: Springer International Publishing. doi:10.1007/978-3-319-19120-1
Poloni, E., Bouville, F., Schmid, A. L., Pelissari, P. I., Pandolfelli, V. C., Sousa, M. L., et al. (2022). Carbon ablators with porosity tailored for aerospace thermal protection during atmospheric re-entry. Carbon 195, 80–91. doi:10.1016/j.carbon.2022.03.062
Porter, M. M., Adriaens, D., Hatton, R. L., Meyers, M. A., and McKittrick, J. (2015). BIOMECHANICS. Why the seahorse tail is square. Sci. (New York, N.Y.) 349, aaa6683. doi:10.1126/science.aaa6683
Porter, M. M., and Ravikumar, N. (2017). 3d-printing a ’family’ of biomimetic models to explain armored grasping in syngnathid fishes. Bioinspir. Biomim. 12, 066007. doi:10.1088/1748-3190/aa8294
Prabhulkar, S., Tian, H., Wang, X., Zhu, J. J., and Li, C. Z. (2012). Engineered proteins: Redox properties and their applications. Antioxidants redox Signal. 17, 1796–1822. doi:10.1089/ars.2011.4001
Prater, T., Werkheiser, N., Ledbetter, F., Timucin, D., Wheeler, K., and Snyder, M. (2019). 3d printing in zero g technology demonstration mission: Complete experimental results and summary of related material modeling efforts. Int. J. Adv. Manuf. Technol. 101, 391–417. doi:10.1007/s00170-018-2827-7
Prescott, T., Mitchinson, B., and Grant, R. (2011). Vibrissal behavior and function. Scholarpedia 6, 6642. doi:10.4249/scholarpedia.6642
Prescott, T., Pearson, M., Mitchinson, B., Sullivan, J. C., and Pipe, A. (2009). Whisking with robots. IEEE Robot. Autom. Mag. 16, 42–50. doi:10.1109/MRA.2009.933624
Qu, T. Y., Zuo, L. J., Chen, J. D., Shi, X., Zhang, T., Li, L., et al. (2020). Biomimetic electrodes for flexible organic solar cells with efficiencies over 16%. Adv. Opt. Mat. 8, 2000669. doi:10.1002/adom.202000669
Raj, M., Patil, S. P., and Markert, B. (2020). Mechanical properties of nacre-like composites: A bottom-up approach. J. Compos. Sci. 4, 35. doi:10.3390/jcs4020035
Ramasubramanian, M. K., Barham, O. M., and Swaminathan, V. (2008). Mechanics of a mosquito bite with applications to microneedle design. Bioinspir. Biomim. 3, 046001. doi:10.1088/1748-3182/3/4/046001
Rasheed, R. M., and Weislogel, M. M. (2019). “The unrealized potential of superhydrophobic substrates in advanced life support systems,” in 49th International Conference on Environmental Systems.
Ren, K., and Yu, J. (2021). Research status of bionic amphibious robots: A review. Ocean. Eng. 227, 108862. doi:10.1016/j.oceaneng.2021.108862
Roa, M. A., Nottensteiner, K., Grunwald, G., Negro, P. L., Cuffolo, A., Andiappane, S., et al. (2019). In-space robotic assembly of large telescopes.
Roa, M. A., Nottensteiner, K., Wedler, A., and Grunwald, G. (2017). Robotic technologies for in-space assembly operations. Adv. Space Technol. Robotics Automation.
Rognant, M., Cumer, C., Biannic, J. M., Roa, M. A., Verhaeghe, A., and Bissonnette, V. (2019). “Autonomous assembly of large structures in space: A technology review,” in 8TH European Conference For Aeronautics and Aerospace Sciences (EUCASS). doi:10.13009/EUCASS2019-685
Ross, M., and Vedda, J. A. (2018). The policy and science of rocket emissions. El Segundo, CA, USA: The Aerospace Corperation.
Rossini, L., Seidl, T., Izzo, D., and Summerer, L. (2007). “Beyond astronaut’s capabilities: A critical review,” in 57th International Astronautical Congress.
Roy, K. (2022). The solar shield concept: Current status and future possibilities. Acta Astronaut. 197, 368–374. doi:10.1016/j.actaastro.2022.02.022
Rusinek, R. (2020). Sound transmission in the first nonlinear model of middle ear with an active implant. Math. Problems Eng. 2020, 1–23. doi:10.1155/2020/4580467
Sacco, E., and Moon, S. K. (2019). Additive manufacturing for space: Status and promises. Int. J. Adv. Manuf. Technol. 105, 4123–4146. doi:10.1007/s00170-019-03786-z
Sadeghi, A., Mondini, A., Del Dottore, E., Mattoli, V., Beccai, L., Taccola, S., et al. (2016). A plant-inspired robot with soft differential bending capabilities. Bioinspir. Biomim. 12, 015001. doi:10.1088/1748-3190/12/1/015001
Sahlabadi, M., and Hutapea, P. (2018). Tissue deformation and insertion force of bee-stinger inspired surgical needles. J. Med. Device. 12. doi:10.1115/1.4040637
Sakes, A., van de Steeg, I. A., de Kater, E. P., Posthoorn, P., Scali, M., and Breedveld, P. (2020). Development of a novel wasp-inspired friction-based tissue transportation device. Front. Bioeng. Biotechnol. 8, 575007. doi:10.3389/fbioe.2020.575007
Salamatian, A. A., and Bren, K. L. (2022). Bioinspired and biomolecular catalysts for energy conversion and storage. FEBS Lett. doi:10.1002/1873-3468.14533
Samal, S. K., Dash, M., Declercq, H. A., Gheysens, T., Dendooven, J., Voort, P., et al. (2014). Enzymatic mineralization of silk scaffolds. Macromol. Biosci. 14, 991–1003. doi:10.1002/mabi.201300513
Sameoto, D., Khungura, H., Benvidi, F. H., Asad, A., Liang, T., and Bacca, M. (2022). “Space applications for gecko-inspired adhesives,” in Biomimicry for aerospace. Editors V. Shyam, M. Eggermont, and A. F. Hepp (Amsterdam, Netherlands: Elsevier), 423–458. doi:10.1016/B978-0-12-821074-1.00016-5
Sannigrahi, A. K. (2017). Removal of active man-made orbital debris – A great challenge to space scientists. Int. J. Sci. Res. (IJSR).
Schranz, M., Umlauft, M., Sende, M., and Elmenreich, W. (2020). Swarm robotic behaviors and current applications. Front. Robot. AI 7, 36. doi:10.3389/frobt.2020.00036
Sethi, S., Ge, L., Ci, L., Ajayan, P. M., and Dhinojwala, A. (2008). Gecko-inspired carbon nanotube-based self-cleaning adhesives. Nano Lett. 8, 822–825. doi:10.1021/nl0727765
Shahinpoor, M. (2011). Biomimetic robotic venus flytrap (dionaea muscipula ellis) made with ionic polymer metal composites. Bioinspir. Biomim. 6, 046004. doi:10.1088/1748-3182/6/4/046004
Shan, M., Guo, J., and Gill, E. (2016). Review and comparison of active space debris capturing and removal methods. Prog. Aerosp. Sci. 80, 18–32. doi:10.1016/j.paerosci.2015.11.001
Shanks, K., Senthilarasu, S., Ffrench-Constant, R. H., and Mallick, T. K. (2015). White butterflies as solar photovoltaic concentrators. Sci. Rep. 5, 12267. doi:10.1038/srep12267
Sharma, D. K., and Purohit, G. (2014). “Nature inspired solar pv panels tracking using sunflower based heliotropism for higher efficiency of solar pv power system,” in 6th World Conference on Photovoltaic Energy Conversion, 855–856.
Sharma, S., and Sarkar, P. (2019). “Biomimicry: Exploring research, challenges, gaps, and tools,” in Research into design for a connected world, smart innovation, systems and technologies. Editor A. Chakrabarti (Singapore: Springer Nature Singapore Pte Ltd.), Vol. 134, 87–97. doi:10.1007/978-981-13-5974-3_8
Shi, X., Wang, F., Cheng, Z., Liang, H., Dong, Y., and Chen, X. (2021). Numerical analysis of the biomimetic leaf-type hierarchical porous structure to improve the energy storage efficiency of solar driven steam methane reforming. Int. J. Hydrogen Energy 46, 17653–17665. doi:10.1016/j.ijhydene.2021.02.171
Shunk, G. K., Gomez, X. R., Kern, C., and Averesch, N. (2021). A self-replicating radiation-shield for human deep-space exploration: Radiotrophic fungi can attenuate ionizing radiation aboard the international space station. bioRxiv. doi:10.1101/2020.07.16.205534
Siddique, S. H., Hazell, P. J., Wang, H., Escobedo, J. P., and Ameri, A. A. (2022). Lessons from nature: 3d printed bio-inspired porous structures for impact energy absorption – a review. Addit. Manuf. 58, 103051. doi:10.1016/j.addma.2022.103051
Solga, A., Cerman, Z., Striffler, B. F., Spaeth, M., and Barthlott, W. (2007). The dream of staying clean: Lotus and biomimetic surfaces. Bioinspir. Biomim. 2, S126–S134. doi:10.1088/1748-3182/2/4/S02
Spaeth, M., and Barthlott, W. (2008). Lotus-Effect<sup>®</sup>: Biomimetic Super-Hydrophobic Surfaces and their Application. Adv. Sci. Technol. 60, 38–46. doi:10.4028/www.scientific.net/AST.60.38
Speck, O., and Speck, T. (2019). An overview of bioinspired and biomimetic self-repairing materials. Biomimetics (Basel, Switz. 4. doi:10.3390/biomimetics4010026
Stacy, N., and D’Amico, S. (2018). “Autonomous swarming for simultaneous navigation and asteroid characterization,” in AAS/AIAA Astrodynamics Specialist Conference.
Stamm, K., Saltin, B. D., and Dirks, J. H. (2021). Biomechanics of insect cuticle: An interdisciplinary experimental challenge. Appl. Phys. A 127, 329. doi:10.1007/s00339-021-04439-3
Stokes, H., Akahoshi, Y., Bonnal, C., Destefanis, R., Gu, Y., Kato, A., et al. (2019). Evolution of iso’s space debris mitigation standards. First Int’l. Orbital Debris Conf.
Su, I., Qin, Z., Saraceno, T., Krell, A., Mühlethaler, R., Bisshop, A., et al. (2018). Imaging and analysis of a three-dimensional spider web architecture. J. R. Soc. Interface 15, 20180193. doi:10.1098/rsif.2018.0193
Su, Y., Hou, X., Li, L., Cao, G., Chen, X., Jin, T., et al. (2020). Study on impact energy absorption and adhesion of biomimetic buffer system for space robots. Adv. Space Res. 65, 1353–1366. doi:10.1016/j.asr.2019.12.006
Sun, M., Liang, A., Watson, G. S., Watson, J. A., Zheng, Y., and Jiang, L. (2012). Compound microstructures and wax layer of beetle elytral surfaces and their influence on wetting properties. PloS one 7, e46710. doi:10.1371/journal.pone.0046710
Tamagawa, T., Uchiyama, K., Otsubo, R., Yuasa, T., Zhou, Y., Mihara, T., et al. (2020). Multiplexing lobster-eye optics: A concept for wide-field x-ray monitoring. J. Astron. Telesc. Instrum. Syst. 6, 1. doi:10.1117/1.jatis.6.2.025003
Tauber, F. J., Auth, P., Teichmann, J., Scherag, F. D., and Speck, T. (2022). Novel motion sequences in plant-inspired robotics: Combining inspirations from snap-trapping in two plant species into an artificial venus flytrap demonstrator. Biomimetics (Basel, Switz. 7, 99. doi:10.3390/biomimetics7030099
Thielen, M., Schmitt, C. N. Z., Eckert, S., Speck, T., and Seidel, R. (2013). Structure-function relationship of the foam-like pomelo peel (citrus maxima)-an inspiration for the development of biomimetic damping materials with high energy dissipation. Bioinspir. Biomim. 8, 025001. doi:10.1088/1748-3182/8/2/025001
Tilton, M., Borjali, A., Isaacson, A., Varadarajan, K. M., and Manogharan, G. P. (2021). On structure and mechanics of biomimetic meta-biomaterials fabricated via metal additive manufacturing. Mater. Des. 201, 109498. doi:10.1016/j.matdes.2021.109498
Torres, F. G., Malásquez, M., and Troncoso, O. P. (2015). Impact and fracture analysis of fish scales from arapaima gigas. Mater. Sci. Eng. C 51, 153–157. doi:10.1016/j.msec.2015.02.034
Tow, K. H., Chow, D. M., Vollrath, F., Dicaire, I., Gheysens, T., and Thévenaz, L. (2018). “From spider webs to a biomimetic optical fibre sensor,” in Optical Fiber Communication Conference. doi:10.1364/OFC.2018.W1K.4
Trentlage, C., Hensel, R., Holzbauer, R., Stoll, E., Arzt, E., and Makaya, A. (2018). “Development of gecko-adhesive materials for applications,” in 69th International Astronautical Congress.
Trentlage, C., and Stoll, E. (2015). The applicability of gecko adhesives in a docking mechanism for active debris removal missions.
Trivailo, P., and Kojima, H. (2016). “Dynamics of the net systems, capturing space debris,” in Transactions of the Japan society for aeronautical and space sciences, aerospace technology Japan, 57–66. doi:10.2322/tastj.14.Pr_57
Turick, C. E., Ekechukwu, A. A., Milliken, C. E., Casadevall, A., and Dadachova, E. (2011). Gamma radiation interacts with melanin to alter its oxidation-reduction potential and results in electric current production. Bioelectrochemistry Amst. Neth. 82, 69–73. doi:10.1016/j.bioelechem.2011.04.009
United Nations (2010). Space debris mitigation guidelines of the committee on the peaceful uses of outer space. San Francisco, CA, USA: United Nations.
Vasileiou, T., Llorens, J. M., Buencuerpo, J., Ripalda, J. M., Izzo, D., and Summerer, L. (2021). Light absorption enhancement and radiation hardening for triple junction solar cell through bioinspired nanostructures. Bioinspir. Biomim. 16, 056010. doi:10.1088/1748-3190/ac095b
Vasileiou, T., and Summerer, L. (2020). A biomimetic approach to shielding from ionizing radiation: The case of melanized fungi. PloS one 15, e0229921. doi:10.1371/journal.pone.0229921
[Dataset] Velco, S. A. (1955). Improvements in or relating to a method and a device for producing a velvet type fabric: Swiss patent.
Vidoni, R., Mimmo, T., Pandolfi, C., Cesco, S., and Valentinuzzi, F. (2013). Mimicking the thigmotropic behaviour of climbing plants to design a tactile-based grasping device for the space environment.
Vidoni, R., Mimmo, T., and Pandolfi, C. (2015). Tendril-based climbing plants to model, simulate and create bio-inspired robotic systems. J. Bionic Eng. 12, 250–262. doi:10.1016/S1672-6529(14)60117-7
Walheim, S., Schimmel, T., Barczewski, M., Speichermann-Jägel, L., Droll, R., Dullenkopf-Beck, S., et al. (2021). “Passive air lubrication: Demonstrating a research vessel coated with a hull of air,” in 13th Symposium on High-Performance Marine Vehicles (HIPER).
Wan, C., and Gorb, S. N. (2021). Body-catapult mechanism of the sandhopper jump and its biomimetic implications. Acta biomater. 124, 282–290. doi:10.1016/j.actbio.2021.01.033
Wang, C., Chen, Y., Chen, M., Han, L., Qian, H., and Xu, Y. (2013). “A novel exterior climbing robot design for space station,” in International Conference on Robotics and Biomimetics, 1722–1727. doi:10.1109/ROBIO.2013.6739716
Wang, J., Chen, W., Xiao, X., Xu, Y., Li, C., Jia, X., et al. (2021a). A survey of the development of biomimetic intelligence and robotics. Biomim. Intell. Robotics 1, 100001. doi:10.1016/j.birob.2021.100001
Wang, M., Zang, X. Z., Fan, J. Z., and Zhao, J. (2008). Biological jumping mechanism analysis and modeling for frog robot. J. Bionic Eng. 5, 181–188. doi:10.1016/S1672-6529(08)60023-2
Wang, X., Zhao, M., Zhang, L., Li, K., Wang, D., Zhang, L., et al. (2022). Liquid metal bionic instant self-healing flexible electronics with full recyclability and high reliability. Chem. Eng. J. 431, 133965. doi:10.1016/j.cej.2021.133965
Wang, Y., Gong, Y., Huang, S., Xing, X., Lv, Z., Wang, J., et al. (2021b). Memristor-based biomimetic compound eye for real-time collision detection. Nat. Commun. 12, 5979. doi:10.1038/s41467-021-26314-8
Wang, Y., Jing, X., Dai, H., and Li, F. M. (2019). Subharmonics and ultra-subharmonics of a bio-inspired nonlinear isolation system. Int. J. Mech. Sci. 152, 167–184. doi:10.1016/j.ijmecsci.2018.12.054
Wang, Y., Liu, Q., Lan, Z. F., Zhang, B., Zhang, H. Q., Liu, J. W., et al. (2021c). Strong and tough bioinspired nacre-like b4c/al functionally graded materials with eliminated abrupt interfaces. Compos. Commun. 25, 100741. doi:10.1016/j.coco.2021.100741
Wanieck, K. (2022). Biomimetics for technical products and innovation: An overview for applications. Wiesbaden: Springer Fachmedien Wiesbaden. doi:10.1007/978-3-658-33150-4
Wanieck, K., Fayemi, P. E., Maranzana, N., Zollfrank, C., and Jacobs, S. (2017). Biomimetics and its tools. Bioinspired, Biomim. Nanobiomaterials 6, 53–66. doi:10.1680/jbibn.16.00010
Weissengruber, G. E., Egger, G. F., Hutchinson, J. R., Groenewald, H. B., Elsässer, L., Famini, D., et al. (2006). The structure of the cushions in the feet of african elephants (loxodonta africana). J. Anat. 209, 781–792. doi:10.1111/j.1469-7580.2006.00648.x
Wen, L., Weaver, J. C., and Lauder, G. V. (2014). Biomimetic shark skin: Design, fabrication and hydrodynamic function. J. Exp. Biol. 217, 1656–1666. doi:10.1242/jeb.097097
Weyda, F., and Kodrík, D. (2020). New functionally ultrastructural details of the honey bee stinger tip: Serrated edge and pitted surface. J. Apic. Res. 1, 875–878. doi:10.1080/00218839.2020.1837545
Widholm, S., and Jawaharlal, M. (2016). “Analysis of cassowary casques for shock absorption,” in Proceedings of the 2016 International Mechanical Engineering Congress & Exposition. doi:10.1115/IMECE2016-65120
Wiles, J. T., and Schurko, A. (2020). Identifying prospective genes that function during dna repair in the bdelloid rotifer adineta vaga. FASEB J. 34, 1. doi:10.1096/fasebj.2020.34.s1.06480
Williams, A., Arritt, B., Diaz-Aguado, M., Taft, B., and Nieuwkoop, A. (2007). “Biologically inspired thermal-structural satellite panels,” in 48th AIAA/ASME/ASCE/AHS/ASC Structures, Structural Dynamics, and Materials Conference. doi:10.2514/6.2007-2183
Williams, T. S. (2022). “Advancing research efforts in biomimicry to develop nature-inspired materials, processes for space exploration and more efficient aircraft,” in Biomimicry for aerospace. Editors V. Shyam, M. Eggermont, and A. F. Hepp (Amsterdam, Netherlands: Elsevier), 385–421. doi:10.1016/B978-0-12-821074-1.00014-1
Wooten, M. B., and Walker, I. D. (2015). “A novel vine-like robot for in-orbit inspection,” in 45th International Conference on Environmental Systems, 1–32. doi:10.1093/oso/9780198854913.003.0001
Wu, Y., Du, J., Liu, G., Ma, D., Jia, F., Klemeš, J. J., et al. (2022). A review of self-cleaning technology to reduce dust and ice accumulation in photovoltaic power generation using superhydrophobic coating. Renew. Energy 185, 1034–1061. doi:10.1016/j.renene.2021.12.123
Wu, Y., Yan, C., Wang, Y., Gao, C., and Liu, Y. (2021). Biomimetic structure of chitosan reinforced epoxy natural rubber with self-healed, recyclable and antimicrobial ability. Int. J. Biol. Macromol. 184, 9–19. doi:10.1016/j.ijbiomac.2021.06.037
Xiang, H., Yang, X., Ke, L., and Hu, Y. (2020). The properties, biotechnologies, and applications of antifreeze proteins. Int. J. Biol. Macromol. 153, 661–675. doi:10.1016/j.ijbiomac.2020.03.040
Xie, Z., Chen, X., Chen, D., Chen, W., and Zhao, Y. (2021). Initial design and implementation of a space quadruped crawling robot prototype. Adv. Astronaut. Sci. Technol. 4, 173–181. doi:10.1007/s42423-021-00090-5
Xin, Y., Tian, H., Guo, C., Li, X., Sun, H., Wang, P., et al. (2016). A biomimetic tactile sensing system based on polyvinylidene fluoride film. Rev. Sci. Instrum. 87, 025002. doi:10.1063/1.4941736
Xu, B., Yang, Y., Zhang, B., Yan, Y., and Yi, Z. (2020). Bionic design and experimental study for the space flexible webs capture system. IEEE Access 8, 45411–45420. doi:10.1109/ACCESS.2020.2978108
Xu, Q., Zhang, W., Dong, C., Sreeprasad, T. S., and Xia, Z. (2016). Biomimetic self-cleaning surfaces: Synthesis, mechanism and applications. J. R. Soc. Interface 13, 20160300. doi:10.1098/rsif.2016.0300
Yan, G., Zou, H. X., Wang, S., Zhao, L. C., Wu, Z. Y., and Zhang, W. M. (2021). Bio-inspired vibration isolation: Methodology and design. Appl. Mech. Rev. 73. doi:10.1115/1.4049946
Yang, J., Gu, D., Lin, K., Zhang, Y., Guo, M., Yuan, L., et al. (2022). Laser additive manufacturing of bio-inspired metallic structures. Chin. J. Mech. Eng. Addit. Manuf. Front. 1, 100013. doi:10.1016/j.cjmeam.2022.100013
Yang, S., and Jiang, L. (2020). Biomimetic self-assembly of subcellular structures. Chem. Commun. 56, 8342–8354. doi:10.1039/D0CC01395A
Yang, Y., Song, X., Li, X., Chen, Z., Zhou, C., Zhou, Q., et al. (2018). Recent progress in biomimetic additive manufacturing technology: From materials to functional structures. Adv. Mater. 2018, e1706539. doi:10.1002/adma.201706539
Yang, Y., and Zhang, W. (2014). Et arm: Highly compliant elephant-trunk continuum manipulator. ICIRA 2014, 288–299. doi:10.1007/978-3-319-13966-1_29
Yaraghi, N. A., and Kisailus, D. (2018). Biomimetic structural materials: Inspiration from design and assembly. Annu. Rev. Phys. Chem. 69, 23. doi:10.1146/annurev-physchem-040215-112621
Yen, J., Helms, M., Goel, A., Tovey, C., and Weissburg, M. (2014). “Adaptive evolution of teaching practices in biologically inspired design,” in Biologically inspired design. Editors A. Goel, D. A. McAdams, and R. B. Stone, 153–199. doi:10.1007/978-1-4471-5248-4_7
Yi, Z., Zhang, Y., and Peters, J. (2018). Biomimetic tactile sensors and signal processing with spike trains: A review. Sensors Actuators A Phys. 269, 41–52. doi:10.1016/j.sna.2017.09.035
Yilmaz, Ö., Aouf, N., Checa, E., Majewski, L., and Sanchez-Gestido, M. (2017). Thermal analysis of space debris for infrared based active debris removal. Proc. IMechE Part G. J. Aerosp. Eng. 2017, 1–13. doi:10.1177/ToBeAssigned
Yoon, J., Hou, Y., Knoepfel, A. M., Yang, D., Ye, T., Zheng, L., et al. (2021). Bio-inspired strategies for next-generation perovskite solar mobile power sources. Chem. Soc. Rev. 50, 12915–12984. doi:10.1039/D0CS01493A
Yu, D., Wang, G., and Zhao, Y. (2018). On-orbit measurement and analysis of the micro-vibration in a remote-sensing satellite. Adv. Astronaut. Sci. Technol. 1, 191–195. doi:10.1007/s42423-018-0027-z
Yu, X., Liu, C., Zhang, Y., Xu, H., Wang, Y., and Yu, W. (2020). Multispectral curved compound eye camera. Opt. Express 28, 9216–9231. doi:10.1364/OE.385368
Yu, Y. S. W., Graff, M. M., and Hartmann, M. J. Z. (2016). Mechanical responses of rat vibrissae to airflow. J. Exp. Biol. 219, 937–948. doi:10.1242/jeb.126896
Yue, S., Rind, F. C., Keil, M. S., Cuadri, J., and Stafford, R. (2006). A bio-inspired visual collision detection mechanism for cars: Optimisation of a model of a locust neuron to a novel environment. Neurocomputing 69, 1591–1598. doi:10.1016/j.neucom.2005.06.017
Zeng, Z., Wang, C., Siqueira, G., Han, D., Huch, A., Abdolhosseinzadeh, S., et al. (2020). Nanocellulose-mxene biomimetic aerogels with orientation-tunable electromagnetic interference shielding performance. Adv. Sci. (Weinh). 7, 2000979. doi:10.1002/advs.202000979
Zhang, J., Wu, F., Wang, C., Mei, Z., Han, A., and Xie, D. (2020). The effect of suction side tubercles on torque output of a steam turbine low-pressure last stage blade. Energies 13, 1889. doi:10.3390/en13081889
Zhang, K., Lu, K., Gu, X., Fu, C., and Zhao, S. (2021). Dynamic behavior analysis and stability control of tethered satellite formation deployment. Sensors (Basel, Switz. 22, 62. doi:10.3390/s22010062
Zhang, W., Hu, Y., Li, Y., Ma, K., Wei, Y., Yang, J., et al. (2022a). Versatile like a seahorse tail: A bio-inspired programmable continuum robot for conformal grasping. Adv. Intell. Syst. 4, 2200263. doi:10.1002/aisy.202200263
Zhang, W., Xu, J., and Yu, T. X. (2022b). Dynamic behaviors of bio-inspired structures: Design, mechanisms, and models. Eng. Struct. 265, 114490. doi:10.1016/j.engstruct.2022.114490
Zhao, P., Li, B., Shi, W., Wang, W., Zhang, K., Yan, Z., et al. (2017). “The model research of satellite space laser communication based on compound eye array,” in 14th International Bhurban Conference on Applied Sciences & Technology (IBCAST), 722–726. doi:10.1109/IBCAST.2017.7868132
Zhao, P., Liu, J., and Wu, C. (2020). Survey on research and development of on-orbit active debris removal methods. Sci. China Technol. Sci. 63, 2188–2210. doi:10.1007/s11431-020-1661-7
Zhao, S., Du, H., Ma, Z., Xiao, G., Liu, J., Jiang, Y., et al. (2022). Efficient fabrication of ternary coupling biomimetic superhydrophobic surfaces with superior performance of anti-wetting and self-cleaning by a simple two-step method. Mater. Des. 223, 111145. doi:10.1016/j.matdes.2022.111145
Zhao, Y., Jin, L., Zhang, P., and Li, J. (2018). Inverse displacement analysis of a hyper-redundant elephant’s trunk robot. J. Bionic Eng. 15, 397–407. doi:10.1007/s42235-018-0030-z
Zhou, B., Han, G., Zhang, Z., Li, Z., Feng, Y., Ma, J., et al. (2021). Aramid nanofiber-derived carbon aerogel film with skin-core structure for high electromagnetic interference shielding and solar-thermal conversion. Carbon 184, 562–570. doi:10.1016/j.carbon.2021.08.067
Zhou, D., Chen, F., Handschuh-Wang, S., Gan, T., Zhou, X., and Zhou, X. (2019). Biomimetic extreme-temperature- and environment-adaptable hydrogels. ChemPhysChem 20, 2139–2154. doi:10.1002/cphc.201900545
Keywords: biomimetics, bio-inspired, space systems, on-orbit manufacture, debris removal, solar power
Citation: Banken E and Oeffner J (2023) Biomimetics for innovative and future-oriented space applications - A review. Front. Space Technol. 3:1000788. doi: 10.3389/frspt.2022.1000788
Received: 22 July 2022; Accepted: 02 December 2022;
Published: 07 March 2023.
Edited by:
Ugo Lafont, European Space Research and Technology Centre (ESTEC), NetherlandsReviewed by:
Lorenzo Scalera, University of Udine, ItalyCopyright © 2023 Banken and Oeffner. This is an open-access article distributed under the terms of the Creative Commons Attribution License (CC BY). The use, distribution or reproduction in other forums is permitted, provided the original author(s) and the copyright owner(s) are credited and that the original publication in this journal is cited, in accordance with accepted academic practice. No use, distribution or reproduction is permitted which does not comply with these terms.
*Correspondence: Elisabeth Banken, ZWxpc2FiZXRoLmJhbmtlbkBjbWwuZnJhdW5ob2Zlci5kZQ==
Disclaimer: All claims expressed in this article are solely those of the authors and do not necessarily represent those of their affiliated organizations, or those of the publisher, the editors and the reviewers. Any product that may be evaluated in this article or claim that may be made by its manufacturer is not guaranteed or endorsed by the publisher.
Research integrity at Frontiers
Learn more about the work of our research integrity team to safeguard the quality of each article we publish.