- 1The Abdus Salam International Centre for Theoretical Physics, Trieste, Italy
- 2IDEA, Fundación Instituto de Estudios Avanzados, Caracas, Bolivarian Republic of Venezuela
This review of miniaturised instrumentation is motivated by the ongoing and forthcoming exploration of the confirmed, or candidate ocean worlds of the Solar System. It begins with a section on the evolution of instrumentation itself, ranging from the early efforts up to the current rich-heritage miniaturised mass spectrometers approved for missions to the Jovian system. The geochemistry of sulphur stable isotopes was introduced for life detection at the beginning of the present century. Miniaturised instruments allow the measurement of geochemical biosignatures with their underlying biogenic coding, which are more robust after death than cellular organic molecules. The role of known stable sulphur isotope fractionation by sulphate-reducing bacteria is discussed. Habitable ocean worlds are discussed, beginning with analogies from the first ocean world known in the Solar System that has always being available for scientific exploration, our own. Instrumentation can allow the search for biosignatures, not only on the icy Galilean moons, but also beyond. Observed sulphur fractionation on Earth suggests a testable “Sulphur Hypothesis”, namely throughout the Solar System chemoautotrophy, past or present, has left, or are leaving biosignatures codified in sulphur fractionations. A preliminary feasible test is provided with a discussion of a previously formulated “Sulphur Dilemma”: It was the Galileo mission that forced it upon us, when the Europan sulphur patches of non-ice surficial elements were discovered. Biogenic fractionations up to and beyond δ34S = −70‰ denote biogenic, rather than inorganic processes, which are measurable with the available high sensitivity miniaturised mass spectrometers. Finally, we comment on the long-term exploration of ocean worlds in the neighbourhood of the gas and ice giants.
Revisiting the “Sulphur Dilemma”
Information on Ocean Worlds that is Coded in Stable Isotopes
We should take a look at what the Galileo mission taught us, regarding the search for life. In the near future, with the JUpiter ICy moons Explorer (JUICE) mission Grasset et al. (2013a) and the NASA proposed Europa Clipper mission (Howell and Pappalardo, 2020), there are several options for interpreting the information that is coded in the sulphur isotopes that have been deposited on the Galilean moons.
The epoch-making Galileo Mission was responsible for the discovery of intriguing surficial deposits of sulphur (patches) on Europa’s frozen surface. Subsequent measurements ratified this discovery. It was the first proposal that was put forward, that these chemical elements may be exogenous, namely that their origin may have been from the neighbouring satellite Io. Sulphur was detected by the Galileo mission on the Europan surficial patches: The presence of sulphur compounds on the Europan surficial ice was confirmed by NIMS measurements, Near-Infrared Mapping Spectrometer (Carlson et al., 2002). The data matches the distribution of an ultraviolet absorber that had been suggested earlier McEwen (1986), and confirmed later on (Grundy et al., 2007). A suite of measurements, still from Galileo have led us to the conclusion that the source of the surficial sulphur is endogenous, including, Solid-State Imaging (SSI), NIMS and the Ultraviolet Spectrometer (UVS) (Fanale et al., 1999).
A Europa Lander Mission Concept for Solving the Sulphur Dilemma
The Europan endogenous surficial sulphur could have been metabolically processed by a submarine biota. This hypothesis is testable, as at the level of isotopic modifications the sulphur could have left measurable traces. They would be detectable with the sensitivity of the available instrumentation that has already been approved for the forthcoming missions. All of these queries can be summarised in a Sulphur Dilemma, which was first formulated soon after the Galileo mission ended its activities on September 23, 2003 (Chela-Flores, 2006):
What is the source of the S patches? Could they be endogenous and at the same time biogenic?
A NASA Europa lander, which at present is only a proposed astrobiology mission concept Pitesky and Hand (2020) has, nevertheless, been advocated for over two decades (Phillips and Chyba, 2001). Such a lander could search for the biosignatures, which we have pointed out in earlier publications and especially in this review. Likely sites for can be retrieved from the NIMS data (cf., Information On Ocean Worlds that is Coded in Stable Isotopes). These measurements suggest that a Europa lander be placed especially on the area close to 0–30°N and longitudes 240 and 270, in correspondence with the S patches, as suggested by our comments above (McCord et al., 1998).
Autochthonous Microbes Could Alter the Surface of Europa
It is possible to answer the question whether the early Earth microbial life could mirror the emergence of Europan microorganisms, at least as far as their biochemistry is concerned. Metabolism of autochthonous microbes could alter substantially the S surficial deposits. The answer lies encoded in the isotopic fractionation of the sulphur atoms that would have been involved. Such alterations are measurable with the accuracy that is available in the latest miniaturised mass spectrometers that would be compatible with payloads allowed for a Europa lander.
Ocean Worlds Past and Present
Past Ocean Worlds on Venus and Mars
Both Venus and Mars were possible hosts of ancient oceans, probably not unlike the terrestrial ones.Firstly, the possibility of an early Venusian life-friendly environment was raised earlier on (Donahue et al., 1982). In addition, on the question of habitability of Venus, additional papers were published in the early 1980s: Firstly, numerical simulations suggested that habitability of Venusian oceans was not excluded in its first billion years, with oceans persevering for possible up to twice that time (Grinspoon and Bullock, 2007). There is evidence that initially Venus was an ocean world (Kasting et al., 1984; Kasting, 1988; Donahue and Russell, 1997; Grinspoon and Bullock, 2003).
In addition to the early approaches to the question of an early Venusian ocean, a recent three-dimensional model militates in favour of the ocean lasting until recent geological times, including potential habitability conditions (Way et al., 2016). The possibility of some form of extremophilic life has also been discussed in a variety of possible environments and the possibility of panspermia to and from our own planet (Schulze-Makuch and Irwin, 2004).
Secondly, on a second terrestrial planet—Mars—there could have been a global ocean in its northern hemisphere, so that the Red Planet can be added to the list of (past) ocean worlds (Clifford and Parker, 2001; Fairén et al., 2003).
Volcanic eruptions, contemporary with the presence of the early ocean, were possible sources of abundant sulphur compounds. These chemical elements would be expected to have been deposited on the ancient Martian surface. An early ecosystem can be tested by ruling out, or detecting, fractionation codified in the sulphur compounds that were deposited. Testing S isotopes as biosignatures is a possibility that has already been raised for Mars (Chela-Flores, 2018). Such identification of biosignatures will be in a more favourable position with the forthcoming landing missions Tianwen-1 (CNSA, landed on May 14, 2021), Perseverance (NASA, landed on February 18, 2021) and Rosalind Franklin (Roscosmos and ESA, expected to land in 2023).
Analogies From the First Ocean World Known in the Solar System
Some of the confirmed ocean worlds (OWs) have icy surfaces over subsurface oceans. On Earth we have a valuable analogy in Antarctica’s McMurdo Dry Valley Lakes (DVL). These lacustrine environments (Doran et al., 2010; Chela-Flores and Seckbach, 2011) have biotopes that are known to survive under difficult constraints, including being permanently covered by ice (cf., Tables 1–3).
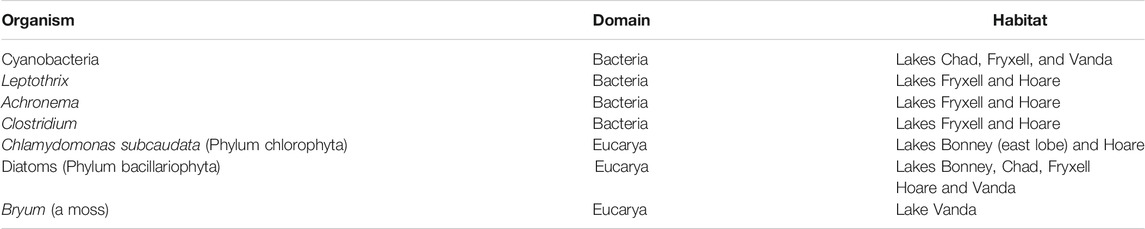
TABLE 2. Microbial life in the Dry Valleys lakes, Antarctica (Chela-Flores, and Seckbach, 2011).
Lake Joyce is an interesting lake, also ice covered all the year-round. An analogous water environment lying underneath an icy cover is Lake Untersee, whose maximum depth is 169 m (Wand et al., 1997). Being in central Queen Maud Land in East Antarctica, Untersee is significant as an analogy for the ocean worlds: The lake ice cover is 2–6 m thick which may have persisted for over 100,000 years. Below 80 m hydrogen sulfide is present, associated with decreased sulfate concentrations, probably arising from bacterial reduction of sulfate. It is known that stromatolites are present in the lake bottom (Andersen et al., 2011). But resurfacing also hints at submerged geologic activity. The icy Galilean moons cryovolcanism may resemble terrestrial silicate volcanism. Earth-like geologic activity includes a candidate for the origin of life, namely, hydrothermal vents (Wächtershäuser, 1990).
Confirmed Ocean Worlds in the Jovian System
Going beyond the Martian orbit, we could persevere with a systematic search for sulphurous material on the surface of the Galilean icy worlds. We may assume a testable “Sulphur Hypothesis” (throughout the Solar System chemoautotrophy, past or present, has left, or are leaving biosignatures codified in sulphur fractionations).
Under this hypothesis, the non-ice chemical elements may have their source in the moon’s interior biota; for a test we should identify surficial locations, where it is most likely to detect chemical elements arising from the subsurface ocean. One prominent spot is undoubtedly the dark and red-coloured material in the young depression Castalia Macula (0°N, 225°W), which has been pointed out by Louise Prockter and Paul Schenk in the first decade of this century (Prockter and Schenk, 2005). The tests we have proposed earlier (cf., Stable Isotopes From Ore Genesis Can Be Used as Biosignatures) can detect, or rule out, the presence of a significant biogenic signal if surficial sulphur has been processed by oceanic microbial life.
One of the natural phenomena that could contribute to yield the ocean chemical contents in places like Castalia Machia is cryovolcanism (Fagents, 2003). The patchy nature of the surficial S deposits argues strongly against the possible source being from the neighbouring volcanic Galilean moon, Io (Carlson et al., 1999).
In the foreseeable future, direct geochemical tests on the furthest ocean worlds are not possible, but as we will see in this section much information has been gathered for including their moons, as either confirmed, or candidate ocean worlds.
Confirmed Ocean Worlds in the Saturnian System
Two satellites of Saturn have been the focus of attention for several missions including the two confirmed ocean worlds Enceladus and Titan (Hendrix et al., 2018). Enceladus Explorer is a mission concept by the German Aerospace Center together with German universities for an unprecedented coupled system for probing this moon’s exterior, orbiter and its interior, at least its surficial ice (cf., Table 4 for references and full names of all the missions referred to below). In addition, coming from NASA, Christopher McKay, and colleagues have proposed to measure biosignatures on Enceladus, the ELSAH mission, with a payload that would include a sophisticated instrumentation, for mass spectrometry (McKay, 2008). On the other hand, and once again focusing on the same intriguing ocean world, the ELF mission hopes to probe its subsurface ocean. Instead, not only for Enceladus, but also for Titan, the E2T mission is an international collaboration between two major space agencies dedicated to both Enceladus and Titan.
In spite of the rich assortment of options, in 2019 NASA gave a priority in their New Frontier Programme to the mission Dragonfly to Titan to characterize the habitability of Titan. The Dragonfly would use a lander with rotors to take off, fly, and land at new sites to characterize the habitability of Titan’s environment and to search for biosignatures.
Finally, there is a set of another four Saturnian satellites that are presently candidate ocean worlds: Mimas, Tethys, Rhea, Iapetus, where there is only basic foundation for this possibility (Hendrix et al., 2018). However, new missions for the Saturnian system is required to promote these four Saturnian moons to the rank of confirmed ocean worlds. Exceptionally, at the end of 2004 and in 2007, there were close flybys of Iapetus during the Cassini mission.
The Evolution of Instrumentation up to Miniaturised Mass Spectrometers
The Exploration of Confirmed or Candidate Ocean Worlds
In the long-term planning, the search of biosignatures should focus mainly on two of the Galilean moons, Europa and Ganymede (cf., The Robustness of Geochemical Biosignatures), since there are missions by ESA and NASA that in the short term will be equipped with appropriate instrumentation. The case of Mars as a previous ocean world is also discussed. Clearly, it is instructive to review the evolution of instrumentation towards a stage when they have been approved by the space agencies.
Cryobots and Hydrobots for the Exploration of Ocean Worlds
Soon after the Galileo mission arrived in the Jovian system, it was suggested that for icy moons with internal oceans coupled instruments could be useful. The hydrobot-cryobot intended to melt through the icy surface into the ocean underneath (cf., Figure 1A).
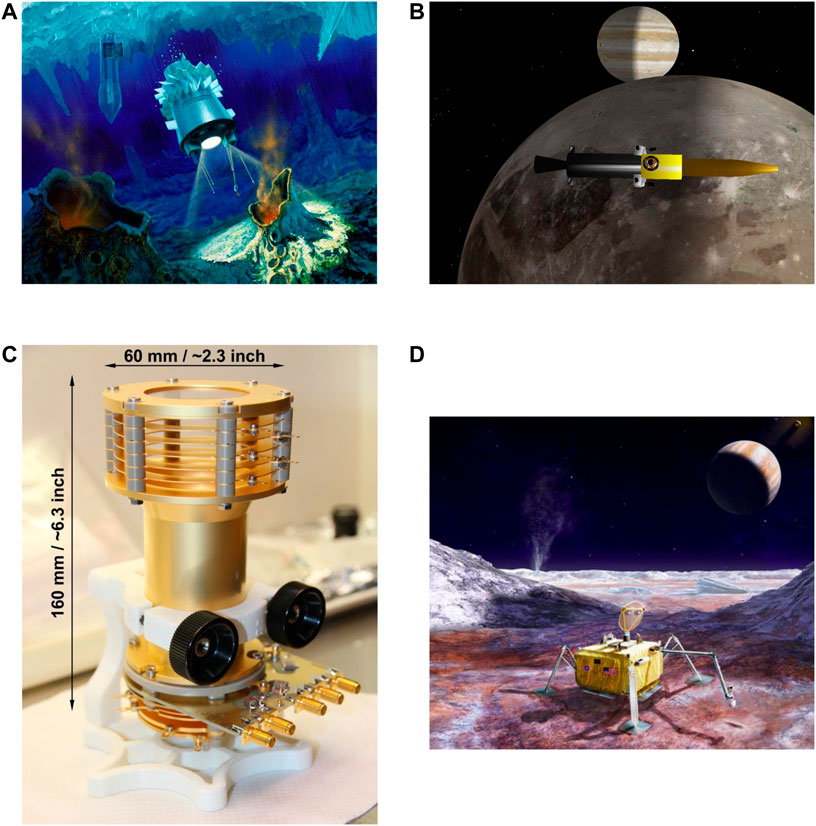
FIGURE 1. A vehicle referred to as a cryobot was intended to carry a small submersible (a hydrobot) equipped with a relevant payload. The hydrobot was based on previous submersible experience for research in oceanography (Horvath et al., 1997). Image courtesy NASA/JPL-Caltech. As an alternative to landers in space exploration, penetrators were high speed impacting projectiles designed to investigate the subsurface of icy moons, including Europa, advocated by the UK Penetrator Consortium and European proposers, besides our Abdus Salam International Centre for Theoretical Physics, Trieste, Italy (Gowen et al., 2011), a technological initiative to overcome challenges of the standard exploration, carried on in 2021, but more significantly still, the strategy has been advocated for the direct exploration of the icy surface of Europa and other Galilean worlds in our cosmic neighbourhood. Image published with permission of the Mullard Space Science Laboratory (UCL, UK). The miniature mass analyzer of the LIMS system of the University of Bern is displayed. The mass analyzer is held by a structure for bench testing before it will be integrated into the vacuum chamber. CC the University of Bern, Switzerland.An artist conception of a mission concept, for a first lander on an extraterrestrial ocean world, a Europa lander (Pitesky and Hand, 2020). Image courtesy NASA/JPL-Caltech.
It is at present no longer being considered feasible as we proposed it. However, we should underline that the cryobot-type of instrumentation, as a melting drill head for the exploration of subsurface planetary ice layers has subsequently attracted the attention of researchers (Weiss et al., 2008).
The question remains open whether in the future the exploration of ocean worlds may benefit from a more advanced version of hydrobots. For instance, the proposal of a hydrobot submersible has been used subsequently by the concept mission ENDURANCE. This probe could eventually be used for the direct exploration of an ocean world, such as Europa (Bortman, 2010). The possibility of extremophiles that are known on terrestrial conditions to persevere and survive in anoxic conditions, can be thought of as models of possible Europan biota that a hydrobot-type of probe could eventually detect with an appropriate payload.
Penetrators as Alternative Instruments for Ocean Worlds
A second suggestion that we found as an attractive instrument for probing the icy surfaces of the ocean worlds was called a penetrator (Gowen et al., 2011, cf., Figure 1B), which had previously been suggested for use in the context of lunar exploration (Smith et al., 2009).
These mini-missiles would have been delivered from orbit to reach and penetrate the icy surface. In spite of its advantages some subsequent variations the main space agencies have not included them in payloads of future missions. Yet, at present there is still considerable interest in penetrators (Bagrov et al., 2021).
It should not escape our attention that the basic idea of the penetrator, as a means to deliver an impacting descent probes for the Galilean moons has subsequently been extended. The alternative projectile has been suggested in order to simplify the costs and technological challenges of direct exploration of the icy surfaces of ocean worlds with landers (Wurz et al., 2017). But the possible alternative impacting probe would be an implementation of an earlier Europa Descent Probe of approximately cubic shape, unlike the traditional cylindrical penetrator.
After these pioneering efforts for exploring the ocean worlds, which we have illustrated in Figure 1A,B, a significant string of publications has followed with the view of participating in the forthcoming missions to the Galilean moons. We have presented the corresponding evolution of instrumentation as the timeline in Table 5:

TABLE 5. Timeline: instrumentation for ocean worlds. The early preliminary efforts (1997–2011), in which the author participated, are illustrated in Figures 1A,B. The more recent miniaturised instruments that are meant to contribute to the exploration of the nearest Galilean ocean worlds, are available in several papers mainly from the University of Bern, Switzerland, have been cited in Heritage of Miniaturised Instruments for Forthcoming Missions and illustrated in Figure 1C.
Heritage of Miniaturised Instruments for Forthcoming Missions
The most successful instruments that have been selected for forthcoming space missions are mass spectrometers. From the point of view of probing the ocean worlds for biosignatures, they have many advantages to which we shall return in Biosignatures From the Geochemistry of Stable Isotopes. Their most attractive feature is the degree of miniaturisation that has been achieved (Tulej et al., 2015).
Miniaturisation of mass spectrometers has been developed specifically for space exploration. In various previous applications, miniaturised mass spectrometers have been used in the payload of space orbiters, as well as in rovers. There are many studies aiming at the exploration of the Solar System (Tulej et al., 2011; Tulej et al., 2016; Wiesendanger et al., 2017; Wiesendanger et al., 2018a; Wiesendanger et al., 2018b).
In Figure 1C, we illustrate a recent example of the process of miniaturisation of mass spectrometry. It shows one of its components—a mass analyzer—which takes ionized masses and separating them according to charge to mass ratios. This instrument is responsible to deliver the information received to the detector, where it is converted into a digital output.
Some more exciting recent research is relevant in this context. Firstly, cutting-edge instrumentation that is currently ready for the flight on the PEP JUICE mission (including the NIM instrument), which will fly in 2022. The NIM instrument is designed to measure exospheres of Jupiter satellites during flyby (Lasi et al., 2020). This work addresses instruments designed for a future Europa lander. Secondly, miniaturized mass spectrometry with the intention of being included in future payloads is also being considered (Föhn, et al., 2021). Finally, some work now is focusing on a lander instrument on Europa, particularly on a coupling of the mass analyser with sample introduction/laser ablation/desorption ion source (Origin instrument). This instrument is more like LMS with the laser ablation/desorption ion source (Ligterink, et al., 2020).
To implement the experimental tests on biogeochemical biomarkers on Europa and Ganymede, we have to wait some time for the results of two forthcoming missions.
There are two possibilities for the use this instrumentation in the exploration of the icy Galilean moons. The first of the two missions to launch will be the ESA mission JUpiter ICy moons Explorer (JUICE), whose main objective will be on Ganymede (Grasset et al., 2013b). In this mission there has been significant progress in relevant miniaturised mass spectrometry. In fact, JUICE will include in its payload a Particle Environment Package, which includes a Neutral and Ion Gas Mass Spectrometer (Abplanalp et al., 2009; Meyer et al., 2017). Potentially, this new instrumentation is capable of testing whether isotopes of sulphur can be used as biomarkers, as explained in Biosignatures From the Geochemistry of Stable Isotopes below.
The second mission to launch will be the NASA Europa Clipper mission. After the major work achieved by the Galileo mission, it intends to investigate habitability of Europa. It has the capability to measure biogenic stable S-isotope fractionation. Besides, a mission concept for a landed spacecraft to the surface of Europa is under consideration. The MAss SPectrometer for Planetary EXploration/Europa (MASPEX) will be included in the Europa Clipper scientific payload (Pappalardo et al., 2013).
Even as a mission concept, a Europa lander remains an appealing project of NASA (Figure 1D).
This lander has been discussed in the scientific community for a long time, going back at least to the Sixth Trieste Conference (Phillips and Chyba, 2001). The possibility of including a mass spectrometer in its payload Pitesky and Hand (2020) would add significantly to the first possibility of testing the Sulphur Hypothesis, a critical question in efforts to search for biognatures (cf., Biosignatures From the Geochemistry of Stable Isotopes).
Biosignatures From the Geochemistry of Stable Isotopes
The Robustness of Geochemical Biosignatures
The distribution of life in the Universe is the third “chapter” of astrobiology, which has been given a suggestive name, a Second Genesis (McKay et al., 2001). We are suggesting in this paper and some of our previous ones that one way to search for a second genesis is with the help of stable isotope biochemistry. Metabolic alteration of the environment, seems to be more robust than the alternative search for the biomolecules of life, amongst them the amino acids, lipids or the monomers of the nucleic acids, as it is often done (Zhang et al., 2021). To test for the difference between biogenic as opposed to abiogenic remains as one of the outstanding challenges in astrobiology (McKay, 2008).
Stable Isotopes From Ore Genesis Can Be Used as Biosignatures
Ore is a term which applies to any mineral (metalliferous, or non-metal) from which the metallic, or non-metallic element may be profitably extracted. Ore genesis refers to mineral deposits formation underneath the terrestrial surface. Based on the analysis of (34S/32S), petroleum geologists used the analysis of ore genesis for understanding the provenance of sulphur. Besides, the light stable isotopes, including S, have provided information about mineral deposition, demonstrating that ore formation is a surficial phenomenon, rather than having their origin in magmas. This topic has been reviewed earlier (Ohmoto, 1986). As opposed to the above work on ore genesis, astrobiologists can use the experience of geochemists by taking advantage of an extraordinary phenomenon in microbiology that has been widely studies since the middle of last century (Kaplan, 1975): sulphur is highly fractionated by microbes, in such a way that biogenically processed S can readily be distinguished from terrestrial mantle sulphur (Ireland, 2013). This is a remarkable phenomenon in the context of astrobiology. Indeed, biogeochemical biomarkers have long been used in astrobiology (Chela-Flores, 2006). This approach for the detection of biologic, rather than inorganic fractionation processes, is currently being exploited in a terrestrial context (Kring et al., 2021). This work goes back to its roots based on the well-established studies of ore genesis.
We refer to our earlier discussions for details related to the introduction of the “delta parameter” δ34S. This term is significant for the search of geochemical biosignatures (Chela-Flores and Seckbach, 2011; Chela-Flores et al., 2015):
where a standard is chosen referring to a troilite from the Barringer crater in the Canyon Diablo meteorite (CDM). To sum up, we already possess the technology which will facilitate the measurements with the necessary sensibility to test the icy surface for signs of life in the Europan ocean.
Distribution of Sulphur in the Solar System
In spite of remarkable progress in chemical evolution since the landmark paper of Stanley Miller (1953), there is yet no consensus for a theory of life’s origin (Davis and McKay, 1996). This situation suggests that one possibility to make further progress in life’s emergence would be to make testable hypotheses to find pathways for deeper aspects of the origin of life in the Universe.
There is wide past experience in assessing the presence of biogenicity in terrestrial environments. (Canfield and Thamdrup, 1994; Kohn et al., 1998; Popa et al., 2004; Shen and Buick, 2004; Sim et al., 2011; Kring et al., 2021).
Motivated by the Solar System S abundances, we adopt a ‘Sulphur Hypothesis':
Throughout the Solar System, sulphur-processing microorganisms emerged early and have left, or are leaving, biosignatures codified in sulphur fractionations.
This is a testable hypothesis with the available instrumentation. One motivation for the Hypothesis is the role in chemoautotrophy by a type of bacteria that consumes sulphate and produces sulphide, as a waste product. These microorganisms are ancient sulphate-reducers. We will return to them.
For a long time, we have known from the exploration of the inner Solar System that sulphur is ubiquitous (Gibson, 1982), examples of which have been given more recently. Sulphur is present, not only on the Earth, but also on the other terrestrial planets: Mercury Weider et al. (2016), Venus Sandor et al. (2010) and Mars (McLennan and Grotzinge, 2009). But more significantly, the missions Galileo and Cassini support this view, since the giant planets have no less abundances of “metals” (elements heavier than helium), relative to hydrogen than the inner planets. Indeed, in those giant planets S abundances are much higher than observed in the Sun (Owen et al., 1999). In fact, the Jovian S abundance (measured by Galileo) exceeds Solar by a factor of three (Atreya et al., 2003).
Returning to our own planet, sulphur in the fossil record extends some three billion years before the present. There is evidence for the ancient emergence of sulphate-reducing microorganisms, which go back to the Archean: The phenomenon of sulphate reduction has been confirmed in terrestrial sediments 3.47 billion years before the present. At that time, in Australia they produced strong stable isotope depletions in pyrite embedded in barite (Shen et al., 2001; Shen and Buick, 2004).
Discussion of Innovative Missions for the Gas and Ice Giants
Biogeochemical Biosignatures Beyond Mars and Jupiter
Due to the large distances involved, in the foreseeable future it will not be possible to apply the robust biogeochemical biosignatures discussed in Biosignatures From the Geochemistry of Stable Isotopes of the present review, but the planning still continues in the main space agencies. In spite of this handicap, the preliminary efforts of previous missions, Cassini, New Horizons and Dawn have given us a general view of the 19 confirmed and candidate ocean worlds of the Solar System, although, alas it will not be in the near future when we will have the first indications of an extant inhabited world in our cosmic neighbourhood.
In Table 6 we have gathered information on the exploration of some of the possibly habitable worlds of the outer Solar System of which we have little information. Some mission concepts have been formulated for some time now. Even though they have not received support from the main space agencies, they should be considered amongst astrobiology’s most urgent future projects.
Candidate Ocean Worlds Amongst the Moons of the Ice Giants
The search for biosignatures should be an objective for the Ice Giants Uranus and Neptune, especially the candidate ocean worlds amongst their moons: The Neptunian satellite Triton and the five Uranian moons, which are candidates for ocean worlds (Miranda, Ariel, Umbriel, Titania, Oberon). In spite of the fact that they are likely ocean worlds, in the future we still need orbiters around Uranus, as in the past it was the case for the Saturnian system with its Cassini mission (Hofstadter et al., 2017).
In Table 7 we have gathered some proposals of missions, not supported in the current programmes of the main space agencies, which may throw some light on additional ocean moons around and beyond Uranus and Neptune, for instance: the Neptunian moon Triton, Pluto, Ceres, and the Saturnian moon Dione.
Candidate Ocean Worlds in Our Cosmic Neighbourhood
We have some remarkable moons in the outer Solar System, Triton being one of them (Gaeman et al., 2012; Nimmo and Spencer, 2014). It is conceivable that the geochemical biosignatures could eventually be applicable in those strange environments. The work of Hussmann and co-workers (2006) has taken into consideration, not only the above-mentioned satellite of Neptune, but in addition, moons of Saturn, Uranus, and Pluto as well (Rhoden et al., 2015).
Even tantalising opportunities are waiting in planetary science for deeper insights on the ocean worlds beyond the gas giants (Chela-Flores, 2017). But as mentioned in Biogeochemical Biosignatures Beyond Mars and Jupiter, this aspect of the exploration of the Solar System has not been favoured by the space-faring nations. Some of the most significant proposals for future missions to the ice giants await the exploration of the least known ocean worlds of our cosmic neighbourhood.
Author Contributions
The author confirms being the sole contributor of this work and has approved it for publication.
Funding
This review follows a kind invitation from one of the associate editors to join the very new section Microgravity in the Frontiers journal Space Technology. We acknowledge our gratitude for having been granted a full waiver.
Conflict of Interest
The author declares that the research was conducted in the absence of any commercial or financial relationships that could be construed as a potential conflict of interest.
Publisher’s Note
All claims expressed in this article are solely those of the authors and do not necessarily represent those of their affiliated organizations, or those of the publisher, the editors and the reviewers. Any product that may be evaluated in this article, or claim that may be made by its manufacturer, is not guaranteed or endorsed by the publisher.
Acknowledgments
The author would like to thank Professor Alan Smith, Director Space Domain, Mullard Space Science Laboratory, University College London for kind permission to publish Figure 1B. He is also grateful to Marek Tulej, member of Laser Mass Spectrometry Laboratory in Physics Institute Planetary Sciences and Space Research Division, University of Bern, Switzerland, for his wise counselling regarding recent work on instrumentation that is intended for exploration of the Jovian ocean worlds. He would also like to thank Ian Crawford, Professor of Planetary Science and Astrobiology, Department of Earth and Planetary Sciences, Birbeck Colleege, University of London, UK for help in the later stages of writing the present work.
References
Abplanalp, D., Wurz, P., Huber, L., Leya, I., Kopp, E., Rohner, U., et al. (2009). A Neutral Gas Mass Spectrometer to Measure the Chemical Composition of the Stratosphere. Adv. Space Res. 44, 870–878. doi:10.1016/j.asr.2009.06.016
Andersen, D. T., Sumner, D. Y., Hawes, I., Webster-brown, J., and McKay, C. P. (2011). Discovery of Large Conical Stromatolites in Lake Untersee, Antarctica. Geobiology. 9 (3), 280–293. doi:10.1111/j.1472-4669.2011.00279.x
Arridge, C. S., Achilleos, N., Agarwal, J., Agnor, C. B., Ambrosi, R., André, N., et al. (2014). The Science Case for an Orbital Mission to Uranus: Exploring the Origins and Evolution of Ice Giant Planets. Planet. Space Sci. 104, 122–140. doi:10.1016/j.pss.2014.08.009
Atreya, S. K., Wong, M. H., Owen, T. C., Mahaffy, P. R., and Niemann, H. B. (2003). Comparison of the Atmospheres of Jupiter and Saturn: Deep Atmospheric Composition, Cloud Structure, Vertical Mixing, and Origin. Planet. Space Sci. 47, 1243–1262. doi:10.1016/s0032-0633(99)00047-1
Bagrov, A., Leonov, V. A., Leun, E. V., and Sysoev, V. K. (2021). Hyperspeed Penetrator to Deliver Research Equipment to Interstellar Wanderers. Conference: XLIV ACADEMIC SPACE CONFERENCE: Dedicated to the Memory of Academician S.P. Korolev and Other Outstanding Russian Scientists – Pioneers of Space Exploration. AIP Conf. Proc. 2318 (1), 190002. doi:10.1063/5.0038658
Barnes, J. W., Turtle, E. P., Trainer, M. G., and Lorenz, R. (2017). Dragonfly: Exploring Titan's Surface with a New Frontiers Relocatable Lander. Am. Astronomical Soc. DPS meeting #49, id.219.02.
Bocanegra-Bahamón, T., Colm, B., Marc Costa, S., Dominic, D., Ingo, G., Kostas, K., et al. (2015). MUSE – Mission to the Uranian System: Unveiling the Evolution and Formation of Ice Giants. Adv. Space Res. 55, 2190–2216. doi:10.1016/j.asr.2015.01.037
Bortman, H. (2010). Antarctic Diving Robot Practices for Europa. Available at: http://www.ictp.it/∼chelaf/Antarctic_robot_for_Europa.pdf.
Canfield, D., and Thamdrup, B. (1994). The Production of 34S-Depleted Sulfide During Bacterial Disproprtionation of Elemental Sulfur. Science. 266, 1973–1975. doi:10.1126/science.11540246
Carlson, R. W., Anderson, M. S., Johnson, R. E., Schulman, M. B., and Yavrouian, A. H. (2002). Sulfuric Acid Production on Europa: the Radio- Lysis of Sulfur in Water Ice. Icarus. 157, 456–463. doi:10.1006/icar.2002.6858
Carlson, R. W., Johnson, R. E., and Anderson, M. S. (1999). Sulfuric Acid on Europa and the Radiolytic Sulfur Cycle. Science. 286, 97–99. doi:10.1126/science.286.5437.97
Chela-Flores, J. (2010). Instrumentation for the Search of Habitable Ecosystems in the Future Exploration of Europa and Ganymede. Int. J. Astrobiology. 9, 101–108. Available at:doi:10.1017/s1473550410000029
Chela-Flores, J. (2017). Instrumentation for Testing whether the Icy Moons of the Gas and Ice Giants are Inhabited. Forum Article. Astrobiology J. 17, 958–961. Available at: http://www.ictp.it/∼chelaf/ABJ_2017_3.pdf. doi:10.1089/ast.2016.1621
Chela-Flores, J. (2018). Testing S Isotopes as Biomarkers for Mars. Int. J. Astrobiology. 18, 436–439. Available at:doi:10.1017/s1473550418000393
Chela-Flores, J. (2006). The sulphur Dilemma: are There Biosignatures on Europa’s Icy and Patchy Surface? Int. J. Astrobiol. 5, 17–22. Available at:doi:10.1017/s1473550406002862
Chela-Flores, J., Cicuttin, A., Crespo, M. L., and Tuniz, C. (2015). Biogeochemical Fingerprints of Life: Earlier Analogies with Polar Ecosystems Suggest Feasible Instrumentation for Probing the Galilean Moons. Int. J. Astrobiol. 14, 427–434. Available at: http://www.ictp.it/∼chelaf/ss333. doi:10.1017/s1473550414000391
Chela-Flores, J., and Seckbach, J. (2011). “The Dry Valley Lakes, Antarctica: from Sulfur Stains on Earth to Sulfur Stains in the Jovian System. Instruments, Methods, and Missions for Astrobiology XIV,” in Proceedings of the SPIE. Editors R B. Hoover, Pl. C. W. Davies, G. V. Levin, and A. Y. Rozanov. 8152, 81520R–81520R-81528R. doi:10.1117/12.898763
Christophe, B., Spilker, L. J., Anderson, J. D., Andre, N., Asmar, S. W., Aurnou, J., et al. (2012). OSS (Outer Solar System): a Fundamental and Planetary Physics Mission to Neptune, Triton and the Kuiper Belt. Exp. Astron. 34, 203–242. doi:10.1007/s10686-012-9309-y
Clifford, S. M., and Parker, T. J. (2001). The Evolution of the Martian Hydrosphere: Implications for the Fate of a Primordial Ocean and the Current State of the Northern plains. Icarus. 154, 40–79. doi:10.1006/icar.2001.6671
Davis, W. L., and McKay, C. P. (1996). Origins of Life: A Comparison of Theories and Application to Mars. Origins Life Evol. Biosph. 26, 61–73. doi:10.1007/bf01808160
Donahue, T. M., Hoffman, J. H., Hodges, R. R., and Watson, A. J. (1982). Venus Was Wet: A Measurement of the Ratio of Deuterium to Hydrogen. Science. 216, 630–633. doi:10.1126/science.216.4546.630
Donahue, T. M., and Russell, C. T. (1997). “The Venus Atmospher e and Ionosphere and their Interaction With the Solar Wind: An Overview,” in Venus II Geology, Geophysics, Atmosphere, and Solar Wind Environment. Editors S. W. Baugher, D. M. Hunten, and R. J. Phillips (Tucson: Univ. of Ariz. Press), 3–31.
Doran, P. T., Berry Lyons, W., and McKnight, D. M. (2010). Life in Antarctic Deserts and Other Cold Dry Environments. Cambridge, United Kingdom: Cambridge University Press.
Eigenbrode, J., Gold, R. E., McKay, C. P., Hurford, T., and Davila, A. (2018). Searching for Life in an Ocean World: The Enceladus Life Signatures and Habitability (ELSAH) mission Concept. 42nd COSPAR Scientific Assembly. 14-22 July. California, USA: PasadenaAbstract id. F3.6-3-18.
Fagents, S. A. (2003). Considerations for the Effusive Cryovolcanism on. Europa: the post-Galileo Perspective. J. Geophys. Res. 108 (E12), 5139. doi:10.1029/2003je002128
Fairén, A. G., Dohm, J. M., Baker, V. R., de Pablo, A., Ruiz, J., Ferris, J. C., et al. (2003). Episodic Flood Inundations of the Northern plains of Mars. Icarus. 165, 53–67. doi:10.1016/s0019-1035(03)00144-1
Fanale, F. P., Granahan, J. C., McCord, T. B., Hansen, G., Hibbitts, C. A., Carlson, R., et al. (1999). Galileo’s Multiinstrument Spectral View of Europa’s Surface Composition. Icarus. 139, 179–188. doi:10.1006/icar.1999.6117
Föhn, M., Tulej, M., Galli, A., Vorburger, A. H., Lasi, D., Wurz, P., et al. (2021). Description of the Mass Spectrometer for the Jupiter Icy Moons Explorer Mission. IEEE. 2021, 14. doi:10.1109/AERO50100.2021.9438344
Gaeman, J., Hier-Majumdera, S., and Roberts, J. H. (2012). Sustainability of a Subsurface Ocean within Triton’s Interior. Icarus. 220, 339–347. doi:10.1016/j.icarus.2012.05.006
Gibson, E. K. (1982). A Review of Distributions of Sulfur in Solar System Objects. Abstracts of Papers Presented to the Conference on Planetary Volatiles, Held in Alexandria, Minnesota, October 9-12, 1982. Sponsored by the Lunar and Planetary Institute, NASA, and the National Science Foundation. Houston, TX 77058: LPI Contribution 488, published by the Lunar and Planetary Institute, 3303 Nasa Road 1, 41.
Gowen, R. A., Smith, A., Fortes, A. D., Barber, S., Brown, P., Church, P., et al. (2011). Penetrators for In Situ Sub-surface Investigations of Europa. Adv. Space Res. 48, 725–742. Available at: http://www.ictp.it/∼chelaf/ss333. doi:10.1016/j.asr.2010.06.026
Grasset, O., Bunce, E. J., Coustenis, A., Dougherty, M. K., Erd, C., Hussmann, H., et al. (2013a). Review of Exchange Processes on Ganymede in View of its Planetary Protection Categorization. Astrobiology. 13, 991–1004. doi:10.1089/ast.2013.1013
Grasset, O., Dougherty, M. K., Coustenis, A., Bunce, E. J., Erd, C., Titov, D., et al. (2013b). JUpiter ICy Moons Explorer (JUICE): An ESA Mission to Orbit Ganymede and to Characterise the Jupiter System. Planet. Space Sci. 78, 1–21. doi:10.1016/j.pss.2012.12.002
Grinspoon, D. H., and Bullock, M. A. (2003). Did Venus Experi Ence One Great Transition or Two? Bull. Am. Astron. Soc. 35, 44–03.
Grinspoon, D. H., and Bullock, M. A. (2007). Astrobiology and Venus Exploration. Geophys. Monogr. Am. Geophys. Union. 176, 191. doi:10.1029/176gm12
Grundy, W. M., Buratti, B. J., Cheng, A. F., Emery, J. P., Lunsford, A., McKinnon, W. B., et al. (2007). New Horizons Mapping of Europa and Ganymede. Science 318, 234–236. doi:10.1126/science.1147623
Hendrix, A. R., Hurford, T. A., Barge, L. M., Bland, M. T., Bowman, J. S., Brinckerhoff, W., et al. (2018). The NASA Roadmap to Ocean Worlds. Astrobiology. 19, 1–27. doi:10.1089/ast.2018.1955
Hofstadter, M., Simon, A., Atreya, S., Banfield, D., Fortney, J., Hayes, A., et al. (2017). A Vision for Ice Giant Exploration. Planetary Science Vision 2050 Workshop, 27–28 February and 1 March, 2017. Washington, DC: LPI Contribution No. 1989, id.8115.
Horvath, J., Carsey, F., Cutts, J., Jones, J., Johnson, E., Landry, B., et al. (1997). “Searching for Ice and Ocean Biogenic Activity on Europa and Earth. Instruments, Methods and Missions for Investigation of Extraterrestrial Microorganisms,” in Proc. SPIE 3111. Editor R.B. Hoover, 490–500. Available at: http://www.ictp.it/∼chelaf/searching_for_ice.html.
Howell, S. M., and Pappalardo, R. T. (2020). NASA’s Europa Clipper: a mission to a Potentially Habitable Ocean World. Nat. Commun. 11, 1311. doi:10.1038/s41467-020-15160-9
Hussmann, H., Sohl, F., and Spohn, T. (2006). Subsurface Oceans and Deep Inter- Iors of Medium-Sized Outer Planet Satellites and Large Trans-neptunian Objects. Icarus. 185, 258–273. doi:10.1016/j.icarus.2006.06.005
Ireland, T. R. (2013). Recent Developments in Isotope-Ratio Mass Spectrometry for Geochemistry and Cosmochemistry. Rev. Scientific Instr. 84, 011101–011121. doi:10.1063/1.4765055
Kaplan, I. R. (1975). Stable Isotopes as a Guide to Biogeochemical Processes. Proc. R. Soc. Lond. Ser. B. 189, 183–211. doi:10.1098/rspb.1975.0052
Kasting, J. F., Pollack, J. B., and Ackerman, T. P. (1984). Response of Earth’s Atmosphere to Increases in Solar Flux and Implications for Loss of Water From Venus. Icarus. 57, 335–343. doi:10.1016/0019-1035(84)90122-2
Kasting, J. F. (1988). Runaway and Moist Greenhouse Atmospheres and the Evolution of Earth and Venus. Icarus. 74, 472–494. doi:10.1016/0019-1035(88)90116-9
Kohn, M. J., Riciputi, L. R., Stakes, D., and Orange, D. L. (1998). Sulfur Isotope Variability in Biogenic Pyrite: Reflections of Heterogeneous Bacterial Colonization? Am. Mineral. 83, 1454–1468. doi:10.2138/am-1997-11-1234
Konstantinidis, K., Flores Martinez, C. L., Dachwald, B., Ohndorf, A., Dykta, P., Bowitz, P., et al. (2015). A lander mission to Probe Subglacial Water on Saturn׳s Moon Enceladus for Life. Acta Astronautica. 106, 63–89. doi:10.1016/j.actaastro.2014.09.012
Kring, D. A., Whitehouse, M. J., and Schmieder, M. (2021). Microbial Sulfur Isotope Fractionation in the Chicxulub Hydrothermal System. Astrobiology. 21, 103. doi:10.1089/ast.2020.2286
Lasi, D., Meyer, S., Piazza, D., Lüthi, M., Nentwig, A., Gruber, M., et al. (2020). Decisions and Trade-Offs in the Design of a Mass Spectrometer for Jupiter's Icy Moons. IEEE Aerospace Conf. doi:10.1109/aero47225.2020.9172784
Ligterink, N. F. W., Grimaudo, V., Moreno-García, P., Lukmanov, R., Tulej, M., Leya, I., et al. (2020). ORIGIN: a Novel and Compact Laser Desorption – Mass Spectrometry System for Sensitive In Situ Detection of Amino Acids on Extraterrestrial Surfaces. Scientific Rep. 10, 9641. doi:10.1038/s41598-020-66240-1
Mansell, J., Kolencherry, N., Hughes, K., Arora, A., Chye, H. S., Coleman, K., et al. (2017). Oceanus: A Multi-Spacecraft Flagship mission Concept to Explore Saturn and Uranus. Adv. Space Res. 59, 2407–2433. doi:10.1016/j.asr.2017.02.012
McCord, T. B., Hansen, G. B., Clark, R. N., Martin, P. D., Hibbitts, C. A., Fanale, F. P., et al. (1998). Non-Water-ice Constituents in the Surface Material of the Icy Galilean Satellites From the Galileo Near-Infrared Mapping Spectrometer Investigation. J. Geophys. Res. 103 (E4), 8603–8626. doi:10.1029/98je00788
McEwen, A. S. (1986). Exogenic and Endogenic Albedo and Color Patterns on Europa. J. Geophys. Res. 91, 8077–8097. doi:10.1029/jb091ib08p08077
McKay, C. P. (2008). An Approach to Searching for Life on Mars, Europa, and Enceladus. Space Sci. Rev. 135, 49–54. doi:10.1007/s11214-007-9229-8
McKay, C. P., Chela-Flores, J., Owen, T. y., and Raulin, F. (2001). “The Search for a Second Genesis in the Solar System,” in (2001). The First Steps of Life in the Universe (Dordrecht, Netherlands: Kluwer Academic Publishers), 269–277. doi:10.1007/978-94-010-1017-7_47
McLennan, S. M., and Grotzinge, J. P. (2009). “Sulfur and the Sulfur Cycle on Mars,” in 40th Lunar and Planetary Science Conference, 2152.
Meyer, S., Tulej, M., and Wurz, P. (2017). Mass Spectrometry of Planetary Exospheres at High Relative Velocity:direct Comparison of Open- and Closed-Source Measurements. Geosci. Instrum. Method. Data Syst. 6, 1–8. doi:10.5194/gi-6-1-2017
Miller, S. L. (1953). A Production of Amino Acids under Possible Primitive Earth Conditions. Science. 117, 528–529. doi:10.1126/science.117.3046.528
Mitri, G., Postberg, F., Soderblom, J. M., Wurz, P., Tortora, P., Abel, B., et al. (2018). Explorer of Enceladus and Titan (E2 T): Investigating Ocean Worlds’ Evolution and Habitability in the Solar System. Planet. Space Sci. 155, 73–90. doi:10.1016/j.pss.2017.11.001
Multari, R. A., Cremers, D. A., Dupre, J. M., and Gustafson, J. E. (2010). The Use of Laser-Induced Breakdown Spectroscopy for Distinguishing between Bacterial Pathogen Species and Strains. Appl. Spectrosc. 64 (7), 750–759. doi:10.1366/000370210791666183
Nimmo, F., and Spencer, J. (2014). Powering Triton's Recent Geological Activity by Obliquity Tides: Implications for Pluto Geology. Icarus. 246, 2–10. doi:10.1016/j.icarus.2014.01.044
Ohmoto, H. (1986). Stable Isotope Geochemistry of Ore Deposits. Œ. 16, 491–559. doi:10.1515/9781501508936-019
Owen, T., Mahaffy, P., Niemann, H. B., Atreya, S. K., Donahue, T. M., Bar-Nun, A., et al. (1999). A New Constraint on the Formation of Giant Planets. Nature. 402, 269–270. doi:10.1038/46232
Pappalardo, R. T., Vance, S., Bagenal, F., Bills, B. G., Blaney, D. L., Blankenship, D. D., et al. (2013). Science Potential from a Europa Lander. Astrobiology. 13, 740–773. doi:10.1089/ast.2013.1003
Phillips, C. B., and Chyba, C. F. (2001). “Europa: Prospects for an Ocen and Life,” in The First Steps of Life in the Universe. Editors J. Chela-Flores, T. Owen, and F. Raulin (Dordrecht, Netherlands: Kluwer Academic Publishers), 25–34.
Pitesky, J. K. P., and Hand, C. B. (2020). The Europa Lander Mission Concept: Situ Exploration of an Ocean World. San Francisco: AGU Fall Meeting 2020, 0511–P101.
Popa, R., Kinkle, B. K., and Badescu, A. (2004). Pyrite Fram- Boids as Biomarkers for Iron-Sulfide Systems. Geomicrobiol J. 21, 193–206. doi:10.1080/01490450490275497
Prockter, L. M., and Schenk, P. (2005). Origin and Evolution of Castalia Macula, an Anomalous Young Depression on Europa. Icarus. 177, 305–326. doi:10.1016/j.icarus.2005.08.003
Reh, K., Spilker, L., Lunine, J. I., Waite, J. H., Cable, M. L., Postberg, F., et al. (2016). “Enceladus Life Finder: the Search for Life in a Habitable Moon,” in 2016 IEEE Aerospace Conference(IEEE), 1–8. doi:10.1109/aero.2016.7500813
Rhoden, A. R., Henning, W., Hurford, T. A., and Hamilton, D. P. (2015). The interior and Orbital Evolution of Charon as Preserved in its Geologic Record. Icarus. 246, 11–20. doi:10.1016/j.icarus.2014.04.030
Riedo, A., Bieler, A., Neuland, M., Tulej, M., and Wurz, P. (2013a). Performance Evaluation of a Miniature Laser Ablation Time-Of-Flight Mass Spectrometer Designed for In Situ Investigations in Planetary Space Research. J. Mass. Spectrom. 48, 1–15. doi:10.1002/jms.315710.1002/jms.3104
Riedo, A., Neuland, M., Meyer, S., Tulej, M., and Wurz, P. (2013b). Coupling of LMS with Fs-Laser Ablation Ion Source: Elemental and Isotope Composition Measurements. J. Anal. Atom. Spectrom. 28, 1256–1269. doi:10.1039/c3ja50117e
Riedo, A., Meyer, S., Heredia, B., Neuland, M., Bieler, A., Tulej, M., et al. (2012). Highly Accurate Isotope Composition Measurements by a Miniature Laser Ablation Mass Spectrometer Designed for In Situ Investigations on Planetary Surfaces. Planet. Space Sci. 87, 1–13. doi:10.1002/jms.3104
Sandor, B. J., Clancy, R. T., Moriarty-Schieven, G., and Mills, F. P. (2010). Sulfur Chemistry in the Venus Mesosphere from SO2 and SO Microwave Spectra. Icarus. 208, 49–60. doi:10.1016/j.icarus.2010.02.013
Schulze-Makuch, D., and Irwin, L. N. (2004). Reassessing the Possibility of Life on Venus: Proposal for an Astrobiology Mission. Astrobiology. 2, 197. doi:10.1089/15311070260192264
Shen, Y., Buick, R., and Canfield, D. E. (2001). Isotopic Evidence for Microbial Sulphate Reduction in the Early Archaean Era. Nature. 410, 77–81. doi:10.1038/35065071
Shen, Y., and Buick, R. (2004). The Antiquity of Microbial Sulfate Reduction. Earth-Science Rev. 64, 243–272. doi:10.1016/s0012-8252(03)00054-0
Sim, M. S., Bosak, T., and Ono, S. (2011). Large Sulfur Isotope Fractionation Does Not Require Disproportionation. Science. 333, 74–77. doi:10.1126/science.1205103
Smith, A., Crawford, I. A., Gowen, R. A., Ball, A. J., Barber, S. J., Church, P., et al. (2009). LunarEX-A Proposal to Cosmic Vision. Exp. Astron. 23, 711–740. doi:10.1007/s10686-008-9109-6
Tulej, M., Iakovleva, M., Leya, I., and Wurz, P. (2011). A Miniature Mass Analyser for In-Situ Elemental Analysis of Planetary Material–Performance Studies. Anal. Bioanal. Chem. 399, 2185–2200. doi:10.1007/s00216-010-4411-3
Tulej, M., Neubeck, A., Ivarsson, M., Riedo, A., Neuland, M. B., Meyer, S., et al. (2015). Chemical Composition of Micrometer-Sized Filaments in an Aragonite Host by a Miniature Laser Ablation/ionization Mass Spectrometer. Astrobiology. 15, 1–14. doi:10.1089/ast.2015.1304
Tulej, M., Meyer, S., Lüthi, M., Lasi, D., Galli, A., Piazza, D., et al. (2016). Experimental Investigation of the Radiation Shielding Efficiency of a MCP Detector in the Radiation Environment Near Jupiter’s Moon Europa: Nuclear Instruments and Methods. Phys. Res. Section B. 383, 21–37. doi:10.1016/j.nimb.2016.06.008
Turrini, D., Politi, R., Peron, R., Grassi, D., Plainaki, C., Bar- bieri, M., et al. (2014). The Comparative Exploration of the Ice Giant Planets With Twin Spacecraft: Unveiling the History of Our Solar System. Planet. Space Sci. 104, 93–107. doi:10.1016/j.pss.2014.09.005
Wächtershäuser, G. (1990). Evolution of the First Metabolic Cycles. PNAS. 87, 200–204. doi:10.1073/pnas.87.1.200
Wand, U., Schwarz, G., Bruggemann, E., and Brauer, K. (1997). Evidence for Physical and Chemical Stratification in Lake Untersee (central Dronning Maud Land, East Antarctica). Antarctic Sci. 9 (1), 4345. doi:10.1017/s0954102097000060
Way, M. J., Del Genio, A. D., Kiang, N. Y., Sohl, L. E., Grinspoon, D. H., Aleinov, I., et al. (2016). Was Venus the First Habitable World of Our Solar System? Geophys. Res. Lett. 43, 8376–8383. doi:10.1002/2016GL069790
Weider, S. Z., Nittler, L. R., Murchie, S. L., Peplowski, P. N., McCoy, T. J., Kerber, L., et al. (2016). Evidence From MESSENGER for Sulfur‐ and Carbon‐Driven Explosive Volcanism on Mercury. Geophys. Res. Lett. 43, 3653–3661. doi:10.1002/2016gl068325
Weiss, P., Yung, K. L., Ng, T. C., Komle, N., Kargl, G., and Kaufmann, E. (2008). Study of a Melting Drill Head for the Exploration of Subsurface Planetary Ice Layers. Planet. Space Sci. 56, 1280–1292. doi:10.1016/j.pss.2008.04.004
Wiesendanger, R., Tulej, M., Riedo, A., Frey, S., Shea, H., Wurz, P., et al. (2017). Improved Detection Sensitivity for Heavy Trace Elements Using a Miniature Laser Ablation Ionisation Mass Spectrometer. J. Anal. Atom Spectrom. 32 (11), 2182–2188. doi:10.1039/c7ja00193b
Wiesendanger, R., Tulej, M., Grimaudo, V., Cedeño-López, A., Lukmanov, R., Riedo, A., et al. (2018a). A Method for Improvement of Mass Resolution and Isotope Accuracy for Laser Ablation Time‐of‐flight Mass Spectrometers. J. Chemometrics - Wiley. 33, e3081. doi:10.1002/cem.3081
Wiesendanger, R., Wacey, D., Tulej, M., Neubeck, A., Ivarsson, M., Grimaudo, V., et al. (2018b). Chemical and Optical Identification of Micrometer-Sized 1.9 Billion-Year-Old Fossils by Combining a Miniature Laser Ablation Ionization Mass Spectrometry System with an Optical Microscope. Astrobiology. 18, 1071–1080. doi:10.1089/ast.2017.1780
Wurz, P., Abplanalp, D., Tulej, M., and Lammer, H. (2012). A Neutral Gas Mass Spectrometer for the Investigation of Lunar Volatiles. Planet. Space Sci. 74, 264–269. doi:10.1016/j.pss.2012.05.016
Wurz, P., Lasi, D., Thomas, N., Piazza, D., Galli, A., Jutzi, M., et al. (2017). An Impacting Descent Probe for Europa and the Other Galilean Moons of Jupiter. Physics, Geology. Earth, Moon, Planets. 120, 113. doi:10.1007/s11038-017-9508-7
Keywords: habitability, Ocean worlds, miniaturisation of mass spectrometers, stable isotope geochemistry, sulphur geochemistry, JUpiter ICy moons Explorer mission, Europa Clipper, terrestrial analogies
Citation: Chela-Flores J (2021) Miniaturised Instrumentation for the Detection of Biosignatures in Ocean Worlds of the Solar System. Front. Space Technol. 2:703809. doi: 10.3389/frspt.2021.703809
Received: 30 April 2021; Accepted: 12 July 2021;
Published: 27 July 2021.
Edited by:
Andreas Riedo, University of Bern, SwitzerlandReviewed by:
Khaled. Y Kamal, Texas A&M University, United StatesFrancesco Romanò, Arts et Metiers ParisTech, France
Copyright © 2021 Chela-Flores. This is an open-access article distributed under the terms of the Creative Commons Attribution License (CC BY). The use, distribution or reproduction in other forums is permitted, provided the original author(s) and the copyright owner(s) are credited and that the original publication in this journal is cited, in accordance with accepted academic practice. No use, distribution or reproduction is permitted which does not comply with these terms.
*Correspondence: Julian Chela-Flores, Y2hlbGFmQGljdHAuaXQ=