- 1Department of Sustainable Soils and Crops, Rothamsted Research, Harpenden, United Kingdom
- 2Department of Forestry and Environmental Management, Mzuzu University, Mzuzu, Malawi
- 3Environment and Natural Resources Management Department, Lilongwe University of Agriculture and Natural Resources, Lilongwe, Malawi
- 4Animal Science Department, Lilongwe University of Agriculture and Natural Resources, Lilongwe, Malawi
- 5School of Biology, University of Leeds, Leeds, United Kingdom
- 6School of Earth and Environment, University of Leeds, Leeds, United Kingdom
Conservation agriculture (CA) is widely promoted as an agroecology-based approach for soil conservation. Several studies have focused on effects of CA on crop yields and soil moisture dynamics in sub-Saharan Africa, with limited focus on effects of CA on soil organic carbon (SOC) and associated fractions. We collected representative soil samples from 30 paired farms under CA and conventional tillage in Mzimba district, north of Malawi to determine effects of tillage and soil depth on soil physico-chemical properties, total SOC, and organic carbon fractions. Undisturbed soil cores were collected for bulk density measurements. Different SOC pools were determined using the soil fractionation method, while soil physico-chemical analyses were conducted using standard laboratory methods on disturbed soil samples. Soil organic carbon content ranged from 0.4-1.8% in CA plots. This was significantly larger than SOC contents of 0.4-1.5% measured under conventional tilled plots. Tillage type and soil depth had significant interaction effects on SOC. For example, larger contents of SOC were measured at depths of 0-10 cm compared to 10-30 cm under CA plots. Soil depth had significant effects on most soil properties compared to tillage. Examples include Heavy Particulate Organic Matter-Carbon (POM-C) fraction, Mineral Associated Organic Matter-Carbon (MAOM-C), nitrogen in MAOM fraction and nitrogen in the Light POM fractions. These were larger in the 0-10 cm soil depth than in the 10-30 cm soil depth. In contrast, tillage type only had significant effects on the Heavy POM-C and MAOM-C fractions, which were larger under CA than conventional tilled plots. Conservation agriculture showed capacity to improve total SOC and its associated fractions, a finding relevant towards understanding effects of land management on carbon storage. However, challenges of competing residue use as feed, mulch, and fuel continue to impede mulching under CA systems. Longer term studies and use of alternative mulching options could be employed to recognise noticeable changes in other SOC beneficial pools in fields under CA.
Highlights
● Soil depth significantly influenced most of the soil properties unlike tillage.
● Tillage type and soil depth had a significant interactive effect on SOC.
● Reduced tillage had significant effects on selected carbon pools.
● MAOM was significantly larger within the 10-30 cm depth than 0-10 cm soil depth.
● Tillage type and soil depth significantly affected C in the heavy POM and MAOM fractions.
1 Introduction
In sub-Saharan Africa (SSA), agriculture extends beyond its economic function to become a vital lifeline for communities and a cornerstone of regional stability (1). Predominantly managed by smallholder farmers, this crucial industry is confronted with escalating challenges such as soil degradation, climate change shocks and use of unimproved technologies (2–4). As traditional farming methods prove increasingly ineffective and the adoption of modern technologies remains sluggish, the resilience of this agricultural lifeline in the region is at risk (5).
Malawi, a sub-Saharan African country, relies on agriculture as the backbone of the economy, providing livelihoods for most of its population. Agriculture contributes 30% of Malawi’s Gross Domestic Product and generates over 80% of national export earnings (6). It occupies approximately 56% of the country’s land area, encompassing 5.3 million hectares out of a total of 9.4 million hectares. The agricultural sector also meets a minimum of 65% of the manufacturing sector’s raw material demands and remains the primary employer for 64% of the country’s workforce (7–9). Despite its importance, 90% of cultivated land is managed under a customary tenure system by smallholder farmers, with only 10% allocated to estate-commercial farming (10). The smallholder farmer tillage system, characterised by the annual construction of planting ridges, commonly known as conventional tillage practices, has firmly entrenched itself as a fundamental aspect of subsistence farming (11).
This conventional tillage system contributes significantly to soil degradation through extensive land clearing (i.e. removal and/or burning of plant debris, weeds, and crop residues) (12). Furthermore, in Malawi, most smallholder farmers adhere to traditional cultivation methods with minimal adoption of modern inputs and conservation technologies for soil and water management. It is estimated that ~12% of cultivated land incorporates contour ridges, crucial for mitigating soil erosion and conserving water resources, posing tremendous setbacks to agriculture productivity and environmental degradation (13).
The detrimental effects of land ploughing are manifold, leading to soil compaction, diminished water infiltration, reduced soil aeration, and depletion of organic matter content (11). These consequences not only compromise soil properties but also escalate farming costs (14). Furthermore, studies have shown that ploughing contributes to the formation of hardpan layers, decreasing hydraulic conductivity (15). The loss of soil organic matter due to conventional tillage not only diminishes soil fertility (16), but also renders crops increasingly vulnerable to inter-seasonal droughts, leaving agricultural systems ill-equipped to withstand the disruptive impacts of climate change (17). Amidst escalating soil degradation and the ever-pressing global challenges of climate change, the call to adopt sustainable agricultural practices in Malawi has become an urgent and pivotal imperative. Conservation agriculture (CA) is an agro ecology based agricultural technology for efficient use of resources, integrated natural resources use (soil water, and biological resources), combined with external inputs (18–21). It is centred around three main principles of minimum soil disturbance, at least 30% ground cover with crop residues, or live mulch and, diversified crop sequences and associations involving at least three different crops in rotations and/or in intercropping systems. Vanlauwe et al. (22) proposed appropriate use of fertilizers as a 4th principle of CA, especially in low nutrient input systems of sub-Saharan Africa. No-tillage practices reduce mechanical soil disturbance (23), permanent soil cover shields against erosion (14), and crop diversification fosters soil biodiversity and nutrient balance while mitigating pest and disease pressure (24). This farming practice aims to enhance soil fertility, biodiversity, and ecosystem resilience while promoting sustainable crop production (25, 26).
While CA is based on these main principles, it is often practised differently in different fields, farms, and regions. Conservation agriculture has emerged as a sustainable agricultural practice offering a viable solution to current food security and environmental challenges emerging from agricultural practices. Conservation agriculture stands as a pivotal approach in sustainable agricultural development, particularly in these regions where soil degradation threatens food security and livelihoods (27). Its adoption has been widely promoted to mitigate soil erosion, enhance soil fertility, and improve water retention, ultimately boosting agricultural productivity while conserving natural resources (14, 25, 28) and offering resilience to climate change (17).
In recent years, research on CA in Southern Africa has predominantly focused on the agronomic and yield impacts of this management practice (27, 29). In addition, several studies also looked at effects of CA on different soil physico-chemical properties including water infiltration, soil moisture and total soil organic carbon (SOC) (14, 16, 27, 30), with fewer studies focusing on CA’s effects on different SOC pools and stocks. Soil organic carbon stocks give an indication of SOC quantities within a given area enabling us to understand effects of tillage (i.e. soil compaction) on carbon quantities.
A broader focus on effects of different tillage practices on soil organic matter (SOM) fractions could additionally provide a more accurate prediction of soil vulnerability to management, disturbance, and climate change to better inform land management for C storage. This is mainly because the particulate organic matter (POM) fraction is relatively short lived and likely to be more vulnerable to environmental changes than the mineral associated organic matter (MAOM) fraction, which has a larger residence time in the soil and has a lower vulnerability to disturbance (31). Understanding these aspects, especially under smallholder farmer management, is critical for evaluating the long-term sustainability and resilience of CA systems. To address this knowledge gap, we conducted a study in the Mzimba district of Malawi, to assess the influence of tillage practices on soil properties, SOC content and stocks, and organic matter dynamics. The main objective of the study was to assess the impact of different tillage practices, specifically CA versus conventional tillage, on soil physico-chemical properties and organic carbon fractions. We hypothesised soils under CA would exhibit higher levels of SOC content and stocks including in different associated fractions in the soil, and enhanced soil physico-chemical properties compared to soils under conventional tillage due to reduced soil disturbance and enhanced carbon retention.
2 Methods
The study was conducted in Zombwe (11° 19' 0" South, 33° 50' 0" East) and Manyamula (11° 94' 13" South, 33° 44' 40" East) Extension Planning Areas (EPA) in Mzimba district, northern Malawi. Soils in this district are medium to light textured and moderately fertile. Zombwe EPA is underlain by gneiss of basement complex characterised by very deep well drained soils. The soils are brown and red with a fine and medium texture. Manyamula EPA lies in the southern plains of Mzimba district and is characterised by medium to fine textured, red soils, that are deep and well drained. The soils are medium over fine textured. A gneiss of the basement complex and old alluvial deposits underlay these gently undulating plains. The upper 50 cm layer of the soils in both EPAs is usually acidic to moderately acidic (pH 5.0-6.0) with a rather low total nitrogen (0.50-0.12%) and low nutrient retention capacity (5-10 me/100 g) (32).
Annual rainfall ranges from 650 to 1300 mm (33). This normally falls between November and March, with most rains received in December and January. Malawi has a wide range of mean temperatures. Mzimba district has a warm tropical climate, with temperatures typically varying between 10°C and 29°C over the course of the year. Average monthly maximum temperatures range from 27 to 33°C with October and early November being the warmest months. The average monthly minimum temperatures range from 0 to 10°C. The winter season, starting in May experiences the lowest temperature, with the months of June and July being the coldest (34).
Smallholder farmers in Malawi commonly burn crop residues from the previous year’s crops or feed them to livestock (16). The Government of Malawi and its implementing partners have been promoting CA as one of its strategies to redress land degradation, increase climate change resilience and improve crop productivity. However, there are still fewer farmers practising CA in Mzimba district compared to other districts in Malawi (35). Conservation agriculture implementing farmers in this district practice reduced tillage and crop rotation including with grain legumes such as cowpea (Vigna unguiculata [L.] Walp) and the staple maize (Zea mays L.) cereal crop. Mulching is often done with crop residues from the previous season. Maize grain yields in Malawi are generally low, yielding less than 1.3 t ha-1 against a potential of 6 – 8 t ha-1 (36). Therefore, farmers are encouraged to practise CA as a strategy to improve soil structure, water holding capacity and subsequently crop yields (16). On the other hand, the conventional tillage system consists of annual ridge construction before planting, for soil inversion. While some farmers implement crop rotation under this tillage system, none of these would implement mulching through crop residue retention.
2.1 Soil sampling
Soil samples were randomly collected in November 2019 from 30 farms with fields under both CA and conventional tillage (Figure 1), before planting of fields for the new cropping season. Field sites had variable durations for which CA and conventional tillage had been implemented, ranging from two to six years for CA. Specifically, ~18% of farmers practised CA for two years, with a similar percentage practising CA for four, and five years, respectively. The majority (36%) of farmers practised CA for three consecutive years, while only 9% of the farmers practised CA for six consecutive years. Earlier studies by Bhattacharyya et al. (37) and Mondal et al. (38) classified four to five years of CA implementation as a short-term period. Additionally, a lack of grain yield improvements within 5-10 years of adoption (26) has resulted in these years being classified as a transition period (39). It is within this background context that we categorised all these farmers as short-term CA adopters.
Soil samples were randomly collected from 0-10 and 10-30 cm depths from CA and conventional tilled paired plots. Paired plots were located next to each other, within the same topography and soil type. The approximate distance between the fields was less than 500 meters. Where no conventional treatment was available on the farm, fields were sampled from neighbouring farms, observing the described homogeneity criteria. Three replicates of undisturbed soil samples were randomly collected from respective tillage management practices and soil depths using a soil core ring. These soil samples were collected for gravimetric soil moisture content and bulk density measurements using the coring method. Similarly, five soil sub-samples were collected from respective tillage management practices and soil depths using an auger. These sub-samples were homogenised and composited into one sample per field for determination of various soil physico-chemical properties and different pools of soil organic matter fractions. In total, 30 paired plots were sampled, and comprised of 60 fields (30 CA and 30 conventional tilled plots), amounting to 480 soil samples, considering the described sampling design.
2.2 Sample preparation and laboratory analyses
The soil samples were placed in pre-labelled bags and air-dried at the Lilongwe University of Agriculture and Natural Resources (LUANAR) in Malawi. Soil physico-chemical properties (bulk density, available phosphorus, C:N ratio, total carbon, total nitrogen, soil texture) were analysed using standard laboratory procedures at LUANAR. Soil texture was analysed using the hydrometer method (40). Soil moisture content was determined using the gravimetric method. Subsequently, the air-dried samples were analysed for available phosphorus, SOC, and soil pH. Available phosphorus was determined using the Mehlich-3 method, while NH4+ and NO3- were determined using the colorimetric technique. Soil pH was analysed using potentiometric methods in water (1:2.5). Soil organic carbon was analysed using the modified Walkley-Black method (41). A sub-sample was collected from each soil sample and sent to the University of Leeds Soil Laboratory for SOM fractionation.
Soil samples for SOM fractionation were sieved to <2 mm before analysis. Soil particles with a diameter of less than 2 mm were shook in deionised water. The solution was then centrifuged, and decanted. The remaining solid part was subjected to dispersion and density floatation in sodium polytungstate (SPT) and beads, and then shook for 18 hours. After this step, the part with particles smaller than 1.85g cm-3 was defined as light particulate organic matter (Light POM). After wet sieving, the remaining part was divided into heavy particulate organic matter (Heavy POM) and mineral-associated organic matter (MAOM) according to whether the particle diameter was greater than 53 μm. A summary of the fractionation procedure for determination of different SOM pools is presented in Figure 2.
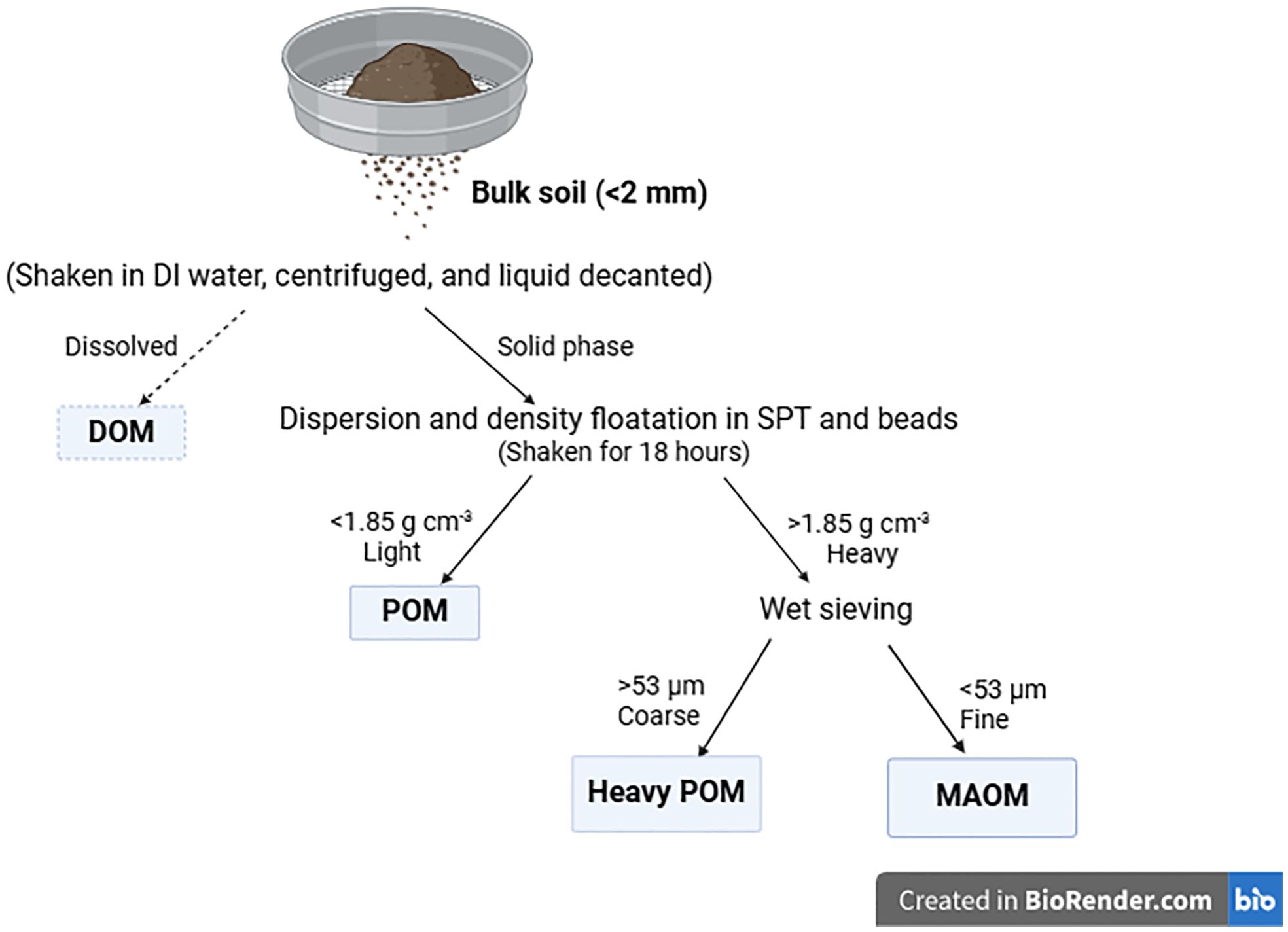
Figure 2. Soil Organic Matter Fractionation procedure implemented in this study. Dashed arrow and box indicate that the Dissolved Organic Matter (DOM) fraction was not analysed in this study. Light POM, Light Particulate Organic Matter; Heavy POM, Heavy Particulate Organic Matter; MAOM, Mineral Associated Organic Matter; DI, Deionised water; SPT, Sodium polytungstate. Created in https://BioRender.com.
2.3 Data analysis
Data were tested for normality before being subjected to a two-way Analysis of Variance (ANOVA) using GenStat 23rd edition (42). Tillage type and soil depth were classed as treatment factors and soil properties including pH, Total N, Total P, texture, total SOC, Light POM-C, Heavy POM-C and MAOM-C as response variables. Tillage and soil depth interaction effects on defined soil properties were also analysed using GenStat. The honestly significant difference (HSD) test, also referred to as the Tukey’s test, was used to separate tillage type and soil depth treatment means at P = 0.05. We calculated SOC stocks in different tillage systems and across the two soil sampling depths by multiplying SOC content (%), bulk density (g cm-3) and depth (cm) within which a soil sample was collected (i.e. 10 cm for the 0-10 cm sampling depth and 20 cm for the 10-30 cm sampling depth). SOC stocks were then presented in t ha-1. Soil physico-chemical data is presented as Tables. Graphs are presented using SigmaPlot version 15 to denote differences between treatment factors in selected soil properties.
3 Results
3.1 Soil physico-chemical properties under contrasting tillage practises and soil depth
Fields were clustered into the sandy loam (N=29), loamy sand (N=24), and sandy clay loam textural classes (N=14). Soil depth had more significant effects on soil properties than tillage type. For example, soil depth had significant effects on available P, Heavy POM-C, MAOM-C, C:N ratio, Heavy POM, MAOM, N-MAOM, N-Light, SOC, pH, total C and total N. In contrast, tillage type had significant effects on available P, Heavy POM-C, MAOM-C, SOC, pH, total N, gravimetric soil moisture content and bulk density. Soil organic carbon was the only soil property where a significant interaction effect between tillage type and depth was reported. Supplementary Figure S1 shows rainfall distribution across two sites. Rainfall data were for two stations at Mzimba Boma and the other at Mzuzu, further north of Mzimba. Rainfall distribution is presented to show effects on data from Manyamula EPA close to Mzimba Boma weather station and Zombwe EPA close to Mzuzu weather station. Cumulative rainfall of 851-, 808- and 693-mm year-1 was received in Mzimba while cumulative rainfall of 839, 906 and 845 mm year-1 was received in Mzuzu during the 2018, 2019 and 2020 rainfall seasons, respectively (Supplementary Figure S1).
3.2 Effect of tillage type, soil depth and their interaction on bulk density
Bulk density ranged from 1.21 – 1.85 g cm-3. Tillage had significant effects on bulk density (P<0.001) (Figure 3). Conventional tillage had the largest soil bulk density measurements of 1.51 g cm-3 (range 1.25 – 1.80) while CA plots had bulk density measurements of 1.45 g cm-3 (range 1.21 – 1.71). Similar mean BD values of 1.51 g cm-3 were measured under conventional plots at the 0-10 cm and 10-30 cm soil depths. These were significantly larger than values of 1.43 (0-10 cm) and 1.47 g cm-3 (10-30 cm) measured under CA plots. Overall, soil depth had no significant effects on bulk density (P=0.060). Similarly, there were no interaction effects of tillage type and soil depth on bulk density (P=0.065).
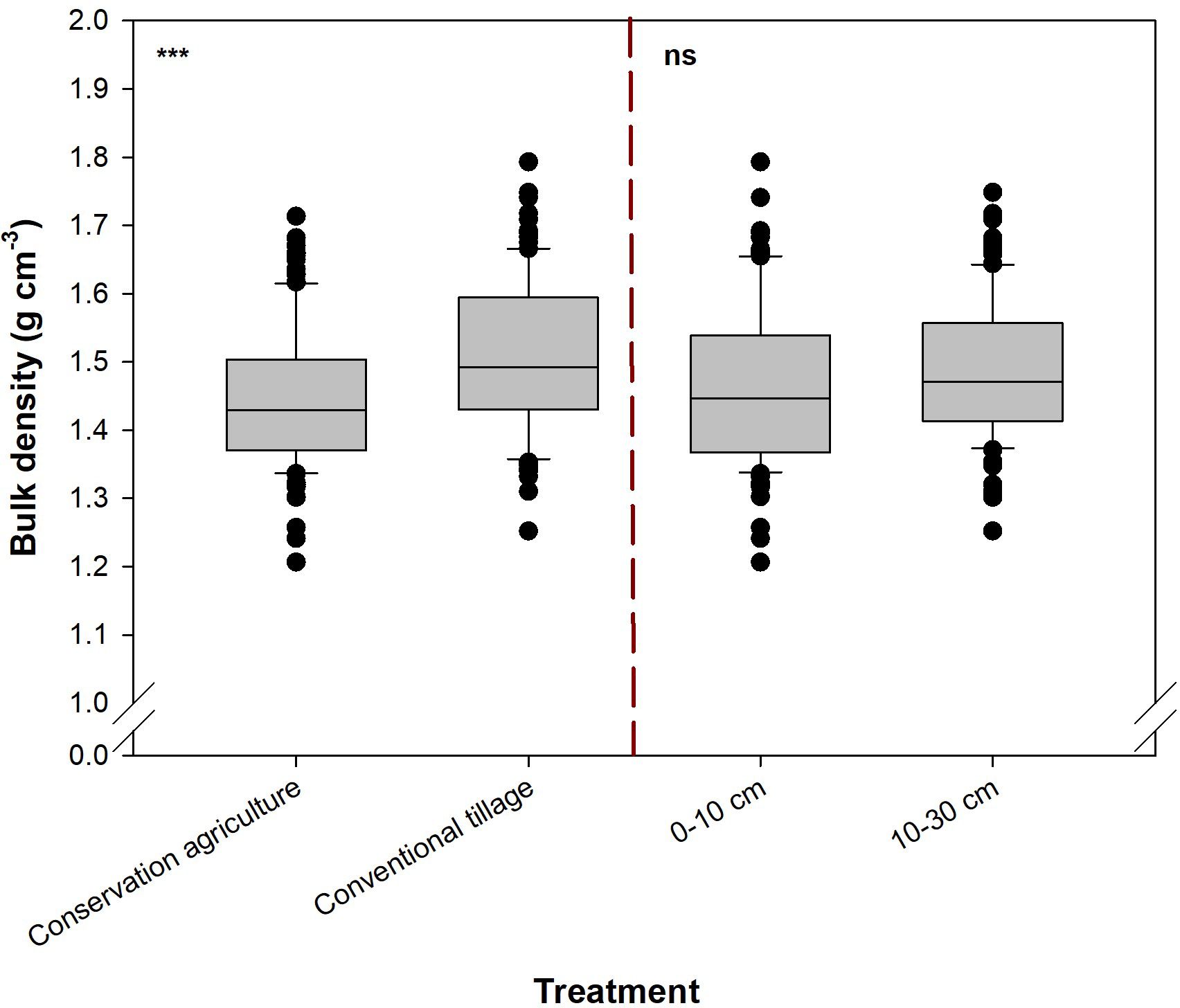
Figure 3. Bulk density measured in farmers’ fields under different tillage and soil depths in Mzimba, Malawi. *** indicate significant treatment effects. ns indicate no significant treatment effects.
3.3 Effect of tillage type, soil depth and their interaction on gravimetric soil moisture content
Gravimetric soil moisture content ranged from 1.8 – 20.2%. One field reported a gravimetric soil moisture content of 82.0%. This was regarded to as an outlier using the Inter Quartile Range (IQR) method in R which calculates the upper and lower bounds for outliers, and therefore excluded from the analysis. Tillage type had significant effects on gravimetric soil moisture content (P=0.034) (Figure 4). Conservation agriculture had the largest gravimetric soil moisture content of 8.9% (range 3.5 – 20.2%), while conventional tilled plots had gravimetric soil moisture content of 7.9% (range 1.8 – 14.2%). Overall, soil depth had no significant effects on gravimetric soil moisture content (P=0.197) and there was no interaction effect between tillage type and soil depth on gravimetric soil moisture content (P=0.146). Conservation agriculture had gravimetric soil moisture content of 9.2% and 8.5% within the 0-10 cm and 10-30 cm soil depths, respectively, and conventional tillage had comparable gravimetric soil moisture content of 7.9% and 8.0% within the 0-10 cm and 10-30 cm soil depths, respectively.
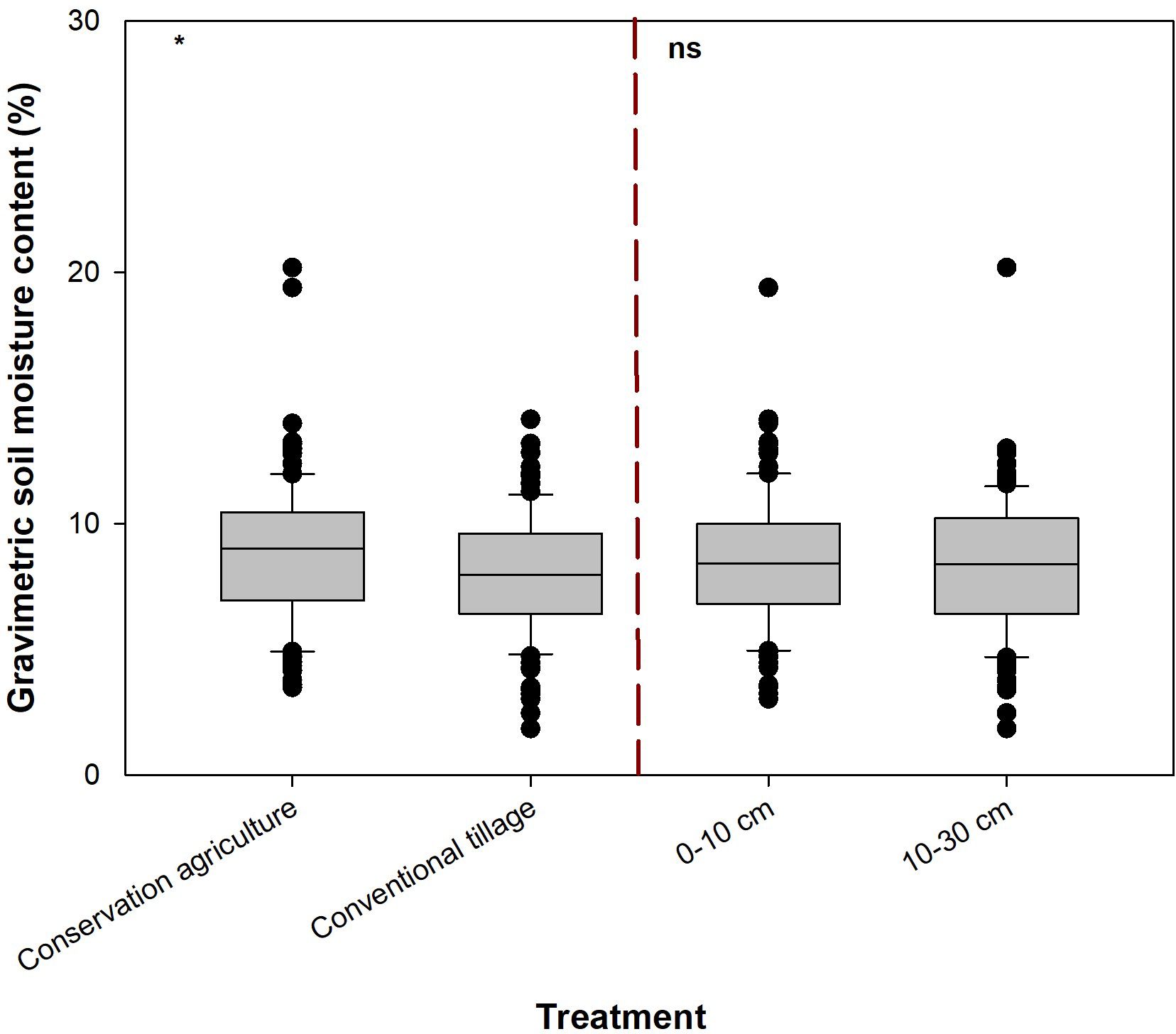
Figure 4. Gravimetric soil moisture content measured in farmers’ fields under different tillage and soil depths in Mzimba, Malawi. * indicate significant treatment effects. ns indicate no significant treatment effects.
3.4 Effect of tillage type, soil depth and their interaction on SOC content and stocks in different fractions
Soil organic carbon content ranged from 0.4 – 1.8%. Tillage type had significant effects on SOC content (P<0.001) (Figure 5). Conservation agriculture had the largest SOC content of 1.0% (range 0.4 – 1.8%), while conventional tilled plots had a SOC content of 0.8% (range 0.4 – 1.5%). Similarly, soil depth had significant effects on SOC (P<0.001). The largest SOC content, 1.0% (range 0.5 – 1.8%), was reported at soil depths of 0-10 cm, irrespective of tillage management. This were 25% larger than the 0.8% (range 0.4 – 1.2%) SOC content measured at soil depths of 10-30 cm. There was a significant (P=0.025) interaction effect between tillage type and soil depth on SOC content, the only variable for which there was a significant interaction between these factors. In this regard, CA plots had a mean SOC content of 1.1% at soil depths of 0-10 cm. This was 22% larger than mean contents (0.9%) measured at 10-30 cm depths under the same tillage management option. Similarly, conventional tillage plots had a mean SOC content of 0.8% at 0-10 cm soil depth. This was 14% larger than mean SOC contents of 0.7% measured at 10-30 cm depths under the same tillage management option.
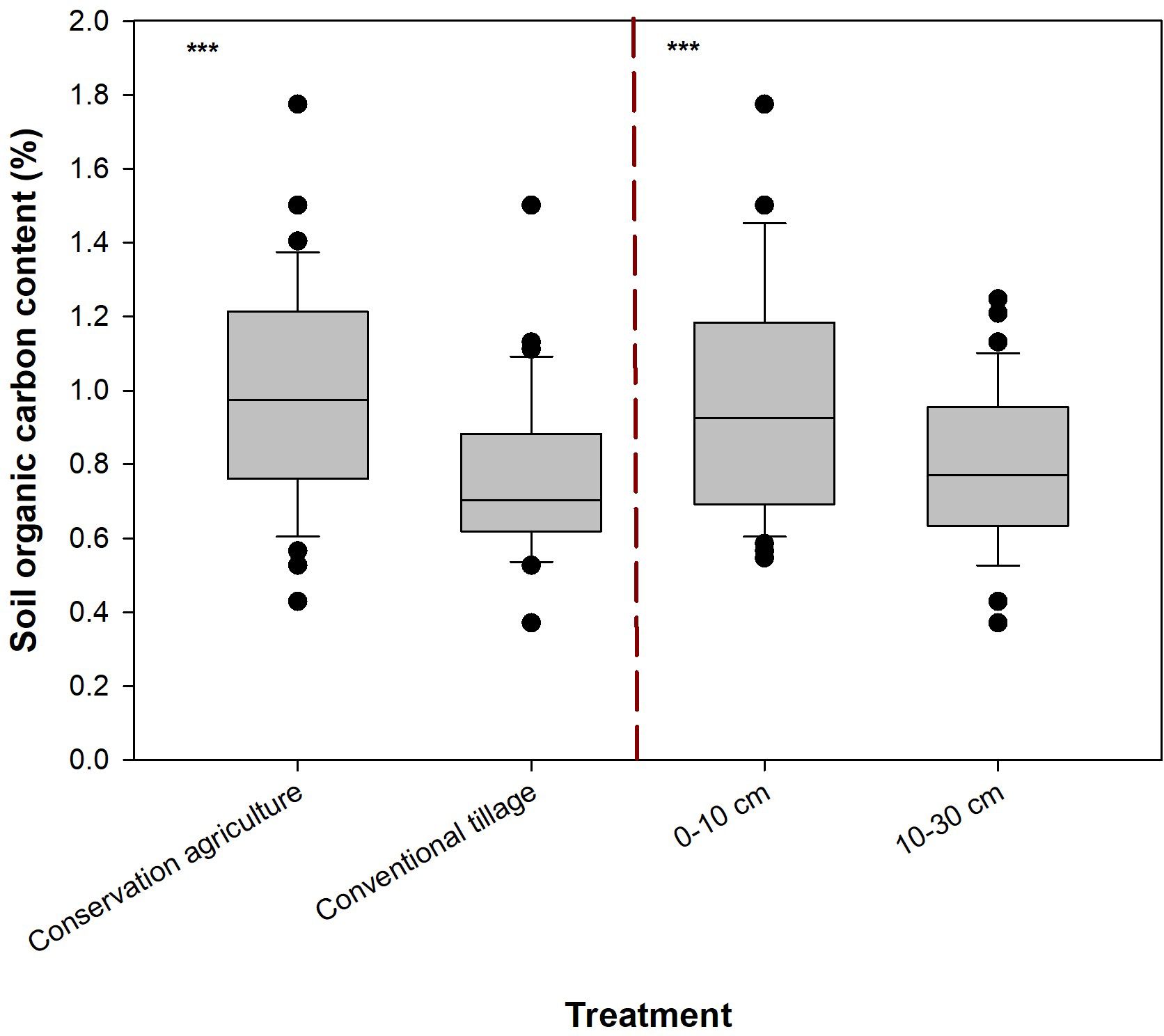
Figure 5. Soil organic carbon content measured in farmers’ fields under different tillage and soil depths in Mzimba, Malawi. *** indicate significant treatment effects.
Soil organic carbon stocks ranged from 7.6 to 37.3 t ha-1 (Supplementary Table S1). Tillage type had significant (P <0.05) effects on SOC stocks, with the largest SOC stocks averaging 20.4 t ha-1 reported in CA plots (Table 1). In contrast, conventional tilled plots had SOC stocks of 17.1 t ha-1. Within the 0-10 cm soil sampling depth, CA plots had significantly (P<0.001) larger SOC stocks of 15.9 t ha-1 compared to SOC stocks of 12.1 t C ha-1 in the conventional tilled plots (Table 1). While CA plots had slightly larger SOC stocks of 24.9 t C ha-1 within the 10-30 cm soil sampling depth, this was not significantly different (P>0.05) from SOC stocks of 22.0 t C ha-1 measured in conventional tilled plots (Table 1). Tillage type had significant effects on the following SOC fractions: Heavy POM-C (P=0.026) and MAOM-C (P=0.023) (Table 2). Larger contents of the C in the Heavy POM fraction of 0.13% was reported in CA than conventional tillage plots, which measured contents of 0.11%. Similarly, larger contents of the C-MAOM fraction of 2.14% was measured under CA plots than contents of 1.96% measured under conventional tilled plots. Soil depth had significant effects on the Heavy POM-C (P<0.001), MAOM-C (P<0.001), Heavy POM (P<0.001), MAOM (P<0.001), N-MAOM (P<0.001) and N-Light (P=0.013) fractions (Figure 6). A similar trend of larger contents of SOC pools at soil depths of 0-10 cm was reported for most fractions apart from the MAOM fraction which had contents of 19.0% at soil depths of 10-30 cm compared to 14.0% at the 0-10 cm soil depth. Specifically, the Heavy POM-C, MAOM-C, Heavy POM, N-MAOM and N-Light fractions had contents of 0.15, 2.34, 83.0, 0.20 and 0.97% respectively, at soil depth of 0-10 cm (Table 3). In contrast, contents of 0.10, 1.76, 77.0, 1.56 and 0.68% were measured in the Heavy POM-C, MAOM-C, Heavy POM, N-MAOM and N-Light fractions respectively, at soil depths of 10-30 cm (Table 3).
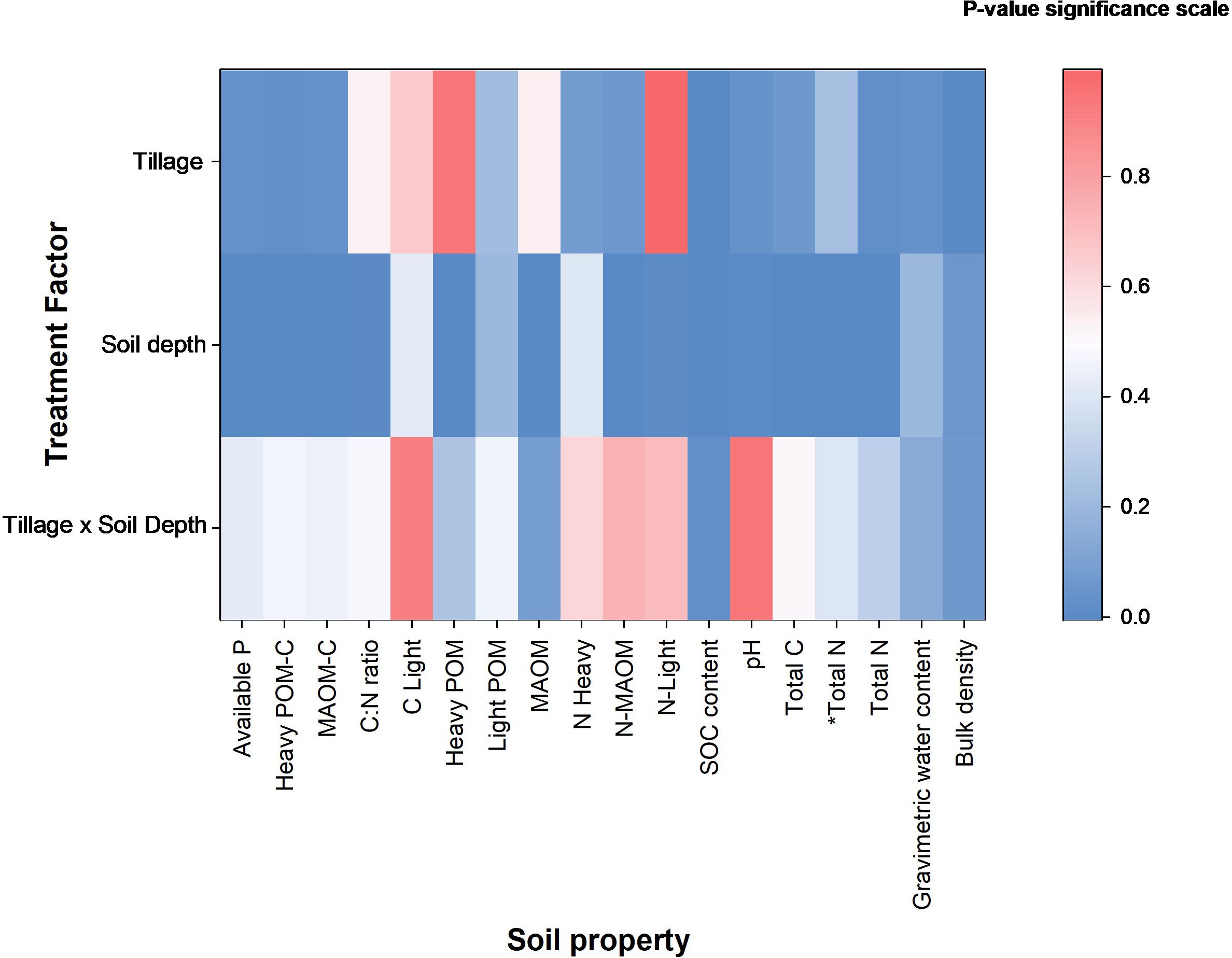
Figure 6. Heat map showing effects of tillage and soil depth on different soil properties. Available P, Available Phosphorus; Heavy POM-C, Carbon in the Heavy Particulate Organic Matter fraction; MAOM-C, Carbon in the Mineral Associated Organic Matter fraction; C:N, Carbon to Nitrogen ration; C-Light, Carbon in the Light Particulate Organic Matter fraction; Heavy POM, Heavy Particulate Organic Matter; Light POM, Light Particulate Organic Matter; MAOM, Mineral Associated Organic Matter; N-Heavy, Nitrogen in the Heavy Particulate Organic Matter fraction; N-MAOM; Nitrogen in the Mineral Associated Organic Matter fraction; N-Light, Nitrogen in the Light Particulate Organic Matter fraction; SOC content, Soil Organic Carbon content; Total C, Total Carbon measured using the fractionation method; *Total N, Total Nitrogen measured using the fractionation method; Total N, Total Nitrogen measured using the colorimetric method.
3.5 Effect of tillage type, soil depth and their interaction on other soil properties
Tillage type and soil depth had significant effects on available P, pH, and total N. In addition to these parameters, total C and C:N ratio were the only additional parameters significantly affected by soil depth. Available P was significantly larger in CA (35.6 mg kg-1) than conventional tillage (28.1 mg kg-1) plots. Similarly, available P was significantly larger in 0-10 cm (38.9 mg kg-1) than 10-30 cm (24.9 mg kg-1) soil depths. Soil pH was significantly larger (P=0.038) in CA (5.0) than conventional tillage (4.8) plots. Similarly, soil pH was significantly larger in 0-10 cm (5.0) than 10-30 cm (4.8) soil depths (P=0.009) (Table 3). There was a general trend of significantly larger total C and C:N ratio in 0-10 than 10-30 cm soil depths. For example, a larger mean C:N ratio of 11.9 was measured within the 0-10 cm soil depth than a mean ratio of 11.1 measured within the 10-30 cm depth.
4 Discussion
4.1 Contribution of conservation agriculture to soil properties in farmers’ fields
We assessed effects of different tillage practices and residue management on soil properties across two soil depths in smallholder farmers’ fields. These fields were under variable timescales of CA and conventional tillage implementation. Conservation agricultural practices significantly affected most tested soil physical properties (i.e. BD and gravimetric soil moisture content), in addition to soil chemical properties. Consistently lower BD was measured in CA plots compared to conventional tilled plots. The lower BD under CA plots and a consistently larger soil moisture content are both indications of lower compaction, improved soil pore spaces and water holding capacity, all indicators of improved soil structure. In contrast, soil depth significantly influenced both soil physical and chemical properties, with larger values attained within the 0-10 cm soil depth compared to the 10-30 cm soil depth. Such an effect of soil depth on diverse soil physical and chemical properties could be attributed to differences in SOC content between the two layers, which could be the driving factor for all the other soil properties. Ligowe et al. (16) reported an increase in SOM, soil aggregate stability and earthworm population in a CA trial after 5-years of CA implementation compared to the conventional control trial. Soil organic matter content exerts a strong effect on most soil properties and functions (43) by regulating hydrological functions, soil structure (44, 45) and below ground microbial communities (46). Strategies which improve SOC accumulation in soil are likely to result in a general improvement in all other plant essential soil properties and thus should be promoted in low-nutrient input systems.
Crop residue management was significantly different between the CA and conventional tilled systems assessed in this study. The annual carbon input from maize stover, averaged over the CA sites for the evaluated harvest year was 1.15 t C ha-1, all of which was left on the field as mulch. In contrast, most of the maize stover was removed from the fields in the conventional tilled system, with only 0.07 t C ha-1 remaining on the soil surface. This difference in the total amount of carbon inputs from aboveground biomass help explain the increase in SOC stocks in the CA systems in this study, as observed in other work (16, 27, 47, 48).
Two of CA’s main principles of mulching/residue retention and crop diversification play a critical role in SOC management. The only drawback of employing these principles in smallholder settings are competing needs for crop residues as feed and fuel (49) and a generally low biomass yield from the staple grain legumes (i.e. ground nut, common bean, and cowpea) (50, 51). Alternative mulching strategies such as use of often overlooked recalcitrant straw material (46), which are quantitatively important components of SOM (52); and production of green manure cover crops could be promoted. This could be done in conjunction with the National Agriculture Extension Services through curriculum reform and/or training to ensure pro-active crop residue management for SOM build-up (15, 53, 54).
Soil organic carbon was the only parameter where significant interaction effects of tillage type and soil depth were observed. Significantly larger SOC contents were measured under CA plots within a soil depth of 0-10 cm. For example, 1.1% SOC content was measured under CA within a 0-10 cm soil depth compared to 0.8% SOC content within conventional tilled plots under the same soil depth. This translates to ~38% larger SOC content under CA than conventional tilled plots. Increasing SOC stocks could offset carbon dioxide emissions. Sperow (55) reported that no till increases SOC stocks and reduced carbon dioxide emissions in the US, at a lower cost than other alternatives such as geological storage whose costs are high due to fuel costs and the technological advancements required. While carbon credits are increasingly being recognised in European farming systems (56), most recognised projects in sub-Saharan Africa are outside the scope of CA and SOC sequestration (57).
4.2 Effects of conservation agriculture on different SOC pools and stocks
We assessed effects of CA on SOC content and its different pools. Most studies on CA focused on total SOC and/or SOM without specific focus on CA’s effect on the different SOC pools. Our study reported significant effects of CA on Heavy POM-C, MAOM-C, Total C and Total N. In addition to Total C and Total N, soil depth had significant effects on Heavy POM, MAOM, N-MAOM, and N-Light. Larger contents were measured within the 0-10 cm soil depth for most of these fractions except the MAOM content which was larger in the 10-30 cm soil depth. Most CA ridging or drilling is done to the 10 cm soil depth for seed and fertilizer placement. In this regard, CA has potential to significantly influence different pools of SOM in different soil depths in a positive way. Most soil functions are driven by readily available forms of SOM, hence the focus of most studies (46, 58–60). The MAOM fraction has a relatively lower C:N ratio compared to the POM fraction making it more nutrient-dense and assimilable by plants and microbes (61). This characteristic of MAOM makes it a key fraction in SOM storage and turnover, thus contributing to global C cycling (62–65).
Soil organic carbon stocks were significantly larger in CA plots compared to conventional tilled plots, irrespective of soil depth. This could be attributed to minimum soil disturbance which reduces soil erosion and protects carbon from oxidative losses, residue retention through mulching and incorporation of legumes which improves soil fertility and biomass production (66, 67). Our study also indicated significantly larger SOC stocks of 15.9 t ha-1 in the 0-10 cm soil depth than stocks of 12.1 t C ha-1 in conventional tilled plots, within the same soil sampling depth of 0-10 cm. The difference in SOC stocks between the two systems could be attributed not only to mulch retention in CA systems, but also to a more effective role of root than shoot addition to C accumulation as reported earlier in a similar no-till tropical Ferralsol (68). However, SOC stocks within the 10-30 cm soil sampling depth in CA and conventional tilled plots were comparable and not statistically significant possibly due to limited residue incorporation into the soil in both cropping systems. While reduced soil disturbance in CA systems promotes deep burrowing earthworms which subsequently contribute to organic matter build-up (69), alternative strategies which ensure organic matter incorporation into deeper soil depths could be promoted to improve SOC stocks. This includes root decomposition from production of deep-rooted crops such as cassava and sweet potatoes and deep seed placement during dry planting of maize; a strategy often practised to cope with climate uncertainty in Africa (70).
4.3 Implication of tillage and organic management on ancillary crop nutrition benefits
Findings from this study showed larger contents of SOC under CA compared to conventional tilled plots. This could be attributed to mulching and crop diversifications, through rotations and intercropping practised by farmers as part of CA’s three main principles. Recent evidence showed that organic inputs increase grain micronutrient concentration (i.e. zinc, iron and vitamin A) in maize, rice, wheat and tomatoes (71). In this review on contribution of regenerative agriculture to crop nutrition, organic inputs included animal and green manures, cover crops, crop rotations, crop residues, composts and biochars, some of which are used by smallholder farmers in their CA plots. Similarly, reduced tillage showed some evidence (33-66% of relevant papers) of increased grain zinc concentration in wheat. While CA is mainly promoted for efficient use of resources and integrated use of natural resources (i.e. soil, water and biological resources), this recent scoping review showed the potential of CA to yield ancillary benefits of improved crop nutrition, with a subsequent contribution to human health in sub-Saharan Africa. Several other studies reported improved crop nutrition benefits under CA systems. For example, Chilimba et al. (72) reported improved grain selenium concentration in maize grown under a CA cropping system in Malawi, albeit with selenium fertilizer application. However, annual application of selenium fertilizer was recommended under CA managed fields due to selenium fixation into humus bound forms (73). This beneficial contribution of CA to food nutrition systems suggest that crop nutritional quality should also be included as a primary outcome from implementation of CA under smallholder farming systems.
5 Conclusions
Conservation agriculture has potential to improve soil properties under low nutrient input systems. Conservation agriculture increased SOC contents among other soil properties, with different SOC contents measured in different soil depths. Our study is among the few to explicitly focus on effects of CA on different pools of SOC in Malawi, with larger contents of carbon reported in the Heavy POM-C and MAOM-C fractions. Larger values of soil properties reported within the 0-10 cm soil depth compared to the 10-30 cm soil depth could be attributed to organic matter retention, including root decomposition. Cultivation of deeper-rooted crops under CA systems could potentially ensure enhancement of SOC and other soil properties within the 10-30 cm soil depths. While CA showed positive benefits on a diverse suite of soil properties, its adoption under smallholder farming systems is still largely impeded by competing needs for crop residues as feed, fuel and mulch, and the rather labour-intensive nature of the practise due to limited financial resources to purchase machinery. Use of alternative mulching materials such as straw, production of high biomass yielding cover crops and Government support with input subsidies could ensure wider adoption of CA by smallholder farmers and broader recognition of its impacts on soil conservation and carbon sequestration. Future studies should be supported to focus on medium- to-longer term effects of CA on often overlooked but potentially beneficial pools of SOC.
Data availability statement
The raw data supporting the conclusions of this article will be made available by the authors, without undue reservation.
Author contributions
MGM-K: Data curation, Formal analysis, Visualization, Writing – original draft, Writing – review & editing. ISL: Writing – original draft, Writing – review & editing. AT: Data curation, Investigation, Project administration, Writing – review & editing. TNG: Data curation, Investigation, Project administration, Writing – review & editing. HMRG: Supervision, Writing – review & editing. MVG: Conceptualization, Funding acquisition, Methodology, Writing – review & editing.
Funding
The author(s) declare financial support was received for the research, authorship, and/or publication of this article. This work was supported by the Biotechnology and Biological Sciences Research Council (BBSRC) through UK Research and Innovation as part of the Global Challenges Research Fund, AFRICAP programme, grant number BB/P027784/1. Rothamsted Research receives strategic funding from BBSRC; Rothamsted contributors acknowledge support from the Growing Health (BB/X010953/1) Institute Strategic Programme.
Acknowledgments
Authors acknowledge farmers involved in this study for their participation. The authors also wish to thank Professor Francesca Cotrufo from Colorado State University for their support with the soil organic matter fractionation methodology.
Conflict of interest
The authors declare that the research was conducted in the absence of any commercial or financial relationships that could be construed as a potential conflict of interest.
Publisher’s note
All claims expressed in this article are solely those of the authors and do not necessarily represent those of their affiliated organizations, or those of the publisher, the editors and the reviewers. Any product that may be evaluated in this article, or claim that may be made by its manufacturer, is not guaranteed or endorsed by the publisher.
Supplementary material
The Supplementary Material for this article can be found online at: https://www.frontiersin.org/articles/10.3389/fsoil.2024.1481275/full#supplementary-material
Supplementary Figure 1 | Cumulative rainfall received in sites next to Mzimba and Mzuzu weather stations for 2018, 2019 and 2020 rainfall season.
Supplementary Table 1 | SOC stocks (t ha-1) by site, management system and soil depth.
Abbreviations
Available P, Available phosphorus; CA, Conservation Agriculture; Heavy POM-C, Carbon in the Heavy Particulate Organic Matter fraction; MAOM-C, Carbon in the Mineral Associated Organic Matter fraction; C:N ratio, Carbon: Nitrogen ratio; Heavy POM, Heavy Particulate Organic Matter; MAOM, Mineral Associated Organic Matter; N-MAOM, Nitrogen in the Mineral Associated Organic Matter fraction; N-Light, Nitrogen in the Light Particulate Organic Matter fraction; SOC, Soil Organic Carbon; Total N, Total Nitrogen; Total C, Total Carbon; Light POM, Light Particulate Organic Matter fraction; N-Heavy, Nitrogen in the Heavy Particulate Organic Matter fraction.
References
1. World Bank. Agriculture and food. World Bank (2021). Available at: https://www.worldbank.org/en/topic/agriculture/overview (Accessed May 21, 2024).
2. FAO. The State of Food and Agriculture 2022: Moving forward on food security and nutrition. Food and Agriculture Organisation (2022). Available at: https://www.fao.org/publications/sofa/2022/en/ (Accessed May 21, 2024).
3. Masso C, Nziguheba G, Mutegi J, Galy-Lacaux C, Wendt J, Butterbach-Bahl K, et al. Soil fertility management in sub-Saharan Africa. In: Lichtfouse E, editor. Sustainable agriculture reviews. Springer, Cham (2017). p. 205–31.
4. Thornton PK, van de Steeg J, Notenbaert A, Herrero M. The Impact of Climate Change on Livestock and Livestock Systems in Developing Countries. A review of what we know and what we need to know. Agric Systems. (2009) 101:113–27. doi: 10.1016/j.agsy.2009.05.002
5. Sasson A. Food security for Africa: an urgent global issue. Agric Food Secur. (2012) 1:2. doi: 10.1186/2048-7010-1-2
6. Government of Malawi. Malawi Growth and Development Strategy. From Poverty to Prosperity 2006 – 2011. (2006). Malawi: Ministry of Development Planning and Cooperation.
7. Government of Malawi. Malawi Growth and Development Strategy III (MGDS III) 2017–2022. (2016). Malawi: Ministry of Economic Planning and Development.
9. DAES. Department of agricultural Extension Services: Staff Rationalization Report – 2008. Malawi (2008). Malawi: Ministry of Agriculture.
10. Smale M. Maize is life; Malawi’s delayed green revolution. World Dev. (1995) 23:819–831. doi: 10.1016/0305-750X(95)00013-3
11. Mloza-Banda HR, Makwiza CN, Mloza-Banda ML. Soil properties after conversion to conservation agriculture from ridge tillage in Southern Malawi. J Arid Environments. (2016) 127:7–16. doi: 10.1016/j.jaridenv.2015.11.001
12. Chikowo R, Mapfumo P, Nyamugafata P, Giller KE, Nyamangara J. Maize productivity and mineral N dynamics following different soil fertility management practices on a depleted sandy soil in Zimbabwe. Afr J Agric Res. (2009) 4:230–9. doi: 10.1016/j.agee.2003.08.009
13. Zulu B, Snapp S. Malawi’s agricultural input subsidy programme and soil health: Opportunities for sustainable intensification. Geographical J. (2019) 185:315–28.
14. Thierfelder C, Wall PC. Effects of conservation agriculture techniques on infiltration and soil water content in Zambia and Zimbabwe. Soil Tillage Res. (2009) 105:217–27. doi: 10.1016/j.still.2009.07.007
15. Eze S, Dougill AJ, Banwart SA, Hermans T, Ligowe IS, Thierfelder C. Impacts of Conservation agriculture on soil structure and hydraulic properties of Malawian agricultural systems. Soil Tillage Res. (2020) 201:104639. doi: 10.1016/j.still.2020.104639
16. Ligowe IS, Nalivata PC, Njoloma J, Makumba W, Thierfelder C. Medium-term effects of conservation agriculture on soil quality. Afr J Agric Res. (2017) 12:2412–20. doi: 10.5897/AJAR2016.11092
17. Steward PR, Thierfelder C, Dougill AJ, Ligowe IS. Conservation agriculture enhances resistance of maize to climate stress in a Malawian medium-term trial. Agric Ecosyst Environment. (2019) 277:95–104. doi: 10.1016/j.agee.2018.07.009
18. FAO. Conservation agriculture: Case studies in Latin America and Africa Vol. 78. . Rome, Italy: Food and Agriculture Organisation Soils Bulletin Soils Bulletin (2002).
19. FAO. Conservation agriculture (2008). Italy: Food and Agriculture Organization of the United Nations. Rome. Available online at: http://www.fao.org/ag/ca/index.html (Accessed 10 June 2024).
20. Giller KE, Witter E, Corbeels M, Tittonell P. Conservation agriculture and smallholder farming in Africa: The heretics’ view. Field Crops Res. (2009) 114:23–34. doi: 10.1016/j.fcr.2009.06.017
21. Pittelkow CM, Liang X, Linquist BA, van Groenigen KJ, Lee J, Lundy ME, et al. Productivity limits and potentials of the principles of conservation agriculture. Nature. (2015) 517:365–8. doi: 10.1038/nature13809
22. Vanlauwe B, Wendt J, Giller KE, Corbeels M, Gerard B, Nolte C. [amp]]lsquo;A fourth principle is required to define conservation agriculture in Sub-Saharan Africa: the appropriate use of fertilizer to enhance crop productivity’. Field Crops Res. (2014) 155:10–3. doi: 10.1016/j.fcr.2013.10.002
23. Derpsch R, Friedrich T, Kassam A, Hongwen L. Current status of adoption of no-till farming in the world and some of its main benefits. Int J Agric Biol Eng. (2010) 3:1–25. doi: 10.3965/j.issn.1934-6344.2010.01.001-025
24. Pooniya V, Zhiipao RR, Biswakarma N, Jat SL, Kumar D, Parihar CM, et al. Long-term conservation agriculture and best nutrient management improves productivity and profitability coupled with soil properties of a maize–chickpea rotation. Sci Rep. (2021) 11:10386. doi: 10.1038/s41598-021-89737-9
25. Hobbs PR, Sayre K, Gupta R. The role of conservation agriculture in sustainable agriculture. Philos Trans R Soc B: Biol Sci. (2008) 363:543–55. doi: 10.1098/rstb.2007.2169
26. Rusinamhodzi L, Corbeels M, van Wijk MT. A meta-analysis of long-term effects of conservation agriculture on maize grain yield under rain-fed conditions. Agron Sustain Dev. (2011) 32:657–63. doi: 10.1007/s13593-011-0040-2
27. Thierfelder C, Wall PC. Effects of conservation agriculture on soil quality and productivity in contrasting agro-ecological environments of Zimbabwe. Soil Use Manage. (2012) 28:209–20. doi: 10.1111/j.1475-2743.2012.00406.x
28. Lal R. Soil erosion and the global carbon budget. Environ Int J. (2003) 29:437–50. doi: 10.1016/S0160-4120(02)00192-7
29. Mupangwa W, Twomlow S, Walker S. Reduced tillage, mulching and rotational effects on maize (Zea mays L.), cowpea (Vigna unguiculata (Walp) L.) and sorghum (Sorghum bicolor L. Moench) yields under semi-arid conditions. Field Crops Res. (2012) 132:139–48. doi: 10.1016/j.fcr.2012.02.020
30. Thierfelder C, Mhlanga B. Short-term yield gains or long-term sustainability? – A synthesis of Conservation Agriculture long-term experiments in Southern Africa. Agriculture Ecosyst Environ. (2022) 326:107812. doi: 10.1016/j.agee.2021.107812
31. Lugato E, Lavallee JM, Haddix ML, Panagos P, Cotrufo MF. Different climate sensitivity of particulate and mineral-associated soil organic matter. Nat Geosci. (2021) 14:295–300. doi: 10.1038/s41561-021-00744-x
32. Paris S. Land resources appraisal of Mzuzu Agricultural Development Division. Malawi Govenment. Ministry of Agriculture, Land Husbandry Branch: United Nations Development Programme: Food and Agriculture Organization of the United Nations (1991).
33. Mzimba District Planning Department. Mzimba District Socio-economic Profile. Mzimba: Mzimba District Assembly (2008).
34. Gama AC, Mapemba LD, Masikati P, Homann-Kee Tui S, Crespo O, Bandason E. Modeling Potential Impacts of Future Climate Change in Mzimba District, Malawi 2040-2070 An Integrated Biophysical and Economic Modelling Approach (2014). International Food Policy Research Institute (IFPRI) Working Paper 08 (Accessed 10 June 2024).
35. Mwambungu C. Factors that influence farmers’ decision to upscale conservation agriculture on their farmland: a case study of Mzimba district, northern Malawi. Iceland: United Nations University Land Restoration Training Program (2019). Available at: https://www.grocentre.is/static/gro/publication/722/document/mwambungu2019.pdf (Accessed April 18, 2024).
36. Ellis F, Kutengule M, Nyasulu A. Livelihoods and rural poverty reduction in Malawi. World Dev. (2003) 31:1495–510. doi: 10.1016/S0305-750X(03)00111-6
37. Bhattacharyya R, Das TK, Das S, Abir D, Patra AK, Agnihotri R, et al. Four years of conservation agriculture affects topsoil aggregate-associated 15nitrogen but not the 15nitrogen use efficiency by wheat in a semi-arid climate. Geoderma. (2019) 337:333–40. doi: 10.1016/j.geoderma.2018.09.036
38. Mondal S, Poonia SP, Mishra JS, Bhatt BP, Karnena KR, Saurabh K, et al. Short-term (5 years) impact of conservation agriculture on soil physical properties and organic carbon in a rice–wheat rotation in the Indo-Gangetic plains of Bihar. Eur J Soil Sci. (2020) 71:1076–89. doi: 10.1111/ejss.12879
39. Derpsch R, Franzluebbers AJ, Duiker SW, Reicosky DC, Koeller K, Friedrich T, et al. Why do we need to standardize no-tillage research? Soil Tillage Res. (2014) 137:16–22. doi: 10.1016/j.still.2013.10.002
40. Gee GW, Bauder JW. Particle size analysis. In: Klute A, editor. Methods of Soil analysis. Part 1, 2nd Edition. W1: ASA and SSSA, Madison (1986). p. 383–411.
41. Anderson JM, Ingram JSI eds. Tropical soil biology and fertility. A handbook of methods. 2nd ed. Wallingford, UK: CAB International (1993).
42. VSN International. Genstat for windows. 23rd Edition. Wallingford, UK: VSN International (2024). Available at: https://vsni.co.uk/software/genstat (Accessed December 16, 2024).
43. Hatten J, Liles G. A ‘healthy’ balance – The role of physical and chemical properties in maintaining forest soil function in a changing world. Chapter 15. In: Busse M, Giardina CP, Morris DM, Debbie S, editors. Page-Dumroese. Developments in Soil Science, vol. 36 (2019). p. 373–96. doi: 10.1016/B978-0-444-63998-1.00015-X
44. Pikul JL Jr., Zuzel JF. Soil crusting and water infiltration affected by long-term tillage and residue management. Soil Sci Soc America J. (1994) 58:1524–30. doi: 10.2136/sssaj1994.03615995005800050036x
45. Franzluebbers AJ. Water infiltration and soil structure related to organic matter and its stratification with depth. Soil Tillage Res. (2002) 66:197–205. doi: 10.1016/S0167-1987(02)00027-2
46. Hoffland E, Kuyper TW, Comans RNJ, Creamer RE. Eco-functionality of organic matter in soils. Plant Soil. (2020) 455:1–22. doi: 10.1007/s11104-020-04651-9
47. Page KL, Dang YP, Dalal RC. The ability of conservation agriculture to conserve soil organic carbon and the subsequent impact on soil physical, chemical, and biological properties and yield. Front Sustain Food Syst. (2020) 4:31. doi: 10.3389/fsufs.2020.00031
48. Shumba A, Chikowo R, Thierfelder C, Corbeels M, Six J, Cardinael R. Mulch application as the overarching factor explaining increase in soil organic carbon stocks under conservation agriculture in two 8-year-old experiments in Zimbabwe. SOIL. (2024) 10:151–65. doi: 10.5194/soil-10-151-2024
49. Antwi-Agyei P, Atta-Aidoo J, Guodaar L, Dougill A. Building climate resilience through crop residue utilization: Experiences of Ghanaian smallholder farmers. Clim Resilience Sustainability. (2023) 2:e255. doi: 10.1002/cli2.55
50. Mapfumo P. Comparative analysis of the current and potential role of legumes in Integrated Soil Fertility Management in southern Africa. In: Bationo A, Waswa B, Okeyo JM, Maina JK, Mokwunye U, editors. Fighting Poverty in Sub-Saharan Africa: The Multiple Roles of Legumes in Integrated Soil Fertility Management (2011). p. 175–200.
51. Nezomba H, Mtambanengwe F, Tittonell P, Mapfumo P. Point of no return? Rehabilitating degraded soils for increased crop productivity on smallholder farms in eastern Zimbabwe. Geoderma. (2015) 239-240:143–55. doi: 10.1016/j.geoderma.2014.10.006
52. Barré P, Quénéa K, Vidal A, Cécillon L, Christensen BT, Kätterer T, et al. Microbial and plant-derived compounds both contribute to persistent soil organic carbon in temperate soils. Biogeochemistry. (2018) 140:81–92. doi: 10.1007/s10533-018-0475-5
53. Muwaniki C, McGrath S, Manzeke-Kangara MG, Wedekind V, Chamboko T. Curriculum reform in agricultural vocational education and training in Zimbabwe: Implementation challenges and possibilities. J Vocational Adult Continuing Educ Training. (2022) 5:22. doi: 10.14426/jovacet.v5i1.248
54. Manzeke-Kangara MG, Muwaniki C, Siziba S, Chamboko T, Mtambanengwe F, Wedekind V. Evolution of Agricultural Extension in Zimbabwe: Emerging technologies, Training needs and Future Possibilities. South Afr J Agric Extension. (2024) 52:21–55. doi: 10.17159/2413-3221/2024/v52n2a14969
55. Sperow M. What might it cost to increase soil organic carbon using no-till on U.S. cropland? Carbon Balance Manage. (2020) 15:26. doi: 10.1186/s13021-020-00162-3
56. European Union. Sustainable carbon cycles - Carbon farming. Accompanying the Communication from the Commission to the European Parliament and the Council. Sustainable Carbon Cycles (2021). Brussels. Available online at: https://climate.ec.europa.eu/document/download/d3529f84-0f18-40ee-ab72-124ba786fb5a_en (Accessed 18 April 2024).
57. World Bank. Stories from the Field - A Look at World Bank Carbon Finance Projects in Africa (2024). The World Bank Group. Available online at: https://www.worldbank.org/en/topic/climatechange/publication/projects-reducing-emissions-earning-carbon-credits-africa (Accessed 18 April 2024).
58. Abrar MM, Xu M, Shah SAA, Aslam MW, Aziz T, Mustafa A, et al. Variations in the profile distribution and protection mechanisms of organic carbon under long-term fertilization in a Chinese Mollisol. Sci Total Environ. (2020) 723:138181. doi: 10.1016/j.scitotenv.2020.138181
59. Rocci KS, Lavallee JM, Stewart CE, Cotrufo MF. Soil organic carbon response to global environmental change depends on its distribution between mineral-associated and particulate organic matter: A meta-analysis. Sci Total Environment. (2021) 793:148569. doi: 10.1016/j.scitotenv.2021.148569
60. Li J, Shangguan Z, Deng L. Free particulate organic carbon plays critical roles in carbon accumulations during grassland succession since grazing exclusion. Soil Tillage Res. (2022) 220:105380. doi: 10.1016/j.still.2022.105380
61. Williams EK, Fogel ML, Berhe AA, Plante AF. Distinct bioenergetic signatures in particulate versus mineral-associated soil organic matter. Geoderma. (2018) 330:107–16. doi: 10.1016/j.geoderma.2018.05.024
62. Gao J, Jansen B, Cerli C, Helmus R, Mikutta R, Dultz S, et al. Organic matter coatings of soil minerals affect adsorptive interactions with phenolic and amino acids. Eur J Soil Sci. (2018) 69:613–24. doi: 10.1111/ejss.12562
63. Kleber M, Bourg IC, Coward EK, Hansel CM, Myneni SCB, Nunan N. Dynamic interactions at the mineral–organic matter interface. Nat Rev Earth Environment. (2021) 2:402–21. doi: 10.1038/s43017-021-00162-y
64. Heckman K, Hicks Pries CE, Lawrence CR, Rasmussen C, Crow SE, Hoyt AM, et al. Beyond bulk: Density fractions explain heterogeneity in global soil carbon abundance and persistence. Global Change Biol. (2022) 28:1178–96. doi: 10.1111/gcb.16023
65. Sokol NW, Whalen ED, Jilling A, Kallenbach C, Pett-Ridge J, Georgiou K. Global distribution, formation and fate of mineral-associated soil organic matter under a changing climate: A trait-based perspective. Funct Ecol. (2022) 36:1411–29. doi: 10.1111/1365-2435.14040
66. Six J, Conant RT, Paul EA, Paustian K. Stabilization mechanisms of soil organic matter: Implications for C-saturation of soils. Plant Soil. (2002) 241:155–76. doi: 10.1023/a:1016125726789
67. Bohoussou YN, Kou YH, Yu WB, Lin BJ, Virk AL, Zhao X, et al. Impacts of the components of conservation agriculture on soil organic carbon and total nitrogen storage: A global meta-analysis. Sci Total Environment. (2022) 842:156822. doi: 10.1016/j.scitotenv.2022.156822
68. dos Santos NZ, Dieckow J, Bayer C, Molin R, Favaretto N, Pauletti V, et al. Forages, cover crops and related shoot and root additions in no-till rotations to C sequestration in a subtropical Ferralsol. Soil Tillage Res. (2011) 111:208–18. doi: 10.1016/j.still.2010.10.006
69. Vidal A, Blouin M, Lubbers I, Capowiez Y, Sanchez-Hernandez JC, Calogiuri T, et al. The role of earthworms in agronomy: Consensus, novel insights and remaining challenges. In: Sparks DL, editor. Advances in Agronomy, vol. 181. Elsevier, Amsterdam: Academic Press (2023). p. 1–78. doi: 10.1016/bs.agron.2023.05.001
70. Lana MA, Vasconcelos ACF, Gornott C, Schaffert A, Bonatti M, Volk J, et al. Is dry soil planting an adaptation strategy for maize cultivation in semi-arid Tanzania? Food Secur. (2018) 10:897–910. doi: 10.1007/s12571-017-0742-7
71. Manzeke-Kangara MG, Joy EJM, Lark RM, Redfern S, Eilander A, Broadley MR. Do agronomic approaches aligned to regenerative agriculture improve the micronutrient concentrations of edible portions of crops? A scoping review of evidence. Front Nutr. (2023) 10:1078667. doi: 10.3389/fnut.2023.1078667
72. Chilimba ADC, Young SD, Black CR, Rogerson KB, Ander EL, Watts MJ, et al. Maize grain and soil surveys reveal suboptimal dietary selenium intake is widespread in Malawi. Sci Rep. (2011) 1:72. doi: 10.1038/srep00072
Keywords: bulk density, climate change mitigation, climate-smart agriculture, Heavy Particulate Organic Matter-Carbon, Light Particulate Organic Matter-Carbon, Mineral Associated Organic Matter-Carbon, soil fractionation
Citation: Manzeke-Kangara MG, Ligowe IS, Tibu A, Gondwe TN, Greathead HMR and Galdos MV (2025) Soil organic carbon and related properties under conservation agriculture and contrasting conventional fields in Northern Malawi. Front. Soil Sci. 4:1481275. doi: 10.3389/fsoil.2024.1481275
Received: 15 August 2024; Accepted: 30 December 2024;
Published: 04 February 2025.
Edited by:
Emmanuel Arthur, Aarhus University, DenmarkReviewed by:
Ashim Datta, Central Soil Salinity Research Institute (ICAR), IndiaMuhammad Mohsin Abrar, Zhongkai University of Agriculture and Engineering, China
Copyright © 2025 Manzeke-Kangara, Ligowe, Tibu, Gondwe, Greathead and Galdos. This is an open-access article distributed under the terms of the Creative Commons Attribution License (CC BY). The use, distribution or reproduction in other forums is permitted, provided the original author(s) and the copyright owner(s) are credited and that the original publication in this journal is cited, in accordance with accepted academic practice. No use, distribution or reproduction is permitted which does not comply with these terms.
*Correspondence: Muneta G. Manzeke-Kangara, Z3JhY2Uua2FuZ2FyYUByb3RoYW1zdGVkLmFjLnVr