- 1School of Environment, Resources and Sustainability, University of Waterloo, Waterloo, ON, Canada
- 2Department of Chemistry, University of Waterloo, Waterloo, ON, Canada
- 3Department of Plant Agriculture, University of Guelph, Guelph, ON, Canada
Modeling plays an important role in predicting the long-term effects of biochar on soil organic carbon dynamics. The objective of our study was to apply the Century model to assess changes in temporal soil organic carbon in soil amended with manure and nitrogen fertilizer (MN), with manure and biochar (MB) or with manure, nitrogen fertilizer and biochar (MNB). We determined that, after 115 years, soil organic carbon stocks could not reach a steady state (equilibrium) or pre-cultivation levels, regardless of amendment type. Our results showed that a biennial input of manure and nitrogen fertilizer (MN) led to a 84% increase in soil organic carbon compared to a 79% (MNB) and 70% (MB) increase when amendments contained biochar. However, the quantity of organic matter input from crop residues and amendments was sufficient to increase the active fraction, with a turnover time of months to years, by 86%. In fact, carbon associated with the slow fraction, with a turnover time of 20 to 50 years, was the key driver for soil organic carbon accumulation in all amendment types. Although the passive fraction is the most stable form of carbon in the soil, with a turnover time of 400 to 100 years, once manure and biochar were added to the soil, this fraction increased up to 32%. Our results provided further insight into the ability of Century to accurately predict changes in soil organic carbon stocks when a combination of manure, nitrogen fertilizer or biochar were added to soil. Century predicted soil organic carbon stocks within -1% to +9% of measured values. However, further fine-tuning of the model is required since biochar undergoes chemical transformations (e.g., ageing) and changes soil physical parameters (e.g., bulk density) that can not be currently accounted for in the Century model. Addressing these limitations of Century will also help to increase the relationship between measured and predicted values.
1 Introduction
Over the last 200 years, land conversion followed by intensive agricultural practices was the primary agent for drastic losses of soil organic carbon (1). For example, land conversion from undisturbed ecosystems to managed agroecosystems depleted soil organic carbon stocks by 30% to 50% within five decades in temperate environments (2). Due to the decline in soil organic carbon stocks, coupled with soil erosion and declining soil fertility, sustainable approaches to agricultural management (e.g., conservation agriculture) emerged (3). To date, conservation agriculture has helped to improve soil structure, microbial diversity and biomass, water infiltration and storage, and plant nutrient availability due to greater soil organic matter retention (3). Thus, increased levels of soil organic matter are the driving force associated with improved soil properties under conservation agriculture (4).
Sandhu et al. (5) suggested that biochar, a carbon rich material produced by the pyrolysis (thermochemical conversion) of biomass, could also be used as an organic amendment to help ameliorate degraded soils. This is because biochar influences soil chemical and physical properties that encourage microbial activity (6). To date, most studies took place in tropical agroecosystems (7). This is because biochar raises soil pH, which improves soil microbial activity, and consequently increases soil fertility (7). Since soils in the temperate zone are inherently more fertile than tropical soils, the effect of biochar on soil physicochemical and biological properties and its ability to improve soil productivity remains inconclusive (8). However, Lévesque et al. (8) concluded that in temperate agroecosystems a broader view of biochar, moving beyond soil and crop productivity, may be preferable due to its potential to sequester carbon. Although biochar properties can vary widely depending on the type of feedstock and pyrolysis conditions, its aromatic structure causes it to be exceptionally recalcitrant, resisting chemical and biological degradation, making it stable in soil for hundreds of years (9). Thus, biochar has received attention from numerous researchers as a potential pathway for carbon sequestration and climate change mitigation (5).
To date, the majority of studies (>60%) evaluated the impact of biochar on soil using high application rates ranging from 1000 g m-2 to 3000 g m-2 (10). High biochar application rates were based on research that suggested more than 1000 g m-2 are required to observe a positive impact on temperate soil physicochemical properties (9). However, it is currently difficult to obtain a sufficient supply of high-quality biochar for large-scale agricultural applications (11). Additionally, Oelbermann et al. (11) suggested that high application rates are not economically feasible due to a shortage of high-quality biochar. In a systematic review of more than 25 meta-analyses on the use of biochar in agriculture, Schmidt et al. (12) observed a shift in the approach to biochar research in agriculture. They found that a handful of studies incorporated a low application rate of biochar in combination with other amendments including manure, mineral fertilizers, or other waste products (e.g., biosolids). These studies, however, focused on how a low biochar dosage affected soil properties and crop productivity (13–16), its impact on climate change resilience (17), with minimal information on its carbon sequestration potential (18).
Because of the extended time frame required to evaluate changes in soil organic carbon stocks, process-based models are a powerful approach to understand the drivers of long-term soil organic carbon dynamics and provide an opportunity to identify if biochar addition to agricultural soil causes carbon accumulation or loss (19). The Century model is one of the most widely used biogeochemical models due to its ability to predict soil organic carbon changes in diverse environments ranging from forests and grasslands to agricultural soil (20). But biochar modeling remains limited (21), including the outcome on changes in soil organic carbon with low biochar application rates (22). Furthermore, incorporating measured data from field studies with biochar into models is missing (23), and existing studies evaluated long-term biochar mineralization based on data collected from laboratory or short-term field studies (21). The objective of our study was to use the Century model to evaluate long-term changes in soil organic carbon stocks in soil amended with a low biochar application rate when blended with manure, and (or) nitrogen fertilizer. We also evaluated changes in the associated active, slow, and passive carbon fractions, and determined the relationship between measured and predicted values.
2 Methods
2.1 Field site description and management
The field site was located on a commercial poultry-cash crop farm in Bayfield, Ontario, Canada (43°34’45.8”N, 81°39’52.2”W). The area was located 183 m above sea level with a 1.5% slope. Weather data (30-year mean) was obtained from a nearby weather station located in Dashwood (43°20’48.8”N, 81°38’07.6”W), Ontario with a mean annual temperature of 8.3°C and an average annual precipitation of 858 mm (Figure 1). The soil was classified as a uniform calcareous Grey-Brown Luvisol with a sandy loam texture. In the years before biochar addition (prior to May 2016), commercial farming practices of the study site included an annual rotation of maize (Zea mays L.) and soybean (Glycine max Merr. L.). Poultry manure, based on switchgrass bedding (Panicum virgatum L.), was added in April at 600 g m-2 and topped off with nitrogen fertilizer at 135 kg N ha-1 in the years when maize was produced (Table 1). The soil was cultivated in April of each field season using a disc harrow (0-20 cm) and weeds were controlled with N-phosphonomethyl glycine (Glyphosate).
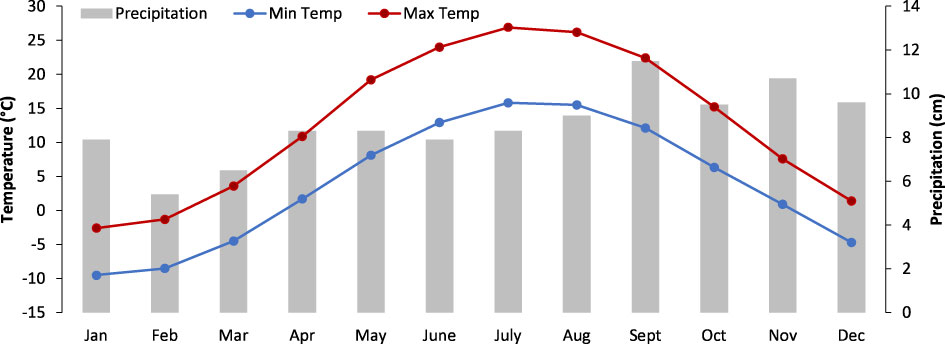
Figure 1 Thirty-year average climate data used to calibrate Century. Climate data was obtained from the Dashwood, Ontario Meteorological Station (Environment and Climate Change Canada). Minimum and maximum temperature are represented by the blue and red line graph and precipitation by the grey bar graph.
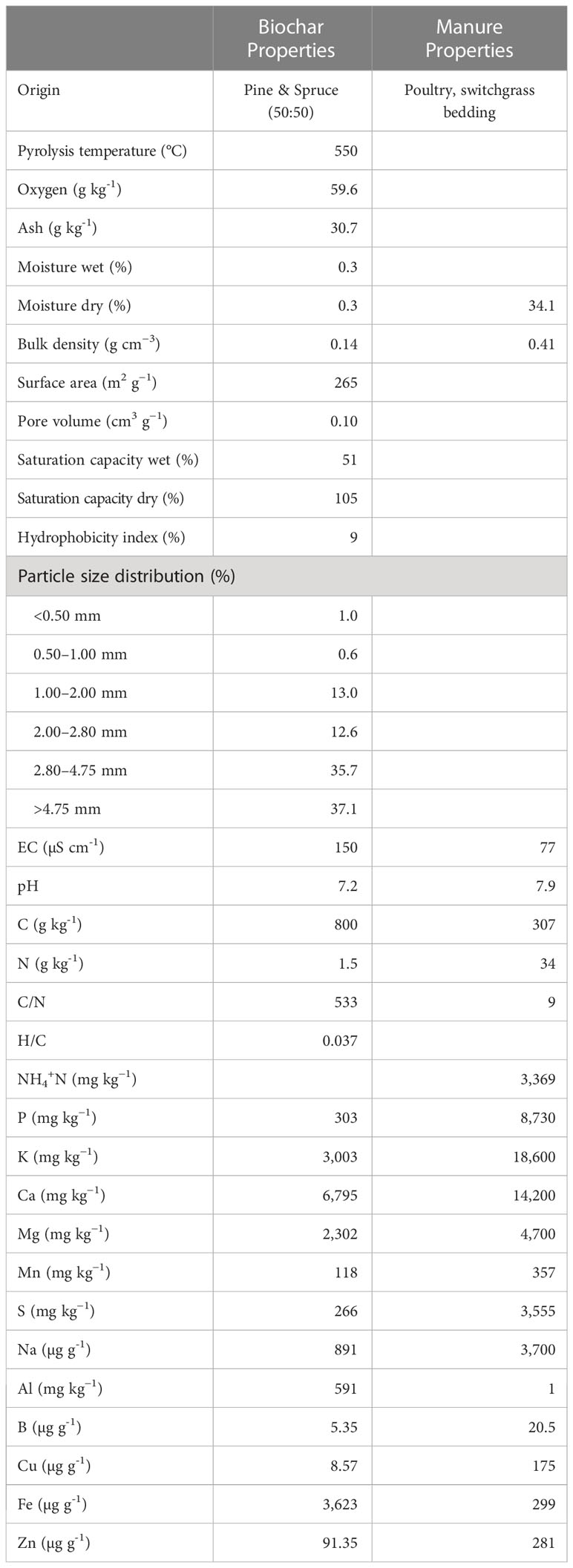
Table 1 Physicochemical characteristics of the manure and biochar applied to soil in southern Ontario, Canada.
The field experiment was established in May 2016 and included 600 g m-2 poultry manure + 13.5 g N m-2 fertilizer (MN); 300 g m-2 poultry manure + 300 g m-2 biochar (MB); and 300 g m-2 poultry manure, 13.5 g N m-2 fertilizer + 300 g m-2 biochar (MNB). The experimental design was a randomized design, and each treatment was replicated three times. The plot size for each treatment replicate was 10 m x 10 m, with a 3 m border between plots. Biochar was added using a drop spreader and incorporated into the soil using a Salford RTS vertical tillage unit to ensure uniform distribution of the biochar. Commercial farm management operations, including herbicide additions and fertilizer application rates, used standard agronomic practices for this region of southwestern Ontario, Canada. The field study was conducted over three growing seasons, beginning in May 2016 with a maize crop and biochar addition, a soybean crop in 2017 and a maize crop in 2018. Biochar was added to the respective treatment replicates only in 2016, whereas manure and nitrogen fertilizer were added biennially when maize was produced. No soil amendments were added during the years soybeans were produced. The biochar was provided by Titan Carbon Smart Technologies (Saskatoon, Saskatchewan, Canada). The feedstock of the biochar was a 1:1 mix of pine (Pinus spp.) and spruce (Picea spp.) (Table 1). Biochar was produced using slow pyrolysis (550°C, 15 min).
2.2 In field data collection
Prior to the initiation of the study, five soil samples (0-20 cm) were collected in April 2016 from each treatment replicate to determine baseline values. Further soil samples were collected from each treatment replicate after crop harvest in October 2016, 2017, and 2018. Fresh soil was used to quantify pH using a 1:1 soil and ultrapure water mixture using a BioKit AB15B (Houston, TX, USA) pH meter (24). The remaining soil was air dried and sieved to 2 mm. Carbonates were removed by washing 2 g of soil with 50 mL of 0.5 M HCl (25). The soil-acid solution was shaken three times over 24 h on a reciprocating shaker at 200 rpm (Heidolpj Unimax 1010 DT, Schwabach, Germany). Following a settling period of 30 min, the acid was removed using a pipette and discarded (25). The acid treated soil was washed by adding 50 mL ultrapure water and shaking the soil at 200 rpm for 15 minutes, after which the water was removed with a pipette and discarded (25). The washing procedure was repeated daily for four days after which the soil was dried at 30°C for two days, ground in a ball mill (Retsch® ZM1, Haan, Germany) and analyzed for soil organic carbon and total nitrogen using a Costech 4010 (Valencia, USA) elemental analyzer.
2.3 Century parameterization
Century is a site-specific model based on the interactions between climate, crops, and soil. Parameterization of the model can be achieved by modifying climate, soil chemical, physical and biological characteristics, and agroecosystem management practices. Plant production is a sub-model in century that stimulates aboveground and belowground biomass productivity of forests, grasslands, savannas, or crops (26). The soil organic matter sub-model predicts changes in soil active, slow, and passive carbon fractions based on microbial decomposition of crop residues and resultant microbial products that are the foundation in the formation of humus (20). Of the total soil organic matter pool, approximately 2% to 4% is composed of the active fraction that includes soil microbes and microbial products with a turnover time of several months to a few years (27). The slow fraction composes 45% to 65% of the total soil organic matter pool with a turnover time of 20 to 50 years and includes resistant plant material acquired from stabilized soil microbial products and structural plant material (27). The passive fraction contains 30% to 40% of the total soil organic matter pool and is represented by chemically and physically protected mineral associated organic material (20). Soil texture controls the extent to which the decomposed compounds are stabilized (26). The Century model was originally developed to determine changes in soil organic carbon stocks for grassland ecosystems (26), but the model can also simulate changes in temperate and tropical environments that include various land management systems ranging from row cropping to complex agroecosystems (28, 29). To date, Century is one of the most widely used models because of its ability to simulate changes in soil organic carbon in diverse environments (20).
We used version 4.0 of the Century model to simulate changes in soil organic carbon and its associated fractions (0-20 cm) after a low dosage and one-time addition of biochar. The model was initiated for >10,000 years under a mixed deciduous-coniferous forest which is native to this region of southwestern Ontario, Canada to estimate equilibrium in plant productivity and soil organic carbon levels i.e., when soil organic carbon stabilizes and there are no further changes with time. After equilibrium was attained the first year of simulation began in 1882 with grass/hay for livestock production (Table 2). Monthly average maximum and minimum temperature and monthly total precipitation (30-year average) were obtained from a nearby Environment and Climate Change Canada meteorological station managed in Dashwood, Ontario (Figure 1). After initializing soil organic carbon levels, land management events were scheduled according to actual historical events prior to the addition of biochar in 2016 (Table 2). Soil properties prior to the addition of biochar (Table 3) were obtained from Jiang (30). The proportion of soil organic carbon initial values for the active fraction was 3%, 65% for the slow fraction and 32% for the passive fraction (31). The OMAD.100 file was changed and reflected the addition of 184 g C m-2 manure for MN, or 91 g C m-2 manure + 240 g C m-2 biochar for MB and MNB (Table 4). The C/N ratio of the manure and biochar were also adjusted accordingly (Table 4). An additional file in FERT.100 was created to reflect the addition of 13.5 g m-2 nitrogen fertilizer to MN and MNB. Crop parameters for maize and soybeans were modified to accommodate regional crop production values (Table 4). Default values set by Century were used for atmospheric nitrogen deposition and nitrogen fixation rates. All other parameters used in this simulation were provided by Century and modified parameters are listed in Table 4. We used a monthly time-step for simulation output to align predicted values with their corresponding month of soil sampling in the field.
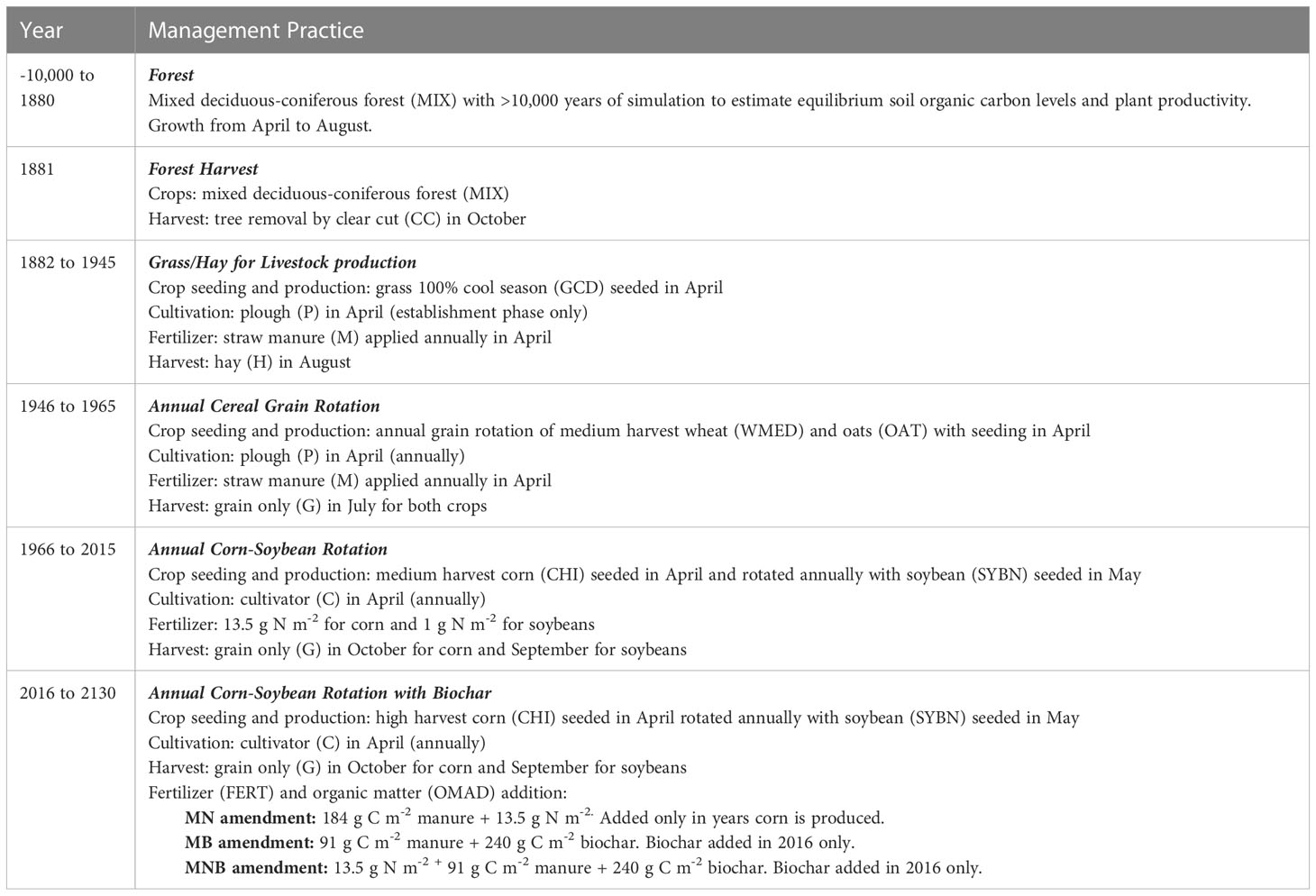
Table 2 Scheduling of agricultural management practices in Century based on actual historical events that occurred at the field site in Ontario, Canada.
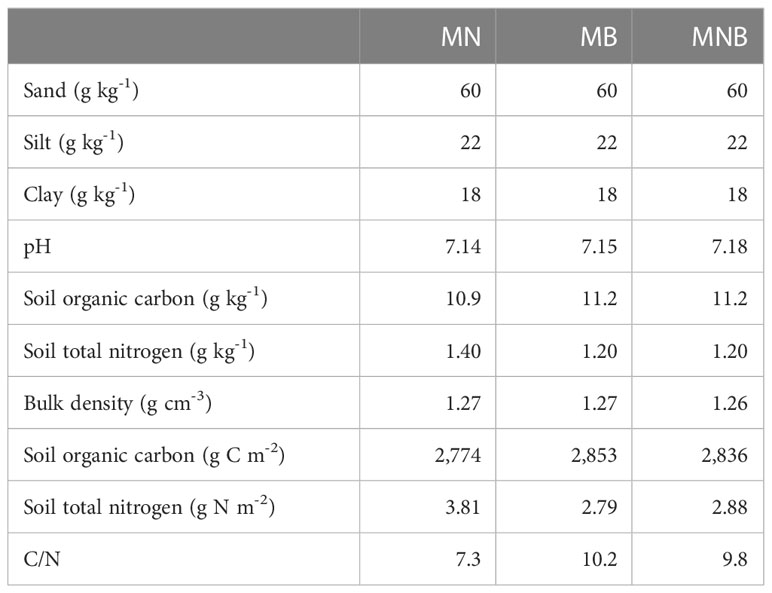
Table 3 Soil physicochemical characteristics (0-20 cm) prior to adding manure and biochar in 2016 at the field site in southern Ontario, Canada (adapted from 30).
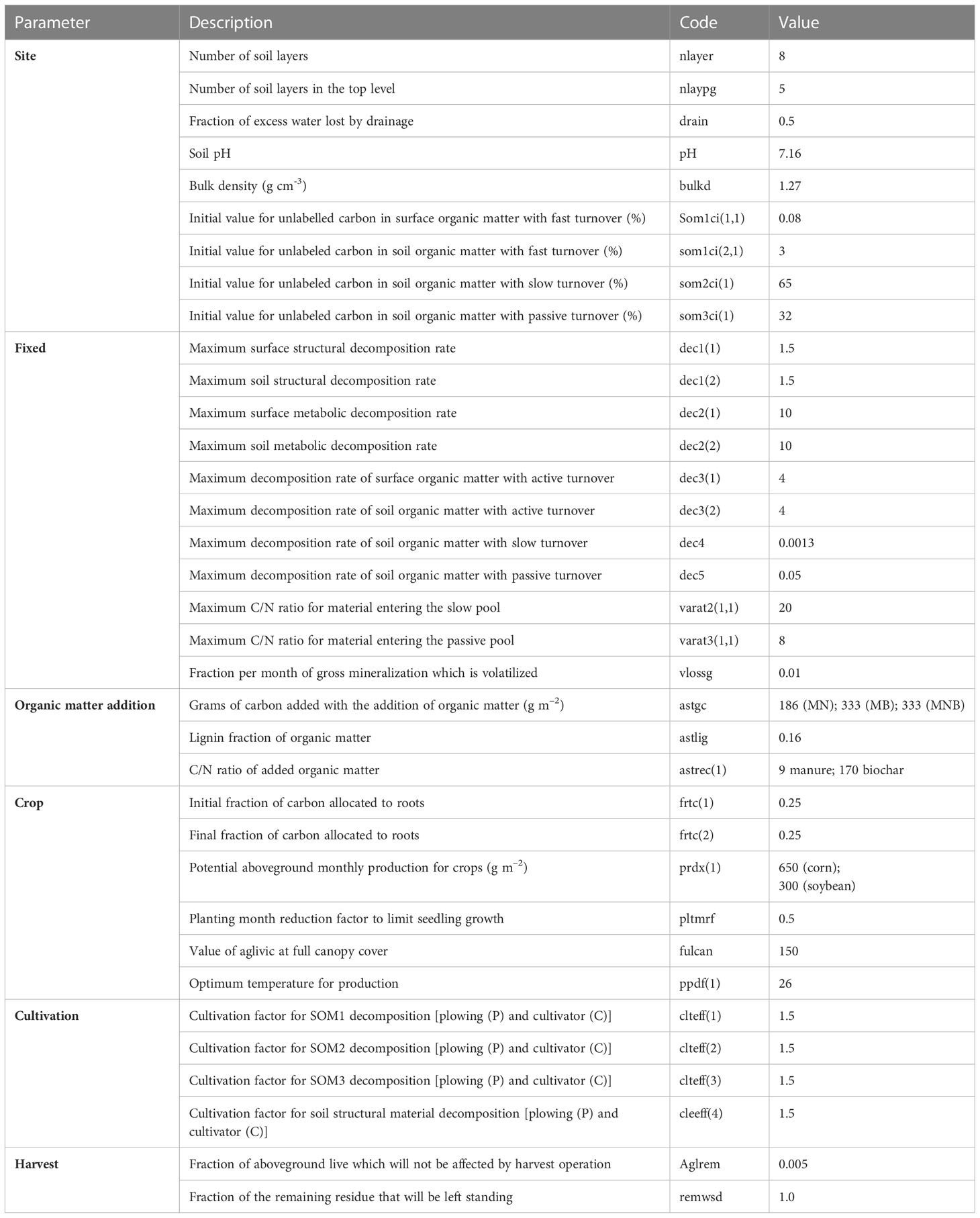
Table 4 Century parameters measured or modified during model calibration for soil amended with manure + N fertilizer (MN), manure + biochar (MB), and manure, N fertilizer + biochar (MNB) in southern Ontario, Canada.
2.4 Statistical analysis
Measured field data were compared with predicted data using the regression function in SPSS version 28 (33). The following metrics were used to determine the strength of the relationship between measured and predicted values: correlation coefficient (r), coefficient of determination (r2), adjusted r2, root mean squared deviation (RMSD), root mean squared error (RMSE), the mean difference (M) between measured and predicted values of soil organic carbon, the coefficient of residual mass (CRM) to determine if the model over- or under estimated values of soil organic carbon, and modeling efficiency (EF) which compares predicted values to the average measured values (34, 35). The threshold probability level for determining goodness of fit was P < 0.05.
3 Results
The introduction of grass and hay forage for livestock production between 1882 and 1945 caused soil organic carbon stocks to decline. In fact, the soil organic carbon stock decreased by 12% when mixed deciduous-coniferous forest was converted to grass and hay forage production in 1882. Soil organic carbon stocks further declined by 16% during the annual cereal grain rotation (1946 to 1965) and declined by another 23% during the annual corn-soybean rotation between 1966 and 2015 (Figure 2). Soil organic carbon stocks began to recover in 2016 with the addition of manure or manure blended with biochar and (or) nitrogen fertilizer. However, 115 years, pre-1881 soil organic carbon stock that accumulated under a mixed deciduous-coniferous forest were not reached regardless of amendment type added (Figure 2).
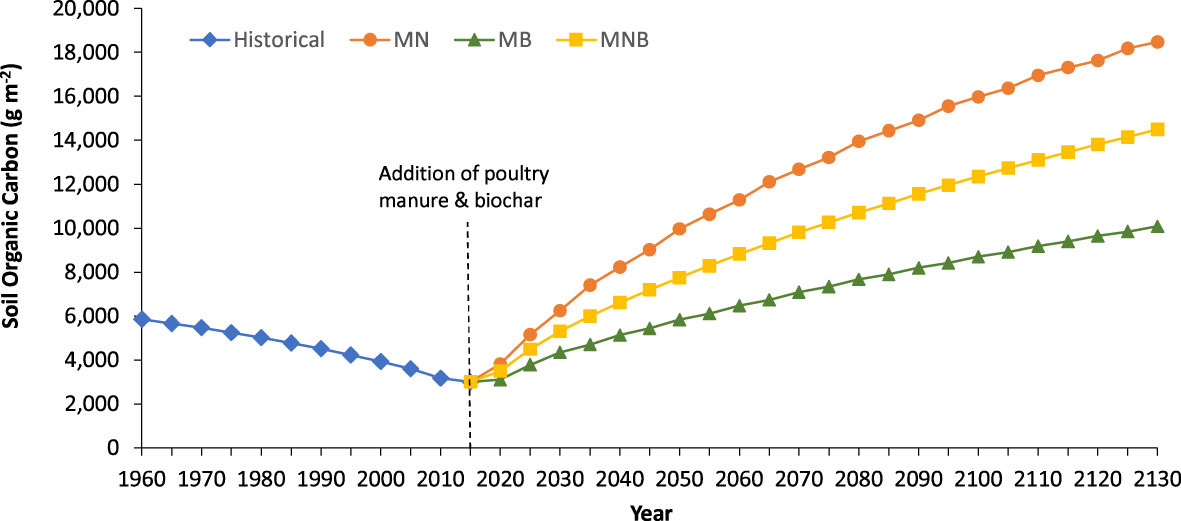
Figure 2 Soil organic carbon predicted by Century in soil amended with manure + N fertilizer (MN), manure + biochar (MB), and manure + N fertilizer + biochar (MNB) in southern Ontario, Canada beginning in 2016 (dashed vertical line). Prior to 2016, the site was under a corn-soybean rotation that included the addition of nitrogen fertilizer (c.f., Table 2 for historical management practices).
When sustainable agroecosystem management practices began in 2016, the Century predicted an 84% increase, after 115 years, in soil organic carbon when 600 g m-2 manure was blended with nitrogen fertilizer (MN). But when manure was cutback to 300 g m-2 and blended with 300 g m-2 biochar (MB), the soil organic carbon stock increased only by 70%. However, when 300 g m-2 of manure was blended with biochar (300 g m-2) and nitrogen fertilizer (MNB), the soil organic carbon stock increased by 79% (Figure 2).
When manure or manure blended with biochar and (or) nitrogen fertilizer and added to the soil starting in 2016, all carbon fractions increased (Figure 3). For example, the active and slow fractions showed the greatest increase in soil organic carbon. An increase in the active fraction ranged from 68% to 86% and increase in the slow fraction ranged from 78% to 88%. For both active and slow fractions, the MN amendment type always had the greatest increase followed by the MNB and MB amendment types. Although the passive fraction is the most stable form of carbon in the soil, the addition of manure with nitrogen fertilizer (MN) led to a 32% increase in this fraction. Likewise, the presence of biochar in MB and MNB led to a 12% and 23% increase, respectively, in the passive fraction.
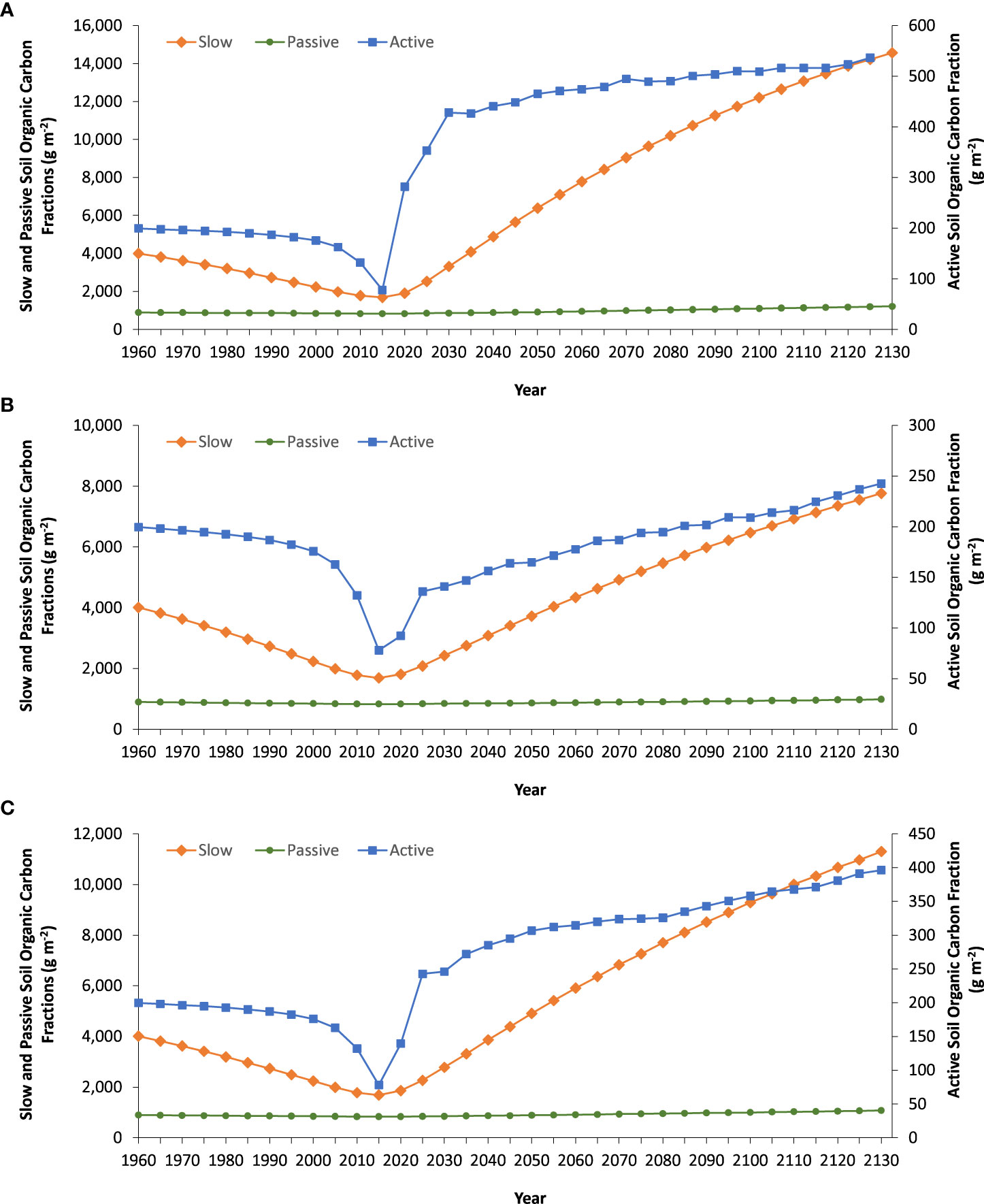
Figure 3 Soil organic carbon fractions predicted by Century in soil amended with (A) manure + N fertilizer (MN), (B) manure + biochar (MB), and (C) manure + N fertilizer + biochar (MNB) in southern Ontario, Canada beginning in 2016. Prior to 2016, the site was under a corn-soybean rotation that included the addition of nitrogen fertilizer.
Model performance was very good to good with a strong relationship between measured field data and predicted values for soil organic carbon stocks in MN and MB, with P-values ranging from 0.005 to 0.017 (Table 5). Model performance for MNB was poor and did not show a significant relationship (P=0.341) between measured and predicted values. The near zero values for the coefficient of residual mass (CRM) for all amendment types indicated a lack of bias in the distribution of the predicted values with respect to the measured values. Century overestimated soil organic carbon stocks in MN by 9% and by 4% in MNB but underestimated by 1% for MB (Figure 4). However, overall model performance was good since EF values were close to 1.0, indicating a near perfect fit.
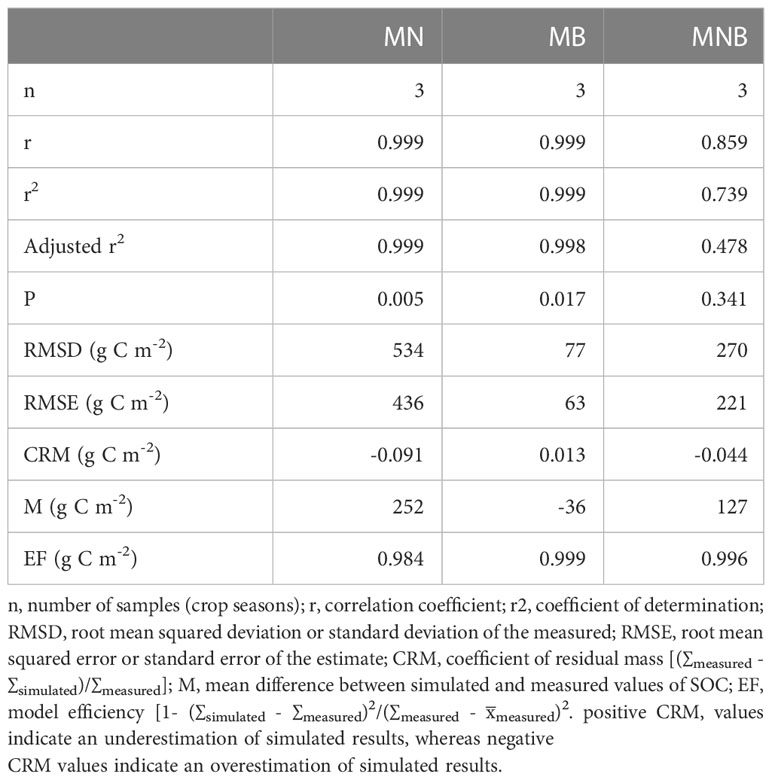
Table 5 Statistical tests applied for agreement between measured and predicted values of soil organic carbon stocks in soil amended with manure + N fertilizer (MN), manure + biochar (MB), and manure + N fertilizer + biochar (MNB) in southern Ontario, Canada.
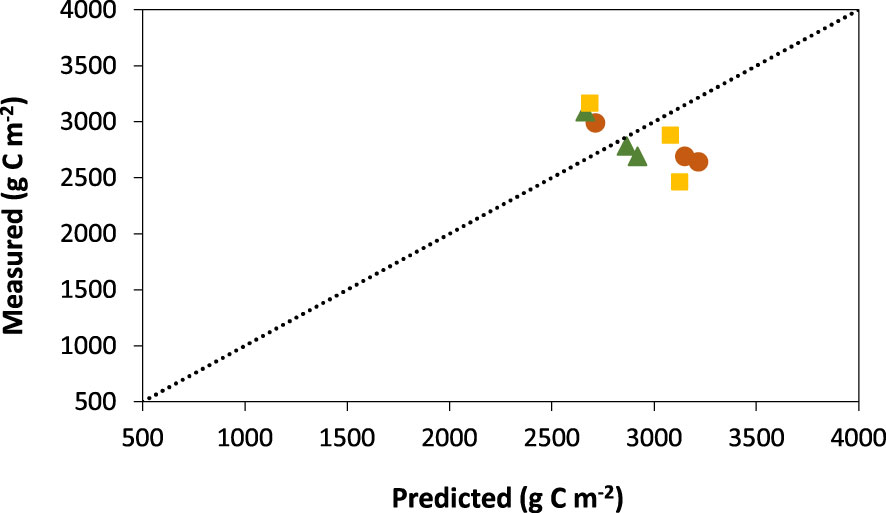
Figure 4 Relationship between measured and predicted values of soil organic carbon in soil amended with manure + N fertilizer (MN; orange circles), manure + biochar (MB; green triangles), and manure + N fertilizer + biochar (MNB; yellow squares) in southern Ontario, Canada.
4 Discussion
4.1 Soil organic carbon stock predictions
We found that land conversion from mixed deciduous-coniferous forest to grass and hay production for livestock forage, and subsequent conversion to row crop production led to a rapid decline in soil organic carbon stocks and associated carbon fractions. Such a decline in soil organic carbon stocks upon land conversion to agriculture have been observed on a global scale (36), and losses will likely be heightened in the future due to a changing climate (37) since warming enhances microbial activity and the transformation of soil organic carbon (1). Additionally, agriculture also causes a fundamental disruption in the soil’s steady state due to a drastic reduction in the quantity and quality of organic matter returned to the soil that leads to a depletion of soil organic carbon stocks (39, 40). For example, crop residue removal has increased from 0.5 Pg C y-1 to 1.6 Pg C y-1 between 1910 and 2005 due to heightened demands for food, fibre, and fuel (39, 41). Furthermore, Li et al. (42) determined that a 25% reduction in organic matter input caused a 1.6% decrease in soil organic carbon. In fact, we found an 51% decrease in soil organic carbon over 113 years, representing the time from initiation of agriculture in 1881 to 2015. Our results corroborate with those of Powlson et al. (43) who reviewed data from multiple long-term experiments in temperate and tropical agroecosystems. They determined that soil organic carbon stocks declined between 38% and 67% compared to pre-cultivation levels (43). Although our study showed a considerable increase (84%) in soil organic carbon stocks over 115 years once manure and (or) biochar were added, we found that pre-cultivation soil organic carbon stocks were not reached. Similarly, Powlson et al. (43) found that increases in soil organic carbon levels ranged from 60% to 70% of pre-cultivation values once conservation practices were initiated. But they concluded that soil organic carbon stocks will likely never approach pre-cultivation levels (43). This is because the rate of soil organic carbon loss and accumulation is influenced by land management practices including the quantity and quality of residue returned to the soil and the type and frequency of tillage (39, 44). Furthermore, there is an upper limit of carbon storage that depends on the soil’s inherent soil organic carbon level which is closely tied to soil texture (45). However, we also found that after 115 years of manure and (or) biochar addition, soil organic carbon equilibrium was not reached. This was likely due to an inherently low soil organic carbon level in the coarse textured soil (60% sand, 22% silt, 18% clay) used in our study. This suggested that soil amended with manure and (or) biochar has a greater carbon sequestration potential if the soil texture is coarse, since silt and clay content control the upper level (saturation) of soil carbon sequestration (46).
Although carbon input in MB and MNB (331 g C m-2) was almost double compared to that in MN (184 g C m-2), soil organic carbon accumulation was greater in MN after 115 years. This was due to the biennial addition of manure (i.e., in the years when maize was produced) since Century was scheduled to mirror commercial farming practices that took place at the field site. Conversely, Century was scheduled, paralleling field site management practices, only by a one-time addition of biochar to MB and MNB. Therefore, over the 115-year simulation carbon input from manure was cumulative and contributed to the greater soil organic carbon stock in MN. We therefore recommend that a more frequent low dosage of biochar should be applied in future field studies that can further increase soil organic carbon stocks. For example, Dil and Oelbermann (22) used Century and determined that an annual addition of biochar at 200 g C m-2 increased soil organic carbon stock by 10,522 g m-2 over 150 years in a light textured soil. However, the study by Dil and Oelbermann (22) did not integrate field data into their simulation. Although there is a wide variation in the ability of manure to increase soil organic carbon stocks over the long-term (>5 years), a recent meta-analysis by Gross and Glaser (47) determined that manure increased soil organic carbon stocks, regardless of environmental conditions. For example, they found a 40% increase in soil organic carbon after 20 years in temperate agroecosystems (47). However, our results were comparable to those by Jenkinson and Rayner (48) who predicted changes in soil organic carbon over a 120-years using field data from long-term research sites in England. Furthermore, nitrogen availability becomes unrestricted when a legume is integrated into the rotation (49). The greater nitrogen availability from the legume decreases the mineralization of old carbon and leads to the accumulation of soil organic carbon (50). This could also explain the difference in soil organic carbon stocks in our study (e.g., MN) compared to Gross and Glaser (47), who did not include legumes in their meta-analysis.
Compared to the pre-2016 land management practice, amendments with biochar also increased soil organic carbon stocks, over the 115-year simulation, by ≥70%. This is because biochar, due to incomplete combustion, is the only plant-derived organic matter that as a higher intrinsic stability than other sources of organic matter (51). Although biochar is stable over the long-term, it is also affected by microbial degradation depending on the pyrolysis conditions under which it was produced (52). For example, a high pyrolysis temperature, carbon to nitrogen ratio and a pH >8.1 caused an increase in soil organic carbon (52). However, biochar produced with a low pyrolysis temperature and narrow carbon to nitrogen ratio, like that used in our study, is more readily available to the microbial community (52). This could also explain the lower soil organic carbon stock predicted by Century in our study in MB and MNB compared to MN. Furthermore, the soil used in our study was sandy, which does not provide a physical and chemical protection mechanism for the biochar, allowing the readily decomposable fractions to be more accessible to the microbial community (52).
The greater accumulation of soil organic carbon in MNB, compared to MB, over the 115-year simulation was likely due to the presence of nitrogen fertilizer combined with manure and biochar. Previous studies showed that an effective way to promote efficient soil organic matter formation includes the input of a diverse source of organic materials (1). This is because diverse sources of organic matter (i.e., molecular diversity) causes an energy limitation on microbes, decreasing soil organic matter mineralization (53). Additionally, van Groenigen et al. (54) found that an increasing input of nitrogen leads to an accumulation of soil organic carbon which, in our study, likely contributed to the greater soil organic carbon stock in MNB. In a 15-week mesocosm study, Ibrahim et al. (55) determined that biochar blended with fertilizer regulated carbon mineralization because more nitrogen substrates were available to the microbial community that depended on nitrogen. Although blending biochar with manure and a source of mineralizable nitrogen is contradictory with respect to potentially greater greenhouse gas emissions, results from a previous study at the same field site as our study showed no significant difference in CO2 and N2O emissions among amendment types (16). We also determined that model performance for MNB was poor, compared to MB, and it did not show a significant relationship between measured and predicted values. We recommend that more reliable simulation outcomes should be based on a greater number of data points (e.g., number of years) from field measurements to provide a more robust comparison with predicted values.
Soil organic carbon fractions decreased upon initiation of agricultural activities in 1882, with little change in the passive fraction. However, all three fractions increased after 2016, once manure and (or) biochar were added, where the greatest rise was observed in the active and slow fractions. This suggested that the active and slow carbon fractions were controlled by land-use change (i.e., change from the pre-cultivation forest ecosystem to cultivated agroecosystems) and different agroecosystem management practices (i.e., change from grass/hay production to cereal and maize crop rotations). Land conversion and changes in land-use management decreased primary production, soil nutrient status and the quantity and quality of organic matter returned to the soil compared to pre-cultivation levels (39). This likely led to the mineralization of old carbon sources, causing a decline in the various carbon fractions (56). However, a sufficient input of organic matter, and therefore an increase in each of the carbon fractions, occurred once manure and (or) biochar were applied to the soil. For example, Gross and Glaser (47) determined that soil with a low initial soil organic carbon stock will have a large response to the addition of organic matter and show no depletion with time. Also, the addition of organic matter increased nitrogen availability, which affected microbial growth and the mineralization of new carbon derived from the amendments (57). This suggested that carbon stabilization is controlled by the quantity and quality of the organic residues entering the soil ecosystem.
The greater accumulation of carbon in the active and slow fractions in MN, compared to MB and MNB, was due to the biennial addition of manure (47). However, the one-time addition of biochar, when blended with manure and nitrogen fertilizer (MNB), led to a similar accumulation of carbon in the active and slow fractions as MN. This suggested that biochar increased soil organic carbon stabilization within the organo-mineral fraction (60). Additionally, our results showed that the active and slow carbon fractions did not reach equilibrium during the 115-year simulation period. Gross and Glaser (47) suggested that soil carbon saturation not only depends on the properties of the soil and of the organic matter added to the soil, but also on the inherent soil organic carbon content. They found that soil with an inherently low soil organic carbon content requires a longer time to reach equilibrium compared to soil with an inherently high soil organic carbon content (47).
Input from the various carbon sources such as manure or manure blended with biochar likely stimulated the microbial community to become more active, which may have preserved the active carbon pool despite disturbance from tillage. For example, Ouyang et al. (61) also found that the addition of manure counteracted the impact of tillage on the active soil organic carbon fraction in northeastern China. Lorenz and Lal (52) also determined that when biochar was added to a coarse-textured soil, it caused an increase in soil-specific surface area that enhanced soil nutrient cycling and microbial activity. Furthermore, our modeling results determined that carbon associated with the slow fraction was the key driver in soil organic carbon accumulation once manure and (or) biochar were added to the soil. We also found that the slow fraction resisted the impact of disturbance from cultivation with a disc harrow (0-20 cm depth) and soil organic carbon was maintained by input of manure and (or) biochar. This is because the slow fraction is physically protected and has a more chemically complex structure, which makes it more resistant to decomposition than the active fraction (20). Additionally, Carvalho-Leite et al. (62) determined that carbon accumulation in the slow fraction is related to the quantity carbon and nutrients added to the soil. Although Cong et al. (63) found that nitrogen fertilizer decreased carbon in the passive fraction, our simulation results showed a 29% increase in the passive fraction in MNB, compared to a 18% in crease in MN and MB. Pulcher et al. (21) suggested that biochar carbon may not be directly mineralized to CO2 but may transfer to carbon pools with different degradation rates. This is because the biochar used in our study has a hydrogen-to-organic carbon ratio lower than 0.4, which is considered highly stable with carbon remaining in soil for more than 100 years (64). Accordingly, results from our study predicted that a one-time addition of manure blended with biochar and nitrogen fertilizer (MNB) can stabilize a greater proportion of the carbon in the passive fraction than when blending manure with nitrogen fertilizer (MN) or when bending manure with biochar (MB).
4.2 Model performance and limitations
Differences between measured and predicted values in our study ranged from +9% to -1.3% and was within range of other studies. For example, Alves Primo et al. (29) determined that Century over- and underestimated soil organic carbon stocks up to 15% in semiarid slash and burn and agroforestry systems in Brazil. Similarly, in temperate agroecosystems soil organic carbon stocks deviated between +10% to -15% between measured and predicted values (38, 65). However, our study was limited by the availability of only three years of field data for model validation. Discrepancies between measured and predicted values in our study were also due to Century’s failure to accommodate changes in bulk density with increasing soil organic carbon stocks. Instead, Century assumes bulk density as a constant parameter throughout the simulation (58). With increasing soil organic carbon stocks, bulk density decreases with time, influencing the quantity of carbon sequestered (47). For example, Lorenz and Lal (52) noted that biochar could reduce soil bulk density by 3 to 31%. Various environmental factors including fluctuating moisture and temperature conditions, freeze-thaw events and microbial activity control the dissolution, oxidation and fragmentation of biochar that can change its physicochemical characteristics (59). For example, Wang et al. (32) found that soil minerals accumulated on the surface of the aged biochar and suggested that this improved soil physical stability which could lead to enhanced carbon sequestration. Therefore, the soil organic carbon stock in amendments with biochar may be an underestimation in our study since Century does not account for biochar ageing. However, it is also crucial to consider how soil physicochemical properties and soil amendments (e.g., manure, biochar) are affected by future climate change scenarios which could affect long-term soil organic carbon stocks. For example, Jiang et al. (17) determined that short-term climate effects of elevated CO2, warming or the combined effect of elevated CO2 and warming did not influence biochar. But they found that climate effects influenced how carbon was accessed by the microbial community in amendments with manure and biochar (17). Furthermore, leaching and bioturbation cause the downward translocation and stabilization of biochar within the soil profile (52). However, translocation of carbon (i.e., biochar) to deeper soil horizons is not accounted for in Century since the model only predicts soil organic carbon stocks to a 20 cm depth.
5 Conclusion
We evaluated if soil amended with biochar is able to sequester a greater quantity of soil organic carbon over the long-term (115 years), compared to soil without biochar, using the Century soil organic matter model. After 115 years, none of the amendment types were able to reach pre-cultivation soil organic carbon stocks and none of the amendment types reached equilibrium. The lower input of organic matter from crop residues and amendments, compared to a mixed deciduous-coniferous forest likely contributed to the inability to reach pre-cultivation soil organic carbon stocks. This was likely due to the inherently low level of soil organic carbon of the soil used in our study which required a longer time to reach a steady state. We found that Century predicted the greatest increase in soil organic carbon when manure was blended with fertilizer compared to soil amended with biochar. Although carbon input in amendments containing biochar had more carbon, the low dosage and one-time addition of biochar caused the slower accumulation of soil organic carbon compared to the biennial addition of manure. We also determined a greater increase in soil organic carbon when biochar was blended with manure and nitrogen fertilizer likely due to a greater regulation of carbon mineralization since more nitrogen substrates were available to the microbial community. Thus, biochar blended with manure and nitrogen fertilizer may have a different effect on soil organic carbon compared to biochar blended with manure only. Carbon associated with the slow fraction was the key driver in soil organic carbon accumulation for all amendment types. Although Century was able to predict soil organic carbon stocks within ±9% of measured values, we recommend that fine tuning the model to address some of its current limitations with respect to biochar (i.e., bulk density, ageing, bioturbation), which will strengthen the relationship between measured and predicted values.
Data availability statement
The raw data supporting the conclusions of this article will be made available by the authors, without undue reservation.
Author contributions
MO contributed to conception and design of the field study. RJ and MM organized the database and performed the analysis of the data collected in the field. MO wrote and edited the manuscript. MO contributed to funding acquisition. All authors contributed to the article and approved the submitted version.
Funding
We thank the Ontario Ministry of Agriculture, Food and Rural Affairs (OMAFRA) for providing funding (grant number ND2015-2708). The University of Waterloo and the Canadian Foundation for Innovation (grant number 12896) for providing research infrastructure. The Ontario Graduate Scholarship provided funding to RJ and the Natural Sciences and Engineering Research Council provided funding to MM.
Acknowledgments
We thank J. Poelman and T. Murray for providing access to, and management of, the field site, and J. Bakos of Titan Carbon Smart Technologies, Saskatchewan for the generous supply of the biochar. We also thank the reviewers and editors for their insightful suggestions to help improve this manuscript.
Conflict of interest
The authors declare that the research was conducted in the absence of any commercial or financial relationships that could be construed as a potential conflict of interest.
Publisher’s note
All claims expressed in this article are solely those of the authors and do not necessarily represent those of their affiliated organizations, or those of the publisher, the editors and the reviewers. Any product that may be evaluated in this article, or claim that may be made by its manufacturer, is not guaranteed or endorsed by the publisher.
References
1. Cotrufo FM, Lavallee JM. Soil organic matter formation, persistence, and functioning: a synthesis of current understanding to inform its conservation and regeneration. Adv Agronomy (2022) 172:166–231. doi: 10.1016/bs.agron.2021.11.002
2. Lal R. Beyond COP 21: potential and challenges of the “4 per thousand” initiative. J Soil Water Conserv (2016) 71:20A025A. doi: 10.2489/jswc.71.1.20A
3. Verhulst N, Govaerts B, Verachtert E, Castellanos-Navarrete A, Mezzalama M, Wall PC, et al. Conservation agriculture, improving soil quality for sustainable production systems? In: Lal R, Stewart BA, editors. Food security and soil quality. Boca Raton, Florida: CRC Press, Taylor and Francis (2010). p. 137–208.
4. Lal R. Sequestering carbon and increasing productivity by conservation agriculture. J Soil Water Conserv (2015) 70:55A–62A. doi: 10.2489/jswc.70.3.55A
5. Sandhu SS, Ussiri DAN, Kumar S, Chintala R, Papiernik SK, Malo DD, et al. Analyzing impacts of three types of biochar on soil carbon fractions and physicochemical properties in a corn-soybean rotation. Chemosphere (2017) 184:473–81. doi: 10.1016/j.chemosphere.2017.05.165
6. Kolb SE, Fermanich KJ, Dornbush ME. Effect of charcoal quantity on microbial biomass and activity in soil. Soil Sci Soc America J (2009) 73:1173–81. doi: 10.2136/sssaj2008.0232
7. Basak BB, Sarkar B, Saha A, Sarkar A, Mandal S, Biswas JK, et al. Revamping highly weathered soils in the tropics with biochar application: what we know and what is needed. Sci Total Environment (2022) 822:15346. doi: 10.1016/j.scitotenv.2022.153461
8. Lévesque V, Oelbermann M, Ziadi N. Biochar in temperate soils: opportunities and challenges. Can J Soil Science (2022) 102:1–26. doi: 10.1139/cjss-2021-0047
9. Atkinson CJ, Fitzgerald JD, Hipps NA. Potential mechanisms for achieving agricultural benefits from biochar application to temperate soils: a review. Plant Soil (2010) 337:1–18. doi: 10.1007/s11104-010-0464-5
10. Liu X, Zhang A, Ji C, Joseph S, Bian R, Li L, et al. Biochar’s effect on crop productivity and the dependence on experimental conditions - a meta-analysis of literature data. Plant Soil (2013) 373:583–91. doi: 10.1007/s11104-013-1806-x
11. Oelbermann M, Berruti F, Lévesque V. Biochar and its use in soil: lessons from temperate agriculture. World J Agric Soil Science (2020) 5:1–9. doi: 10.33552/WJASS.2020.05.000610
12. Schmidt HP, Kammann C, Hagemann N, Leifeld J, Bucheli TD, Sanchez Monedero MA, et al. Biochar in agriculture – a systematic review of 26 global meta-analyses. Global Change Biol Bioenergy (2021) 13:1708–30. doi: 10.1111/gcbb.12889
13. Dil M, Oelbermann M, Xue W. An evaluation of biochar pre-conditioned with urea ammonium nitrate on maize (Zea mays l.) production and soil biochemical characteristic. Can J Soil Science (2014) 94:551–62. doi: 10.4141/cjss-2014-010
14. Mechler MA, Jiang WR, Silverthorn TK, Oelbermann M. Impact of biochar on soil characteristics and temporal greenhouse gas emissions: a field study from southern Canada. Biomass Bioenergy (2018) 118:154–62. doi: 10.1016/j.biombioe.2018.08.019
15. Liang JF, Li QW, Gao JQ, Feng JG, Zhang XY, Wu YQ, et al. Biochar rhizosphere addition promoted phragmites australis growth and changed soil properties in the yellow river delta. Sci Total Environment (2021) 761:143291. doi: 10.1016/j.scitotenv.2020.143291
16. Jiang RW, Mechler MA, Oelbermann M. Softwood biochar and greenhouse gas emissions: a field study over three growing seasons on a temperate agricultural soil. Can J Soil Science (2022) 102:197–211. doi: 10.1139/cjss-2021-0160
17. Jiang RW, Galo M, Oelbermann M. Soybean and soil responses to biochar amendment in controlled environments with elevated temperature and carbon dioxide. Can J Soil Science (2022) 102:65–76. doi: 10.1139/cjss-2020-0133
18. Elkhlifi Z, Iftikhar J, Sarraf M, Ali B, Saleem MH, Ibranshahib I, et al. Potential role of biochar on capturing soil nutrients, carbon sequestration and managing environmental challenges: a review. Sustainability (2023) 15:2527. doi: 10.3390/su15032527
19. Longo M, Dal Ferro N, Izzauralde RC, Furlan L, Chiarini F, Morari F. Deep SOC stock dynamics under contrasting management systems: is the EPIC model ready for carbon farming implementation? Eur J Agron (2023) 145:126771. doi: 10.1016/j.eja.2023.126771
20. Schimel J. Modeling ecosystem-scale carbon dynamics in soil: the microbial dimension. Soil Biol Biochem (2023) 178:108948. doi: 10.1016/j.soilbio.2023.108948
21. Pulcher R, Balugani E, Ventura M, Greggio N, Marazza D. Inclusion of biochar in a c dynamics model based on observations from an 8-year field experiment. Soil (2022) 8:199–211. doi: 10.5194/soil-8-199-2022
22. Dil M, Oelbermann M. Evaluating the long-term effects of nitrogen-enriched biochar on soil organic carbon and nitrogen using the century model. In: Oelbermann M, editor. Sustainable agroecosystems in climate change mitigation. Wageningen, NL: Wageningen Academic Publishers (2014). p. 249–68.
23. Lefebvre D, Williams A, Meersmans J, Kirk GJD, Sohi S, Goglio P, et al. Modeling the potential for soil carbon sequestration using biochar from sugarcane residues in Brazil. Nat Sci Rep (2020) 10:19479. doi: 10.1038/s41598-020-76470-y
24. Miller RO, Kissel DE. Comparison of soil pH methods on soils of north America. Soil Sci Soc America J (2010) 74:310–6. doi: 10.2136/sssaj2008.0047
25. Dyer L, Oelbermann M, Echarte L. Soil carbon dioxide and nitrous oxide emissions during the growing season from temperate maize-soybean intercrops. J Plant Nutr Soil Science (2012) 175:394–400. doi: 10.1002/jpln.201100167
26. Parton WJ, Stewart JWB, Cole CV. Dynamics of c, n, p and s in grassland soils: a model. Biogeochemistry (1988) 5:109–31. doi: 10.1007/BF02180320
27. Metherell AK, Harding LA, Cole CV, Parton WJ. CENTURY soil organic matter model environment. Fort Collins, Colorado: USDA-ARS (1993).
28. Oelbermann M, Echarte L, Marroquin L, Morgan S, Regehr A, Vachon KE, et al. Estimating soil carbon dynamics in intercrop and sole crop agroecosystems using the century model. J Plant Nutr Soil Sci (2017) 180:241–51. doi: 10.1002/jpln.201600578
29. Alves Primo A, Américo de Araújio Neto R, Bozzi Zeferino L, Pavia Fernandes EF, Ambrósio de Araújio Filho J, Pellegrino Cerri CE, et al. Slash and burn management or rotation agroforestry systems: a comparative study for carbon sequestration by century model simulation. J Environ Manag (2023) 336:117594. doi: 10.1016/j.jenvman.2023.117594
30. Jiang RW. The effect of biochar amendment on the health, greenhouse gas emissions and climate change resilience of soil in a temperate agroecosystem. Waterloo, Ontario, Canada: University of Waterloo (2019). Masters Thesis.
31. Oelbermann M, Voroney RP. An evaluation of the century model to predict soil organic carbon: examples from Costa Rica and Canada. Agroforestry Syst (2011) 82:37–50. doi: 10.1007/s10457-010-9351-6
33. Piñeiro G, Perelman S, Guerschman JP, Paruelo JM. How to evaluate models: observed vs. predicted or predicted vs. observed. Ecol Modeling (2008) 216:316–22. doi: 10.1016/j.ecolmodel.2008.05.006
34. Warton DI. Eco-stats data analysis in ecology: from t-tests to multivariate abundances. Heidelberg, Germany: Springer Verlag (2022).
35. Machmuller MB, Kramer MG, Cyle TK, Hill N, Hancock D, Thompson A. Emerging land use practices rapidly increase sol organic matter. Nat Commun (2015) 6:6995. doi: 10.1038/ncomms7995
36. Lugato E, Lavallee JM, Haddix ML, Panagos P, Cotrufo MF. Different climate sensitivity of particulate and mineral-associated soil organic matter. Nat Geosci (2021) 14:295–300. doi: 10.1038/s41561-021-00744-x
37. Janzen HH, van Groenigen KJ, Powlson DS, Schwinghamer T, van Groenigen JW. Photosynthetic limits of carbon sequestration in croplands. Geoderma (2022) 416:115810. doi: 10.1016/j.geoderma.2022.115810
38. Amundson R. Soil biogeochemistry and the global agricultural footprint. Soil Security (2022) 6:100022. doi: 10.1016/j.soisec.2021.100022
39. Krausmann F, Erb KH, Gingrich S, Haberl H, Bondeau A, Gaube V, et al. Global human appropriation of net primary production doubled in the 20th century. Proc Natl Acad Science (2013) 110:10324–9. doi: 10.1073/pnas.1211349110
40. Li C, Zhuang Y, Frolking S, Galloway J, Harris R, Moore B III. Modeling soil organic carbon change in croplands of China. Ecol Applications (2003) 13:327–36. doi: 10.1890/1051-0761(2003)013[0327:MSOCCI]2.0.CO;2
41. Powlson DS, Poulton PR, Glendining MJ, Macdonald AJ, Goulding KWT. Is it possible to attain the same soil organic matter content in arable agricultural soils as under natural vegetation? Outlook Agric (2022) 51:91–104. doi: 10.1177/00307270221082113
42. von Haden AC, Kucharik CJ, Jackson RD, Marín-Spiotta E. Litter quantity, litter chemistry, and soil texture control changes in soil organic carbon fractions under bioenergy cropping systems of the north central U.S. Biogeochemistry (2019) 143:313–26. doi: 10.1007/s10533-019-00564-7
43. Sanderman J, Hengl T, Fiske GJ. Soil carbon debt of 12,000 years of human land use. Proc Natl Acad Science (2017) 114:9575–80. doi: 10.1073/pnas.1706103114
44. Matus FJ. Fine silt and clay content is the main factor defining maximal c and n accumulation in soils: a meta-analysis. Nat Sci Rep (2021) 11:6438. doi: 10.1038/s41598-021-84821-6
45. Gross A, Glaser B. Meta-analysis on how manure applications changes soil organic carbon storage. Nat Sci Rep (2021) 11:5516. doi: 10.1038/s41598-021-82739-7
46. Jenkinson DS, Rayner JH. The turnover of soil organic matter in some of the rothamtsed classical experiments. Soil Science (1977) 123:298–305. doi: 10.1097/00010694-197705000-00005
47. Stockmann U, Adams MA, Crawford JW, Field DJ, Henakaarchchi N, Jenkins M, et al. The knowns, known unknowns and unknowns of sequestration of soil organic carbon. Agric Ecosyst Environ (2013) 164:80–9. doi: 10.1016/j.agee.2012.10.001
48. Craine JM, Morrow C, Fierer N. Microbial nitrogen limitation increases decomposition. Ecology (2007) 88:2105–13. doi: 10.1890/06-1847.1
49. Rumpel C, Chabbi A, Marschner B. Carbon storage and sequestration in subsoil horizons: knowledge, gaps and potentials. In: Lal R, Lorenz K, Hüttl RF, Schneider BU, von Braun J, editors. Heidelberg, Germany: Springer Verlag (2012). p. 445–63.
50. Lorenz K, Lal R. Carbon sequestration in agricultural ecosystems. Heidelberg, Germany: Springer Verlag (2018).
51. Lehmann J, Hansel CM, Kaiser C, Kleber M, Maher K, Manzoni S. Persistence of soil organic carbon caused by functional complexity. Nat Geosci (2020) 13:529–34. doi: 10.1038/s41561-020-0612-3
52. van Groenigen KJ, Six J, Hungate BA, van Kessel C. Element interactions limit soil carbon storage. Proc Natl Acad Sci (2006) 103:6571–4. doi: 10.1073/pnas.0509038103
53. Ibrahim MM, Zhang H, Guo L, Chen Y, Heiling M, Zhou B, et al. Biochar interaction with chemical fertilizer regulates soil organic carbon mineralization and the abundance of key c-cycling-related bacteria in rhizosphere soil. Eur J Soil Biol (2021) 106:103350. doi: 10.1016/j.ejsobi.2021.103350
54. Schwendemann L, Pendall E, Potvin C. Surface soil organic carbon pools, mineralization and CO2 efflux rates under different land-use types in central Panama. In: Tscharntke T, Leuschner C, Zeller M, Guhardja E, Bidin A, editors. The stability of tropical rainforest margins, linking ecological, economic and social constraints of land use and conservation. Berlin, Germany: Springer Verlag (2007). p. 109–31.
55. Lugato E, Berti A, Giardini L. Soil organic carbon (SOC) dynamics with and without residue incorporation in relation to different nitrogen fertilisation rates. Geoderma (2006) 135:315–21. doi: 10.1016/j.geoderma.2006.01.012
56. Singh BP, Cowie AL. Long-term influence of biochar on native organic carbon mineralization in a low-carbon clayey soil. Nat Sci Rep (2014) 4:3687. doi: 10.1038/srep03687
57. Ouyang W, Shan Y, Hao F, Lin C. Differences in soil organic carbon dynamics in paddy fields and dyrlands in northeast China using the CENTURY model. Agric Ecosyst Environment (2014) 194:38–47. doi: 10.1016/j.agee.2014.05.003
58. Carvalho Leite LF, De Sa´Mendonça E, de Almeida Machado PLO, Fernandes Filho EI, Liima Neves JC. Simulating trends in soil organic carbon of an acrisol under no-tillage and disc-plow systems using the century model. Geoderma (2004) 120:283–95. doi: 10.1016/j.geoderma.2003.09.010
59. Cong R., Wang X., Xu M., Ogle , S.M., Parton W.J.. Evaluation of the CENTURY model using long-term fertilization trials under corn-wheat cropping systems in the typical croplands of China. PloS one (2014) 9(4):e95142. doi: 10.1371/journal.pone.0095142
60. Woolf D, Lehmann J, Cowie A, Cayuela ML, Whitman T, Sohi S. Biochar for climate change mitigation: navigating from science to evidence-based policy. In: Lal R, Stewart BA, editors. Soil and climate. Boca Raton, Florida: CRC Press, Taylor and Francis (2021). p. 219–48.
61. Alvarez R. Estimation of carbon losses by cultivation from soils of the Argentina pampa using the century model. Soil Use Manag (2001) 17:62–6. doi: 10.1111/j.1475-2743.2001.tb00010.x
62. Bricklemyer RS, Miller PR, Turk PJ, Paustian K, Keck T, Nielsen GA. Sensitivity of the century model to scalerelated soil texture variability. Soil Sci Soc America J (2007) 3:784–92. doi: 10.2136/sssaj2006.0168
63. Pennock DJ, Frick AJ. The role of field studies in landscape-scale applications of process models: an example of soil redistribution and soil organic carbon modeling using CENTURY. Soil Tillage Res (2001) 58:183–91. doi: 10.1016/S0167-1987(00)00167-7
64. Wang L, O’Connor D, Rinklebe J, Ok YS, Tsang DCW, Shen Z, et al. Biochar aging: mechanisms, physicochemical changes, assessment, and implications for field applications. Environ Sci Technol (2020) 54:14797–814. doi: 10.1021/acs.est.0c04033
Keywords: carbon fractions, carbon sequestration, Century model, organic matter, soil amendments, temperate agriculture
Citation: Oelbermann M, Jiang RW and Mechler MA (2023) Predicting changes in soil organic carbon after a low dosage and one-time addition of biochar blended with manure and nitrogen fertilizer. Front. Soil Sci. 3:1209530. doi: 10.3389/fsoil.2023.1209530
Received: 20 April 2023; Accepted: 29 May 2023;
Published: 20 June 2023.
Edited by:
Muhammad Bilal Khan, Ayub Agriculture Research Institute, PakistanReviewed by:
Rongjun Bian, Nanjing Agricultural University, ChinaMark Radosevich, The University of Tennessee, Knoxville, United States
Copyright © 2023 Oelbermann, Jiang and Mechler. This is an open-access article distributed under the terms of the Creative Commons Attribution License (CC BY). The use, distribution or reproduction in other forums is permitted, provided the original author(s) and the copyright owner(s) are credited and that the original publication in this journal is cited, in accordance with accepted academic practice. No use, distribution or reproduction is permitted which does not comply with these terms.
*Correspondence: Maren Oelbermann, bW9lbGJlcm1hbm5AdXdhdGVybG9vLmNh