- Department of Soil Science, “Luiz de Queiroz” College of Agriculture, University of São Paulo, Piracicaba, São Paulo, Brazil
As Soil Scientists, we are gathering important and valuable knowledge about the chemical, physical and biological processes in soil, and with the increasing effects of climate change, this knowledge may play a pivotal role in the future of our planet. However, we must revisit crucial points in our past to understand how humanity’s evolution has shaped the current state of soil health. Furthermore, we must also consider that we are funded and supported by the society in which we live, and therefore social and political factors will inevitably play a part in the future of soil health. In this review, we address important historical aspects of crop development and soil microbiome combined with the provision of key ecosystem services to ensure soil sustainability. In addition, we provide a brief overview of key concepts related to soil health, including the criteria of the selection of indicators for soil health assessment, whilst focusing on the role of soil biology. Moreover, we provide an overview of research conducted across diverse biomes in Brazil, highlighting approaches to assess soil health in both agroecosystems and natural ecosystems. We also emphasize the significance of harnessing beneficial plant-microorganism interactions as an ecologically sustainable strategy for enhancing soil health. Finally, we conclude the review by discussing potential advancements in soil health assessment in Brazil, and their potential application in broader agricultural and forestry contexts.
1 Introduction
In modern agriculture or forestry, we are revisiting the fundamental principles of these activities, but with an advanced approach, that integrates cutting-edge science and technology. Throughout the evolutionary progression of agricultural management, multiple phases and approaches have been implemented with the aim of optimizing the efficiency and productivity of crop and forest cultivation in order to feed our growing populations and to achieve and cover all human needs. Significant progress was made through the gradual replacement of manual farm labor with wooden and metal tools, and later machinery. Furthermore, the pioneering work of Norman Borlaug (known as the father of the Green Revolution) shed light on the crucial role of industrial fertilizers and pesticides, among other innovations, to increase plant productivity (1). The first responses to this new management technique were very positive, especially in Africa, India, and Latin America, among others, saving millions of people from hunger and death (2). However, after some time, several negative results of these practices were detected, as the disappearance of most biological soil quality due to the intoxication of soil by the use of agrochemicals, or even by chemical fertilizers. Among the unwanted consequences, we can also mention soil, air, and water pollution, causing loss of crop productivity and soil health (SH) (3). For instance, consider SH as a state in which the soil maintains all its original qualities, especially the soil microbiota and the soils initial physical, chemical and biological attributes and production potential (4). The definition of SH shall be explained in detail subsequently in this review, which will be mostly focused on the Brazilian context.
Nowadays, Brazil has developed high-tech agriculture, using hundreds of specialized machineries, including airplanes and drones, all working together over vast areas to cultivate huge amounts of crops. However, this progress has also led to a significant increase in income inequality, with wealth becoming increasingly concentrated. This situation exerts immense pressure on further expansion, endangering our native tropical forests due to continuous deforestation, burning processes, and illegal exportation of precious timber, allegedly driven by the demand for more land for plantations or cattle ranching (5).
There are additional threats to the environment in Brazil, such as the illegal prospecting of gold and precious stones, which results in the pollution of rivers with mercury, for example, used in the extraction process to separate the valuable metal from other materials. As a result, in the Amazon, the world’s largest tropical rainforest, indigenous people and legitimate landowners are being forcefully dislocated from their native lands by miners searching for precious metals and stones (6–8). However, the Amazon rainforest is not the only Brazilian biome at risk of destruction. Deforestation, burning, and loss of biodiversity are also occurring in the swampland of Pantanal, in the Pampa, and the Cerrado and Caatinga regions with semiarid climates (9, 10).
Understanding the real impact of these mentioned points is also essential if we seek eco-friendly strategies in agriculture and forestry that go beyond increasing productivity. It is widely known that the biological aspect of agroecosystems has been overlooked for a significant period, and efforts are currently underway to address this gap. Markedly, there is a large effort of the governance worldwide encouraging the sustainability, food protection and biofortification and organic cultivation based on the use of biological inputs as a new strategy, but in fact, we are just imitating the natural environment (11).
Throughout this review, we revisit the past to obtain a better understanding of what we are doing in the present with our most valuable natural resources, the soil, that could affect our future. In addition, here we cover subjects such as the use of mycorrhizal symbiosis, bacteria inoculation, soil health in agroecosystems and natural ecosystems across different Brazilian biomes, the role of forests in a context of climate change and other key points related to the ecosystem services.
2 Historical development of plantations and soil health in Brazil
Agriculture and forestry initially originated in Europe and Asia, and subsequently expanded to tropical regions (e.g., Brazil) where we have copied certain management practices from temperate regions (particularly Europe) or tropical regions in Asia. Typically, Brazilian tropical soils are less fertile and more prone to erosion due to factors such as excessive tilling, heavy rainfall causing runoff and reduced water infiltration, resulting in substantial loss of soil and nutrients that are carried into rivers and eventually into the ocean. Hence, the implementation of no-tillage management practices in Brazil has been a significant advancement, promoting improved crop productivity, soil structure, and biodiversity over time (12). This technique is called “planting on straw”, to express its collaboration with soil fertility through the increase of soil organic matter (SOM) in soil (Figure 1). However, the first field experiments in this direction taught us, that it would take a long time, of about five years, to attain a greater crop productivity. If we try to modify our methodology from one year to the other, we will lose most of the expected yield, and only after several years of revitalizing the soil to make it healthy again, we may obtain increased yields (14, 19, 20).
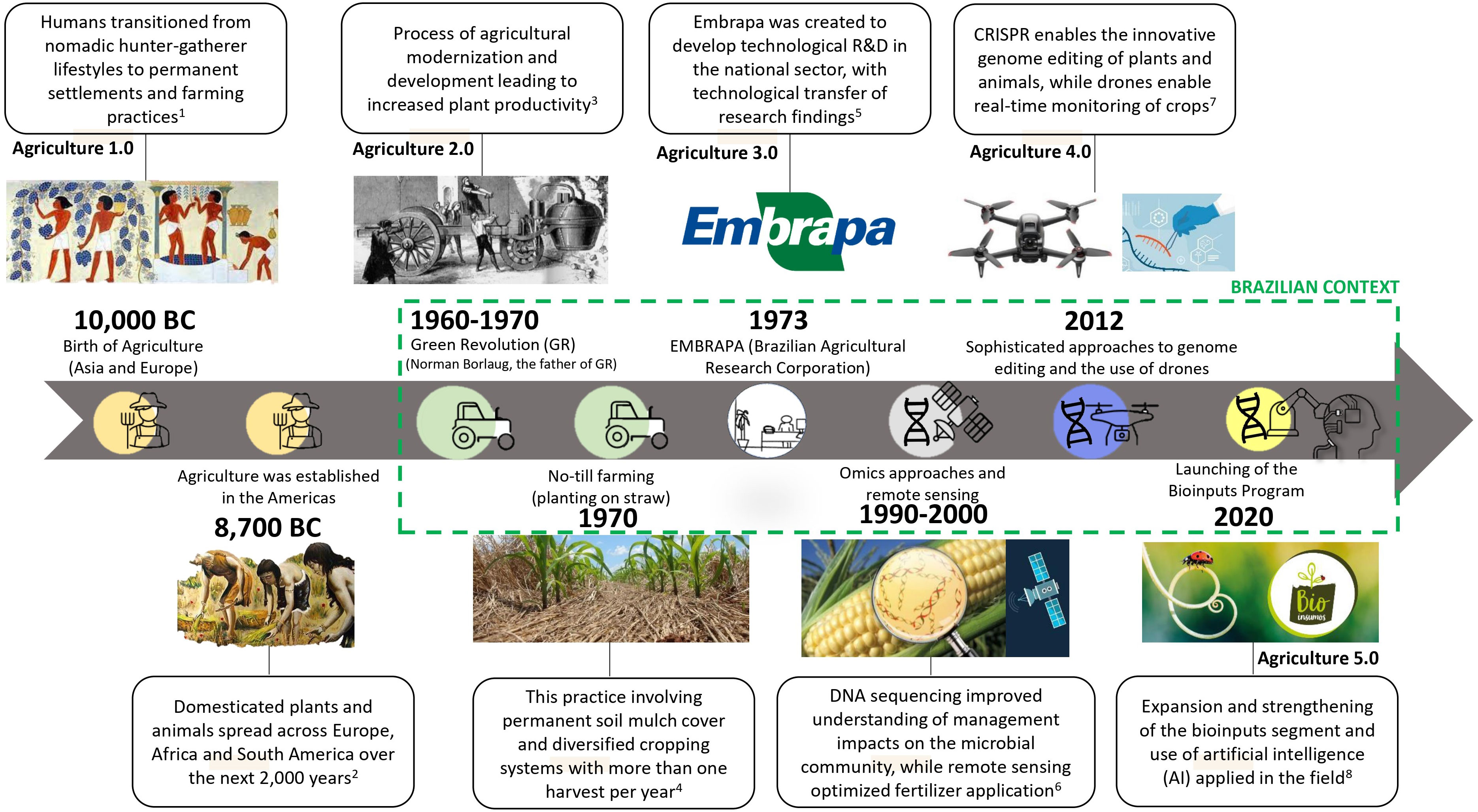
Figure 1 Diagrammatic representation of the timeline events discussed in this review. Information combined of 1Mazoyer and Roudart (11), 2National Geographic (13), 3Briggs (2), 4Possamai et al. (14), 5EMBRAPA (15), 6Schulte et al. (16), 7Ricroch et al. (17), and 8MAPA (18).
Several soil health assessment tools have become available and used since the 1990s in North America, Europe, and China (21). The concept of soil health has evolved as part of a global movement to raise awareness about the crucial role of soil in environmental quality. This has been supported by numerous studies that emphasize the importance of various soil ecosystem services (22, 23). In Brazil, Dra. Elke JBN Cardoso team’s from the University of São Paulo has pioneered in soil health research, highlighting the significance of soil biology for soil health (4) and ecosystem services, particularly in agricultural and forest environments. The study of soil health in Brazil is currently gaining momentum, with the Soil Health & Management Research Group (SOHMA) led by Dr. Maurício Roberto Cherubin also from the University of São Paulo (USP). In addition, the Dra. Ieda Mendes team’s from the Brazilian Agricultural Research Corporation (EMBRAPA) has playing a pivotal role in advancing of this field.
Soil health depends on the different activities that occur in and on the soil and may be understood as being the sum of all characteristics, which allow a soil to have a certain potential to produce well in accordance with its fertility potential, providing food for humans and animals (22). Therefore, SH is an integrated system of management practices that improve environmental quality through efficient utilization resources, thereby enhancing the quality of life for farmers and society, both present and future generations. To achieve its potential, optimal functioning of biogeochemical activities and ecosystem services is essential. In contrast, conventional agriculture, characterized by increased soil degradation and reliance on industrial fertilizers and pesticides, stands in opposition to soil health goals (24–26).
A living and healthy soil shares several characteristics of living beings, for example, soil respiration, which is the result of the summed respiration of all organisms, which harbor in soil. On the other hand, soils are the normal substrate for crop production, and only healthy and fertile soils can produce high yields. Soil health has a tripartite basis, since it comprises the soil’s physical, chemical, and biological properties. Therefore, when evaluating soil health in agroecosystems or forests, it is crucial to assess attributes related to these three aspects. Typically, a minimum set of attributes includes total organic carbon, pH, plant-available nutrients (such as P, K), soil density, penetration resistance (27, 28), visual evaluation of soil structure (VESS) (29), basal respiration, and soil microbial biomass (30). Additionally, enzymatic activity (e.g., β-glucosidase, phosphatases, and arylsulfatase) (31), soil aggregation, and porosity (32) may also be included.
3 Criteria for soil health assessment indicators
Soil health should be understood as a comprehensive principle that cannot be directly measured in the field or laboratory but can be indirectly inferred through indicators related to soil health (21). The multifunctionality and diversity of soil require several indicators to be quantified, but due to cost, collinearity issues, and the complexity of relationships, a minimum set of data needs to be selected (22, 33, 34). Therefore, indicators should fulfil specific criteria, as summarized in Figure 2. These criteria include relevance, sensitivity, validity, feasibility, and informativeness.
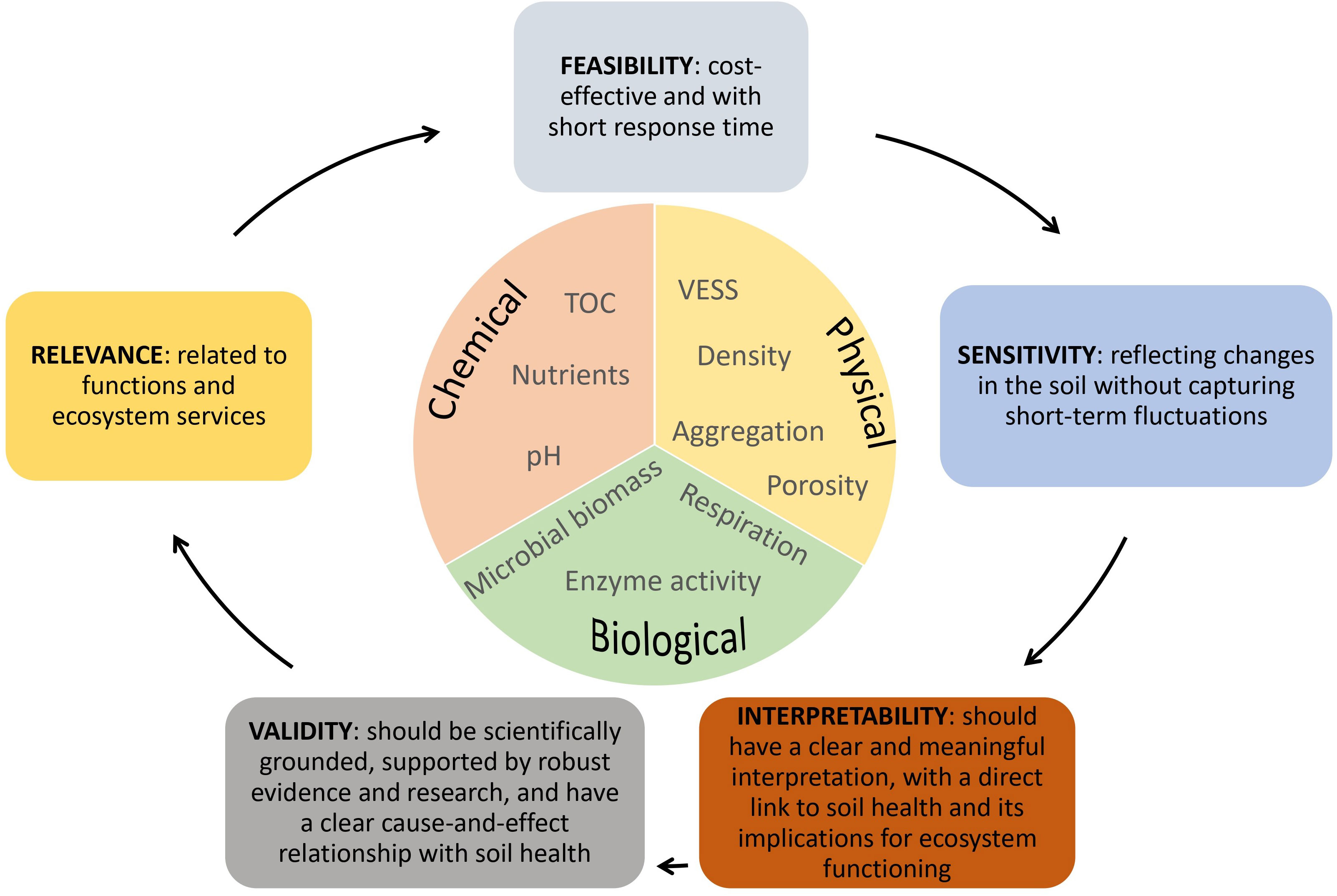
Figure 2 Criteria and examples of commonly used soil indicators for assessing soil health in agroecosystems.
The selection of indicators is aligned with the relationship between the indicators and the target object of study, such as soil threats and ecosystem services. These different sets of indicators are used with different weights in practice. Finally, the integration of indicator scores is performed, generating a final index. This requires the use of statistical and computational methods to relate them to the assessment objective. Acceptable target ranges for soil health indicators are generally specific to the soil and land use (23, 35, 36). Chemical indicators make up at least 40% of the indicators in 90% of soil health assessment schemes, highlighting the continued importance of chemical properties in quantifying soil health and the longstanding emphasis on plant production. Biological indicators still constitute less than 20% of the indicators, even as the total number of indicators used by a given scheme increases, despite their importance for soil health management (37).
4 The role of soil biology in soil health
The cultivated crops or forest trees, being living beings, also possess their own microbiome, similar to the ones that have been described for humans and animals. Those microbiomes live in close association with their living host plants, which become colonized inside and/or outside by their respective microbes, which surpass the number of plant cells. For plants, the most significant part that is colonized by microorganisms is the rhizosphere, and those microbiomes are the most important and necessary factor to define and modulate plant development. The major activity of the rhizosphere organisms consists of the degradation or mineralization of the soil organic material, whereby furnishing the chemical nutrients needed by the plant and modulating the nutrient cycles of macro and micronutrients (38).
The importance of soils rich in organic matter to maintain a productive agriculture is already present in antique descriptions on agriculture by the old romans or tribal ancestral farmers. The utilization of organic materials for agriculture also is very popular among people in developing countries with little technological advance, and in places with low civilizational influence (39). The organic material in soils is the energetic food consumed by the microbial world. Many different microorganisms are also specialized for the symbiotic or associative interaction with plants. Here, we must highlight the ones that are plant growth promoting bacteria (PGPB) and arbuscular mycorrhizal fungi (AMF), exerting many different roles (40, 41). We have provided additional information on the use of PGPB and AMF in agriculture and forestry in sections 9.1 and 9.2 (Figure 3), emphasizing their significance in a broader context. Archaea also play a key role in some biochemical transformations, such as the oxidation of ammonia (NH3) to nitrite () in the soil, although previously we thought this was due exclusively to soil bacteria (42).
Soil macro and mesofauna play a significant role in shaping physical soil attributes, as many of them reside within the soil or in the litter layer. “Soil engineers”, such as earthworms, ants, termites, and certain bugs including coleopterans and their larvae, are among the best indicators of this activity, as they move freely through the soil, build galleries or nests, and can transport large amounts of soil between horizons. Soil fauna can also serve as indicators of soil health stages, as they facilitate seed and microbe movement, consume plants, insects, or microbes, and regulate biodiversity (4).
However, there is a lack of information on how soil biota, which relates to soil functions and ecosystem services, can be managed, and this deserves attention. Disseminating the importance of biological indicators for soil health and developing assessment tools that meet the criteria for soil health indicators, is essential and represents the next frontier in soil health research.
In line with this, soil enzyme activity has emerged as a potential indicator to compose a soil health index in Brazil. Launched by the Brazilian Agricultural Research Corporation in 2019, the soil biological analysis (BioAS) represents an advancement in the relationship between soil biology and soil health in Brazil (43). The BioAS quantifies the activity of arylsulfatase and β-glucosidase enzymes in the soil, which are associated with sulphur and carbon cycles, respectively, and are highly correlated with SOM and grain yield. The BioAS also involves the calculation of Soil Quality Indexes (IQS), which are calculated based on both chemical and biological properties (IQSFertbio). Furthermore, in 2020, the Brazilian government introduced The National Bioinputs Program with the goal of expanding and enhancing the bioinputs sector. This program provides farmers with technologies, products, processes, knowledge, and information related to a wide range of biologically based inputs used in various agricultural practices, including soil nutrition, pest control, post-harvest management, and agroindustry (18).
5 The interaction between ecosystem services and soil health
Ecosystem services are defined as the benefits that humans obtain from ecosystems, such as provisioning services (e.g., food, water), regulating services (e.g., climate regulation, water purification), cultural services (e.g., recreational and aesthetic value), and supporting services (e.g., nutrient cycling, soil formation) (44, 45). The study of soil health encompasses the assessment of various ecosystem services, aiming to accurately represent and balance the multifunctionality of ecosystems. This is achieved through the systematic evaluation of benefits and trade-offs associated with multifunctional systems used for managing ecosystem services, such as those found in agroecosystem soils (46).
The ecosystem services are perhaps the most fundamental attributes for sustainable agriculture. Ecosystem services are a gift of nature, acting through the activity of living beings, which live in all soils or other environments, as long as they preserve their original health. Thus, soil health depends on various types of living beings, which, acting together, provide optimal conditions for plant growth, without any cost to humans (16). As mentioned earlier, ecosystem services are the direct or indirect benefits obtained from natural ecosystems by means of complex improvements offered to agriculture or forestry (45). Those services are furnished without any costs by many living organisms, as bacteria, fungi, soil invertebrates, insects, and many others, although those services would be very expensive, if we had to pay for them. Among those, we can mention SOM decomposition and nutrient cycling by bacteria, soil fauna acting as soil engineers or bees in pollination of flowers, plant microbes helping in plant development, bacteria in biological nitrogen fixation, bioremediation, biological control, nitrification, among many others (45).
Native forests are a prime example of ecosystem services in action, regardless of local climate. Trees in these forests continuously regenerate according to their natural lifespan and death, while the understory thrives without the need for additional inputs like industrial fertilizers. Organic materials from leaf litter and tree debris provide nourishment for soil fauna and microbes, and mixed stands in native forests optimize their fertilization potential. As a result, native forests support the biodiversity of plants, animals, and microbes that interact and create a complex network of interdependence and mutual benefits. Even predators play a crucial role in maintaining population control within their community, contributing to long-term equilibrium (45).
The Amazon Forest is a typical example of an equilibrated ecosystem, containing 10 to 15% of the world’s fauna and plants, despite occupying less than 4.7% of the continental area. The indigenous people who have inhabited the forest for over 12 thousand years serve as its guardians, using their traditional knowledge and ethical practices to sustainably coexist with the environment. The Amazon Forest plays a crucial role in maintaining global biodiversity, regulating water cycles and climate patterns in Brazil and beyond. Given the broad context of the role of soil health and key concepts, we will now focus on soil health in agroecosystems, natural forests and the role of forests in the context of climate change.
6 Soil health in agroecosystems and forestry
The term agroecosystem refers to a complex system introduced into a natural ecosystem, with agricultural production being the main objective. Notably, the international and domestic demand for food, biofuels, and fibres has been increasing and needs to be further met to fulfil the demand (47). Much of this growing demand is expected to be met through intensification of agroecosystem production, particularly in tropical regions (48). However, the intensification of agrochemical use combined with the intensive traffic of agricultural machinery can result in soil degradation and losses of SOM (49). In addition, it can cause soil compaction, increased risk of erosion, reduced soil fertility, higher greenhouse gas emissions, and reduction in soil biodiversity (4). Consequently, these issues impact water quality and use, human and animal health, climate, and biodiversity equilibrium, which are intensified in tropical and subtropical conditions.
Given this context, it is urgent to take action in order to increase sustainable management practices. These practices are generally based on the principles of minimal disturbance of the soil profile (such as no-till and reduced tillage). In addition, it is aimed at increasing plant species diversity through crop rotation, and the maintenance of crop residues on the soil surface, as well as organic fertilization, and the use of biological inputs. Furthermore, in a context of Agriculture 5.0 (Figure 1) the use of precision agriculture has become an interesting tool for farmers, allowing the monitoring of crops (48, 50). Management practices, which increase soil organic carbon, are often emphasized because small changes can have multiple cascading effects on soil health. As soil organic carbon increases, it helps to maintain or improve soil aggregation, reduce water and wind erosion, decrease soil erosion, enhance water retention, thus providing greater resistance to drought. Several other benefits of improved soil health can be mentioned, such as: (i) lower incidence of pests and pathogens, (ii) increased productivity, (iii) economic profit, (iv) water and air quality, (v) increased soil biodiversity, (vi) increased carbon sequestration in soil contributing to food security and climate change mitigation (48, 51–53).
Currently, Brazil is one of the world’s largest producers of agricultural products, with significant advances in crop productivity (206% increase) and grain production (394% increase) over the past 40 years (54). During the 2022/2023 crop season, Brazil is expected to achieve its highest grain production in history, with 312 million tons produced, with a focus on soybean and maize crops, which are projected to reach 153.5 and 125.8 million tons, respectively (55). The evolution of Brazilian agriculture has been driven mainly by the introduction of improved cultivars through genetic breeding, conservationist soil management, mechanization, public agricultural financing, and investment in technologies (56). The adoption of conservationist systems has been established in cultivated areas worldwide, increasing by 70% from 2009 to 2016. In Brazil, conservationist agricultural systems cover over 32 million hectares out of the 60 million hectares of cultivated land (52). This transition to conservationist management systems has occurred gradually over decades, mainly due to the evolution of management practices for grain production (57).
On the other hand, the intensification of land use without adequate knowledge has direct implications on the physical, chemical, and biological properties of the soil. As a result, in order to prevent negative consequences resulting from inadequate management, soil health assessment has been developed to provide information on the agronomic and environmental impacts of different agricultural systems (22, 23, 27, 52, 58, 59).
Among the various tools available in the literature, the Soil Management Assessment Framework (SMAF) (60) stands out as a precise, sensitive, and dynamic tool for assessing soil changes induced by different land uses and management practices (60, 61). The SMAF was originally developed and applied predominantly in North American soils. In Brazil, Cherubin et al. (62) introduced the SMAF for assessing the impacts of land use changes (native vegetation - pasture - sugarcane). Since then, the use of SMAF has been spreading in Brazil with the aim of evaluating the effects of different land uses, management practices, changes in land use, and crop systems (agricultural, pasture, planted forests) on soil health (28, 47, 63, 64).
Recently, visual assessments have been developed to provide a simple evaluation of soil structural quality directly in the field (65, 66). Among these, the Visual Evaluation of Soil Structure (VESS), developed by Ball et al. (67) and refined by Guimarães et al. (68), is a quick and accessible process that can be easily carried out in the field. The VESS scores provide an initial assessment of the overall state of soil health (35). In Brazil, several studies have applied the VESS assessment (29, 61, 69, 70).
Da Luz et al. (27) used SMAF as a strategy to assess the effects of different land uses on soil health in southern Brazil. They found that the conversion of native vegetation areas into long-term agricultural lands reduced soil health. However, conservationist systems such as no-till farming, with or without integrated crop-livestock systems, showed promising alternatives to improve soil health compared to degraded pastures or conventional sugarcane cultivation. Consistent with these results, Cherubin et al. (61) observed that 20 years of grain cultivation (maize, soybean, and oat) reduced soil functioning capacity by 64%, while native vegetation operated at 80% of its capacity.
The expansion of crops into degraded pastures is considered a sustainable strategy to expand sugarcane cultivation in Brazil (62). However, studies indicate that well-managed pastures through liming and nutrient management, high-yielding forage grasses, and weed control (71) can increase soil health compared to sugarcane cultivation. Additionally, land use for grain production under no-till farming, combined with integrated crop-livestock systems in Arenosols, contributes to improved soil health, reaching a functioning capacity of 56% of its potential capacity (27).
Currently, Brazil stands out as the world’s largest producer of sugarcane, accounting for 40.5% of global production (72). Compaction from mechanized operations is considered the main cause of reduction in soil capacity to sustain its essential physical functions (73). Da Luz et al. (28) found that uncontrolled traffic increased soil density, penetration resistance, reduced total porosity in the surface layer, and increased scores in the VESS assessment in sugarcane cultivation. On the other hand, controlled traffic in sugarcane cultivation was considered an efficient strategy to reduce physical soil degradation. Aligned with these results, Santos et al. (74) observed that machine traffic for crop management induced soil profile compaction in the MATOPIBA region of Brazil. Additionally, they found that the physical quality index of the soil in the studied sites was sensitive to the impacts of land use changes, leading them to function below their potential.
The increasing demand for bioenergy worldwide has contributed to the intensification of sugarcane planting due to its potential for biofuel production (47). Brazil has established itself in recent years as a leader in the development of sustainable strategies for utilizing sugarcane residues due to the large availability of straw resulting from the transition to mechanized harvesting without burning (75). Recent studies have indicated negative effects on soil functions due to indiscriminate removal of this straw (76, 77). Cherubin et al. (47) observed in Brazilian tropical conditions that sandy soils are more susceptible to soil degradation than clayey soils, indicating that straw removal should be avoided in this type of soil. According to SMAF, sandy and clayey soils functioned at 41–56% and 67–86% of their total potential, respectively. Meanwhile, low to moderate removal rates resulted in minimal detrimental effects on soil health.
Soil health assessment has been applied in various fields that utilize soil science as a research subject, such as agriculture, forestry, and others (35, 47, 78). In the forestry sector, there has been a growing need to assess the impact of land use on soil health and forest productivity in order to ensure environmental and economic sustainability, given the expansion of planted forests in Brazil. However, to date, few studies have assessed soil health in planted forests.
The removal of residues from forest plantations for use as an energy source is another point to be studied. In this regard, Eucalyptus cultivation stands out due to the large amount of residues generated during harvesting. In Brazil, the removal of forest residues for energy generation is going against the established practice of minimum tillage, a well-established conservationist practice in many regions of the country (79). Rocha et al. (80) found that the removal of forest residues reduced the production of Eucalyptus grandis by 40%. In a second rotation of E. grandis with consecutive residue removal (2004–2012), Rocha (81) observed a 15% reduction in wood volume at 4.5 years of tree age. In this sense, in addition to productivity, the levels and quality of SOM are significantly reduced when total residue removal occurs (80, 81).
This fact is concerning, especially in tropical soil conditions where SOM plays a fundamental role in soil health (81). The removal of residues can affect several indicators used to measure soil health. In this context, São José et al. (82) evaluated the impact of different residue management practices in eucalyptus forests. It was observed that the highest values of soil health were obtained in treatments where all residues (bark, branches, leaves, and litter) or partial residues (branches, leaves, and litter) from the previous rotation remained on the soil. These authors argue that eucalyptus productivity is dependent on soil health, and the main drivers of its reduction are associated with SOM depletion and the reduction of soil biological activity. They highlight the importance of this tool for monitoring the quality and sustainability of eucalyptus production.
Negative effects on soil properties were observed when E. grandis grew under a monocropping system (79, 83). However, when intercropped with nitrogen-fixing plant species, E. grandis has less impact on the soil, as these nitrogen-fixing species mitigate the negative effects (83, 84). In particular, Acacia mangium has been proposed as a suitable nitrogen-fixing plant species to be intercropped with E. grandis (85, 86). Therefore, intercropping E. grandis with A. mangium plantations has the potential to improve soil health in Brazilian tropical soils. Recently, Pereira et al. (31) applied the SMAF to assess soil health under monocropping (E. grandis: E or A. mangium: A) and intercropping forest (E. grandis + A. mangium: E+A) systems. Interestingly, soil health scores were significantly higher in A and E+A, with values above 0.9. However, the authors mentioned that only chemical indicators showed significant differences in soil health scores, while no changes were detected in physical and biological soil health scores among the treatments.
Adopting Agroforestry Systems (AFS) is an efficient and low-cost strategy to reverse soil degradation (87), while aiding in the maintenance and enhancement of soil health. This is achieved through increased carbon sequestration in vegetation and soil, restoration of nutrient cycling, among other ecological and socio-economic benefits (88). Matos et al. (89) evaluated the implementation of AFS on areas previously occupied by extensive grazing in Brazil. They found that AFS restore soil health in surface layers, particularly improving chemical soil components (12.79%) and physical soil components (6.5%), thereby enhancing the provision of key ecological functions of the soil.
Publications mentioning the terms soil quality and/or soil health, according to Simon et al. (90), have substantially increased in the last seven years. However, less than 10% of studies have focused on comprehensive evaluation of soil health, including integrated assessment of chemical, physical, and biological soil indicators. These authors also highlighted existing gaps for advancing scientific study of soil health in Brazil, such as new concepts, assessment frameworks, sampling and data analysis methods, interpretation curves, on-farm monitoring protocols, dissemination of assessment among Brazilian farmers, environmentalists, and other stakeholders. Continuing to advance and invest in research on soil health in Brazil and worldwide is essential, considering that maintaining healthy soils is crucial for achieving the United Nations’ Sustainable Development Goals in the coming decades (37, 91).
7 Soil health in native forests: a brief view across Brazilian biomes
Various global forest biomes provide a wide diversity of ecosystem services. Therefore, understanding their relevance and degradation mechanisms is important for making sustainable decisions aimed at the maintenance and preservation of these ecosystem services offered by biomes. In this way, we explore the Brazilian biomes with the objective of highlighting their potential for maintaining ecosystem services and raising awareness of the extensive degradation they have experienced in recent years. This is a topic of utmost importance in order to advance soil health studies in these biomes and develop focused projects for restoration and preservation.
Brazil has its territory occupied by six biomes (Amazon, Atlantic Forest, Cerrado, Caatinga, Pantanal and Pampa). The Amazon is the largest biome in Brazil, occupying 4,196,943 km2, which corresponds to 49.3% of the Brazilian territory. Geographically, six complete Brazilian states and most of the states of Mato Grosso and eastern Maranhão comprise the Amazon biome. This biome encompasses the Amazon rainforest, considered the largest tropical forest in the world, and it equally occupies large areas of other South American countries, such as Bolivia, Colombia, Ecuador, French Guiana, Guyana, Peru, Suriname and Venezuela. However, the Brazilian part no doubt is the largest. Considering all the above countries, the Amazon biome covers an area of 6,700,000 km2, and consequently, its ecological role in maintaining vital ecosystem services is well recognized worldwide (92, 93).
For example, in terms of ecological restoration in Brazil in the last 30 years, Guerra et al. (94) revealed that the Atlantic Forest and the Amazon are the Brazilian biomes with the highest number of studies, representing 56% and 22%, respectively. In other words, 78% of scientific efforts are involved in finding strategies to mitigate or measure the deforestation process in the Amazon rather than increase the capacity of this biome to provide ecosystem services. Botelho et al. (95) demonstrated that extensive areas of the Amazon rainforest are crossed by rural ways, which separate relatively small patches of forest vegetation. The whole extension of those roads corresponds to nine times the distance between our planet and the moon. Furthermore, the losses of the Amazon rainforest are linked altogether to the loss of indigenous land rights and violations of human rights, and therefore, to take care of this problem is a holistic approach involving scientific, political, economic, social and cultural sectors (96).
Although the Amazon is known for its great biodiversity, which includes vegetation and habitat, most soils in this biome are dystrophic, that is, they present low base saturation and often are high in aluminum contents and the pH is acidic (97). This increases its fragility for intensive management systems based on high nutrient exports from the soil, such as extensive cattle ranching (livestock), which is considered one of the culprits of deforestation, as well as agricultural monocultures based on slash-and-burn that rely on synthetic fertilizers to achieve high yields (98, 99).
Despite criticism of the economic pricing of ecosystem services (100), some efforts in the Amazon rainforest have been made to compensate and encourage landowners to improve land management practices for the maintenance and provision of ecosystem services (101, 102). However, a myriad of bottlenecks are related to how to assess soil health and how to price it.
Briefly, we explain some of these bottlenecks. First, it is necessary to assess the soil health based on its chemical, physical and biological characteristics. However, there is a plethora of these variables within each of these categories, and it is not feasible to consider them all due to the high costs. Therefore, the first step is to select a minimum dataset that will then be integrated and interpreted into an overall soil health index (Figure 2) (4, 60, 90, 103). Second, soil physical and chemical characteristics have been widely studied, but the soil biological traits are still ongoing, and the ecosystem services provided by the soil microbiota to human well-being in its different ecological niches has to be taken into account (104–106). Third, it is necessary to consider the land use change based on the best management practices, such as forest-pasture and forest-cropland, which may change geographically across this biome (107–110). Fourth, recent fires, environmental degradation combined with a changing climate, as well as illegal gold mining in indigenous territories which has spiked during recent decades in the Brazilian Amazon, have a tremendous ecological and economic impact (111, 112). Fifth, the instability of national and world prices, especially due to the context of Brazilian policies that promote the destruction of the Amazon rainforest and other biomes (113, 114).
Compared to the other Brazilian biomes, Pampa and Pantanal are the smallest, occupying together 326,851 km2, which corresponds to 3.9% of the Brazilian territory. The Pampa biome is located in the State of Rio Grande do Sul (southern Brazil), but also comprises Uruguay and parts of Argentina. Whilst most parts of the Pantanal are located in the States of Mato Grosso and Mato Grosso do Sul (central-western Brazil), transpassing the borders to Bolivia and Paraguay. The vegetation of these biomes is different from one another, as Pampa presents natural grassland vegetation dominated by grassy prairie and grass steppe in lowland plains. Since Pantanal exhibits a mixture of vegetation from the Amazon, Cerrado, and Atlantic Forest biomes in wetland plains characterized by their seasonal flooding cycle and monomodal hydrological signature (93, 115, 116).
Among all biomes, Pantanal, Pampa and Caatinga are the least studied in terms of ecological restoration (94). The Pantanal was recognized as the most preserved biome with almost 80% of the native vegetation well conserved, while in the Pampa only 40% is still covered by the original vegetation (116–119). However, in 2020, this area was impacted by fires by the global changing climate, cattle ranching intensification, and poor or unknown information about the soil health status, which could be decisive in its total destruction (120, 121). According to Lovejoy and Nobre (122), this scenario is related to increased deforestation and widespread use of fire in the Amazon combined with changes in the hydrological cycle. It is a unique type of vegetation in the whole world. However, it is already in danger of disappearing, together with its also unique biodiversity. Besides the anthropogenic fires, another problem is global heating, which causes longer periods of drought, thus impeding the repetitive yearly inundations to which it is adapted. A further problem, very specific to Pantanal soils, is that most of the time the uppermost soil level is sandy, and, below it, there is a burrowed straw-rich level, which stems from fallen vegetation, and remains dry for a long period. This subterranean straw is often responsible for the fire to move below the surface, and returns as superficial fires at a distance. Thus, the person who just finished extinguishing the visible flames will have to start all over again. If he does not perceive the new flames at a distance, it may be the beginning of another great fire.
As in the Amazon and other biomes, the Pampa has suffered severe fragmentation due to its widespread use for livestock production, reflecting effects on soil health indicators, such as microbial diversity, carbon and nitrogen stocks (123), and soil structure and aggregates (124). Thus, visible efforts were necessary to find a set of in-field indicators capable of monitoring changes in soil biological functioning that can be used to establish agricultural policies and design new strategies of cropping system management (125). In addition, an in-laboratory indicator (in this case, permanganate oxidizable carbon) was proposed as feasible and achievable to indicate soil health status in soils of the Pampa biome (126). However, it is important to consider that the selection of these indicators should use an integrated approach, rather than simple correlation metrics, as we know that correlation implies association, but not causation (127).
8 The role of forests in the context of rising effects of climate change
Forests cover about a third (4.06 billion hectares) of the Earth’s surface (128) and play an essential role in maintaining the Earth’s climate system, such as the maintenance of atmospheric moisture, haze and cloud water capture, groundwater infiltration and recharge, moisture transport, and precipitation patterns through evapotranspiration. Therefore, maintaining Earth surface albedo, local and global temperatures, biodiversity, and carbon storage (129–131). In fact, forests, overall, play a very important and fundamental role in capturing atmospheric carbon through photosynthesis, incorporating the carbon during growth, while maintaining it in the tree’s structure, as long as the forest is growing, recomposing the lost tree parts of the forest, always on healthy soils. However, deforestation and forest degradation continue to proceed at alarming levels and this impacts the maintenance of several forest functions, as previously mentioned (132), directly influencing the provision of ecosystem services (133–135).
From 1990 to 2020, it was possible to estimate that more than 420 million hectares of forest were lost globally due to deforestation (128), with agriculture being the main culprit. Altogether, between 1992 and 2014, a total of 33.7 million hectares were degraded and 30.8 million hectares were deforested in Brazil (136). In the Amazon, which represents approximately 92% of the forests in the Brazilian territory, 788,353 km2 were lost due to deforestation between 1975 and 2018. While the period from 2019 to 2022 registered new deforestation records in the Amazon, with a loss of 45,586 km2, equivalent to 5.8% of the total area deforested over a period of 43 years (137).
Among the main consequences of deforestation and forest degradation, reducing resilience to climate change has to be shown as a topic of great importance in the current environmental scenario (138, 139). The reduction of forests reflects significant increases in the release of stored carbon, in addition to preventing plants from absorbing more carbon dioxide (CO2) from the atmosphere (140). According to the same author, since 1850, about 30% of all CO2 emissions originate from deforestation. Furthermore, studies indicate that climate change is expected to increase temperatures across the tropics, with variability in rainfall and more extreme events such as intense storms, droughts, and wildfires (141, 142) on local to regional scales (143, 144). Healthy forests thrive on healthy soils, where the soil structure is preserved, and where the soil is rich in carbon and nitrogen, besides a pulsating diversity of soil fauna and microorganisms, with the incidence of all types of ecosystem services. It is crucial to highlight that, despite the presented evidence, evaluations of soil health with the objective of measuring the effects of greenhouse gas emissions and soil carbon sequestration on climate have been neglected (37).
Climate change projections reveal that carbon sequestration is one of the most critical ecosystem services for mitigating global climate change (145). Within this context, we assume that carbon storage by forests contributes significantly to reducing the rates of CO2 emitted into the atmosphere by anthropogenic activities through the photosynthetic action of the trees. The forest can capture CO2, convert it into living biomass (leaves, branches, trunks, roots), and later store it in the soil through the deposition of these materials and root exudates. In 2011, using estimates of carbon stocks and fluxes, this carbon stock was evaluated as being equivalent to 861 ± 66 Gt of carbon, with 42% distributed in live biomass, 8% in dead wood, 5% in litter, and 44% in soil. The carbon storage in forests differs between them, as follows: 55% in tropical forests, 32% in boreal forests, and 14% in temperate forests (146). Each year, since 2000, it has been estimated that forests have removed an average of 2 billion tons of carbon from the atmosphere (147).
Tropical forests are among the most diverse and productive biomes that store large amounts of carbon (148, 149). Despite this importance, tropical forests have been rapidly degraded by anthropogenic action, mainly through land use changes (150, 151). A global estimate of the impact of land use changes revealed that 133 million tons of carbon from soils were transferred to the atmosphere, due to historical human activity, with Brazil being among the ten largest emitters of CO2 in the world (152).
Brazil is one of the top five largest CO2-emitting nations in the world. The primary sources of emissions are land use change and agriculture (153). Nowadays, in Brazil, many highly deforested and burned forest areas reverted their action as carbon sinks into areas of strong CO2 emissions. The high values of such CO2 is attributed to the advance in deforestation in Brazilian forest ecosystems, especially in the Amazon and Atlantic Forest biomes, which have high levels of carbon stocks (154). According to Pearson et al. (155), deforestation and forest degradation are responsible for the second largest anthropogenic source of carbon compounds in the atmosphere, corresponding to approximately 6-25% of global emissions.
Due to the high productivity of tropical forests, initiatives aimed at restoring degraded areas play an essential role in carbon sequestration and consequently mitigation of climate change. Such as afforestation (planting forests where they did not exist before or where they have been absent for 50 years or more) and reforestation (planting trees where forests were recently deforested), and creation of protected areas are fundamental in reverting the present trend (156, 157). Another important initiative was made by the United Nations, which designated the 2020 as The Decade for Ecosystem Restoration (https://www.decadeonrestoration.org/), aiming at reverting the degradation process through different restoration strategies, such as assisted and natural regeneration and agroforestry.
Natural forest regeneration is an efficient and low-cost strategy to increase carbon sequestration (158) and increase biodiversity (159). If done worldwide, natural regeneration could capture up to 70 billion tons of carbon in plants and soil by 2050, equivalent to about seven years of current industrial emissions (160). Capellesso et al. (161) found that the natural regeneration of the Atlantic Forest contributed to increased carbon accumulation and recovery of biodiversity up to about 80 years, with the carbon stock being similar to that observed in older forests (162), and higher than the values found in the Amazon forests (163). In the Amazon, there is much uncertainty regarding carbon sequestration in secondary forests (164) due to low permanence in landscapes (165). Therefore, it is necessary to encourage protection measures and management of secondary forests for large-scale carbon recovery.
Therefore, the conservation and sustainable management of forests within an integrated landscape approach is fundamental for the conservation of the world’s climate, biodiversity, food security, and the well-being of the world population. Furthermore, the role of carbon sequestration in forests to help counteracting global climate heating highlights the importance of public policies.
9 Future advances in soil health assessment in Brazil
The use of new soil health indicators may be interesting if they provide clear information related to the objective of assessing soil health. However, most of these indicators are still in the research and validation stage, and many technological, interpretative, and practical issues need to be overcome. In general, we can observe that advances in soil health science are occurring in three main areas: soil biology, soil data interpretation, and spectroscopy.
One of the key steps to improve our understanding of the soil microbial community and the soil health processes they mediate will be through enhanced microbial genomics and other molecular techniques (166). It is anticipated that these new tools and rapid developments will dramatically increase our understanding of an area of soil science that currently has many unknowns. Consequently, they may produce new indicators that can replace or complement biological indicators of soil health (167). Advances in knowledge about soil biology will improve agricultural sustainability and productivity and help us to overcome the trajectory of global soil degradation in agriculture (21, 168).
With recent investments in soil health databases and developments in data analysis techniques, there is a prospect of improvements in soil health indices such as SMAF (Soil Management Assessment Framework) and CASH (Comprehensive Assessment of Soil Health). These changes are likely to involve the expansion and addition of new classes of indicators, such as soil profile information, inclusion of sensitivity analysis, information obtained from molecular biology methods, and addition of simple visual assessment methods. It is anticipated that CASH and SMAF will continue to evolve as tools for summarizing physical, chemical, and biological soil indicator data in a way that is more useful and helps farmers better understand their most limiting factors. This will facilitate better soil and crop management and result in more sustainable agricultural production systems and reduced soil degradation (34).
A third area where significant advances in soil assessment can be achieved is through spectroscopic techniques (169). For example, near-infrared spectroscopy and remote sensing, measuring various chemical, physical, and biological parameters of the soil in a rapid and cost-effective in situ manner (170, 171). Reflectance spectroscopy has been successfully used to estimate soil health indicators such as organic carbon, total nitrogen, β-glucosidase activity, microbial biomass carbon, particulate organic matter, and soil respiration (172–175). If these data can be obtained at high spatial and temporal resolution, rather than selected sampling locations, soil health indicators can be assessed, reducing the need for expensive laboratory tests. Recently, it was created an online Brazilian Soil Spectral Service (BraSpecS http://besbbr.com.br), which can be used to predict soil attributes, such as soil texture, soil organic carbon content, and many other soil properties (176). This effort may represent a step forward in the soil health studies in the Brazilian context.
As new advancements continue to emerge in this field, combined with the growing bio-based program input in Brazil, new opportunities arise to incorporate important soil microbial groups, such as mycorrhizae and bacteria, in these assessments. In the following section, we provide more information about these aforementioned groups.
9.1 Arbuscular mycorrhizal fungi
More than 90% of all plants interact with arbuscular mycorrhizal fungi (AMF) and many of them show great improvements in growth and productivity. The dependency that plants have on AMF is called “Mycorrhizal dependency”, which is variable from plant to plant, and from species to species. Some of them barely survive without mycorrhizae, whereas several other plant species are benefitted strongly or only a little. As mentioned earlier, the majority of botanical families form associations with AMF, with only a few exceptions. On the other hand, many forest trees, especially those that are native to Europe and other colder regions of the world, establish symbiotic relationships with ectomycorrhizal fungi (EMF) (177).This is mostly the case of conifers, which occupy enormous extensions in the northern hemisphere, while most of the tropical trees are associated with AMF. The AMF may constitute between 5% and 50% of the soil biomass, being the most abundant fungi in soils. Their hyphae can grow up to 50 or 60 m per gram of soil (178). The AMF have a strong effect on the absorption of nutrients of low mobility in the soil, as P, Zn and Cu. N, when in the form of nitrate (), is very soluble and moves by mass flow, while it moves much slower when in the form of ammoniac. In that case, it is absorbed much more rapidly in mycorrhizal plants than in non-mycorrhizal plants (179, 180).
As P is a non-renewable nutrient, it is very important to seek non-waste and reuse strategies. Therefore, let us look at the association between the rhizosphere and P, where we find many bacteria and fungi that solubilize phosphates. However, soluble P ions have limited mobility and can only penetrate the roots when they are in close proximity to the root surface. In contrast, mycorrhizal hyphae, which extend much farther than root hairs, are capable of accessing P ions that are further away, transporting them into the roots and preventing the formation of a narrow zone around the roots where P has already been absorbed by the plant, known as the P depletion zone (181, 182). Mycorrhizae play a crucial role in nutrient absorption, contributing to the uptake of up to 80% of P, 60% of Cu, 25% of N and Zn (Figure 3) (177). In specific studies, it was found that soybeans responded positively to inoculation with Glomus, but not with Gigaspora, indicating a higher functional compatibility with the former as a symbiotic partner (183).
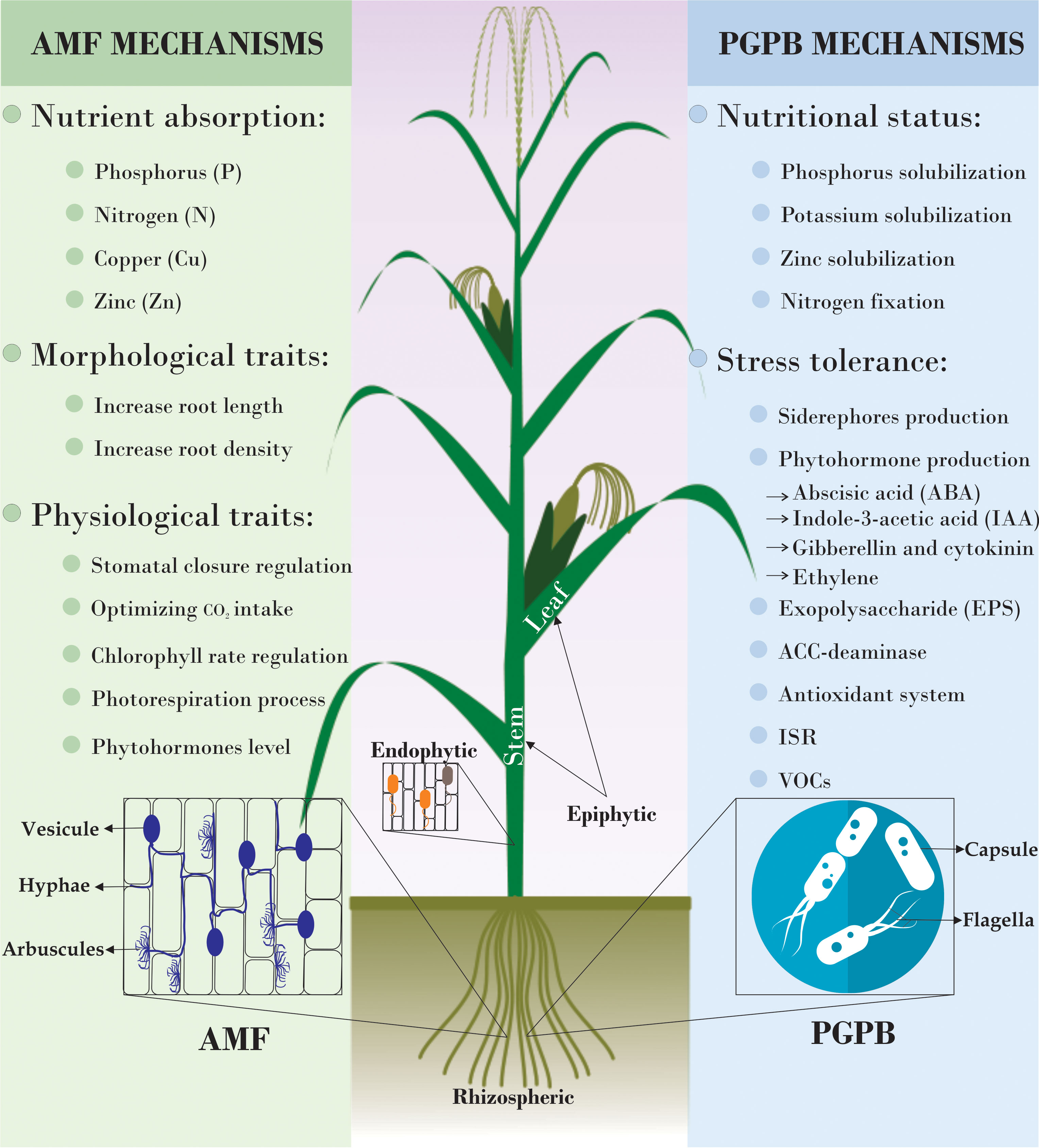
Figure 3 The main mechanisms underlying the beneficial effects of arbuscular mycorrhizal fungi (AMF) and plant growth-promoting bacteria (PGPB) on plant development. ACC-deaminase, 1-aminocyclopropane-1-carboxylate deaminase; ISR, induced systemic resistance; VOCs, volatile organic compounds.
In degraded and infertile tropical soils, it is very important to provide the presence of AMF. Thus, when preparing seedlings of native trees to recuperate degraded areas we should inoculate them with AMF, because they will be able to absorb much more water and nutrients and, quite often, to protect the plants against pathogens and heavy metals, and diminishing aluminum toxicity (183, 184). AMF are very important as carriers of P from soil to plants, but they do not obtain more P from the exterior, i.e., they do not act as solubilizer, to provide more nutrients to plants, as do the biological nitrogen fixing bacteria, for example. Therefore, it is necessary to have a conglomerate with fungi and bacteria. The bacteria solubilize the phosphates and mycorrhizal hyphae take in the soluble ions (Pi) from the soil and carry them into the plants. In the hyphae, P ions form polyphosphates, which reach the fungal arbuscules, where it is exchanged for plant sugars, delivering P to the plant, since these sugars are the energy used by the fungi (185).
Combining different types of mycorrhizae with other microbes (e.g., bacteria) and plant species is also an interesting strategy for a sustainable forest management. For example, if we are planting Eucalyptus seedlings, it is much easier to prepare them inoculated and in consortium with nitrogen-fixing leguminous trees, since they grow much better and obtain their N from the mixed Acacia mangium (31, 86). Furthermore, recent research by Silva et al. (41) has demonstrated that the co-inoculation of AMF with specific bacterial strains has the potential to mitigate the negative effects of drought stress, whilst improve the P uptake by maize plants.
9.2 Plant growth-promoting bacteria
Plant growth-promoting bacteria (PGPB) boost plant development through a variety of mechanisms and interactions with other beneficial microorganisms such as fungi, nematodes, and viruses (186, 187). PGPB can be found in different parts of the plant, including the roots (rhizospheric), leaves and stem (epiphytic), and even within the plant for most of their life cycle (endophytic), establishing different types of associations such as symbiotic, associative, and free interactions (188, 189) (Figure 3).
These interactions can have both direct and indirect mechanisms, with some mechanisms falling into both categories. Briefly, direct mechanism include nutrient solubilization (e.g., phosphorus, potassium and zinc), siderophores production, nitrogen fixation, the production of phytohormones (e.g., abscisic acid (ABA), indole-3-acetic acid (IAA), and ethylene). Indirect mechanisms include exopolysaccharide (EPS) production, the production of ACC-deaminase and volatile organic compounds (VOCs), induced systemic resistance, siderophores production, and activation of the antioxidant system (enzymatic and non-enzymatic) (186).
The beneficial use of PGPB in Brazilian agriculture is noteworthy, demonstrating that these aforementioned mechanisms are responsible for enhancing plant development through improved nutrition, increased tolerance to abiotic stresses such as drought, salinity, acidity, and heat, as well as biotic stresses such as pests and phytopathogens. For example, when maize is inoculated with PGPB strains screened from the Caatinga Biome, it has been observed that these bacteria can mitigate the effects of drought stress and promote biomass growth, with their performance varying depending on soil moisture content (40). Furthermore, the authors found that inoculation with Streptomyces sp. had a positive impact on maize growth under moderate drought conditions, while Arthrobacter sp. had a negative effect on maize plants subjected to a drying-wetting cycle in the soil. Likewise, Santos et al. (190), working with cacti-associated PGPB from Brazilian Caatinga biome, found that the Bacillus sp. strains were able to increase the shoot dry weight of maize plants under water deficit.
Furthermore, the use of PGPB specialized in solubilizing phosphorus, also known as phosphate solubilizing bacteria (PSB), was able to increase sugarcane productivity by 10 Mg ha-1 in Brazilian field conditions, allowing the substitution of fertilizer mineral phosphate by organic or organomineral fertilizer, followed by inoculation with PSB (191). However, the most widely recognized application of PGPB in Brazil is the symbiotic association between soybean and Rhizobium strains, with 50 to 60% of the N required by soybeans provided by the symbiotic association with the bacteria in a process called biological nitrogen fixation (BNF) (192). Indeed, this is validated with data showing that currently 85% of the soybean cultivation area in Brazil is inoculated with these beneficial bacteria (193). In addition, inoculants based on Azospirillum brasilense strains have been increasingly used over the last 13 years to increase plant growth and yield due to improved nutrient uptake and nitrogen fixation, resulting in up to 25% reduction in N-fertilizer usage (194–196). Furthermore, co-inoculation of A. brasilense with rhizobia has been shown to promote growth parameters in soybean and common bean (197).
10 Conclusion
As presented throughout this review, soil health encompasses the chemical, physical, and biological components of soil. Therefore, it is not an exclusive approach advocating the use of only one of them, but rather making efforts to combine them. With the emergence of technology as a key tool, it is necessary to combine it with ancient soil management knowledge and recent research in order to identify the best set of management practices. Currently, the most recommended practices for promoting soil health in agriculture include the use of conservative agricultural practices such as balanced fertilization, crop rotation, organic matter management, integrated pest and disease management, and regular soil monitoring. These practices are designed to protect soil from erosion, enhance its quality, preserve its structure, increase its water and nutrient retention capacity, promote microbial biodiversity, and reduce reliance on chemical pesticides. In the future, farmers will have more tools at their disposal to assess the health of their soil, but this will require government investment in cutting-edge science projects aligned with sustainability goals.
Author contributions
AS, VA, and EC: drafting the work or revising it critically for important intellectual content. AS: proof-reading of the manuscript. VA: proof-reading of the manuscript. EC: final approval of the version to be published. All authors contributed to the article and approved the submitted version.
Funding
This work was funded by the Fundação de Estudos Agrários Luiz de Queiroz (FEALQ), São Paulo Research Foundation (FAPESP, #2016/18944-3, #2019/13436-8, and #2020/12751-4) and by the Coordenação de Aperfeiçoamento de Pessoal de Nível Superior - Brasil (CAPES) - Finance Code 001, whose support is gratefully acknowledged.
Acknowledgments
Our gratitude to the Soil Microbiology team of the Soil Science Department of the University of São Paulo, “Luiz de Queiroz” College of Agriculture (ESALQ/USP). Also, to the collaboration of the technicians Luís Fernando Baldesin and Denise Mescolotti. We thanks Samuel E. Jones for critically reading the text and for all his contributions to improve it.
Conflict of interest
The authors declare that the research was conducted in the absence of any commercial or financial relationships that could be construed as a potential conflict of interest.
Publisher’s note
All claims expressed in this article are solely those of the authors and do not necessarily represent those of their affiliated organizations, or those of the publisher, the editors and the reviewers. Any product that may be evaluated in this article, or claim that may be made by its manufacturer, is not guaranteed or endorsed by the publisher.
References
1. Gellatly K, Dennis DT. Plant biotechnology and GMOs. In: Moo-Young M, editor. Comprehensive biotechnology, 2nd ed. Amsterdam, The Netherlands: Academic Press (2011). p. 9–22. doi: 10.1016/B978-0-08-088504-9.00272-5
2. Briggs J. Green revolution. In: Kitchin R, Thrift N, editors. International encyclopedia of human geography. Amsterdam, The Netherlands: Elsevier Ltd (2009). p. 634–8. doi: 10.1016/B978-008044910-4.00099-7
3. John DA, Babu GR. Lessons from the aftermaths of green revolution on food system and health. Front Sustain Food Syst (2021) 5:644559. doi: 10.3389/fsufs.2021.644559
4. Cardoso EJBN, Vasconcellos RLF, Bini D, Miyauchi MYH, dos Santos CA, Alves PRL, et al. Soil health: looking for suitable indicators. what should be considered to assess the effects of use and management on soil health? Sci Agric (2013) 70:274–89. doi: 10.1590/S0103-90162013000400009
5. Global Wealth Report. (2022). Available at: https://www.credit-suisse.com/about-us/en/reports-research/global-wealth-report.html.
6. da Silva Junior CA, Teodoro PE, Delgado RC, Teodoro LP, Lima M, de Andréa Pantaleão A, et al. Persistent fire foci in all biomes undermine the Paris agreement in Brazil. Sci Rep (2020) 10:16246. doi: 10.1038/s41598-020-72571-w
7. Franco AL, Sobral BW, Silva AL, Wall DH. Amazonian Deforestation and soil biodiversity. Conserv Biol (2019) 33:590–600. doi: 10.1111/cobi.13234
8. Nobre CA, Sampaio G, Borma LS, Castilla-rubio JC, Silva JS, Cardoso M. Land-use and climate change risks in the Amazon and the need of a novel sustainable development paradigm. PNAS (2016) 113:10759–68. doi: 10.1073/pnas.1605516113
9. MapBiomas, Brasil. Deforestation in 2021 increased by 20%, with growth in all biomes (2022). Available at: https://mapbiomas.org/en/deforestation-in-2021-increased-by-20–with-growth-in-all-biomes-1.
10. Pinho PF, Anjos LJS, Rodrigues-filho S, Santos DV, Toledo PM. Projections of Brazilian biomes resilience and socio-environmental risks to climate change. Sustainability Debate (2020) 11:225–41. doi: 10.18472/SustDeb.v11n3.2020.33918
11. Mazoyer M, Roudart L. A history of world agriculture: from the neolithic age to the current crisis. New York, New York, USA: NYU Press (2006).
12. FAO. The food and agricultural organization 2001 (2001). Available at: https://www.fao.org/3/ae371e/ae371e00.pdf.
13. National Geographic. Domestication origins (2022). Available at: https://education.nationalgeographic.org/resource/domestication-origins/ (Accessed 30 March, 2023).
14. Possamai EJ, Luise M, Bartz C, Ralisch R. Adoption of the no-tillage system in paraná state: a (re)view. Rev Bras Cienc Solo (2022) 46:e0210104. doi: 10.36783/18069657rbcs20210104
15. EMBRAPA. Empresa brasileira de pesquisa agropecuária – história da embrapa (2020). Available at: https://www.embrapa.br/en/memoria-embrapa/a-embrapa (Accessed 30 March, 2023).
16. Boyd J, Banzhaf S. What are ecosystem services? the need for standardized environmental accounting units. Ecol Econ (2007) 63:616–26. doi: 10.1016/j.ecolecon.2007.01.002
17. Ricroch A, Clairand P, Harwood W. Use of CRISPR systems in plant genome editing: toward new opportunities in agriculture. Emerg Top Life Sci (2017) 1(2):169–82. doi: 10.1042/ETLS20170085
18. MAPA - Ministério da Agricultura e Pecuária. Principais marcos históricos dos 160 anos do MAPA (2020). Available at: https://www.gov.br/agricultura/pt-br/assuntos/noticias/desde-1860-mapa-acompanhou-a-transformacao-do-setor-agricola-brasileiro (Accessed 30 March, 2023).
19. Fraterrigo JM. Landscape legacies. In: Levin SA, editor. Encyclopedia of biodiversity, 4th ed. Amsterdam, The Netherlands: Elsevier Ltd (2013). p. 524–30. doi: 10.1016/B978-0-12-384719-5.00388-9
20. Lima CEP, Guedes MR, Silva J, Alcântara FA, Madeira NR, Carvalho ADF, et al. Effects of five years adoption of no-tillage systems for vegetables crops in soil organic matter contents. Agric Sci (2018) 9:117–28. doi: 10.4236/as.2018.91009
21. Bünemann EK, Bongiorno G, Bai Z, Creamer RE, De Deyn G, De Goede R, et al. Soil quality–a critical review. Soil Biol Biochem (2018) 120:105–25. doi: 10.1016/j.soilbio.2018.01.030
22. Doran JW, Parkin TB. Defining and assessing soil quality. In: Doran JW, Coleman DC, Bezdicek DF, Stewart BA, editors. Defining soil quality for a sustainable environment. Madison, WI, USA: Soil Science Society of America Journal, Madison (1994). p. 3–21. doi: 10.2136/sssaspecpub35.c1
23. Karlen DL, Mausbach MJ, Doran JW, Cline RG, Harris RF, Schuman GE. Soil quality: a concept, definition, and framework for evaluation (a guest editorial). SSSAJ (1997) 61(1):4–10. doi: 10.2136/sssaj1997.03615995006100010001x
24. Fabiansson SU. Safety of organic foods. In: Motarjemi Y, Moy G, Todd E, editors. Encyclopedia of food safety. Madison, WI, USA: Elsevier Ltd (2014). p. 417–21. doi: 10.1016/B978-0-12-378612-8.00301-2
25. Mie A, Andersen HR, Gunnarsson S, Kahl J, Kesse-guyot E, Rembia E, et al. Human health implications of organic food and organic agriculture: a comprehensive review. Environ Health (2017) 16:111. doi: 10.1186/s12940-017-0315-4
26. Chausali N, Saxena J. Conventional versus organic farming: nutrient status. In: Meena VS, Rakshit A, Srinivasarao C, Meena SK, Stanley J, editors. Advances in organic farming. Amsterdam, The Netherlands: Woodhead Publishing (2021). p. 241–54. doi: 10.1016/B978-0-12-822358-1.00003-1
27. da Luz FB, da Silva VR, Mallmann FJK, Pires CAB, Debiasi H, Franchini JC, et al. Monitoring soil quality changes in diversified agricultural cropping systems by the soil management assessment framework (SMAF) in southern Brazil. Agric Ecosyst Environ (2019) 281:100–10. doi: 10.1016/j.agee.2019.05.006
28. da Luz FB, Gonzaga LC, Castioni GAF, de Lima RP, Carvalho JLN, Cherubin MR. Controlled traffic farming maintains soil physical functionality in sugarcane fields. Geoderma (2023) 432:116427. doi: 10.1016/j.geoderma.2023.116427
29. Franco HHS, Guimarães RML, Tormena CA, Cherubin MR, Favilla HS. Global applications of the visual evaluation of soil structure method: a systematic review and meta-analysis. Soil Tillage Res (2019) 190:61–9. doi: 10.1016/j.still.2019.01.002
30. Cherubin MR, Eitelwein MT, Fabbris C, Weirich SW, Silva RFD, Silva VRD, et al. Qualidade física, química e biológica de um latossolo com diferentes manejos e fertilizantes. Rev Bras Cienc Solo (2015) 39:615–25. doi: 10.1590/01000683rbcs20140462
31. Pereira APA, Cherubin MR, Araujo AS, Santana MC, Medeiros ÉV, Costa DP, et al. Soil health assessment in mixed eucalypt plantations with N2-fixing trees. SSRN (2022), 4069626. doi: 10.2139/ssrn.4069626
32. Farhate CVV, Souza ZMD, Cherubin MR, Lovera LH, Oliveira IND, Guimarães Júnnyor WDS, et al. Soil physical change and sugarcane stalk yield induced by cover crop and soil tillage. Rev Bras Cienc Solo (2022) 46:1–24. doi: 10.36783/18069657rbcs20210123
33. Kosmas C, Kairis O, Karavitis C, Ritsema C, Salvati L, Acikalin S, et al. Evaluation and selection of indicators for land degradation and desertification monitoring: methodological approach. Environ Manage (2014) 54:951–70. doi: 10.1007/s00267-013-0109-6
34. Karlen DL, Veum KS, Sudduth KA, Obrycki JF, Nunes MR. Soil health assessment: past accomplishments, current activities, and future opportunities. Soil Tillage Res (2019) 195:104365. doi: 10.1016/j.still.2019.104365
35. Cherubin MR, Karlen DL, Cerri CE, Franco AL, Tormena CA, Davies CA, et al. Soil quality indexing strategies for evaluating sugarcane expansion in Brazil. PloS One (2016) 11(3):e0150860. doi: 10.1371/journal.pone.0150860
36. Zornoza R, Acosta JA, Bastida F, Domínguez SG, Toledo DM, Faz A. Identification of sensitive indicators to assess the interrelationship between soil quality, management practices and human health. SOIL (2015) 1(1):173–85. doi: 10.5194/soil-1-173-2015
37. Lehmann J, Bossio DA, Kögel-Knabner I, Rillig MC. The concept and future prospects of soil health. Nat Rev Earth Environ (2020) 1:544–53. doi: 10.1038/s43017-020-0080-8
38. Mendes R, Garbeva P, Raaijmakers JM. The rhizosphere microbiome: significance of plant beneficial, plant pathogenic, and human pathogenic microorganisms. FEMS Microbiol Ver (2013) 37:634–63. doi: 10.1111/1574-6976.12028
39. Chandra A, Mcnamara KE, Dargusch P, Chandra A, Mcnamara KE, Climate- PD, et al. Climate-smart agriculture: perspectives and framings. Clim Policy (2018) 18:256–4. doi: 10.1080/14693062.2017.1316968
40. Araújo VLVP, Fracetto GG, Silva AMM, Pereira APA, Freitas CC, Barros FMR, et al. Potential of growth-promoting bacteria in maize (Zea mays l.) varies according to soil moisture. Microbiol Res (2023) 271:127352. doi: 10.1016/j.micres.2023.127352
41. Silva AMM, Jones D, Chadwick DR, Qi X, Cotta SR, Araújo VLVP, et al. Can arbuscular mycorrhizal fungi and rhizobacteria facilitate 33P uptake in maize plants under water stress? Microbiol Res (2023) 271:127350. doi: 10.1016/j.micres.2023.127350
42. Starke R, Siles A, Lima M, Fernandes P, Schallert K, Benndorf D, et al. The structure and function of soil archaea across biomes. J Proteomics (2021) 237:104147. doi: 10.1016/j.jprot.2021.104147
43. Mendes IC, Sousa DMG, Reis Junior FB, Lopes AAC, Souza LM, v. X. Bioanálise de solo: aspectos teóricos e práticos. In Severiano EC, Morais MF, Paula AM editors. Tópicos em Ciec do Solo. Viçosa, MG: Sociedade Brasileira de Ciência do Solo (2019) 10:399–462. Available at: https://docplayer.com.br/191915208-Bioanalise-de-solo-aspectos-teoricos-e-praticos.html. (Accessed 5 May 2023).
44. Costanza R, d’Arge R, De Groot R, Farber S, Grasso M, Hannon B. The value of the world’s ecosystem services and natural capital. Nature (1997) 387(6630):253–60. doi: 10.1038/387253a0
45. Silva AMM, Cardoso EJBN. A sustentabilidade ambiental e os serviços ecossistêmicos. In: Cardoso EJBN, editor. A sustentabilidade ambiental da agricultura e de florestas tropicais – uma visão científica, ecológica, política e social. Curitiba, PR, Brazil: Editora Appris (2021). p. 51–68.
46. Schulte RP, Creamer RE, Donnellan T, Farrelly N, Fealy R, O’Donoghue C, et al. Functional land management: a framework for managing soil-based ecosystem services for the sustainable intensification of agriculture. Environ Sci Policy (2014) 38:45–58. doi: 10.1016/j.envsci.2013.10.002
47. Cherubin MR, Carvalho JLN, Cerri CEP, Nogueira LAH, Souza GM, Cantarella H. Land use and management effects on sustainable sugarcane-derived bioenergy. Land (2021) 10:72. doi: 10.3390/land10010072
48. Foley JA, Ramankutty N, Brauman KA, Cassidy ES, Gerber JS, Johnston M, et al. Solutions for a cultivated planet. Nature (2011) 478:337–42. doi: 10.1038/nature10452
49. Boddey RM, Jantalia CP, Conceição PC, Zanatta JA, Bayer C, Mielniczuk J, et al. Carbon accumulation at depth in ferralsols under zero-till subtropical agriculture. Global Change Biol (2010) 16:784–95. doi: 10.1111/j.1365-2486.2009.02020.x
50. Finger R, Swinton SM, El Benni N, Walter A. Precision farming at the nexus of agricultural production and the environment. Annu Rev Resour Econ (2019) 11:313–35. doi: 10.1146/annurev-resource-100518-093929
51. Moraes Sá JC, Lal R, Cerri CC, Lorenz K, Hungria M, de Faccio Carvalho PC. Low-carbon agriculture in south America to mitigate global climate change and advance food security. Environ Int (2017) 98:102–12. doi: 10.1016/j.envint.2016.10.020
52. Kassam A, Friedrich T, Derpsch R. Global spread of conservation agriculture. Int J Environ Stud (2019) 76(1):29–51. doi: 10.1080/00207233.2018.1494927
53. Silva AP, Babujia LC, Franchini JC, Souza A, Hungria M. Microbial biomass under various soil- and crop-management systems in short and long-term experiments in Brazil. Field Crops Res (2010) 119:20–6. doi: 10.1016/j.fcr.2010.06.012
54. CONAB – Companhia Nacional de Abastecimento. Produção agrícola–safra: série histórica dos grãos (2020). Available at: https://www.conab.gov.br/info-agro/safras/graos (Accessed 30 march 2023).
55. CONAB – Companhia Nacional de Abastecimento. Produção agrícola (2023). Brasília, DF: Acompanhamento da safra Brasileira de Grãos (Accessed 30 march 2023).
56. Mendes LC, Tormena CA, Cherubin MR, Karlen DL. Soil health assessment and maintenance in central and south central Brazil. In: Reicosky D, editor. Managing soil health for sustainable agriculture. Cambridge, UK: Burleigh Dodds Science Publishing (2018). p. 379–415. doi: 10.19103/AS.2017.0033.35
57. Freitas PL, Landers JN. The transformation of agriculture in Brazil through development and adoption of zero tillage conservation agriculture. ISWCR (2014) 2(1):35–46. doi: 10.1016/S2095-6339(15)30012-5
58. Lisboa IP, Cherubin MR, Satiro LS, Siqueira-Neto M, Lima RP, Gmach MR, et al. Applying soil management assessment framework (SMAF) on short-term sugarcane straw removal in Brazil. Ind Crops Prod (2019) 129:175–84. doi: 10.1016/j.indcrop.2018.12.004
59. Obade VDP, Lal R. A standardized soil quality index for diverse field conditions. Sci Total Environ (2016) 541:424–34. doi: 10.1016/j.scitotenv.2015.09.096
60. Andrews SS, Karlen DL, Cambardella CA. The soil management assessment framework. Soil Sci Soc Am J (2004) 68:1945–62. doi: 10.2136/sssaj2004.1945
61. Cherubin MR, Tormena CA, Karlen DL. Soil quality evaluation using the soil management assessment framework (SMAF) in brazilian oxisols with contrasting texture. Rev Bras Cienc Solo (2017) 41:e0160148. doi: 10.1590/18069657rbcs20160148
62. Cherubin MR, Karlen DL, Franco ALC, Cerri CEP, Tormena CA, Cerri CC. A soil management assessment framework (SMAF) evaluation of Brazilian sugarcane expansion on soil quality. Soil Sci Soc Am J (2016) 80:215–26. doi: 10.2136/sssaj2015.09.0328
63. Jimenez LCZ, Queiroz HM, Cherubin MR, Ferreira TO. Applying the soil management assessment framework (SMAF) to assess mangrove soil quality. Sustainability (2022) 14(5):3085. doi: 10.3390/su14053085
64. Marion LF, Schneider R, Cherubin MR, Colares GS, Wiesel PG, da Costa AB, et al. Development of a soil quality index to evaluate agricultural cropping systems in southern Brazil. Soil Tillage Res (2022) 218:105293. doi: 10.1016/j.still.2021.105293
65. Ball BC, Batey T, Munkholm LJ. Field assessment of soil structural quality: a development of the peerdklamp test. Soil Use Manag (2007) 23(4):329–37. doi: 10.1111/j.1475-2743.2007.00102.x
66. Rabot E, Wiesmeier M, Schlüter S, Vogel HJ. Soil structure as an indicator of soil functions: a review. Geoderma (2018) 31:122–37. doi: 10.1016/j.geoderma.2017.11.009
67. Ball BC, Guimarães RML, Cloy JM, Hargreaves PR, Shepherd TG, Mckenzie BM. Visual soil evaluation: a summary of some applications and potential developments for agriculture. Soil Tillage Res (2017) 173:114–24. doi: 10.1016/j.still.2016.07.006
68. Guimarães RML, Ball BC, Tormena CA. Improvements in the visual evaluation of soil structure. Soil Use Manag (2011) 27(3):395–403. doi: 10.1111/j.1475-2743.2011.00354.x
69. Tuchtenhagen IK, de Lima CLR, Bamberg AL, Guimarães RML, Mansonia PM. Visual evaluation of the soil structure under different management systems in lowlands in southern Brazil. Rev Bras Cienc Solo (2018) 42:e0170270. doi: 10.1590/18069657rbcs20170270
70. Guimarães RML, Neves Junior AF, Silva WG, Rogers CD, Ball BC, Montes CR, et al. The merits of the visual evaluation of soil structure method (VESS) for assessing soil physical quality in the remote, undeveloped regions of the Amazon basin. Soil Tillage Res (2017) 173:75–82. doi: 10.1016/j.still.2016.10.014
71. Strassburg BBN, Latawiec AE, Barioni LG, Nobre CA, Silva VP, Valentim JF, et al. When enough should be enough: improving the use of current agricultural lands could meet production demands and spare natural habitats in Brazil. Glob Environ Change (2014) 28:84–97. doi: 10.1016/j.gloenvcha.2014.06.001
72. FAO. FAOSTAT statistical database. Rome, Italy: The Food and Agriculture Organization (2020). Available at: https://www.fao.org/faostat/en/#data/QC. (accessed on 5 May 2023).
73. Keller T, Lamandé M, Naderi-Boldaji M, de Lima RP. Soil compaction due to agricultural field traffic: an overview of current knowledge and techniques for compaction quantification and mapping. In: Saljnikov E, Mueller L, Lavrishchev A, Eulenstein F, editors. Advances in understanding soil degradation. Cham: Springer International Publishing (2022). p. 287–312. doi: 10.1007/978-3-030-85682-3_13
74. Santos RS, Wiesmeier M, Cherubin MR, Oliveira DM, Locatelli JL, Holzschuh M, et al. Consequences of land-use change in brazil’s new agricultural frontier: a soil physical health assessment. Geoderma (2021) 400:115149. doi: 10.1016/j.geoderma.2021.115149
75. Cherubin MR, Oliveira DMDS, Feigl BJ, Pimentel LG, Lisboa IP, Gmach MR. Crop residue harvest for bioenergy production and its implications on soil functioning and plant growth: a review. Sci Agric (2018) 75:255–72. doi: 10.1590/1678-992x-2016-0459
76. Sousa Junior JGDA, Cherubin MR, Oliveira BG, Cerri CEP, Cerri CC, Feigl BJ. Three-year soil carbon and nitrogen responses to sugarcane straw management. Bioenergy Res (2018) 11:249–61. doi: 10.1007/s12155-017-9892-x
77. Castioni GAF, Cherubin MR, Bordonal RDO, Barbosa LC, Menandro LMS, Carvalho JLN. Straw removal affects soil physical quality and sugarcane yield in Brazil. Bioenergy Res (2019) 12:789–800. doi: 10.1007/s12155-019-10000-1
78. De Paul Obade V. Quantifying the interactions of land management practices and agricultural productivity using a soil quality index. Soil Use Manage (2017) 33:639–52. doi: 10.1111/sum.12379
79. Gonçalves JLM, Alvares CA, Higa AR, Silva LD, Alfenas AC, Stahl J, et al. Integrating genetic and silvicultural strategies to minimize abiotic and biotic constraints in Brazilian eucalypt plantations. For Ecol Manag (2013) 301:6–27. doi: 10.1016/j.foreco.2012.12.030
80. Rocha JHT, Gonçalves JLM, Gava JL, Godinho TO, Melo EASC, Bazani JH, et al. Forest residue maintenance increased the wood productivity of a eucalyptus plantation over two short rotations. For Ecol Manag (2016) 379:1–10. doi: 10.1016/j.foreco.2016.07.042
81. Rocha JHT, Gonçalves JLM, Brandani CB, Ferraz AV, Franci AF, Marques ERG, et al. Forest residue removal decreases soil quality and affects wood productivity even with high rates of fertilizer application. For Ecol Manag (2018) 430:188–95. doi: 10.1016/j.foreco.2018.08.010
82. São José JFB, Cherubin MR, Vargas LK, Lisboa BB, Zanatta JÁ, Araújo EF, et al. A soil quality index for subtropical sandy soils under different eucalyptus harvest residue managements. J For Res (2023) 34:243–55. doi: 10.1007/s11676-022-01507-z
83. Pereira APA, Santana MC, Zagatto MR, Brandani CB, Wang JT, Verma JP, et al. Nitrogen-fixing trees in mixed forest systems regulate the ecology of fungal community and phosphorus cycling. Sci Total Environ (2020) 758:143711. doi: 10.1016/j.scitotenv.2020.143711
84. Pereira APA, Durrer A, Gumiere T, Gonçalves JLM, Robin A, Bouillet JP, et al. Mixed eucalyptus plantations induce changes in microbial communities and increase biological functions in the soil and litter layers. For Ecol Manag (2019) 433:332–42. doi: 10.1016/j.foreco.2018.11.018
85. Koutika LS, Cafiero L, Bevivino A, Merino A. Organic matter quality of forest floor as a driver of c and p dynamics in acacia and eucalypt plantations established on a ferralic arenosols, Congo. For Ecosyst (2020) 7:40. doi: 10.1186/s40663-020-00249-w
86. Santana MC, Pereira APA, Souza AJ, Zagatto MRG, Araújo VLVP, Wang JT, et al. Shifts on archaeal community structure in pure and mixed eucalyptus grandis and acacia mangium plantations. For Ecol Manag (2021) 492:119218. doi: 10.1016/j.foreco.2021.119218
87. Celentano D, Rousseau GX, Paixão LS, Lourenço F, Cardozo EG, Rodrigues TO, et al. Carbon sequestration and nutrient cycling in agroforestry systems on degraded soils of Eastern Amazon, Brazil. Agroforestry Syst (2020) 94(5):1781–92. doi: 10.1007/s10457-020-00496-4
88. Feliciano D, Ledo A, Hillier J, Nayak DR. Which agroforestry options give the greatest soil and above ground carbon benefits in different world regions? Agr Ecosyst Environ (2018) 254:117–29. doi: 10.1016/j.agee.2017.11.032
89. Matos PS, Cherubin MR, Damian JM, Rocha FI, Pereira MG, Zonta E. Short-term effects of agroforestry systems on soil health in southeastern Brazil. Agroforestry Syst (2022) 96(5-6):897–908. doi: 10.1007/s10457-022-00749-4
90. Simon CP, Gomes TF, Pessoa TN, Soltangheisi A, Bieluczyk W, de Camargo PB, et al. Soil quality literature in Brazil: a systematic review. Rev Bras Cienc do Solo (2022) 46:e0210103. doi: 10.36783/18069657rbcs20210103
91. Lal R, Horn R, Kosaki T. Soil and sustainable development goals. Stuttgart Germany: Schweizerbart Science Publishers (2018). Available at: https://www.schweizerbart.de/publications/detail/isbn/9783510654253/Soil_and_Sustainable_Development_Goals (accessed on 5 May 2023).
92. IBGE. Mapa de biomas do brasil – primeira aproximação. Rio de Janeiro, 2004 (2004). Available at: ftp://geoftp.ibge.gov.br/mapas/tematicos/mapas_murais/biomas.pdf.
93. IBGE. Biomas e sistema costeiro-marinho do brasil (2019) - série relatórios metodológicos volume 45 - compatível com a escala 1:250 000 (2019). Available at: https://biblioteca.ibge.gov.br/index.php/biblioteca-catalogo?view=detalhes&id=2101676.
94. Guerra A, Reis LK, Borges FLG, Ojeda PTA, Pineda DAM, Miranda CO, et al. Ecological restoration in Brazilian biomes: identifying advances and gaps. For Ecol Manage (2020) 458:117802. doi: 10.1016/j.foreco.2019.117802
95. Botelho J, Costa SCP, Ribeiro JG, Souza CM. Mapping roads in the Brazilian Amazon with artificial intelligence and sentinel-2. Remote Sens (2022) 14:3625. doi: 10.3390/rs14153625
96. Bowman KW, Dale SA, Dhanani S, Nehru J, Rabishaw BT. The degradation of the Amazon rainforest: regional and global climate implications. In: Ongoma V, Tabari H, editors. Climate impacts on extreme weather. Amsterdam, The Netherlands: Elsevier Ltd (2022). p. 217–34. doi: 10.1016/B978-0-323-88456-3.00011-3
97. Anjos LHC, Pereira MG. Estado atual do conhecimento dos solos na região amazônica. In: Wadt PGS, Marcolan AL, Matoso SCG, Pereira MG, editors. Manejo dos solos e a sustentabilidade da produção agrícola na amazônia ocidental, vol. 1. Núcleo Regional Amazônia Ocidental: SBCS (2014). p. 50–65.
98. Finer M, Mamani N. Amazon Deforestation hotspots 2020 (Final). Peru: MAAP (2020). p. 136. Available at: https://maaproject.org/2021/amazon-hotspots-2020-final.
99. WWF. World wildlife fund. unsustainable cattle ranching (2020). Available at: https://wwf.panda.org/discover/knowledge_hub/where_we_work/amazon/amazon_threats/unsustainable_cattle_ranching.
100. Mackey B, Prentice IC, Steffen W, House JI, Lindenmayer D, Keith H, et al. Science and climate change mitigation policy. Nat Publ Gr (2013) 3:552–7. doi: 10.1038/nclimate1804
101. Börner J, Wunder S, Wertz-Kanounnikoff S, Tito MR, Pereira L, Nascimento N. Direct conservation payments in the Brazilian Amazon: scope and equity implications. Ecol Econ (2010) 69:1272–82. doi: 10.1016/j.ecolecon.2009.11.003
102. Grima N, Singh SJ, Smetschka B, Ringhofer L. Payment for ecosystem services (PES) in Latin America: analysing the performance of 40 case studies. Ecosyst Serv (2016) 17:24–32. doi: 10.1016/j.ecoser.2015.11.010
103. Karlen DL, Stott DE. A framework for evaluating physical and chemical indicators of soil quality. In: Doran JW, Coleman DC, Bezdicek DF, Stewart BA, editors. Defining soil quality for a sustainable environment. Madison, WI, USA: Soil Science Society of America Journal, Madison (1994). p. 53–72. doi: 10.2136/sssaspecpub35.c4
104. Saccá ML, Barra Caracciolo A, Di Lenola M, Grenn P. Ecosystem services provided by soil microorganisms. In: Lukac M, Grenni P, Gamboni M, editors. Soil biological communities and ecosystem resilience. Springer: Cham (2017). p. 9–24. doi: 10.1007/978-3-319-63336-7_2
105. Fonseca JP, Hoffmann L, Cabral BCA, Dias VHG, Miranda MR, de Azevedo Martins AC, et al. Contrasting the microbiomes from forest rhizosphere and deeper bulk soil from an Amazon rainforest reserve. Gene (2018) 642:389–97. doi: 10.1016/j.gene.2017.11.039
106. Melo VF, Barros LS, Silva MCS, Veloso TGR, Senwo ZN, Matos KS, et al. Soil bacterial diversities and response to deforestation, land use and burning in north Amazon, Brazil. Appl Soil Ecol (2021) 158:103775. doi: 10.1016/j.apsoil.2020.103775
107. Lal R. Soil carbon sequestration impacts on global climate change and food security. Science (2004) 304:1623–7. doi: 10.1126/science.1097396
108. Cerri CEP, Cerri CC, Maia SMF, Cherubin MR, Feigl BJ, Lal R. Reducing Amazon deforestation through agricultural intensification in the cerrado for advancing food security and mitigating climate change. Sustain (2018) 10:1–18. doi: 10.3390/su10040989
109. Silva-Olaya AM, Olaya-Montes A, Polanía-Hincapié KL, Cherubin MR, Duran-Bautista EH, Ortiz-Morea FA. Silvopastoral systems enhance soil health in the amazon region. Sustain (2022) 14:1–18. doi: 10.3390/su14010320
110. Silva-Olaya AM, Ortíz-Morea FA, Espa GP, Grados D, Gasparatos A, Roberto M. Composite index for soil-related ecosystem services assessment: insights from rainforest-pasture transitions in the Colombian Amazon. Ecosyst Serv (2022) 57:101463. doi: 10.1016/j.ecoser.2022.101463
111. Bowman KW, Dale SA, Dhanani S, Nehru J, Rabishaw BT. The degradation of the Amazon rainforest: regional and global climate implications. In: Ongoma V, Tabari H, editors. Climate impacts on extreme weather. Amsterdam, The Netherlands: Elsevier Ltd (2022). p. 217–34. doi: 10.1016/B978-0-323-88456-3.00011-3
112. Tollefson J. Illegal mining in the Amazon hits record high amid indigenous protests. Nature (2021) 598:15–6. doi: 10.1038/d41586-021-02644-x
113. IBGE. Brazilian Institute of geography and statistics. Brasília, DF, Brazil: IPCA (2022). Available at: https://sidra.ibge.gov.br/pesquisa/snipc/ipca/quadros/brasil/julho-2022.
114. Rajão R, Nobre AD, Cunha ELTP, Duarte TR, Marcolino C, Soares-Filho B, et al. The risk of fake controversies for Brazilian environmental policies. Biol Conserv (2022) 266:109447. doi: 10.1016/j.biocon.2021.109447
115. Silva JSV, Abdon MM, Boock A, Silva MP. Fitofisionomias dominantes em parte das sub-regiões do nabileque e Miranda, sul do pantanal. Pesq agropec Bras (1998). p:1713–9. Available at: https://seer.sct.embrapa.br/index.php/pab/article/view/5049 (accessed on 5 May 2023).
116. Penatti NC, Almeida TIRde, Ferreira LG, Arantes AE, Coe MT. Satellite-based hydrological dynamics of the world’s largest continuous wetland. Remote Sens Environ (2015) 170:1–13. doi: 10.1016/j.rse.2015.08.031
117. Roesch LFW, Vieira FCB, Pereira VA, Schünemann AL, Teixeira IF, Senna AJT, et al. The Brazilian pampa: a fragile biome. Diversity (2009) 1:182–98. doi: 10.3390/d1020182
118. Tomas WM, de Oliveira Roque F, Morato RG, Medici PE, Chiaravalloti RM, Tortato FR, et al. Sustainability agenda for the pantanal wetland: perspectives on a collaborative interface for science, policy, and decision-making. Trop Conserv Sci (2019) 12:1–30. doi: 10.1177/1940082919872634
119. Ribeiro S, Moreira LFB, Overbeck GE, Maltchik L. Protected areas of the pampa biome presented land use incompatible with conservation purposes. J Land Use Sci (2021) 16:260–72. doi: 10.1080/1747423X.2021.1934134
120. Libonati R, Geirinhas J o. L, Silva PS, Russo A, Rodrigues JA, Belém LBC, et al. Assessing the role of compound drought and heatwave events on unprecedented 2020 wildfires in the pantanal. Environ Res Lett (2022) 17(1):015005. doi: 10.1088/1748-9326/ac462e
121. Berlinck CN, Lima LHA, Pereira AMM, Carvalho EAR, Paula RC, Thomas WM, et al. The pantanal is on fire and only a sustainable agenda can save the largest wetland in the world. Braz J Biol (2021) 82:1–2. doi: 10.1590/1519-6984.244200
123. Cecagno D, Anghinoni I, de Andrade Costa SEVG, Brambilla DM, Martins AP, Magiero EC, et al. Long-term nitrogen fertilization in native pasture with Italian ryegrass introduction - effects on soil health attribute indicators. Ciec Rural (2017) 47(5):1–6. doi: 10.1590/0103-8478cr20150635
124. Behrends Kraemer F, Morrás H, Fernández PL, Duval M, Galantini J, Garibaldi L. Influence of edaphic and management factors on soils aggregates stability under no-tillage in mollisols and vertisols of the pampa region, Argentina. Soil Tillage Res (2021) 209:104901. doi: 10.1016/j.still.2020.104901
125. Pheap S, Lefèvre C, Thoumazeau A, Leng V, Boulakia S, Koy R, et al. Multi-functional assessment of soil health under conservation agriculture in Cambodia. Soil Tillage Res (2019) 194:104349. doi: 10.1016/j.still.2019.104349
126. Rodriguez S, Baeza MC, García GV, Domínguez GF, Clemente NL, Studdert GA. Permanganate oxidizable carbon, new soil health indicator for mollisols of the southeastern argentinean pampas? Commun Soil Sci Plant Anal (2022) 53(16):2029–44. doi: 10.1080/00103624.2022.2070631
127. Altman N, Krzywinski M. Points of significance: association, correlation and causation. Nat Methods (2015) 12(10):899–900. doi: 10.1038/nmeth.3587
128. FAO, UNEP. The state of the world’s forests 2020. Rome: Forest biodivers peop (2020). doi: 10.4060/ca8642en
129. Van Der Ent RJ, Savenije HHG, Schaefli B, Steele-Dunne SC. Origin and fate of atmospheric moisture over continents. Water Resour Res (2010) 46(9):9525. doi: 10.1029/2010WR009127
130. Syktus JI, McAlpine CA. More than carbon sequestration: biophysical climate benefits of restored savanna woodlands. Sci Rep (2016) 6(1):1–11. doi: 10.1038/srep29194
131. Ellison D. Background analytical study 2 forests and water. New York, NY, USA: The United Nations Forum on Forests (2018). Available at: https://www.un.org/esa/forests/wp-content/uploads/2018/04/UNFF13_BkgdStudy_ForestsWater.pdf. (accessed on 5 May 2023).
132. Steffen W, Richardson K, Rockström J, Cornell SE, Fetzer I, Bennett EM, et al. Planetary boundaries: guiding human development on a changing planet. Science (2015) 347(6223):1259855. doi: 10.1126/science.1259855
133. Alroy J. Effects of habitat disturbance on tropical forest biodiversity. Proc Natl Acad Sci (2017) 114(23):6056–61. doi: 10.1073/pnas.1611855114
134. Cadman T, Maraseni T, Ma HO, Lopez-Casero F. Five years of REDD+ governance: the use of market mechanisms as a response to anthropogenic climate change. For Policy Econ (2017) 1:79. doi: 10.1016/j.forpol.2016.03.008
135. Pörtner HO, Scholes RJ, Agard J, Archer E, Arneth A, Bai X, et al. Scientific outcome of the IPBES-IPCC co-sponsored workshop on biodiversity and climate change. Bonn, Germany: Intergovernmental Science-Policy Platform on Biodiversity and Ecosystem Services (IPBES) (2021). Available at: https://zenodo.org/record/5101125.
136. Matricardi EAT, Skole DL, Costa OB, Pedlowski MA, Samek JH, Miguel EP. Long-term forest degradation surpasses deforestation in the Brazilian Amazon. Science (2020) 369(6509):1378–82. doi: 10.1126/science.abb3021
137. Da Cruz DC, Benayas JMR, Ferreira GC, Santos SR, Schwartz G. An overview of forest loss and restoration in the Brazilian Amazon. New For (2021) 52:1–16. doi: 10.1007/s11056-020-09777-3
138. Feng Y, Su H, Tang Z, Wang S, Zhao X, Zhang H, et al. Reduced resilience of terrestrial ecosystems locally is not reflected on a global scale. Commun Earth Environ (2021) 2(1):1–11. doi: 10.1038/s43247-021-00163-1
139. Saatchi S, Longo M, Xu L, Yang Y, Abe H, André M, et al. Detecting vulnerability of humid tropical forests to multiple stressors. One Earth (2021) 4(7):988–1003. doi: 10.1016/j.oneear.2021.06.002
140. Quéré C, Andrew R, Friedlingstein P, Sitch S, Hauck J, Pongratz J, et al. Global carbon budget 2018. Earth Syst Sci Data (2018) 10(4):2141–94. doi: 10.18160/GCP-2018
141. Malhi Y, Gardner TA, Goldsmith GR, Silman MR, Zelazowski P. Tropical forests in the anthropocene. Annu Rev Environ Resour (2014) 39(1):125–59. doi: 10.1146/annurev-environ-030713-155141
142. Brando PM, Paolucci L, Ummenhofer CC, Ordway EM, Hartmann H, Cattau ME, et al. Droughts, wildfires, and forest carbon cycling: a pantropical synthesis. Annu Rev Earth Planet Sci (2019) 47:555–81. doi: 10.1146/annurev-earth-082517-010235
143. Jia G, Shevliakova E, Artaxo P, De Noblet-Ducoudré N, Houghton R, House J, et al. Land–climate interactions. In: Shukla PR, Skea J, Calvo Buendia E, Masson-Delmotte V, Pörtner HO, Roberts DC, et al, editors. Climate change and land: an IPCC special report on climate change, desertification, land degradation, sustainable land management, food security, and greenhouse gas fluxes in terrestrial ecosystems’. Geneva, Switzerland: Press-In Press (2019).
144. Douville H, Raghavan K, Renwick J, Allan RP, Arias PA, Barlow M, et al. Water cycle changes. in: climate change 2021: the physical science basis. contribution of working group I to the sixth assessment report of the intergovernmental panel on climate change. Masson-Delmotte V, Zhai P, Pirani A, Connors SL, Péan C, Berger S, et al, editors. Cambridge, England, UK: Cambridge University Press (2021).
145. Millennium ecosystem assessment, M. E. A. Ecosystems and human well-being. Washington, DC: Island press (2005).
146. Pan Y, Birdsey RA, Fang J, Houghton R, Kauppi PE, Kurz WA, et al. A large and persistent carbon sink in the world’s forests. Science (2011) 333(6045):988–93. doi: 10.1126/science.1201609
147. Harris NL, Gibbs DA, Baccini A, Birdsey RA, de Bruin S, Farina M, et al. Global maps of twenty-first century forest carbon fluxes. Nat Clim Chang (2021) 11(3):234–40. doi: 10.1038/s41558-020-00976-6
148. Baccini A, Walker W, Carvalho L, Farina M, Sulla-Menashe D, Houghton RA. Tropical forests are a net carbon source based on aboveground measurements of gain and loss. Science (2017) 358(6360):230–4. doi: 10.1126/science.aam5962
149. Di Marco M, Watson JEM, Currie DJ, Possingham HP, Venter O. The extent and predictability of the biodiversity–carbon correlation. Ecol Lett (2018) 21(3):365–75. doi: 10.1111/ele.12903
150. Aide TM, Clark ML, Grau HR, López-Carr D, Levy MA, Redo D, et al. Deforestation and reforestation of Latin America and the Caribbean (2001–2010). Biotropica (2013) 45(2):262–71. doi: 10.1111/j.1744-7429.2012.00908.x
151. Pörtner HO, Scholes RJ, Agard J, Archer E, Arneth A, Bai X, et al. Scientific outcome of the IPBES-IPCC co-sponsored workshop on biodiversity and climate change. Bonn, Germany: Intergovernmental Science-Policy Platform on Biodiversity and Ecosystem Services (IPBES (2021). Available at: https://zenodo.org/record/5101125.
152. Sanderman J, Hengl T, Fiske GJ. Soil carbon debt of 12,000 years of human land use. Proc Natl Acad Sci (2017) 114(36):9575–80. doi: 10.1073/pnas.1706103114
153. Matthews HD, Graham TL, Keverian S, Lamontagne C, Seto D, Smith T. National contributions to observed global warming. Environ Res Lett (2014) 9:1–9. doi: 10.1088/1748-9326/9/1/014010
154. Nogueira EM, Yanai AM, Fonseca FOR, Fearnside PM. Carbon stock loss from deforestation through 2013 in Brazilian Amazonia. Glob Chang Biol (2015) 21(3):1271–92. doi: 10.1111/gcb.12798
155. Pearson TRH, Brown S, Murray L, Sidman G. Greenhouse gas emissions from tropical forest degradation: an underestimated source. Carbon Balance Manag (2017) 12(1):1–11. doi: 10.1186/s13021-017-0072-2
156. Griscom BW, Adams J, Ellis PW, Houghton RA, Lomax G, Miteva DA, et al. Natural climate solutions. Proc Natl Acad Sci (2017) 114(44):11645–50. doi: 10.1073/pnas.1710465114
157. Matos FAR, Magnago LFS, Aquila Chan Miranda C, de Menezes LFT, Gastauer M, Safar NVH, et al. Secondary forest fragments offer important carbon and biodiversity cobenefits. Glob Chang Biol (2020) 26(2):509–22. doi: 10.1111/gcb.14824
158. Oberleitner F, Egger C, Oberdorfr S, Dullinger S, Wanek W, Hietz P. Recovery of aboveground biomass, species richness and composition in tropical secondary forests in SW Costa Rica. For Ecol Manage (2021) 479:118580. doi: 10.1016/j.foreco.2020.118580
159. Dalmaso CA, Marques MCM, Higuchi P, Zwiener VP, Marques R. Spatial and temporal structure of diversity and demographic dynamics along a successional gradient of tropical forests in southern Brazil. Ecol Evol (2020) 10(7):3164–77. doi: 10.1002/ece3.5816
160. Cook RL, Binkley D, Stape JL. Eucalyptus plantation effects on soil carbon after 20 years and three rotations in Brazil. For Ecol Manage (2016) 359:92–8. doi: 10.1016/j.foreco.2015.09.035
161. Capellesso ES, Cequinel A, Marques R, Luisa Sausen T, Bayer C, Marques MCM. Co-Benefits in biodiversity conservation and carbon stock during forest regeneration in a preserved tropical landscape. For Ecol Manage (2021) 492:119222. doi: 10.1016/j.foreco.2021.119222
162. Pyles MV, Prado-Junior JA, Magnago LFS, de Paula A, Meira-Neto JAA. Loss of biodiversity and shifts in aboveground biomass drivers in tropical rainforests with different disturbance histories. Biodivers Conserv (2018) 27(12):3215–31. doi: 10.1007/s10531-018-1598-7
163. Ferreira J, Lennox GD, Gardner TA, Thomson JR, Berenguer E, Lees AC, et al. Carbon-focused conservation may fail to protect the most biodiverse tropical forests. Nat Clim Chang (2018) 8(8):744–9. doi: s41558-018-0225-7
164. Smith CC, Espírito-Santo FDB, Healey JR, Young PJ, Lennox GD, Ferreira J, et al. Secondary forests offset less than 10% of deforestation-mediated carbon emissions in the Brazilian Amazon. Glob Chang Biol (2020) 26(12):7006–20. doi: 10.1111/gcb.15352
165. Nunes Sm, Oliveira L, Siqueira Jo, Morton DC, Souza CM. Unmasking secondary vegetation dynamics in the Brazilian Amazon. Environ Res Lett (2020) 15(3):034057. doi: 10.1088/1748-9326/ab76db
166. Bouchez T, Blieux AL, Dequiedt S, Domaizon I, Dufresne A, Ferreira S, et al. Molecular microbiology methods for environmental diagnosis. Environ Chem Lett (2016) 14:423–41. doi: 10.1007/s10311-016-0581-3
167. Hartmann M, Frey B, Mayer J, Mader P, Widmer F. Distinct soil microbial diversity under long-term organic and conventional farming. ISME J (2015) 9:1177–94. doi: 10.1038/ismej.2014.210
168. Barrios E. Soil biota, ecosystem services and land productivity. Ecol econ (2007) 64(2):269–85. doi: 10.1016/j.ecolecon.2007.03.004
169. Chabrillat S, Ben-Dor E, Cierniewski J, Gomez C, Schmid T, van Wesemael B. Imaging spectroscopy for soil mapping and monitoring. Surv Geophys (2019) 40:361–99. doi: 10.1007/s10712-019-09524-0
170. Gandariasbeitia M, Besga G, Albizu I, Larregla S, Mendarte S. Prediction of chemical and biological variables of soil in grazing areas with visible- and near-infrared spectroscopy. Geoderma (2017) 305:228–35. doi: 10.1016/j.geoderma.2017.05.045
171. Paz-Kagan T, Shachak M, Zaady E, Karnieli A. A spectral soil quality index (SSQI) for characterizing soil function in areas of changed land use. Geoderma (2014) 230–231:171–84. doi: 10.1016/j.geoderma.2014.04.003
172. Pietikäinen JX, Fritze H. Clear-cutting and prescribed burning in coniferous forest: comparison of effects on soil fungal and total microbial biomass, respiration activity and nitrification. Soil Biol Biochem (1995) 27:101–1. doi: 10.1016/0038-0717(94)00125-K
173. Chang CW, Laird DA, Mausbach MJ, Hurburgh CR Jr. Near-infrared reflectance spectroscopy - principal component regression analysis of soil properties. Soil Sci Soc Am J (2001) 65:480–90. doi: 10.2136/sssaj2001.652480x
174. Veum KS, Sudduth KE, Kremer RJ, Kitchen NR. Estimating a soil quality index with VNIR reflectance spectroscopy. Soil Sci Soc Am J (2015) 79:637–49. doi: 10.2136/sssaj2014.09.0390
175. Paustian K, Collier S, Baldock J, Burgess R, Creque J, DeLonge M, et al. Quantifying carbon for agricultural soil management: from the current status toward a global soil information system. Carbon Manage (2019) 10:567–87. doi: 10.1080/17583004.2019.1633231
176. Demattê JA, Paiva AFDS, Poppiel RR, Rosin NA, Ruiz LFC, Mello FADO, et al. The Brazilian s oil s pectral s ervice (BraSpecS): a user-friendly system for global soil spectra communication. Remote Sens (2022) 14(3):740. doi: 10.3390/rs14030740
177. Smith SE, Read DJ. The symbionts forming arbuscular mycorrhizas. In: Smith SE, Read DJ, editors. Mycorrhizal symbiosis, vol. 2 . Amsterdam, The Netherlands: Elsevier (2008). p. 13–41. doi: 10.1016/B978-012370526-6.50003-9
178. Nogueira MA, Cardoso EJBN. Produção de micélio externo por fungos micorrízicos arbusculares e crescimento da soja em função de doses de fósforo. Rev Bras Cienc do Solo (2000) 24(2):329–38. doi: 10.1590/S0100-06832000000200010
179. George E, Haussler K, Vetterlein D, Gorgus E, Marschner H. Water nutrient translocation by hyphae of glomus mosseae. Can J Bot (1992) 70:2130–213. doi: 10.1139/b92-265
180. Augé RM. Water relations, drought and vesicular-arbuscular mycorrhizal symbiosis. Mycorrhiza (2001) 11(1):3–42. doi: 10.1007/s005720100097
181. Cardoso EJBN, Andreote FD. Microbiologia do solo. Piracicaba: ESALQ (2016). p. 221. doi: 10.11606/9788586481567
182. Nasslahsen B, Prin Y, Ferhout H, Smouni A, Duponnois R. Mycorrhizae helper bacteria for managing the mycorrhizal soil infectivity. Front Soil Sci (2022) 15:979246. doi: 10.3389/fsoil.2022.979246
183. Nogueira MA, Cardoso EJBN. Mycorrhizal effectiveness and manganese toxicity in soybean as affected by soil type and endophyte. Sci Agric (2003) 60(2):329–35. doi: 10.1590/S0103-90162003000200018
184. Lambais MR, Cardoso EJBN. Avaliação da germinação de esporos de fungos micorrízicos vesículo-arbusculares e da colonização micorrízica de stylosanthes guianensis em solo ácido e distrófico. Rev Bras Cienc do Solo (1989) 12(3):249–55.
185. Jiang F, Zhang L, Zhou J, George TS, Feng G. Arbuscular mycorrhizal fungi enhance mineralisation of organic phosphorus by carrying bacteria along their extraradical hyphae. New Phytol (2021) 230(1):304–15. doi: 10.1111/nph.17081
186. Glick BR. Plant growth-promoting bacteria: mechanisms and applications. Scientifica (2012) 2012:963401. doi: 10.6064/2012/963401
187. Poudel M, Mendes R, Costa LA, Bueno CG, Meng Y, Folimonova SY, et al. The role of plant-associated bacteria, fungi, and viruses in drought stress mitigation. Front Microbiol (2021) 3058. doi: 10.3389/fmicb.2021.743512
188. Souza RD, Ambrosini A, Passaglia LM. Plant growth-promoting bacteria as inoculants in agricultural soils. Genet Mol Biol (2015) 38:401–19. doi: 10.1590/S1415-475738420150053
189. Pandey PK, Singh MC, Singh SS, Kumar AK, Pathak MM, Shakywar RC, et al. Inside the plants: endophytic bacteria and their functional attributes for plant growth promotion. Int J Curr Microbiol Appl Sci (2017) 6(2):11–21. doi: 10.20546/ijcmas.2017.602.002
190. Santos AFJ, Morais JS, Miranda JS, Moreira ZPM, Feitoza AFA, Leite J, et al. Cacti-associated rhizobacteria from Brazilian caatinga biome induce maize growth promotion and alleviate abiotic stress. Rev Bras Cienc Agrar (2020) 15:e8221. doi: 10.5039/agraria.v15i3a8221
191. Lopes CM, Silva AMM, Estrada-Bonilla GA, Ferraz-Almeida R, Vieira JLV, Otto R, et al. Improving the fertilizer value of sugarcane wastes through phosphate rock amendment and phosphate-solubilizing bacteria inoculation. J Clean Prod (2021) 298:126821. doi: 10.1016/j.jclepro.2021.126821
192. Salvagiotti F, Cassman KG, Specht JE, Walters DT, Weiss A, Dobermann A. Nitrogen uptake, fixation and response to fertilizer n in soybeans: a review. Field Crops Res (2008) 108:1–13. doi: 10.1016/j.fcr.2008.03.001
193. Castro JRP. Inoculantes. Brasil: Outlook Globalfert (2020). Available at: http://www.anpii.org.br/wp-content/uploads/2020/06/Global-Fert-Inoculantes.pdf.
194. Hungria M, Campo RJ, Souza EM, Pedrosa FO. Inoculation with selected strains of azospirillum brasilense and a. lipoferum improves yields of maize and wheat in Brazil. Plant Soil (2010) 331:413–25. doi: 10.1007/s11104-009-0262-0
195. Marks BB, Megías M, Ollero FJ, Nogueira MA, Araujo RS, Hungria M. Maize growth promotion by inoculation with azospirillum brasilense and metabolites of rhizobium tropici enriched on lipo-chitooligosaccharides (LCOs). AMB Expr (2015) 5:71. doi: 10.1186/s13568-015-0154-z
196. Santos MS, Nogueira MA, Hungria M. Outstanding impact of azospirillum brasilense strains ab-V5 and ab-V6 on the Brazilian agriculture: lessons that farmers are receptive to adopt new microbial inoculants. Rev Bras Cienc Solo (2021) 45:e0200128. doi: 10.36783/18069657rbcs20200128
Keywords: soil quality, sustainability, soil microbes, bio-input, organic matter management
Citation: Silva AMM, Araújo VLVP and Cardoso EJBN (2023) Revisiting the past to understand the present and future of soil health in Brazil. Front. Soil Sci. 3:1172436. doi: 10.3389/fsoil.2023.1172436
Received: 23 February 2023; Accepted: 02 May 2023;
Published: 17 May 2023.
Edited by:
Jay Prakash Verma, Banaras Hindu University, IndiaReviewed by:
Vishal Tripathi, Graphic Era University, IndiaAdemir Araujo, Federal University of Piauí, Brazil
Copyright © 2023 Silva, Araújo and Cardoso. This is an open-access article distributed under the terms of the Creative Commons Attribution License (CC BY). The use, distribution or reproduction in other forums is permitted, provided the original author(s) and the copyright owner(s) are credited and that the original publication in this journal is cited, in accordance with accepted academic practice. No use, distribution or reproduction is permitted which does not comply with these terms.
*Correspondence: Antonio Marcos Miranda Silva, YW50b25pb21hcmNvc0B1c3AuYnI=